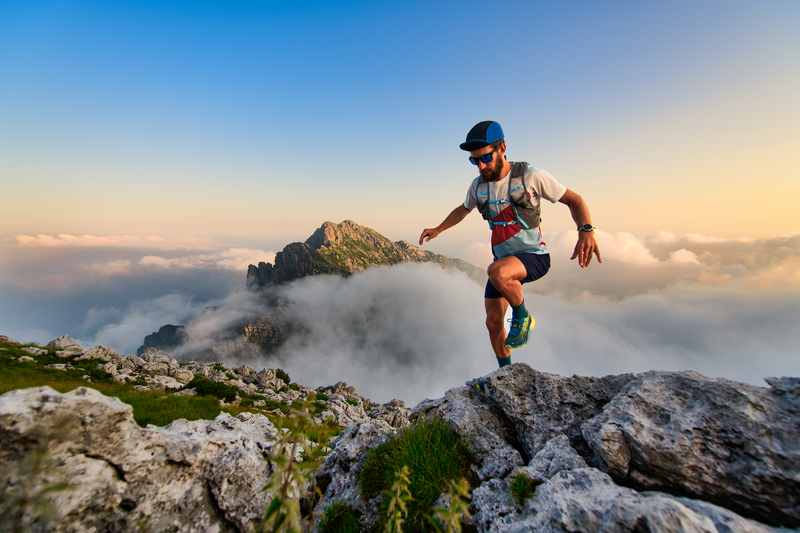
95% of researchers rate our articles as excellent or good
Learn more about the work of our research integrity team to safeguard the quality of each article we publish.
Find out more
ORIGINAL RESEARCH article
Front. Microbiol. , 17 January 2020
Sec. Microbial Physiology and Metabolism
Volume 10 - 2019 | https://doi.org/10.3389/fmicb.2019.03027
D-galacturonate, a key constituent of pectin, is a ubiquitous monomer in plant biomass. Anaerobic, fermentative conversion of D-galacturonate is therefore relevant in natural environments as well as in microbial processes for microbial conversion of pectin-containing agricultural residues. In currently known microorganisms that anaerobically ferment D-galacturonate, its catabolism occurs via the galacturonate-isomerase pathway. Redox-cofactor balancing in this pathway strongly constrains the possible range of products generated from anaerobic D-galacturonate fermentation, resulting in acetate as the predominant organic fermentation product. To explore metabolic diversity of microbial D-galacturonate fermentation, anaerobic enrichment cultures were performed at pH 4. Anaerobic batch and chemostat cultures of a dominant Lactobacillus suebicus strain isolated from these enrichment cultures produced near-equimolar amounts of lactate and acetate from D-galacturonate. A combination of whole-genome sequence analysis, quantitative proteomics, enzyme activity assays in cell extracts, and in vitro product identification demonstrated that D-galacturonate metabolism in L. suebicus occurs via a novel pathway. In this pathway, mannonate generated by the initial reactions of the canonical isomerase pathway is converted to 6-phosphogluconate by two novel biochemical reactions, catalyzed by a mannonate kinase and a 6-phosphomannonate 2-epimerase. Further catabolism of 6-phosphogluconate then proceeds via known reactions of the phosphoketolase pathway. In contrast to the classical isomerase pathway for D-galacturonate catabolism, the novel pathway enables redox-cofactor-neutral conversion of D-galacturonate to ribulose-5-phosphate. While further research is required to identify the structural genes encoding the key enzymes for the novel pathway, its redox-cofactor coupling is highly interesting for metabolic engineering of microbial cell factories for conversion of pectin-containing feedstocks into added-value fermentation products such as ethanol or lactate. This study illustrates the potential of microbial enrichment cultivation to identify novel pathways for the conversion of environmentally and industrially relevant compounds.
Pectin, a commonly occurring polymer in plants, has a linear backbone of D-galacturonate residues or, in the case of rhamnogalacturonan, of alternating D-galacturonate and rhamnose residues. These backbones are decorated with sugars and carbonyl groups of D-galacturonate can be extensively esterified with methyl and acetyl-groups (Khanh et al., 1991; Searle-van Leeuwen et al., 1996). D-galacturonate accounts for approximately 70% of the mass of pectin (Mohnen, 2008). During microbial pectin degradation, it is released by the concerted action of pectinases and accessory enzymes (de Vries and Visser, 2001; Mohnen, 2008; Biz et al., 2014). Knowledge on the pathways involved in microbial fermentation of D-galacturonate is relevant for understanding degradation of plant biomass in anaerobic natural environments and in waste-water treatment processes. It is also highly relevant for the development of anaerobic microbial processes that can convert large-volume, pectin-rich agricultural residues such as apple pomace, citrus peel, and sugar-beet pulp into added-value products (Edwards and Doran-Peterson, 2012; Food and Agriculture Organization of the United Nations, 2016; Marzo et al., 2019).
Of three experimentally demonstrated pathways for D-galacturonate metabolism, two are found in prokaryotes and one in fungi. The prokaryotic oxidative pathway, first demonstrated in a Pseudomonas species (Zajic, 1959), converts D-galacturonate to α-ketoglutarate and CO2 via reactions that together reduce 2 moles of NAD(P)+ to NAD(P)H per mole of D-galacturonate (Zajic, 1959; Chang and Feingold, 1970). In contrast, the reaction sequence that converts D-galacturonate to pyruvate and glycerol in the fungal pathway requires the investment of 2 NAD(P)H per mole of D-galacturonate (Kuorelahti et al., 2005; Martens-Uzunova and Schaap, 2008; Zhang et al., 2011). Neither of these two routes enable redox-cofactor-neutral, fermentative pathways that generate ATP via substrate-level phosphorylation and they have hitherto only been encountered in microorganisms that are able to respire.
Fermentative, anaerobic metabolism of D-galacturonate is firmly associated with a third pathway. First described in Escherichia coli (Kovachevich and Wood, 1955; Ashwell et al., 1960; Cynkin and Ashwell, 1960; Hickman and Ashwell, 1960; Smiley and Ashwell, 1960), this adapted Entner–Doudoroff or isomerase pathway converts D-galacturonate into pyruvate and glyceraldehyde-3-phosphate via 2-keto-3-deoxy-phosphogluconate (KDPG), the characteristic intermediate of the Entner–Doudoroff pathway for sugar dissimilation (Peekhaus and Conway, 1998). The canonical isomerase pathway (Figure 1) involves the activity via uronate isomerase (UxaC, EC 5.3.1.12), tagaturonate reductase (UxaB, EC 1.1.1.58), altronate dehydratase (UxaA, EC 4.2.1.7), and 2-keto-3-deoxy-gluconate kinase (KdgK, EC 2.7.1.45) and 2-keto-3-deoxy-phosphogluconate aldolase (KdgA, EC 4.1.2.14). Alternatively, conversion of tagaturonate into 2-keto-3-deoxy-gluconate can be catalyzed by tagaturonate 3-epimerase (UxuE, EC 5.1.2.7), fructuronate reductase (UxuB, EC 1.1.1.57), and mannonate dehydratase (UxuA, EC 4.2.1.8) (Kovachevich and Wood, 1955; Ashwell et al., 1960; Cynkin and Ashwell, 1960; Hickman and Ashwell, 1960; Smiley and Ashwell, 1960).
Figure 1. The canonical isomerase pathway for D-galacturonate fermentation. Dashed lines represent multiple conversions. Abbreviations indicate the following metabolites and enzyme activities: galUA, galacturonate; tagA, tagaturonate; fruA, fructuronate; mannA, mannonate; KDG, keto-deoxygluconate; KDGP, keto-deoxy-phosphogluconate; GAP, glyceraldehyde-3-phosphate; ac-CoA, acetyl-CoA; ac-P, acetyl-phosphate; pyv, pyruvate; lac, lactate; ac, acetate; UxaC, uronate isomerase; UxuE, tagaturonate 3-epimerase; UxuB, fructuronate reductase; UxuA, mannonate hydratase; KdgK, keto-deoxy-gluconate kinase; KdgA, keto-deoxy-phosphogluconate aldolase; PDH, pyruvate dehydrogenase; PTA, phosphotransacetylase; AckA, acetate kinase; nLDH, D-/L-lactate dehydrogenase.
In both variants of the isomerase pathway, conversion of D-galacturonate into pyruvate and glyceraldehyde-3-phosphate requires the input of 1 ATP and 1 NAD(P)H. Further conversion of glyceraldehyde-3-phosphate via the lower part of the Embden–Meyerhof glycolysis yields one NADH and two ATP. Use of the isomerase pathway therefore enables redox-cofactor-neutral conversion of D-galacturonate into two moles of pyruvate, with a net ATP yield of 1 mol (mol galacturonate)–1 (Grohmann et al., 1994, 1998; Doran et al., 2000). This redox-cofactor neutrality constrains the range of fermentation products that can be generated from D-galacturonate. Acetate, which can be formed from pyruvate via redox-cofactor-neutral, ATP-yielding reactions, is typically found as the main product of microbial D-galacturonate fermentation (Grohmann et al., 1994; Doran et al., 2000; Valk et al., 2018; Kuivanen et al., 2019). For example, in a recent enrichment study on galacturonate performed at pH 8.0, the dominant organism “Candidatus Galacturonibacter soehngenii” predominantly produced acetate by a combination of galacturonate fermentation and acetogenesis (Valk et al., 2018). In bacteria engineered for ethanol production from D-galacturonate via the isomerase pathway, large amounts of more oxidized by-products are formed (Grohmann et al., 1994, 1998; Doran et al., 2000).
As yet undiscovered pathways for D-galacturonate fermentation, that allow for different fermentation product profiles, may exist in nature. Chemical decarboxylation of D-galacturonate to L-arabinose has been reported to occur under relatively mild conditions (Ruff, 1898; McKinnis, 1928; Link and Niemann, 1930) and the possibility that a similar enzyme-catalyzed reaction might occur has been proposed (Hirst, 1942; Gulland, 1943). Decarboxylation of L-arabinose could enable high-yield microbial production of compounds such as ethanol, lactate, or isobutanol. However, no experimental evidence for existence of the required D-galacturonate decarboxylase has yet been found in nature (Fan and Feingold, 1972; Marounek and Dušková, 1999).
Based on comparative genome analysis, Rodionova et al. (2012) suggested an alternative pathway for D-galacturonate metabolism involving epimerization of mannonate to gluconate. Subsequent conversion of gluconate by gluconokinase and 6-phosphogluconate dehydrogenase could also connect of D-galacturonate catabolism to pentose-phosphate metabolism. However, no experimental proof is available for in vivo activity of this pathway or for in vitro activity of the proposed mannonate epimerase.
Enrichment cultivation remains a powerful tool to select micro-organisms that are optimally adapted to individual and combined sets of environmental parameters from natural or industrial environments. Even when grown on a single substrate, population composition and product profiles in such cultures can be strongly influenced by process parameters such as culture pH, biomass retention time, temperature, and dynamic substrate-feeding strategies (Zoetemeyer et al., 1982a; Kleerebezem and van Loosdrecht, 2007; Johnson et al., 2009). It is well established that, in many fermentative microorganisms, cultivation at low pH induces a transition from the production of acids such as acetic or butyric acid to the formation of non-acidic products such as ethanol or butanol (Bahl et al., 1982; Grimmler et al., 2011). Similarly, enrichment cultivation at pH values below the pKa of actic acid (4.76) has been found to select against acetic acid producing microorganisms, presumably due to an uncoupling of the pH gradient across the cytoplastic membrane by the undissociated acid (Eklund, 1983; Salmond et al., 1984).
This study explores whether anaerobic enrichment cultivation at pH 4.0 can lead to the discovery of novel pathways for D-galacturonate fermentation. To this end, anaerobic D-galacturonate-limited enrichment cultivation was performed in shake flasks, after which the dominant organism was isolated. Its product profile during anaerobic growth on D-galacturonate, which differed from that in previously described D-galacturonate fermenting bacteria, was characterized in anaerobic bioreactor batch and chemostat cultures and a combination of genomics, proteomics, and in vitro enzyme-activity analyses was used to investigate the responsible metabolic pathway.
Micro-organisms capable of fermenting D-galacturonate at low pH were enriched by serial transfer in anaerobic shake-flask cultures, grown at pH 4.0 on 4 g L–1 D-galacturonate. The initial cultures were inoculated with a mixture of rotting orange peels, orange-peel-enriched compost (Voragen et al., 2009). After two transfers to fresh medium, 16S-rRNA gene amplicon sequencing was performed. Based on these short 250-nucleotide amplicon sequences, the dominant taxon was the genus Lactobacillus (SINA database 1.2.11, Pruesse et al., 2012), with no other taxa represented by over 1% of the reads (Supplementary Figure S1). A pure culture was obtained by repeated anaerobic plating on galacturonate medium. The isolate grew anaerobically on D-galacturonate, L-arabinose, gluconate, and D-glucuronate in synthetic media supplemented with 0.4 g L–1 yeast extract (Supplementary Table S1).
Whole-genome long-read sequencing of the isolate, augmented with short-read sequencing, enabled assembly into one large (2.7 Mbp) and two smaller (89 and 28 kbp) contigs (Table 1). The full 16S-RNA gene sequence derived from the assembled genome sequence showed 100% identity with that of Lactobacillus suebicus (SINA database 1.2.11, Pruesse et al., 2012), a hetero-fermentative lactic acid bacterium previously isolated from apple and pear mashes (Kleynmans et al., 1989). Analysis with CheckM (Parks et al., 2015) yielded an estimated completeness of the genome sequence of 99.5%, with a GC content of 39.1% and 2811 predicted coding sequences (Table 1). These results are similar to previously published data by Nam et al. (2011) on the genome of L. suebicus DSM 5007 shown in Supplementary Table S2.
Table 1. Statistical data for the assembled and annotated genome sequence of Lactobacillus suebicus LCV1.
The fermentation product profile of D-galacturonate-grown L. suebicus LCV1 was investigated in anaerobic bioreactor batch and chemostat cultures. In anaerobic bioreactor batch cultures, L. suebicus LCV1 exhibited a specific growth rate on D-galacturonate of 0.20 h–1 while acetate and lactate, the sole organic fermentation products, were formed at near-equimolar amounts (Figure 2). A similar product stoichiometry was observed in anaerobic, D-galacturonate-limited chemostat cultures grown at a dilution rate of 0.13 h–1, in which biomass-specific production rates of acetate and lactate were 6.0 ± 0.1 and 5.2 ± 0.1 mmol gx–1 h–1, respectively (Table 2). The biomass yield on D-galacturonate in these anaerobic chemostat cultures was 0.09 ± 0.0 g biomass (g galacturonate) –1.
Figure 2. Anaerobic growth and product formation of in an anaerobic bioreactor batch culture of L. suebicus LCV1 on D-galacturonate (3.3 g L–1) at pH 4 and at 30°C. Symbols: ● D-galacturonate, ■ acetate, □ lactate, and ° optical density. The data shows one of two independent replicates; data from the second experiment are shown in Supplementary Figure S2.
Table 2. Physiological parameters of anaerobic, galacturonate-grown chemostat cultures of Lactobacillus suebicus LCV1.
In the canonical isomerase pathway for D-galacturonate fermentation, this substrate is generally first converted into two molecules of pyruvate via the isomerase pathway (Figure 1). Since this conversion is redox-cofactor neutral, it could only account for formation of equimolar amounts of acetate and lactate if pyruvate reduction to lactate by a lactate dehydrogenase (LDH) were stoichiometrically coupled to oxidative decarboxylation of pyruvate to acetate. The latter could, theoretically, be accomplished by the combined action of a pyruvate-dehydrogenase complex, phosphotransacetylase, and acetate kinase (Figure 1).
The genome sequence of L. suebicus LCV1 showed a full complement of structural genes for the key enzymes of the isomerase pathway (Figure 3 and Table 3). Proteome analysis of D-galacturonate-grown cultures showed high levels of the enzymes of upper part of the adapted ED pathway [uronate isomerase (UxaC, EC 5.3.1.12), tagaturonate 3-epimerase (UxaE, EC 5.1.2.7) and fructuronate reductase (UxuB, EC 1.1.1.57)] (Table 3 and Figure 3). However, under the experimental conditions, mannonate dehydratase (UxuA, EC 4.2.1.8), 2-dehydro-3-deoxygluconokinase (KdgK, EC 2.7.1.45), and 2-dehydro-3-deoxyphosphogluconate aldolase (KdgA, EC 4.1.2.14), three key enzymes in the lower part of the isomerase pathway, were not detected in the proteome (Table 3).
Figure 3. Identified proteins from the isomerase pathway (adapt. EDP), phosphoketolasse pathway (PKP) and acid fermentation plotted as normalized spectral counts in LC-MS-based proteomic analysis. The inset shows the box plot from all identified proteins within a range of 0–250 normalized counts. Only three protein hits were above this range, which are not shown. All proteins detected from the isomerase pathway, phosphoketolase pathway, and acid fermentation were above the 75th percentile of the complete proteome dataset. Bars represent the average value of two biological reactor duplicates, and error bars show the standard deviation between runs. ∗Paralogous genes.
Table 3. Annotated genes identified in the genome of L. suebicus LCV1, and associated expressed gene products identified by shot-gun proteomics for the two putative routes the isomerase pathway and the phosphoketolase pathway.
The three subunits of the pyruvate-dehydrogenase complex (PDH, EC 1.2.4.1; EC 2.3.1.12; EC 1.8.1.4), as well as phosphate transacetylase (PTA, EC 2.3.1.8), acetate kinase (AckA, EC 2.7.2.1), and both L- and D-LDH [L(+)-nLDH, EC 1.1.1.27; D-(-)-nLDH EC 1.1.1.28] were all detected in the proteome (Figure 3 and Table 3). These results indicated that, under the experimental conditions, enzymes for conversion of pyruvate to lactate and acetate were present in L. suebicus LCV1. However, absence of UxuA, KdgK, and KdgA in the proteome indicated that, in the anaerobic galacturonate-grown batch cultures, pyruvate formation from galacturonate did not occur via a complete, canonical isomerase pathway.
The phosphoketolase (PK) pathway in lactic acid bacteria is a well-known route for hetero-fermentative dissimilation of sugars to equimolar amounts of lactate, ethanol, and CO2 (DeMoss et al., 1951; Gunsalus and Gibbs, 1952; Gibbs et al., 1955; Busse et al., 1961). It has previously been proposed that D-galacturonate metabolism via the initial reactions of the isomerase pathway can be linked to the PK pathway by epimerization of mannonate to gluconate (Rodionova et al., 2012), although this mechanism has not been experimentally demonstrated. Consistent with the involvement of such hybrid pathway, proteome analysis of galacturonate-grown cultures of L. suebicus LCV1 showed high levels of a gluconate kinase (GntK, EC 2.7.1.12), 6-phosphogluconate dehydrogenase (GndA, EC 1.1.1.44), and phosphoketolase (XpkA, EC 4.1.2.9), three key enzymes of the PK pathway (Table 3 and Figure 3).
In vitro activity assays were used to investigate the presence of known enzyme reactions involved in the proposed hybrid isomerase-PK pathway in galacturonate-grown L. suebicus LCV1. Under aerobic conditions, cell extracts rapidly oxidized NADH (Table 4). This activity was attributed to an NADH oxidase (NoxA, EC 1.6.3.4), for which a candidate gene (A0A0R1WBZ1, Table 3) and high expression levels of the encoded protein (Figure 3) were detected. Enzyme activity assays that involved NAD+ or NADH were therefore performed under anaerobic conditions. Fructuronate reductase (UxuB, EC 1.1.1.57), a key enzyme of the upper part of the isomerase pathway, was measured with D-tagaturonate and NADH or NADPH as substrates and showed an activity of 0.99 ± 0.01 and 0.2 ± 0.01 μmol (mg protein)–1 min–1, respectively. Cell extracts also showed high activities of NADP+-dependent 6-phosphogluconate dehydrogenase (GndA, EC 1.1.1.44; Table 4), the first enzyme of the PK pathway. Activities of NAD+-dependent LDH [L(+)-nLDH, EC 1.1.1.27; D-(-)-nLDH EC 1.1.1.28] in cell extracts of D-galacturonate-grown L. suebicus LCV1 (Table 4) were similar to those observed in cell extracts of sugar-grown cultures of other Lactobacilli (de Vries et al., 1970; Thomas et al., 1979). Pyruvate-dehydrogenase activity in cell extracts was below detection limit (Table 4).
Table 4. Specific enzyme activities of key enzymes of the isomerase and phosphoketolase pathways of cell extract of galacturonate-grown L. suebicus LCV1 (D = 0.13 h–1, T = 30°C, and pH 4).
Conversion of mannonate to 6-phosphogluconate, a key conversion in the proposed hybrid isomerase-PK pathway, has not previously been demonstrated. To investigate whether this conversion occurs in L. suebicus LCV1, cell extracts were incubated with different substrates and products were analyzed by GC-MS. Incubation (0.5 h) of cell extracts with mannonate did not lead to formation of gluconate (Figure 4A, black line). When ATP was also added, mannonate was predominantly converted to 6-phosphomannonate, thus indicating the activity of a mannonate kinase in the cell extracts. In addition, small amounts of 6-phosphogluconate were formed (Figure 4A, blue line). Upon prolonged incubation of cells extract with mannonate and ATP, formation of 6-phosphomannonate was clearly observed (Figure 4B, red line). In addition, incubation of cell extracts with 6-phosphogluconate led to the formation of 6-phosphomannonate (Figure 4C, black line). These results demonstrated the presence, in galacturonate-grown L. suebicus, of 6-phosphomannonate 2-epimerase activity (for reaction, see Supplementary Figure S6).
Figure 4. Identification of products from the conversion of mannonate or 6-phosphogluconate, in the presence and absence of ATP, with cell extract from L. suebicus LCV1, grown on galacturonate in anaerobic chemostat cultures (D = 0.13 h–1, 30°C, pH 4). (A) 0.5 h incubation of mannonate with cell extract in the absence (black line) and presence of ATP (blue line), (B) incubation of mannonate with cell extract and ATP, start of incubation (black line), and sample taken after 3 h of incubation (red line), and (C) incubation of 6-phosphogluconate, start of incubation (green line), and sample taken after 3 h of incubation (black line). For each experiment, one of two of independent biological duplicate experiments is shown. Data for the duplicate experiments are shown in Supplementary Figure S3. Different retention times of compounds in the panels are caused by a reduced column length for the experiments shown in C. Retention times of the standards (mannonate and 6-phosphogluconate) and the annotated GC-MS profile of mannonate are shown in Supplementary Figures S4, S5, respectively. ∗sugar acid; #lacton-variant of the sugar acid.
Continuous enzyme-activity assays with cell extracts, in which activity of gluconate kinase was coupled to 6-phosphogluconate dehydrogenase, revealed a KM for gluconate of 10 mM and a Vmax of 0.24 μmol (mg protein)–1 min–1 (Figure 5A). This KM was two to three orders of magnitude higher than reported for bacterial gluconate kinases (Zachariou and Scopes, 1985; Izu et al., 1996; Tong et al., 1996). The same continuous assay, using the native, unidentified 6-phosphomannonate epimerase activity as a coupling enzyme, was used to investigate the kinetics of mannonate kinase in L. suebicus LCV1. Although this assay did not contain the demonstrated excess of coupling-enzyme activity required for reliable kinetic analyses of mannonate kinase activity, it indicated the presence of a high-affinity mannonate kinase activity (Figure 5B, estimated KM of 0.4 mM). The observed maximum reaction rate and apparent substrate inhibition at higher substrate concentrations could reflect properties of either the mannonate kinase or of the unidentified epimerase enzyme(s) under the conditions of the in vitro assay.
Figure 5. Effect of (A) gluconate (●, mM) and (B) mannonate concentrations (■, mM) on the specific enzymatic activity (μmol mgprotein–1 min–1) of gluconate kinase and mannonate kinase, respectively, in cell extracts of L. suebicus LCV1, pre-grown on galacturonate in anaerobic chemostat cultures (D = 0.13 h–1, 30°C, pH 4). The average ± mean deviations were derived from duplicate measurements.
Enrichment cultivation at low pH enabled the isolation of a lactic acid bacterium with a product profile that had not previously been observed during anaerobic fermentation of D-galacturonate. In contrast to previously described D-galacturonate-fermenting organisms, the isolate, identified as a strain of L. suebicus, did not produce acetate as single major fermentation product but, instead, produced lactate and acetate at near-equimolar ratios. Due to its lower pKa and lower lipid solubility, the uncoupling effect of lactate on the proton gradient across biological membranes is much less pronounced than that of acetic acid (Leyer et al., 1995; Abbott et al., 2008). Our results are therefore in line with previous studies in which enrichment cultivation at low pH values generated a negative selective pressure against the formation of acetate as predominant fermentation product (Zoetemeyer et al., 1982b; Fang and Liu, 2002; Horiuchi et al., 2002; Temudo et al., 2007). At low extracellular concentrations of lactate, some anaerobic bacteria can couple lactate export via a proton symporter to energy conservation (Ten Brink and Konings, 1986; Trchounian and Trchounian, 2019). If active in L. suebicus, such an “end-product-efflux” mechanism might provide an additional, condition-dependent advantage over formation of only acetate.
Lactobacilli are known for their ability to ferment a wide range of sugars under mildly acidic conditions (van Hylckama Vlieg et al., 2006; Siezen et al., 2011). However, metabolic pathways for D-galacturonate metabolism and the resulting product profiles in Lactobacilli have not previously been studied in detail. In the context of the present study, it is relevant to note that apple and pear mashes, from which L. suebicus has previously been isolated, are rich in pectin and that, during growth on sugars, the metabolism of L. suebicus was characterized as heterofermentative (Kleynmans et al., 1989).
We initially hypothesized that the observed product stoichiometry reflected a simultaneous, redox-cofactor-balanced reduction and oxidative decarboxylation of pyruvate, generated via a canonical isomerase pathway for D-galacturonate metabolism (Figure 1; Wagner et al., 2005; Valk et al., 2018). Although pyruvate dehydrogenase (PDH) complexes are typically inhibited at high NADH/NAD+ ratios (Snoep et al., 1992, 1993), such a concerted action of the PDH complex and an NAD+-linked LDH has been implicated in fermentation of pyruvate by Oenococcus oeni and Leucococcus mesenteroides (Hansen and Henning, 1966; Wagner et al., 2005). However, its involvement inD-galacturonate fermentation by L. suebicus was rejected based on the absence of key enzymes of the lower half of the canonical isomerase pathway in the proteome of galacturonate-grown cultures (Table 3 and Figure 3).
Based on investigations on Thermotoga maritima, a deep-branching, anaerobic, hyperthermophilic bacterium able to degrade a wide range of carbohydrates including pectin (Nelson et al., 1999), Rodionova et al. (2012) proposed that the initial reactions of the isomerase pathway for galacturonate might be coupled to the PK pathway (Figure 6A) by gluconate 2-epimerase (gntE), and a gluconate kinase (gntK, EC 2.1.7.12). This proposal was based on the organization of hexuronate and pectin utilization loci, in which gntE, which was assumed to encode the epimerase activity, was found between the fructuronate reductase (uxuA, EC 1.1.1.58) and gluconate kinase genes. A homology search indicated that gntE homologs also occurred in other bacterial genera, including Lactobacillus, Acidobacterium, and Terriglobus (Rodionova et al., 2012). However, no experimental evidence was provided to show that gntE indeed encoded the proposed epimerase.
Figure 6. Metabolic pathways contributing to fermentative galacturonate fermentation in L. suebicus LCV1 as discussed in this paper. (A) Phosphoketolase pathway and (B) novel pathway proposed in this study that combines known reactions of the upper part of the canonical isomerase pathway and the phosphoketolase pathway, coupled by the concerted action of a mannonate kinase and a 6-phosphomannonate 2-epimerase. Dashed lines represent multiple conversions. Abbreviations indicate the following metabolites and enzyme activities: galUA, galacturonate; tagA, tagaturonate; fruA, fructuronate; mannA, mannonate; mann-6P, 6-phosphomannonate; gln-6P, 6-phosphogluconate; rib-5P, ribulose-5-phosphate; xyl-5P, xylulose-5-phosphate; GAP, glyceraldehyde-3-phosphate; ac-P, acetyl-phosphate; pyv, pyruvate; lac, lactate; ac, acetate; UxaC, uronate isomerase; UxuE, tagaturonate 3-epimerase; UxuB, fructuronate reductase, 1, mannonate kinase; 2, 6-phosphomannonate 2-epimerase; 6PGD, 6-phosphogluconate dehydrogenase (decarboxylating); AraD, ribulose-5-phosphate 4-epimerase; XpkA, phosphoketolase; AckA, acetate kinase; nLDH, D-/L-lactate dehydrogenase.
Discontinuous and continuous enzyme activity assays and product identification by GC-MS showed that, instead of a direct epimerization of mannonate to gluconate followed by phosphorylation of gluconate (Rodionova et al., 2012), L. suebicus LCV1 first phosphorylated mannonate to 6-phosphomannonate, followed by an epimerization to 6-phosphogluconate (Figure 6B). This newly discovered conversion, which couples the isomerase pathway to the PK pathway, involves two enzyme activities, a mannonate kinase and a 6-phosphomannonate/6-phoshogluconate 2-epimerase, that have not previously been described.
The BRENDA database (Schomburg et al., 2017; Jeske et al., 2019) does not list specific mannonate kinases, nor does it list mannonate as a known substrate for gluconate kinases. Indeed, E. coli gluconate kinase (GntK, EC 2.7.1.12) was reported to be unable to use D-mannonate as substrate (Cohen, 1950). The high KM of gluconate kinase activity and the low apparent KM of mannonate kinase in cell extracts (Figure 4) strongly suggest that at least one of the two L. suebicus genes with strong homology to gluconate kinase genes (A0A0R1W2R7, A0A0R1WAU7), whose products were both detected in the proteome of galacturonate-grown cells (Table 3 and Figure 3), encodes a kinase with a much higher affinity for mannonate than for gluconate.
Four Structural Classification of Proteins (SCOP) families harbor 2-epimerases; NAD+-dependent epimerases or dehydratases (CEP1), N-acylglucosamine epimerases (CEP4), NanE-like (CEP5), and UDP-N-acetylglucosamine 2-epimerases (CEP9). All these epimerases act on compounds with a CDP or UDP side-group or on dimers (Senoura et al., 2009; Van Overtveldt et al., 2015). No homology to structural genes for either of the enzyme groups was identified in the L. suebicus LCV1 genome. Based on co-localization with hexuronate and D-gluconate fermentation pathway genes, Rodionova et al. (2012) proposed that the TM0042 gene in T. maritima encoded a gluconate-mannonate 2-epimerase. The predicted protein sequence showed similarity to an aldose 1-epimerase (EC 5.1.3.3) or a 6-phosphoglucose 1-epimerase (EC 5.1.3.15), but no biochemical evidence for its activity was provided. A homologous gene was identified in L. suebicus LCV1 (A0A0R1W1H9) and although the gene was not co-localized with genes of the D-galacturonate fermentation pathway, the encoded protein product was successfully identified with high sequence coverage in the D-galacturonate-grown cultures. Further research is required to investigate whether this protein encodes the 6-phosphomannonate 2-epimerase active in these cultures.
Consistent with the literature on other organisms (Cohen, 1950; McNair Scott and Cohen, 1952, 1956), activity of 6-phosphogluconate dehydrogenases (GndA, EC 1.1.1.44) in cell extracts of L. suebicus showed high activities NADP+ as electron acceptor. While fructuronate reductases typically use NADH as co-factor (Hickman and Ashwell, 1960; Portalier and Stoeber, 1972; Mandrand-Berthelot and Lagarde, 1977; Linster and van Schaftingen, 2004), cell extracts of L. suebicus LCV1 also showed activity of this enzyme with NADPH as electron donor (Table 3). A matching cofactor use off these two enzymes, possibly further facilitated by the expression of a transhydrogenase (PntAB; EC 1.6.1.2, A0A0R1W642; A0A0R1WE53; A0A0R1W6T3), enables redox-cofactor balancing in the integrated isomerase-PK pathway (Figure 6B).
Enzyme purification and characterization, possibly combined with heterologous expression studies and/or generation and analysis of L. suebicus mutants, should resolve the genetic basis for the mannonate kinase and 6-phosphomannonate/6-phosphogluconate-2-epimerases in L. suebicus LCV1. In addition to generating fundamental knowledge on anaerobic metabolism in natural environments of galacturonate, a ubiquitous monomer in plant biomass, such studies are likely to have a considerable industrial relevance. In contrast to the canonical isomerase pathway (van Maris et al., 2006), the integrated isomerase-PK pathway demonstrated in this study enables redox-cofactor neutral conversion of D-galacturonate to a ribulose-5-phosphate and CO2 (Figure 6B). Linking these reactions to the non-oxidative pentose-phosphate pathway would pave the way for new metabolic engineering strategies for high-yield, anaerobic conversion of galacturonate-containing feedstocks to compounds such as ethanol, isobutanol, or lactate.
Unless stated otherwise, liquid medium contained (g L–1): D-galacturonate 4.3; NH4Cl 1.34; KH2PO4 0.78; Na2SO4.10H2O 0.130; MgCl2.6H2O 0.120; FeSO4. 7H2O 0.0031; CaCl2 0.0006; H3BO4 0.0001; Na2MoO4. 2H2O 0.0001; ZnSO4.7H2O 0.0032; CoCl2.H2O 0.0006; CuCl2.2H2O 0.0022; MnCl2.4H2O 0.0025; NiCl2.6H2O 0.0005; EDTA 0.10, tryptone (BD Bacto Difco, ThermoFisher Scientific, United States) 0.6, yeast extract (BD Bacto Difco, ThermoFisher Scientific, United States) 0.4, and vitamin solution (Verduyn et al., 1992) 1 mL L–1; 19 L of mineral solution with yeast extract (concentration adjusted to the final volume, 20 L) was autoclaved for 20 min at 121°C. Subsequently, 86 g D-galacturonate, 8 g tryptone, and 20 mL vitamin solution in 1 L demineralized water was filter sterilized (0.2 μm Mediakap Plus, Spectrum Laboratories, Rancho Dominguez, United States) and added to the autoclaved medium. For bioreactor cultivation, 0.075 mL L–1 Pluronic PE 6100 antifoam (BASF, Ludwigshafen, Germany) was added to the mineral medium before autoclaving.
Anaerobic 50-mL shake flasks containing 30 mL liquid medium were inoculated with 2% (v/v) of a mixed inoculum consisting of rumen content of a grass-fed cow, provided by an artisanal butcher (Slager Jonkers, Est, Netherlands), rotting orange peels and orange-peel-enriched compost. Triplicate shake flask cultures were grown at 30°C in an anaerobic chamber (Bactron III, Shell Lab, Cornelius, NC, United States, gas composition 89% N2, 6% CO2, and 5% H2) and transferred once, upon depletion of the carbon source. After the second enrichment phase, the enrichment cultures were plated on 1% agar plates (4 g L–1 D-galacturonate, 0.4 g L–1 yeast extract, pH 5) under anaerobic conditions. Single colonies were re-streaked thrice and grown in shake flasks on liquid medium. Stocks were stored in 30% (v/v) glycerol at −80°C.
Batch and chemostat cultures were grown in 1.2 L laboratory bioreactors (Applikon, Delft, Netherlands) at a temperature of 30°C. Reactors were stirred at 300 r/min and, to maintain anaerobiosis, sparged with nitrogen gas at a flow rate of 120 mL min–1. Culture pH was controlled at pH 4.0 ± 0.1 by automatic titration (ADI 1030 Biocontroller, Applikon, Delft, Netherlands) with 1 M NaOH. For chemostat cultivation, the dilution rate was set at 0.13 ± 0.01 h–1 and a working volume of 0.50 L was maintained by a peristaltic effluent pump (Masterflex, Cole-Parmer, Vernon Hills, IL, United States) coupled to a level sensor.
Cell-free supernatants were obtained by centrifugation (Heraeus Pico Microfuge, ThermoFisher Scientific, Waltham, NC, United States) of culture samples. Concentrations of galacturonate and extracellular metabolites were analyzed on an Agilent 1100 Affinity HPLC (Agilent Technologies, Santa Clara, CA, United States) equipped with an Aminex HPX-87H ion-exchange column (BioRad, Hercules, CA, United States), operated at 60°C with a mobile phase of 5 mM H2SO4 and at a flow rate of 0.6 mL min–1. Concentrations of CO2 and O2 in bioreactor exhaust gas were measured with a Prima BT Bench Top mass spectrometer MS (Thermo Scientific Fisher, Waltham, NC, United States) after cooling the gas with a condenser (4°C).
20 mL of culture broth samples was filtered over pre-dried and pre-weighed membrane filters (0.2 μm Supor-200, Pall Corporation, New York, NY, United States), washed with demineralized water, dried in a microwave oven (Robert Bosch GmbH, Gerlingen, Germany) for 20 min at 360 W, and reweighed. Carbon and electron balances were constructed based on the number of carbon atoms and electrons per mole, with an assumed biomass composition of CH1.8O0.5N0.2 (Roels, 1983).
2-mL samples from triplicate shake-flask enrichment cultures were centrifuged (13,000 × g, Microfuge, ThermoFisher Scientific, Waltham, NC, United States) and cell pellets were stored −80°C until analysis. Genomic DNA was extracted using the UltraClean DNA isolation kit (Qiagen Inc., CA, United States), following the manufacturer’s instructions. 16S-rRNA gene sequences in the enrichment cultures were analyzed by amplicon sequencing on an Illumina HiSeq sequencer (Novogene Bioinformatics Technology Co., Ltd., Beijing, China). Primers 341F (5′-CCTAYGGGRBGCASCAG-3′) and 805R (5′-GGACTACNNGGGTATCTAAT-3′) were used to generate 250 bp paired end reads and sequences were analyzed as described previously (van den Berg et al., 2017). Representative sequences for the dominant operational taxonomic unites (OTUs) (>1%) were submitted for taxonomic analysis in the SILVA database (SINA, version 1.2.11 (Pruesse et al., 2012) using default settings. The amplicon sequences of the 16S rRNA gene analysis are shown in Supplementary Table S3.
A 250-mL sample from a steady-state chemostat culture of L. suebicus LCV1 was centrifuged for 10 min at maximum speed (4700 × g at 4°C, Sorvall Legend XTR ThermoFisher Scientific, Waltham, NC, United States). The cell pellet was washed with TE buffer (pH 8) and stored at -20°C. DNA was extracted with the Genomic-tip 100/G Kit (Qiagen Inc., CA, United States) according to the manufacturer’s protocol except for the addition of 2.6 mg mL–1 zymolyase (20T, Amsbio, United Kingdom) and 4 mg mL–1 lysozyme (Qiagen, Hilden, Germany) to facilitate cell lysis. The amount of extracted DNA was quantified with a Qubit dsDNA BR assay kit (Thermo Fisher Scientific, Waltham, NC, United States) and its quality was assessed with NanoDropTM 2000 (Thermo Fisher Scientific, Waltham, NC, United States) and Tapestation 2200 technology (Agilent Technologies, Santa Clara, CA, United States). Sequencing was performed in-house on an Illumina MiSeq Sequencer (Illumina, San Diego, CA, United States), with MiSeq® Reagent Kit v3 with 2 × 300 bp read length to obtain a 300 cycle paired-end library with an insert-size of 550 bp using TruSeq PCR-free library preparation yielding 5.92 million reads with a total quantity of 1.78 gigabase sequence. Long-read sequencing was performed with the MinION platform (Oxford Nanopore Technologies, Oxford, United Kingdom). The MinION genomic library was prepared using Nanopore 1-D ligation sequencing kit (SQK-LSK108), using 2–3 μg of input genomic DNA fragmented in a Covaris g-Tube (Covaris, Brighton, United Kingdom) with the 8–10 kb fragments settings according to manufacturer’s instructions. All R9 flow cells (FLO MIN106) were primed with priming buffer and libraries were loaded following manufacturer’s instructions. MinKNOW software (Oxford Nanopore) was used for quality control of active pores and for sequencing. Raw files generated by MinKNOW were base called using Albacore (version 1.2.5; Oxford Nanopore). Reads, in fastq format, with a minimum length of 1000 bp were extracted, yielding 1.63 Gb sequence with an average read length of 6.28 kbp.
Base-calling was performed with Albacore v1.2.6 (Oxford Nanopore Technologies, Oxford, United Kingdom); FASTQ files were obtained and filtered on size (>1000 bp). The long-read genome sequences generated with the MinION platform were de novo assembled using Canu v1.4 (settings: genomesize = 3 m) (Koren et al., 2017); 2.78 Mbp genome into three contigs of which the largest with a length of 2.67 Mb while the two smaller with a length of 89 and 28 kbp paired-end Illumina library was aligned, using BWA (Li and Durbin, 2010), to the assembly and the resulting BAM file (Binary alignment map file) was processed by Pilon (Walker et al., 2014) for polishing the assembly (for correcting assembly errors), using correction of only SNPs and short indels (–fix bases parameter).
The assembled genome was uploaded to the automated Microscope platform (Vallenet et al., 2006, 2017). Manual assessment of pathway annotations was assisted by the MicroCyc (Caspi et al., 2008), Kyoto Encyclopedia of Genes and Genomes (KEGG; Kanehisa et al., 2014) and SwissProt alignment (BLASTP version 2.2.28 +; Altschul et al., 1997) databases. The genome sequence of L. suebicus LCV1 has been deposited at the NCBI archive with the corresponding BioProject ID PRJNA578870. Common and unique coding sequences in the two strains, with L. suebicus DSM5007 annotated with Prokka (Seemann, 2014), were identified with OrthoVenn2 (Xu et al., 2019).
A published protocol (Hansen et al., 2014) was modified to prepare whole protein extracts. Approximately 20 mg biomass (wet weight) were collected and solubilized in a solution consisting of 175 μL B-PER reagent (Illumina, San Diego, CA, United States) and 175 μL triethylammonium bicarbonate (TEAB) buffer [50 mM TEAB, 1% (w/w) sodium deoxycholate (NaDOC), adjusted to pH 8.0]. After addition of 0.1 g glass beads (acid washed, 0.1 mm diameter), cells were disrupted by bead beating with a Fast Prep FP120 (MP Biomedicals, Fisher Scientific, Hampton, NH, United States; 4 bursts of 20 s at a setting of 6 m s–1). Cell debris was removed by centrifugation (15 min at 14,000 × g) and at 4°C in a 5424R centrifuge (Eppendorf AG, Hamburg, Germany). The supernatant was transferred to a new Eppendorf tube and kept at 4°C until further processing. Proteins were precipitated by adding four volumes of ice-cold acetone. The solution was incubated at −20°C for 30 min and centrifuged 15 min at 14,000 × g and at 4°C in a 5424R centrifuge (Eppendorf AG, Hamburg, Germany). The protein pellet was washed twice with 200 μL ice-cold acetone and re-dissolved in 200 mM ammonium bicarbonate containing 8 M urea, to a final concentration of approximately 100 μg protein μL–1; 30 μL of a 10 mM dithiothreitol solution was added to 100 μL of the resulting protein solution. After 1 h incubation at 37°C, 30 μL of a freshly prepared 20 mM 3-idoleacetic acid (IAA) solution was added, followed by 30 min incubation in the dark at room temperature. The solution was then diluted to below 1 M urea with a 200 mM bicarbonate buffer. An aliquot of approximately 25 μg protein was digested overnight at 37°C using sequencing grade trypsin (Promega, Madison, WI, United States), at a trypsin-to-protein ratio of approximately 1: 50. Peptides were desalted using an Oasis HLB 96 well plate (Waters, Mildford, CT, United States) according to the manufacturer’s protocol. The purified peptide eluate was dried with a speed vac concentrator (Thermo Fisher Scientific, Waltham, NC, United States). The dried peptide fraction was resuspended in 3% acetonitrile and 0.1% formic acid solution by careful vortexing. An aliquot corresponding to approximately 50–100 ng protein digest was analyzed using a one-dimensional shot-gun proteomics [EASY nano LC connected with a QE plus Orbitrap mass spectrometer (Thermo Fisher Scientific, Waltham, NC, United States)] (Köcher et al., 2012). Raw mass spectrometry data were analyzed using PEAKS Studio X (Bioinformatics Solutions Inc.1) by searching against a global L. suebicus database downloaded from UniProtKB (July 2018). The search included a GPM crap contaminant database2 and used a decoy fusion for determining false discovery rates (FDRs). Search parameters included 20 ppm parent ion and 0.02 Da fragment ion mass error tolerance, up to three missed cleavage sites, carbamidomethylation as fixed and methionine oxidation and N/Q deamidation as variable modifications. Peptide spectrum matches were filtered against 1% FDR and protein identifications were accepted as being significant when at least two unique peptides were identified. The mass spectrometry proteomics data have been deposited to the Proteome Xchange Consortium via the PRIDE (Vizcaíno et al., 2016) partner repository with the dataset identifier PXD015964.
Cell extract of galacturonate-grown L. suebicus LCV1 (5.1 ± 0.4 g L–1) was incubated for 30 or 180 min at 30°C in a reaction mixture containing triethanolamine (TEA) buffer (100 mM, pH 7.6), 2.5 mM MgCl2.6H2O, 5 mM ATP, and 5 mM mannonate or no ATP and with 5 mM 6-phosphogluconate, unless stated otherwise. Samples were centrifuged (13,000 × g, Microfuge, ThermoFisher Scientific, Waltham, NC, United States) after incubation and the supernatant was collected; 100 μL of the supernatant was frozen at −80°C (U101 Innova freezer, Eppendorf, Hamburg, Germany) and freeze-dried over-night (Mini Lyotrap freeze-dryer, LTE Scientific Ltd., Greenfield, Oldham, United Kingdom). The supernatant was derivatized according to Niedenführ et al. (2016) without addition of AAL-mix and analyzed using a 7890A gas chromatography system (Agilent, Santa Clara, CA, United States) coupled to a 5975C MSD single quadrupole mass spectrometer (Agilent, Santa Clara, CA, United States) according to de Jonge et al. (2011); split ratio of 1: 50 for standards, split ratio of 1: 10 for samples. Identification of the peaks was done via MassHunter Workstation Qualitative Analysis software (Agilent, version B06.00) and comparison to the NIST Standard Reference Database (version 2.0).
Cells were harvested from steady-state chemostat cultures by centrifugation (5 min at 4696 × g and at 4°C, Sorvall Legend X1R, ThermoFisher Scientific, United States) and the pellet was stored at −20°C. Cells were washed and suspended in 100 mM potassium-phosphate buffer (pH 7.5) with 2 mM MgCl2 and protease inhibitors (completeTM Protease inhibitor cocktail, Merck group, Darmstad, Germany). For LDH activity assays, 1 mM dithiothreitol was added instead of protease inhibitors. After cell disruption with a Fast Prep FP120 (MP Biomedicals, Fisher Scientific, Hampton, NH, United States; 4 bursts of 20 s at a setting of 6 m s–1), cell debris was removed by centrifugation [20 min at 4°C and at 20,000 r/min (rotor SS-34) Sorval RC 5C plus] and the resulting cell extract was directly used for enzyme assays. Protein concentrations were determined with the Lowry assay (Lowry et al., 1951).
Activity of LDH was measured according to van Maris et al. (2004), fructuronate reductase (UxuB) according to Linster and van Schaftingen (2004) and PDH according to Flikweert et al. (1997) in an anaerobic environment (Vinyl Anaerobic Chamber; gas phase 2.3% H2 and 97.7% N2; Coy Lab, United States) with an AvaSpec-3648 high-resolution spectrometer with AvaLight-DH-S light source (Avantes, Apeldorn, Netherlands). Aerobic enzyme-activity assays were measured at 340 nm and 30°C with a Hitachi model U-3010 spectrophotometer (Sysmex Europe GmbH, Norderstedt, Germany). Fructuronate reductase (UxuB) was measured in a reaction mixture containing TEA buffer (100 mM, pH 7.6), 2.5 mM MgCl2.6H2O, 0.6 mM NADPH, and cell extract of galacturonate-grown L. suebicus LCV1 (5.1 ± 0.4 g protein L–1). The reaction was stated with the addition of tagaturonate (0.5 mM) (Huisjes et al., 2012). 6-Phosphogluconate dehydrogenase (GndA) was measured as described previously (Scott and Abramsky, 1973), but with a 50 mM potassium-phosphate buffer (pH 7.5). The reaction was started by addition of 6-phosphogluconate (5 mM). Gluconate kinase was measured via a coupled reaction with 6-phoshogluconate dehydrogenase (0.8 U, Saccharomyces cerevisiae, Sigma–Aldrich, St. Louis, MO, United States). The reaction mixture contained TEA buffer (100 mM, pH 7.6), 2.5 mM MgCl2.6H2O, 1 mM ATP, 0.15 mM NADP+ and the reaction was started by addition of gluconate (0.5 mM, unless stated otherwise). The same assay was used for estimation of mannonate-kinase activities, by starting the reaction with mannonate (0.2 mM unless stated otherwise) instead of gluconate and using native L. suebicus 6-mannonate 2-epimerase activity in cell extracts as coupling enzyme. All assays were performed in duplicate and reaction rates were proportional to the amount of cell extract added.
The datasets generated for this study can be found in the NCBI archive BioProject ID PRJNA578870, PRIDE repository PXD015964.
ML, JP, and LV designed the experiments, interpreted the results, and wrote the manuscript. LV performed the enrichment, isolation, and bioreactor experiments. LV and MB analyzed the L. suebicus genome. ML and LV performed the continuous and discontinuous enzymatic assays. MP and CR performed and interpreted MS studies on proteome and metabolites, respectively. All authors read and approved the final manuscript.
This research was supported by the SIAM Gravitation Grant 024.002.002 and the Netherlands Organization for Scientific Research.
The authors declare that the research was conducted in the absence of any commercial or financial relationships that could be construed as a potential conflict of interest.
The authors thank B. Abbas for his help with the 16S rRNA-gene amplicon sequencing, P. de la Torre-Cortés for whole-genome sequencing, and S. L. de Jong and M. Wissink for their contributions to the experimental work as part of their M.Sc. and B.Sc. thesis projects, respectively.
The Supplementary Material for this article can be found online at: https://www.frontiersin.org/articles/10.3389/fmicb.2019.03027/full#supplementary-material
Abbott, D. A., Suir, E., Van Maris, A. J. A., and Pronk, J. T. (2008). Physiological and transcriptional responses to high concentrations of lactic acid in anaerobic chemostat cultures of Saccharomyces cerevisiae. Appl. Environ. Microbiol. 74, 5759–5768. doi: 10.1128/AEM.01030-8
Altschul, S. F., Madden, T. L., Schäffer, A. A., Zhang, J., Zhang, Z., Miller, W., et al. (1997). Gapped BLAST and PSI-BLAST: a new generation of protein database search programs. Nucleic Acids Res. 25, 3389–3402. doi: 10.1093/nar/25.17.3389
Ashwell, G., Wahba, A. J., and Hickman, J. (1960). Uronic acid metabolism in bacteria I purification and properties of uronic acid isomerase in Escherichia coli. J. Biol. Chem. 235, 1559–1565.
Bahl, H., Andersch, W., Braun, K., and Gottschalk, G. (1982). Effect of pH and butyrate concentration on the production of acetone and butanol by Clostridium acetobutylicum grown in continuous culture. Eur. J. Appl. Microbiol. Biotechnol. 14, 17–20. doi: 10.1007/BF00507998
Biz, A., Farias, F. C., Motter, F. A., De Paula, D. H., Richard, P., Krieger, N., et al. (2014). Pectinase activity determination: an early deceleration in the release of reducing sugars throws a spanner in the works! PLoS One 9:e109529. doi: 10.1371/journal.pone.0109529
Busse, M., Kindel, P. K., and Gibbs, M. (1961). The heterolactic fermentation III position of C14 in the products of fructose dissimilation by Leuconostoc mesenteroides. J. Biol. Chem. 236, 2850–2853.
Caspi, R., Foerster, H., Fulcher, C. A., Kaipa, P., Krummenacker, M., Latendresse, M., et al. (2008). The MetaCyc database of metabolic pathways and enzymes and the BioCyc collection of pathway/genome databases. Nucleic Acids Res. 36, 623–631. doi: 10.1093/nar/gkm900
Chang, Y. F., and Feingold, D. S. (1970). D-Glucaric acid and galactaric acid catabolism by Agrobacterium tumefaciens. J. Bacteriol. 102, 85–96.
Cohen, S. S. (1950). Gluconokinase and oxidative path of glucose-6-phosphate utilization. J. Bacteriol. 189, 617–629.
Cynkin, M. A., and Ashwell, G. (1960). Uronic acid metabolism in bacteria IV purification and properties of 2-keto-3-deoxy-D-gluconokinase in Eschericia coli. J. Biol. Chem. 235, 1576–1579.
de Jonge, L. P., Buijs, N. A. A., ten Pierick, A., Deshmukh, A., Zhao, Z., Kiel, J. A. K. W., et al. (2011). Scale-down of penicillin production in Penicillium chrysogenum. Biotechnol. J. 6, 944–958. doi: 10.1002/biot.201000409
de Vries, R. P., and Visser, J. (2001). Aspergillus enzymes involved in degradation of plant cell wall polysaccharides. Microbiol. Mol. Biol. Rev. 65, 497–522. doi: 10.1128/MMBR.65.4.497
de Vries, W., Kapteijn, W. M. C., van der Beek, E. G., and Stouthamer, A. H. (1970). Molar growth yields and fermentation balances of Lactobacillus casei L3 in batch cultures and in continuous cultures. J. Gen. Microbiol. 63, 333–345. doi: 10.1099/00221287-63-3-333
DeMoss, R. D., Bard, R. C., and Gunsalus, I. C. (1951). The mechanism of the heterolactic fermentation: a new route of ethanol formation. J. Biol. Chem. 62, 499–511.
Doran, J. B., Cripe, J., Sutton, M., and Foster, B. (2000). Fermentations of pectin-rich biomass with recombinant bacteria to produce fuel ethanol. Appl. Biochem. Biotechnol. 84–86, 141–153.
Edwards, M. C., and Doran-Peterson, J. (2012). Pectin-rich biomass as feedstock for fuel ethanol production. Appl. Microbiol. Biotechnol. 95, 565–575. doi: 10.1007/s00253-012-4173-2
Eklund, T. (1983). The antimicrobial effect of dissociated and undissociated sorbic acid at different pH levels. J. Appl. Bacteriol. 54, 383–389. doi: 10.1111/j.1365-2672.1983.tb02632.x
Fan, D. F., and Feingold, D. S. (1972). Biosynthesis of uridine diphosphate D-xylose V. UDP-D-glucuronate and UDP-D-galacturonate carboxy-lyase of Ampullariella digitata. Arch. Biochem. Biophys. 148, 576–580. doi: 10.1016/0003-9861(72)90176-2
Fang, H. H. P., and Liu, H. (2002). Effect of pH on hydrogen production from glucose by a mixed culture. Bioresour. Technol. 82, 87–93. doi: 10.1016/S0960-8524(01)00110-9
Flikweert, M. T., Van Dijken, J. P., and Pronk, J. T. (1997). Metabolic responses of pyruvate decarboxylase-negative Saccharomyces cerevisiae to glucose excess. Appl. Environ. Microbiol. 63, 3399–3404.
Food and Agriculture Organization of the United Nations (2016). Crops, Production Quantities of Sugar Beet, Citrus Fruits and Apples in the World. Rome: FAOSTAT.
Gibbs, M., Sokatch, J. T., and Gunsalus, I. C. (1955). Product of labeling of glucose-1-C14 fermentation by homofermentative and heterofermentative lactic acid bacteria. J. Biol. Chem. 70, 572–576.
Grimmler, C., Janssen, H., Krauße, D., Fischer, R. J., Bahl, H., Dürre, P., et al. (2011). Genome-wide gene expression analysis of the switch between acidogenesis and solventogenesis in continuous cultures of Clostridium acetobutylicum. J. Mol. Microbiol. Biotechnol. 20, 1–15. doi: 10.1159/000320973
Grohmann, K., Baldwin, E. A., and Buslig, B. S. (1994). Fermentation of galacturonic acid and other sugars in orange peel hydrolysates by the ethanologenic strain of Escherichia coli. Biotechnol. Lett. 16, 281–286. doi: 10.1007/bf00134626
Grohmann, K., Manthey, J. A., Cameron, R. G., and Buslig, B. S. (1998). Fermentation of galacturonic acid and pectin-rich materials to ethanol by genetically modified strains of Erwinia. Biotechnol. Lett. 20, 195–200. doi: 10.1023/A:1005349112770
Gunsalus, I. C., and Gibbs, M. (1952). The heterolactic fermentation: II. Position of C14 in the products of glucose dissimilation by Leuconostroc mesenteroides. J. Biol. Chem. 194, 871–875.
Hansen, R. G., and Henning, U. (1966). Regulation of pyruvate dehydrogenase activity in Escherichia coli K12. Biochim. Biophys. Acta 122, 355–358. doi: 10.1016/0926-6593(66)90076-2
Hansen, S. H., Stensballe, A., Nielsen, P. H., and Herbst, F.-A. (2014). Metaproteomics: evaluation of protein extraction from activated sludge. Proteomics 14, 2535–2539. doi: 10.1002/pmic.201400167
Hickman, J., and Ashwell, G. (1960). Uronic acid metabolism in bacteria II Purification and properties of D-altronic acid and D-mannonic acid dehydrogenases in Escherichia coli. J. Biol. Chem. 235, 1566–1570.
Hirst, E. L. (1942). Recent progress in the chemistry of the pectic materials and plant gums. J. Chem. Soc. 70–78.
Horiuchi, J. I., Shimizu, T., Tada, K., Kanno, T., and Kobayashi, M. (2002). Selective production of organic acids in anaerobic acid reactor by pH control. Bioresour. Technol. 82, 209–213. doi: 10.1016/S0960-8524(01)00195-X
Huisjes, E. H., Luttik, M. A. H., Almering, M. J. H., Bisschops, M. M. M., Dang, D. H. N., Kleerebezem, M., et al. (2012). Toward pectin fermentation by Saccharomyces cerevisiae: expression of the first two steps of a bacterial pathway for D-galacturonate metabolism. J. Biotechnol. 162, 303–310. doi: 10.1016/j.jbiotec.2012.10.003
Izu, H., Adachi, O., and Yamada, M. (1996). Purification and characterization of the Escherichia coli thermoresistant gluconokinase encoded by the gntK gene. FEBS Lett. 394, 14–16. doi: 10.1016/0014-5793(96)00923-4
Jeske, L., Placzek, S., Schomburg, I., Chang, A., and Schomburg, D. (2019). BRENDA in 2019: a European ELIXIR core data resource. Nucleic Acids Res. 47, D542–D549. doi: 10.1093/nar/gky1048
Johnson, K., Jiang, Y., Kleerebezem, R., Muyzer, G., and van Loosdrecht, M. C. M. (2009). Enrichment of a mixed bacterial culture with a high polyhydroxyalkanoate storage capacity. Biomacromolecules 10, 670–676. doi: 10.1021/bm8013796
Kanehisa, M., Goto, S., Sato, Y., Kawashima, M., Furumichi, M., and Tanabe, M. (2014). Data, information, knowledge and principle: back to metabolism in KEGG. Nucleic Acids Res. 42, D199–D205. doi: 10.1093/nar/gkt1076
Khanh, N. Q., Ruttkowski, E., Leidinger, K., Albrecht, H., and Gottschalk, M. (1991). Characterization and expression of a genomic pectin methyl esterase-encoding gene in Aspergillus niger. Gene 106, 71–77. doi: 10.1016/0378-1119(91)90567-U
Kleerebezem, R., and van Loosdrecht, M. C. M. (2007). Mixed culture biotechnology for bioenergy production. Curr. Opin. Biotechnol. 18, 207–212. doi: 10.1016/j.copbio.2007.05.001
Kleynmans, U., Heinzl, H., and Hammes, W. P. (1989). Lactobacillus suebicus sp. nov., an obligately heterofermentative Lactobacillus species isolated from fruit mashes. Syst. Appl. Microbiol. 11, 267–271. doi: 10.1016/s0723-2020(89)80024-4
Köcher, T., Pichler, P., Swart, R., and Mechtler, K. (2012). Analysis of protein mixtures from whole-cell extracts by single-run nanoLC-MS/MS using ultralong gradients. Nat. Protoc. 7, 882–890. doi: 10.1038/nprot.2012.036
Koren, S., Walenz, B. P., Berlin, K., Miller, J. R., Bergman, N. H., and Phillippy, A. M. (2017). Canu: scalable and accurate long-read assembly via adaptive k-mer weighting and repeat separation. Genome Res. 27, 722–736. doi: 10.1101/gr.215087.116.Freely
Kovachevich, R., and Wood, W. A. (1955). Carbohydrate metabolism by Pseudomonas fluorescens IV purification and properties of 2-keto-3-deoxy-6-phospho-gluconate aldolase. J. Biol. Chem. 213, 757–767. doi: 10.1016/j.mpsur.2013.04.008
Kuivanen, J., Biz, A., and Richard, P. (2019). Microbial hexuronate catabolism in biotechnology. AMB Express 9, 1–11. doi: 10.1186/s13568-019-0737-1
Kuorelahti, S., Kalkkinen, N., Penttilä, M., Londesborough, J., and Richard, P. (2005). Identification in the mold Hypocrea jecorina of the first fungal D-galacturonic acid reductase. Biochemistry 44, 11234–11240. doi: 10.1021/bi050792f
Leyer, G. J., Wang, L. L., and Johnson, E. A. (1995). Acid adaptation of Escherichia coli O157:H7 increases survival in acidic foods. Appl. Environ. Microbiol. 61, 3752–3755.
Li, H., and Durbin, R. (2010). Fast and accurate long-read alignment with Burrows-Wheeler transform. Bioinformatics 26, 589–595. doi: 10.1093/bioinformatics/btp698
Link, K. P., and Niemann, C. (1930). The action of weak mineral acids on uronic acids. J. Am. Chem. Soc. 52, 2474–2480. doi: 10.1021/ja01369a046
Linster, C. L., and van Schaftingen, E. (2004). A spectrophotometric assay of D-glucuronate based on Escherichia coli uronate isomerase and mannonate dehydrogenase. Protein Expr. Purif. 37, 352–360. doi: 10.1016/j.pep.2004.06.015
Lowry, O. H., Rosenbourgh, N. J., Farr, A. L., and Randall, R. J. (1951). Protein measurements with the folin phenol reagent. J. Biol. Chem. 193, 265–276.
Mandrand-Berthelot, M.-A., and Lagarde, A. E. (1977). “Hit-and-run” mechanism for D-glucuronate reductase catalyzed by D-mannonate:NAD oxidoreductase of Escherichia coli. Biochim. Biophys. Acta 483, 6–23. doi: 10.1016/0005-2744(77)90003-1
Marounek, M., and Dušková, D. (1999). Metabolism of pectin in rumen bacteria Butyrivibrio fibrisolvens and Prevotella ruminicola. Lett. Appl. Microbiol. 29, 429–433. doi: 10.1046/j.1472-765X.1999.00671.x
Martens-Uzunova, E. S., and Schaap, P. J. (2008). An evolutionary conserved D-galacturonic acid metabolic pathway operates across filamentous fungi capable of pectin degradation. Fungal Genet. Biol. 45, 1449–1457. doi: 10.1016/j.fgb.2008.08.002
Marzo, C., Diaz, A. B., Caro, I., and Blandino, A. (2019). “Status and perspectives in bioethanol production from sugar beet,” in Bioethanol Production From Food Crops, eds R. Ramesh, and S. Ramachandran (Cadiz: Elsevier Inc.), 158–161.
McKinnis, R. B. (1928). An investigation of the hypothetical combined pentose and the so-called free pentose with inferences on the composition of pectin. J. Am. Chem. Soc. 50, 1911–1915. doi: 10.1021/ja01394a015
McNair Scott, D. B., and Cohen, S. S. (1952). The oxidative pathway of carbohydrate metabolism in Escherichia coli. I The isolation and properties of glucose 6-phosphate dehydrogenase and 6-phosphogluconate dehydrogenase. Biochem. J. 55, 23–32. doi: 10.1042/bj0630593
McNair Scott, D. B., and Cohen, S. S. (1956). The oxidative pathway of carbohydrate metabolism in Escherichia coli. V. Isolation and identification of ribulose phosphate produced from 6-phosphogluconate by the dehydrogenase of E. coli. Biochem. J. 65, 686–689. doi: 10.1042/bj0630593
Mohnen, D. (2008). Pectin structure and biosynthesis. Curr. Opin. Plant Biol. 11, 266–277. doi: 10.1016/j.pbi.2008.03.006
Nam, S.-H., Choi, S.-H., Kang, A., Kim, D.-W., Kim, R. N., Kim, D.-S., et al. (2011). Genome sequence of Lactobacillus suebicus KCTC 3549. J. Bacteriol. 193, 5532–5533. doi: 10.1128/JB.05814-1
Nelson, K. E., Clayton, R. A., Gill, S. R., Gwinn, M. L., Dodson, R. J., Haft, D. H., et al. (1999). Evidence for lateral gene transfer between archaea and bacteria from genome sequence of Thermotoga maritima. Nature 399, 323–329. doi: 10.1038/20601
Niedenführ, S., ten Pierick, A., van Dam, P. T. N., Suarez-Mendez, C. A., Nöh, K., and Wahl, S. A. (2016). Natural isotope correction of MS/MS measurements for metabolomics and 13C fluxomics. Biotechnol. Bioeng. 113, 1137–1147. doi: 10.1002/bit.25859
Parks, D. H., Imelfort, M., Skennerton, C. T., Hugenholtz, P., and Tyson, G. W. (2015). CheckM: assessing the quality of microbial genomes recovered from isolates, single cells, and metagenomes. Genome Res. 25, 1043–1055. doi: 10.1101/gr.186072.114
Peekhaus, N., and Conway, T. (1998). What’s for dinner?: entner-doudoroff metabolism in Escherichia coli. J. Bacteriol. 180, 3495–3502.
Portalier, R. C., and Stoeber, F. R. (1972). La D-mannonate: NAD-oxydoréductase d’Escherichia coli K12. Purification, propriétés et individualité. Eur. J. Biochem. 26, 290–300. doi: 10.1111/j.1432-1033.1972.tb01767.x
Pruesse, E., Peplies, J., and Glöckner, F. O. (2012). SINA: accurate high-throughput multiple sequence alignment of ribosomal RNA genes. Bioinformatics 28, 1823–1829. doi: 10.1093/bioinformatics/bts252
Rodionova, I. A., Scott, D. A., Grishin, N. V., Osterman, A. L., and Rodionov, D. A. (2012). Tagaturonate-fructuronate epimerase UxaE, a novel enzyme in the hexuronate catabolic network in Thermotoga maritima. Environ. Microbiol. 14, 2920–2934. doi: 10.1111/j.1462-2920.2012.02856.x
Ruff, O. (1898). Über die Verwandlung der D-gluconsäure in D-arabinose. Berichte der Dtsch. Chem. Gesellschaft 31, 1573–1577. doi: 10.1002/cber.18980310250
Salmond, C. V., Kroll, R. G., and Booth, I. R. (1984). The effect of food preservatives on pH homeostasis in Escherichia coli. J. Gen. Microbiol. 130, 2845–2850. doi: 10.1099/00221287-130-11-2845
Schomburg, I., Jeske, L., Ulbrich, M., Placzek, S., Chang, A., and Schomburg, D. (2017). The BRENDA enzyme information system–from a database to an expert system. J. Biotechnol. 261, 194–206. doi: 10.1016/j.jbiotec.2017.04.020
Scott, W. A., and Abramsky, T. (1973). Neurospora 6-phosphogluconate dehydrogenase I. Purification and characterization of the wild type enzyme. J. Biol. Chem. 248, 3535–3541.
Searle-van Leeuwen, M. J. F., Vincken, J. P., and Beldman, G. (1996). “Acetyl esterases of Aspergillus niger: purification and mode of action on pectins,” in Progress in Biotechnology 14: Pectins and Pectinaseseds, eds D. Schipper, and A. G. J. Voragen Amsterdam, (Amsterdam: Elsevier).
Seemann, T. (2014). Prokka: rapid prokaryotic genome annotation. Bioinformatics 30, 2068–2069. doi: 10.1093/bioinformatics/btu153
Senoura, T., Taguchi, H., Ito, S., Hamada, S., Matsui, H., Fukiya, S., et al. (2009). Identification of the cellobiose 2-epimerase gene in the genome of Bacteroides fragilis NCTC 9343. Biosci. Biotechnol. Biochem. 73, 400–406. doi: 10.1271/bbb.80691
Siezen, R. J., Bayjanov, J. R., Felis, G. E., van der Sijde, M. R., Starrenburg, M., Molenaar, D., et al. (2011). Genome-scale diversity and niche adaptation analysis of Lactococcus lactis by comparative genome hybridization using multi-strain arrays. Microb. Biotechnol. 4, 383–402. doi: 10.1111/j.1751-7915.2011.00247.x
Smiley, J. D., and Ashwell, G. (1960). Uronic acid metabolism in bacteria III purification and properties of D-altronic acid and D-mannonic acid dehydrases in Escherichia coli. J. Biol. Chem. 235, 1571–1575.
Snoep, J. L., de Graef, M. R., Westphal, A. H., de Kok, A., Teixeira de Mattos, M. J., and Neijssel, O. M. (1993). Differences in sensitivity to NADH of purified pyruvate dehydrogenase complexes of Enterococcus faecalis, Lactococcus lactis, Azotobacter vinelandii and Escherichia coli: implications for their activity in vivo. FEMS Microbiol. Lett. 114, 279–283. doi: 10.1016/0378-1097(93)90284-9
Snoep, J. L., Westphal, A. H., Benen, J. A. E., Teixeira de Mattos, M. J., Neijssel, O. M., and de Kok, A. (1992). Isolation and characterisation of the pyruvate dehydrogenase complex of anaerobically grown Enterococcus faecalis NCTC 775. Eur. J. Biochem. 203, 245–250. doi: 10.1111/j.1432-1033.1992.tb19853.x
Temudo, M. F., Kleerebezem, R., and van Loosdrecht, M. (2007). Influence of the pH on (open) mixed culture fermentation of glucose: a chemostat study. Biotechnol. Bioeng. 98, 69–79. doi: 10.1002/bit
Ten Brink, B., and Konings, W. N. (1986). Generation of a protonmotive force in anaerobic bacteria by end-product efflux. Methods Enzymol. 125, 492–510. doi: 10.1016/S0076-6879(86)25039-9
Thomas, T. D., Ellwood, D. C., and Longyear, V. M. C. (1979). Change from homo- to heterolactic fermentation by Streptococcus lactis resulting from glucose limitation in anaerobic chemostat cultures. J. Bacteriol. 138, 109–117.
Tong, S., Porco, A., Isturiz, T., and Conway, T. (1996). Cloning and molecular genetic characterization of the Escherichia coli gntR, gntK and gntU, the main system for gluconate metabolism. J. Bacteriol. 178, 1584–1590. doi: 10.1128/jb.179.5.1584-1590.1997
Trchounian, A., and Trchounian, K. (2019). Fermentation revisited: how do microorganisms survive under energy-limited conditions? Trends Biochem. Sci. 44, 391–400. doi: 10.1016/j.tibs.2018.12.009
Valk, L. C., Frank, J., de la Torre-Cortes, P., van ’t Hof, M., van Maris, A. J. A., Pronk, J. T., et al. (2018). Galacturonate metabolism in anaerobic chemostat enrichment cultures: combined fermentation and acetogenesis by the dominant sp. nov. “Candidatus Galacturonibacter soehngenii. Appl. Environ. Microbiol. 84:e01370-18. doi: 10.1128/AEM.01370-18
Vallenet, D., Calteau, A., Cruveiller, S., Gachet, M., Lajus, A., Josso, A., et al. (2017). MicroScope in 2017: an expanding and evolving integrated resource for community expertise of microbial genomes. Nucleic Acids Res. 45, D517–D528. doi: 10.1093/nar/gkw1101
Vallenet, D., Labarre, L., Rouy, Z., Barbe, V., Bocs, S., Cruveiller, S., et al. (2006). MaGe: a microbial genome annotation system supported by synteny results. Nucleic Acids Res. 34, 53–65. doi: 10.1093/nar/gkj406
van den Berg, E. M., Elisário, M. P., Kuenen, J. G., Kleerebezem, R., and van Loosdrecht, M. C. M. (2017). Fermentative bacteria influence the competition between denitrifiers and DNRA bacteria. Front. Microbiol. 8:1684. doi: 10.3389/fmicb.2017.01684
van Hylckama Vlieg, J. E., Rademaker, J. L. W., Bachmann, H., Molenaar, D., Kelly, W. J., and Siezen, R. J. (2006). Natural diversity and adaptive responses of Lactococcus lactis. Curr. Opin. Biotechnol. 17, 183–190. doi: 10.1016/j.copbio.2006.02.007
van Maris, A. J. A., Abbott, D. A., Bellissimi, E., van den Brink, J., Kuyper, M., Luttik, M. A. H., et al. (2006). Alcoholic fermentation of carbon sources in biomass hydrolysates by Saccharomyces cerevisiae: current status. Antonie van Leeuwenhoek 90, 391–418. doi: 10.1007/s10482-006-9085-7
van Maris, A. J. A., Winkler, A. A., Porro, D., van Dijken, J. P., and Pronk, J. T. (2004). Homofermentative lactate production cannot sustain anaerobic growth of engineered Saccharomyces cerevisiae: possible consequence of energy-dependent lactate export. Appl. Environ. Microbiol. 70, 2898–2905. doi: 10.1128/AEM.70.5.2898
Van Overtveldt, S., Verhaeghe, T., Joosten, H. J., van den Bergh, T., Beerens, K., and Desmet, T. (2015). A structural classification of carbohydrate epimerases: from mechanistic insights to practical applications. Biotechnol. Adv. 33, 1814–1828. doi: 10.1016/j.biotechadv.2015.10.010
Verduyn, C., van Dijken, J. P., Postma, E., and Scheffers, W. A. (1992). Effect of benzoic acid on metabolic fluxes in yeasts: a continuous-culture study on the regulation of respiration and alcoholic fermentation. Yeast 8, 501–517. doi: 10.1002/yea.320080703
Vizcaíno, J. A., Csordas, A., Del-Toro, N., Dianes, J. A., Griss, J., Lavidas, I., et al. (2016). 2016 update of the PRIDE database and its related tools. Nucleic Acids Res. 44, D447–D456. doi: 10.1093/nar/gkv1145
Voragen, A. G. J., Coenen, G. J., Verhoef, R. P., and Schols, H. A. (2009). Pectin, a versatile polysaccharide present in plant cell walls. Struct. Chem. 20, 263–275. doi: 10.1007/s11224-009-9442-z
Wagner, N., Tran, Q. H., Richter, H., Selzer, P. M., and Unden, G. (2005). Pyruvate fermentation by Oenococcus oeni and Leuconostoc mesenteroides and role of pyruvate dehydrogenase in anaerobic fermentation. Appl. Environ. Microbiol. 71, 4966–4971. doi: 10.1128/AEM.71.9.4966
Walker, B. J., Abeel, T., Shea, T., Priest, M., Abouelliel, A., Sakthikumar, S., et al. (2014). Pilon: an integrated tool for comprehensive microbial variant detection and genome assembly improvement. PLoS One 9:e112963. doi: 10.1371/journal.pone.0112963
Xu, L., Dong, Z., Fang, L., Luo, Y., Wei, Z., Guo, H., et al. (2019). OrthoVenn2: a web server for whole-genome comparison and annotation of orthologous clusters across multiple species. Nucleic Acids Res. 47, W52–W58. doi: 10.1093/nar/gkz333
Zachariou, M., and Scopes, R. (1985). Gluconate kinase from Zymomonas mobilis: isolation and characteristics. Biochem. Int. 10, 367–371.
Zajic, J. E. (1959). Hexuronic dehydrogenase of Agrobacterium tumefaciens. J. Bacteriol. 78, 734–735.
Zhang, L., Thiewes, H., and van Kan, J. A. L. (2011). The D-galacturonic acid catabolic pathway in Botrytis cinerea. Fungal Genet. Biol. 48, 990–997. doi: 10.1016/j.fgb.2011.06.002
Zoetemeyer, R. J., Arnoldy, P., Cohen, A., and Boelhouwer, C. (1982a). Influence of temperature on the anaerobic acidification of glucose in a mixed culture forming part of a two-stage digestion process. Water Res. 16, 313–321. doi: 10.1016/0043-1354(82)90191-9
Keywords: galacturonic acid, pectin degradation, Entner–Doudoroff pathway, enrichment cultivation, heterolactic fermentation, anaerobic metabolism
Citation: Valk LC, Luttik MAH, de Ram C, Pabst M, van den Broek M, van Loosdrecht MCM and Pronk JT (2020) A Novel D-Galacturonate Fermentation Pathway in Lactobacillus suebicus Links Initial Reactions of the Galacturonate-Isomerase Route With the Phosphoketolase Pathway. Front. Microbiol. 10:3027. doi: 10.3389/fmicb.2019.03027
Received: 28 October 2019; Accepted: 17 December 2019;
Published: 17 January 2020.
Edited by:
Jörg Stülke, University of Göttingen, GermanyReviewed by:
Christian U. Riedel, University of Ulm, GermanyCopyright © 2020 Valk, Luttik, de Ram, Pabst, van den Broek, van Loosdrecht and Pronk. This is an open-access article distributed under the terms of the Creative Commons Attribution License (CC BY). The use, distribution or reproduction in other forums is permitted, provided the original author(s) and the copyright owner(s) are credited and that the original publication in this journal is cited, in accordance with accepted academic practice. No use, distribution or reproduction is permitted which does not comply with these terms.
*Correspondence: Jack T. Pronk, ai50LnByb25rQHR1ZGVsZnQubmw=
Disclaimer: All claims expressed in this article are solely those of the authors and do not necessarily represent those of their affiliated organizations, or those of the publisher, the editors and the reviewers. Any product that may be evaluated in this article or claim that may be made by its manufacturer is not guaranteed or endorsed by the publisher.
Research integrity at Frontiers
Learn more about the work of our research integrity team to safeguard the quality of each article we publish.