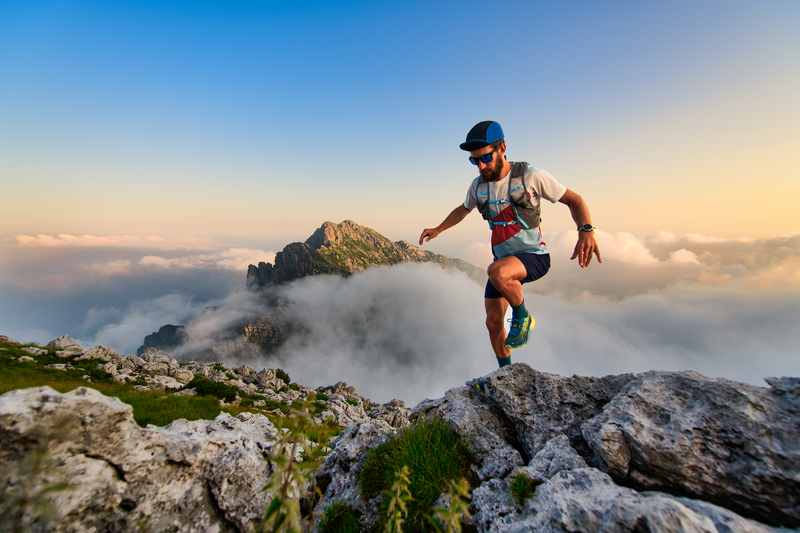
94% of researchers rate our articles as excellent or good
Learn more about the work of our research integrity team to safeguard the quality of each article we publish.
Find out more
ORIGINAL RESEARCH article
Front. Microbiol. , 08 January 2020
Sec. Antimicrobials, Resistance and Chemotherapy
Volume 10 - 2019 | https://doi.org/10.3389/fmicb.2019.02957
This article is part of the Research Topic Exploiting Novel Combined Host- and Pathogen-Directed Therapies for Combating Bacterial Multidrug Resistance View all 12 articles
Infections due to carbapenem-resistant NDM-producing Escherichia coli represent a major therapeutic challenge, especially in situations of pre-existing colistin resistance. The aim of this study was to investigate combinatorial pharmacodynamics of colistin and tigecycline against E. coli harboring blaNDM–5 and mcr-1, with possible mechanisms explored as well. Colistin disrupted the bacterial outer-membrane and facilitated tigecycline uptake largely independent of mcr-1 expression, which allowed a potentiation of the tigecycline-colistin combination. A concentration-dependent decrease in colistin MIC and EC50 was observed with increasing tigecycline levels. Clinically relevant concentrations of colistin and tigecycline combination significantly decreased bacterial density of colistin-resistant E. coli by 3.9 to 6.1-log10 cfu/mL over 48 h at both inoculums of 106 and 108 cfu/mL, and were more active than each drug alone (P < 0.01). Importantly, colistin and tigecycline combination therapy was efficacious in the murine thigh infection model at clinically relevant doses, resulting in >2.0-log10cfu/thigh reduction in bacterial density compared to each monotherapy. These data suggest that the use of colistin and tigecycline combination can provide a therapeutic alternative for infection caused by multidrug-resistant E. coli that harbored both blaNDM–5 and mcr-1.
Infections caused by carbapenem-resistant Enterobacteriaceae (CRE), especially the New Delhi metallo-β-lactamases (NDM)-producing Escherichia coli, have become a global therapeutic challenge in clinical and public health settings (Perez and Bonomo, 2018). In general, isolates carrying blaNDM tend to carry other resistance genes thus limiting treatment options (Falagas et al., 2014; Liu et al., 2019). Currently, the polymyxin antibiotics (polymyxin B and colistin) have reemerged as the last-line therapy against CRE. However, the clinical efficacy of polymyxin antibiotics has been significantly compromised by the widespread emergence of mobile colistin resistance gene mcr-1 (Liu et al., 2016). Worryingly, the MCR-1-producing E. coli that coexist with NDM-1, NDM-5, and NDM-9 have been recently reported worldwide, and these isolates possess resistance to fluoroquinolones, sulfonamides, β-lactams, tetracycline, and aminoglycosides (Du et al., 2016; Yao et al., 2016). Fortunately, the level of mcr-1-mediated colistin resistance is moderate (Sun et al., 2018), thus the use of colistin-based combinations would be of considerable clinical significance.
Tigecycline is the first of glycylcycline class that exhibited mainly bacteriostatic activity (Meagher et al., 2005). Of note, the decreased clinical efficacy and increased mortality have been previously reported regarding tigecycline monotherapy in the treatment of severe infections (Yahav et al., 2011). Therefore, clinicians should avoid tigecycline monotherapy to reserve it as another last-resort drug.
In this study, we systemically investigated the activity of colistin and tigecycline combination at the clinically achievable concentrations in vitro and in a murine thigh infection model against carbapenem-resistant E. coli harboring blaNDM–5, especially in situations of pre-existing the mcr-1 gene and high bacterial burdens. Additionally, we explored the underlying mechanisms of this combination (Figure 1) by determination of bacterial out-membrane integrity and tigecycline accumulation.
Figure 1. Graphic potential mechanisms for increased activity of colistin in combination with tigecycline against E. coli harboring blaNDM–5 and mcr-1: colistin-induced bacterial outer-membrane perturbation and tigecycline accumulation. CST, colistin; TGC, tigecycline.
Five well-described E. coli strains used in this study were 2630 (ST3902, blaNDM–5), 3112 (ST1011, mcr-1), 1320 (ST648; blaNDM–5, mcr-1), 2610 (ST101; blaNDM–5, mcr-1), and 2121 (ST156; blaNDM–5, mcr-1) (Sun et al., 2016a, b; Zhou et al., 2017). The E. coli strain ATCC 25922 (ST73) served as the negative control. The organisms were grown, subcultured, and quantified in cation-adjusted Mueller-Hinton broth (CAMHB) and agar (MHA; Difco Laboratories, Detroit, MI, United States). Colistin (CST), tigecycline (TGC), and other used antibiotics were purchased from Sigma-Aldrich (Shanghai, China) and prepared as fresh stock solutions in sterile water or medium prior to experiments.
The MICs of colistin for each E. coli strain were determined in the absence and presence of twofold increasing tigecycline concentrations (0.13–0.5 mg/L) using a modified broth microdilution method (Wiegand et al., 2008). The interaction of this combination was evaluated in duplicate for each isolate with a checkerboard assay (CST range 0.25–32 mg/L; TGC range 0.015–32 mg/L). Inhibition was read visually to calculate the fractional inhibitory concentration index (FICI), with an FICI ≤ 0.5 deemed synergistic. In addition, cell density was assessed using a spectrometer to estimate cell densities for MacSynergy II analysis (Prichard and Shipman, 1990). The MacSynergy II program uses the Bliss independence algorithm to generate a 3-dimensional response profile of the synergy-antagonism landscape by representing the theoretical indifferent surface. Peaks and troughs represent synergy and antagonism, respectively, and the extents of these were defined using interaction volumes (μM2): <25, additive; 25 to 50, minor but significant; 50 to 100, moderate; and >100, strong synergy or antagonism (Deshpande et al., 2016; Lai et al., 2016). The results were expressed as the mean interaction volumes calculated at the 95% confidence level from three independent experiments.
The 1-N-phenylnaphthylamine (NPN) assay was performed to assess bacterial outer-membrane permeability to colistin as previously described (Buyck et al., 2012). Uptake of NPN by E. coli cells was a measure of the degree of permeability, and the subsequent fluorescence indicated a permeability breakdown (Macnair et al., 2018). Thus, NPN uptake was used to quantitatively indicate the colistin-induced outer membrane disruption. Mid-logarithmic cultures of E. coli strains were washed and suspended in PBS to a density of 109 cfu/mL (i.e., OD600nm = 1.0). Bacterial cells were added to PBS containing NPN (10 μM) and varying concentrations of colistin in black 96-well microplates. After 1 h of incubation at 37°C, fluorescence was read using an EnSight multimode plate reader (PerkinElmer, Waltham, MA, United States) at 355 nm excitation and 405 nm emission wavelengths. NPN uptake (%) was calculated for each E. coli strain as described elsewhere (Macnair et al., 2018). Full NPN uptake (100%) was achieved by adding 100 mg/L of colistin.
The levels of tigecycline accumulation by mcr-1-positive and -negative E. coli strains in the absence and presence of colistin were determined as our previously described (Chen et al., 2017). Overnight cultures of E. coli strains were diluted to 109 cfu/mL into CAMHB and grown in the same medium for 20 min with 10 mg/L of tigecycline alone and in combination with 2 mg/L of colistin. Bacterial cells were collected by centrifugation at 3000 × g for 10 min, washed with sterile normal saline and dried to obtain the dry weight. Bacteria cells were lysed by sonication for 15 min and then centrifuged at 3000 × g for 10 min to remove the cell debris. Tigecycline concentrations in the resulting cell extracts were determined by a LC-MS/MS method (Sun et al., 2019; details are given in the Supplementary Material). All experiments were performed at least five independent biological replicates. Results were expressed as amount of tigecycline incorporated per dry weight of bacteria.
In vitro time-kill experiments were conducted to characterize the activity of the colistin and tigecycline combination using previously described methods (Rao et al., 2016). In brief, overnight E. coli cultures (∼106 or 108 cfu/mL) were exposed to colistin (2 and 8 mg/L) alone and in combination with tigecycline (0.25 mg/L) over a period of 48 h. The choice of colistin concentrations was based on the clinically achievable serum steady-state concentration (Css) and peak concentration (Cmax) in humans, while the tigecycline concentration was chosen to simulate the average Css at the clinical dose of 50 mg every 12 h (Van Wart et al., 2006; Tran et al., 2016; Nation et al., 2017). Serial samples were obtained at 0, 1, 3, 6, 9, 12, 24, 27, 30, 33, and 48 h after incubation at 37°C. Bacterial counts were determined based on the quantitative cultures on MHA plates. Historical time-kill data of colistin alone for portion of study strains were obtained from our previous report (Zhou et al., 2017).
The concentration-effect curves were used to quantitatively evaluate the potency of colistin and tigecycline combination against E. coli strains harboring blaNDM–5 and mcr-1, at initial inoculums of 106 and 108 cfu/mL, respectively. The testing procedure consisted of four groups, and each group included tubes with twofold increasing concentrations of colistin from 0.5 to 16 mg/L, in the absence and presence of tigecycline at 0.13, 0.25, and 0.5 mg/L. After 48 h of incubation, the microbiological response was measured by the log10 change in bacterial density vs. pre-exposure at 0 h. The relationships between colistin concentrations and antibacterial response to single and combination therapies were fit to the Hill sigmoid Emax model: E = E0 + Emax × CN/(EC50N + CN), where E0 is the log10 change in bacterial count without colistin, Emax is the maximal effect, EC50 is the colistin concentration required to achieve 50% of Emax and N is the slope of concentration-effect curve. The PD analysis was carried out by the non-linear least-squares regression in WinNonlin software Version 6.1 (Pharsight, Sunnyvale, CA, United States) (Zhou et al., 2017). The coefficient of determination (R2) was used to estimate the variance of PD regression analysis. Mann-Whitney test was used to compare the parameters of Emax and EC50 between mcr-1-positive and -negative strains. Differences of PD parameter at 106 vs. 108 cfu/mL inoculum were determined using Wilcoxon signed-rank test in GraphPad Prism 8 software (San Diego, CA, United States) and a P value of <0.05 was considered significant.
All animal experimental protocols were approved by South China Agricultural University (SCAU) Institutional Animal Ethics Committee (Guangzhou, China) and performed in accordance with the SCAU Institutional Laboratory Animal Care and Use guidelines. Six-week-old, 25–27 g, specific-pathogen-free, female ICR mice (Hunan SJA Laboratory Animal, Changsha, China) were rendered neutropenic by administration of cyclophosphamide intraperitoneally as previously described (Zhou et al., 2018). Thigh infections with each E. coli were produced by injecting 0.1 mL of bacterial suspension in normal saline (106.5 and 108.5 cfu/mL). At 2 h after infection, mice were randomized to receive (i) no therapy (control), (ii) colistin at 7.5 mg/kg intraperitoneally (i.p.) twice a day (bid), (iii) tigecycline at 5 mg/kg subcutaneously (s.c.) bid, or (iv) combination of colistin and tigecycline. The current usual doses of colistin (3 MIU, equivalent to 240 mg, every 8 h) and tigecycline (100 mg initially, then 50 mg bid) were acceptable for the treatment of severe infections in humans (Meagher et al., 2005; Docobo-Perez et al., 2012). In this study, the drug doses in mice were selected to mimic the pharmacokinetic profiles of human clinical doses of 300 and 200 mg, respectively (Meagher et al., 2005; Karnik et al., 2013; Zhou et al., 2017; Zhao et al., 2018). Control and antibiotic-treated mice were sacrificed at 24 h after start of therapy. Thigh muscles were aseptically removed, homogenized and bacteria were cultured quantitatively using the plate counting method, and results were expressed as the log10 cfu/thigh. Three mice (i.e., six thighs) were included in each group. The Mann-Whitney U-test was used to compare bacterial densities in target tissue between mono- and combination therapies.
The carbapenem-resistant E. coli strains were highly resistant to almost all tested antibiotics (Table 1). As expected, E. coli strain 2630 lacking mcr-1 was susceptible to colistin, with an MIC of 0.5 mg/L in the absence of tigecycline (Table 1). However, the strains that harbored blaNDM–5 and mcr-1 were resistant both to meropenem (MIC ≥ 16 mg/L) and colistin (MIC ≥ 4 mg/L). Interestingly, colistin MICs for mcr-1-positive CRE strains decreased to 1/4 to 1/16 of the original levels as tigecycline concentration was raised from 0 to 0.5 mg/L (Table 1). This was confirmed using the checkerboard assay that showed synergistic effects of the colistin and tigecycline combination. The FICI values varied from 0.38 to 0.5 for all except the colistin susceptible strain 2630 (Table 1). In particular, E. coli 1320 that carried both blaNDM–5 and mcr-1 displayed a highly significant synergistic response to this combination across the range of drug concentrations tested, with a clear peak at 0.5 mg/L tigecycline and 1 or 2 mg/L colistin (Figure 2A). Different degrees of synergy were observed for all study E. coli strains with synergy volumes that ranged from 36.9 to 183 μM2 (Figure 2B).
Table 1. Genotype summary, in vitro antimicrobial susceptibility profiles, and MICs of colistin in the absence and presence of tigecycline at 0.13, 0.25, and 0.5 mg/L against study E. coli strainsa.
Figure 2. In vitro interactions between colistin and tigecycline. (A,B) Synergism as demonstrated using MacSynergy II plots of the three-dimensional dose-response curves. The flat plane represents the predicted indifference between antagonism and synergy. Peaks and troughs represent synergy and antagonism, respectively. Synergy expressed as the calculated interaction volumes (μM2) at a confidence interval of 95%: <25, additive; 25 to 50, minor but significant; 50 to 100, moderate; and >100, strong synergy. (C) Colistin-induced NPN uptake (%) of mcr-1-positive and -negative E. coli strains. The data represents background subtracted fluorescence divided by the fluorescence observed at 100 mg/L of colistin. (D) Accumulations of tigecycline in E. coli strains (dry weight) after exposure to 10 mg/L tigecycline for 20 min in the presence and absence of colistin. Data shown are the means of five independent biological replicates. ∗P < 0.05; ∗∗P < 0.01; and ∗∗∗P < 0.001.
Carriage of mcr-1 in carbapenem-resistant E. coli strains increased their resistance to colistin-induced outer-membrane disruption as expected. NPN uptake in mcr-1-harboring E. coli was significantly less than E. coli 2630 after exposure to colistin at 0.78 to 12.5 mg/L (Figure 2C; P < 0.05), with corresponding colistin MIC increases from 8- to 16-fold (Table 1). The colistin concentrations required to achieve the comparable levels of NPN uptake increased eightfold in mcr-1-positive compared to -negative E. coli strains. For example, 45% of NPN uptake was observed at 0.78 mg/L colistin for colistin-susceptible E. coli 2630, while similar NPN uptake (38% to 53%) occurred at 6.25 mg/L colistin for mcr-1-harboring strains (Figure 2C). It seems that the additional levels of outer-membrane perturbation in a colistin-susceptible strain can be achieved by increasing the concentration of colistin eightfold in mcr-1-harboring E. coli strains. Importantly, when combined with the clinically relevant concentration of colistin at 2 mg/L, intracellular accumulations of tigecycline markedly increased in all study E. coli strains (P < 0.01; Figure 2D). Although the concentration of 2 mg/L colistin alone was insufficient to inhibit growth of E. coli harboring both blaNDM–5 and mcr-1 (Figures 3H-J), it provided sufficient outer-membrane perturbation to facilitate tigecycline uptake and subsequent tigecycline-induced growth inhibition (Figure 2D).
Figure 3. Combinatorial bactericidal activity of colistin and tigecycline against mcr-1-positive and -negative E. coli strains harboring blaNDM–5. (A–L) In vitro time-kill experiments of colistin (2 and 8 mg/L) alone and in combination with tigecycline (0.25 mg/L) against all study E. coli strains at low and high inoculums over 48 h. The horizontal dotted line represents the limit of detection for bacterial count (40 cfu/mL). Historical time-kill data of colistin alone for potion of strains was obtained from our previous study (Zhou et al., 2017). (M,N) The concentration-effect profiles of colistin against E. coli strains harboring both blaNDM–5 and mcr-1 (i.e., 1320, 2610, and 2121) at low (M) and high (N) inoculums following treatment with colistin (0–16 mg/L) at fixed concentrations of tigecycline (0–0.5 mg/L). Each symbol represents the log10 change in bacterial burdens over a 48 h study period. Data points below the line represent killing and points above the line represent growth.
At a low inoculum (106 cfu/mL), colistin alone at 2 mg/L achieved complete the bactericidal activity (>6.3-log10 reduction) over 24 h against colistin-susceptible strain 2630. The activity was not further improved at higher colistin levels or in combination with tigecycline (Figure 3B). Against the colistin-resistant E. coli 1320, the clinically achievable concentrations of colistin resulted in early bactericidal activity only, with a 1.3- to 3.2-log10 reduction in bacterial density, followed by rapid regrowth beyond 6 h. However, complete bacterial eradication was attained with the combination of 8 mg/L colistin and 0.25 mg/L tigecycline (Figure 3D). Similarly, in the presence of 0.25 mg/L tigecycline, substantial killing of E. coli 2610 was achieved with >2 mg/L colistin (Figure 3I). Interestingly, despite the lack of activity that was observed for all colistin monotherapies against E. coli 2121, tigecycline displayed the ability to increase killing activity over 48 h of exposure to colistin (Figure 3J).
Monotherapy with a high colistin concentration (8 mg/L) or the combination of 0.25 mg/L tigecycline and 2 mg/L colistin exhibited sustained bactericidal activity at the high inoculum (108 cfu/mL) of E. coli 2630 (Figure 3F). However, even the high colistin levels of 8 mg/L were inactive for the colistin-resistant strains, whereas in combination with 0.25 mg/L tigecycline resulted in a 2.1- to 3.9-log10 reduction in bacterial density (Figures 3H,K–L). Tigecycline monotherapy at 0.06 or 0.25 mg/L performed no different from the growth control against all study E. coli at both low and high inoculums (Figure 3).
The concentration-effect relationship was fitted to a Hill-type equation (R2 > 0.95), and the PD parameter of EC50 representing colistin potency was significantly greater in mcr-1-harboring strains compared with E. coli 2630 (P < 0.01; Table 2). In addition, the EC50 values at 108 cfu/mL inoculum were 1.5- to 18.4-times higher than those at 106 cfu/mL inoculum (mean = 5.3, P < 0.001). In the three strains that harbored blaNDM–5 and mcr-1, a clear tendency toward higher Emax values were seen with a 108 cfu/mL inoculum, whereas no significant difference was noted at 106 cfu/mL (Table 2).
Table 2. Hill PD parameters describing the concentration-response profiles of colistin (0–16 mg/L) in the presence of fixed tigecycline concentrations (0–0.5 mg/L) at low and high inoculumsa.
Overall, we found similar dose-dependent shifts with increasing tigecycline levels to a lower colistin concentration required to suppress the growth of E. coli at both inoculums (Figures 3M,N). For example, at 106 cfu/mL, inhibition of E. coli 2630 occurred at the colistin concentration of 0.75 mg/L and decreased threefold to 0.25 mg/L in the presence of tigecycline (Supplementary Figure S1C). Carriage of mcr-1 increased the colistin concentration required for growth inhibition to 8 mg/L, which was 11-fold greater than for E. coli 2630 (Figure 3M). However, in combination with tigecycline from 0.13 to 0.5 mg/L, the colistin levels for growth inhibition were only 0.75 mg/L or twofold and fourfold greater than the concentration needed to synergize with tigecycline against E. coli 2630 (Figure 3M and Supplementary Figure S1C). It seems that the mcr-1 gene only provided protection against colistin monotherapy, but not an ability to resist the colistin and tigecycline combination therapy.
During thigh infection with a low initial burden, colistin monotherapy led to decreased bacterial density by 1.62-log10cfu/thigh for colistin-susceptible E. coli 2630, compared to the untreated control at 0 h (Figure 4B). However, for colistin-resistant strains, neither colistin nor tigecycline monotherapy showed a significant reduction in bacterial density after 24 h of therapy. Interestingly, colistin and tigecycline combination proved efficacious, resulting in >2.0 log10cfu/thigh reduction compared to each monotherapy (P < 0.0001, Mann-Whitney U-test; Figures 4C–F). The high initial burden in the murine thigh infection model was used to stimulate the severe infections that result in high mortality, and the effectiveness of combination therapy is a general proof of principle. Monotherapy with colistin or tigecycline did not achieve notable antibacterial effects against E. coli harboring blaNDM–5 and mcr-1 at the high initial inoculum (Figure 5). Importantly, the combination of colistin and tigecycline significantly increased killing activity at 24 h by 1.1- to 2.7- log10cfu/thigh reduction in bacterial density compared to control at 0 h or >2.5-log10cfu/thigh compared to each monotherapy (P < 0.0005, Mann-Whitney U-test; Figures 5D–F).
Figure 4. Efficacy of colistin and tigecycline mono- and combination therapies at 24 h against E. coli ATCC 25922 (A), 2630 (B), 3112 (C) and strains harboring blaNDM–5 and mcr-1 (D–F) in the murine thigh infection model with a low initial burden of 106 cfu/thigh. Colistin (7.5 mg/kg i.p. bid) and tigecycline (5 mg/kg s.c. bid) and the combination were administrated at 2 h post-infection. Horizontal lines represent the mean and standard deviation of bacterial densities for each group (n = 6). Colistin and tigecycline combination therapy resulted in a >2.0 log10cfu/thigh reduction relative to each monotherapy (P < 0.0001, Mann-Whitney U-test).
Figure 5. Efficacy of colistin and tigecycline mono- and combination therapies at 24 h against E. coli ATCC 25922 (A), 2630 (B), 3112 (C) and strains harboring blaNDM–5 and mcr-1 (D–F) in the murine thigh infection model with a high initial burden of 108 cfu/thigh. Colistin (7.5 mg/kg i.p. bid) and tigecycline (5 mg/kg s.c. bid) and the combination were administrated at 2 h post-infection. Horizontal lines represent the mean and standard deviation of bacterial densities for each group (n = 6). Colistin and tigecycline combination therapy resulted in a >2.5 log10cfu/thigh reduction relative to each monotherapy (P < 0.0005, Mann-Whitney U-test).
Treatment options for carbapenem-resistant E. coli infections are very limited especially if the mcr-1 gene is also present in the infecting strains. Tigecycline and colistin are currently the last-resort antibiotics for the treatment of severe infections (Sun et al., 2019). However, tigecycline demonstrates mainly bacteriostatic activity with low serum levels (Van Wart et al., 2006). Concerns have been raised regarding the efficacy of tigecycline monotherapy in the light of decreased clinical success rates (Yahav et al., 2011). Indeed, in the current study, tigecycline monotherapy did not achieve positive outcomes in a murine thigh infection model when the study E. coli strains harbored both blaNDM–5 and mcr-1, despite the fact that most of strains (5/6) remained susceptible to tigecycline except the strain 1320. Fortunately, the presence of mcr-1 only slightly increased the MIC of colistin (Zhou et al., 2017). Consequently, there was a compelling reason to use colistin and tigecycline in combination.
Colistin and tigecycline combination therapy against CRE infection had varying outcomes from synergy to indifference (Bercot et al., 2011; Karaoglan et al., 2013; Rao et al., 2016; Cai et al., 2017; Ku et al., 2017). In this study, combination of clinically achievable concentration of colistin and tigecycline produced a synergistic activity in vitro against E. coli harboring blaNDM–5 and mcr-1, resulting in a >4.0-log10cfu/mL reduction by 48 h. An additional in vivo synergistic effect was indeed observed in the murine thigh model, at both low and high inoculums. Supporting our findings, colistin displayed a similar synergistic interaction with tigecycline for carbapenem-resistant A. baumannii and K. pneumoniae (Pournaras et al., 2011; Karaoglan et al., 2013; Ku et al., 2017). Data from previous case reports also showed beneficial activity of tigecycline and colistin combination therapy against K. pneumoniae bacteremia (Cobo et al., 2008). Interestingly, the higher dose of tigecycline has been shown to be associated with better synergistic outcomes against multidrug-resistant CRE, compared with the conventional dosing regimen (De Pascale et al., 2014; Cai et al., 2017). In contrast, a potential trend toward antagonism was observed at lower tigecycline concentrations (Albur et al., 2012). Of note, previous studies that used this combination employed different methods, and the isolates were not well-described genotypically, making the results difficult to generalize. Here, we demonstrated increased activity of colistin in combination tigecycline against E. coli strains that harbored blaNDM–5 and mcr-1, including the pandemic clonal complex ST648 (Hornsey et al., 2011). The clinical impact of infections due to colistin-resistant NDM-5-producing E. coli is currently unknown, but our findings provide an alternative approach to combat such resistant strains. In support of this view, a recent report indicated that colistin and tigecycline combination was able to prevent the emergence of high-level resistance to these antibiotics (Cai et al., 2017).
The potentiation effect of this combination is most likely related to their different mechanisms of action at separate bacterial targets. Tigecycline acts in the cytoplasm by binding to the ribosomal complex that requires drug to enter the bacterial cells first (Bauer et al., 2004). In general, uptake of tigecycline across the bacterial cell wall and cytoplasmic membrane includes two ways: passive diffusion and an energy-dependent active transport system (Schnappinger and Hillen, 1996; Chopra and Roberts, 2001). In Gram-negative bacteria, the cell wall is surrounded by the outer-membrane and tigecycline moves through membranes via porin channels in the absence of colistin (Roberts, 2003). Colistin resulted in bacterial outer-membrane disruption and instable regions in cytoplasmic membrane that may facilitate tigecycline passive accumulation (Macnair et al., 2018). Supporting this speculation, our NPN uptake and intracellular tigecycline accumulation assays demonstrated that exposure to colistin did promote tigecycline uptake and subsequent tigecycline-induced growth inhibition independent of mcr-1 expression. This scenario has been reported for colistin in combination with minocycline, the prodrug of tigecycline (Liang et al., 2011). However, the precise details of how colistin affects the energy-dependent transport of tigecycline still remain unclear.
Owing to the paucity of novel antibiotics, colistin-based combination therapy was therefore regarded as an alternative approach to combat colistin-resistant CRE infections. A synergistic effect of colistin with amikacin, rifampicin, and osthole has been reported (Lagerback et al., 2016; Liu X. et al., 2016; Zhou et al., 2017, 2019). However, systemic administration of colistin is associated with nephrotoxicity despite the fact that toxicity is dose-dependent and reversible on discontinuation of treatment (Biswas et al., 2012). Therefore, the clinical utility of colistin should be prudent when used in combination with other nephrotoxic antibiotics such as gentamicin and amikacin. Previous nephrotoxicity studies in mice indicated that only mild kidney damage was observed until an accumulated dose of 72 mg/kg colistin, and suggested an acceptable colistin single dose ranges within 40 mg/kg in mice (Cheah et al., 2015; Roberts et al., 2015). Therefore, the much lower colistin dose (7.5 mg/kg) that used in this study should be safe for mice by comparison. In fact, many previous studies have employed 7.5 mg/kg colistin to carry out in vivo efficacy studies in mice (Liu et al., 2016; Zhou et al., 2017; Macnair et al., 2018). In the present study, tigecycline demonstrated bactericidal activity against E. coli harboring blaNDM–5 and mcr-1 when combined with the clinically relevant concentration of colistin at 2 mg/L, which is considered as the appropriate partnered concentration to avoid renal impairment (Tran et al., 2016). Importantly, the combination of tigecycline with colistin we studied here may allow lower colistin dose sparing regimens that reduce nephrotoxicity for treating colistin-resistant CRE infections. Previous comparative observational studies also showed a lower-than-expected toxicity for tigecycline and colistin combination therapy (Zhang et al., 2013). Even patients with kidney disease could benefit from colistin-based combination therapy, when provided with a lower daily dose of colistin achieving comparable efficacy (Falagas et al., 2006; Biswas et al., 2012). In addition, a retrospective cohort study indicated that colistin is a valuable antibiotic with acceptable nephrotoxicity (<7%) and considerable efficacy that depends on daily dose (Falagas et al., 2010).
Our investigation has several limitations. For example, the combination was evaluated in a small number of strains despite the different clonal types. In addition, the murine thigh model is a local infection model, and additional study is needed to evaluate the usefulness of this combination in the clinical setting. Moreover, based on our current results, we do not know whether the colistin-induced increased accumulation of tigecycline in bacterial cells is “drug specific” or more broad range for other antibiotics. Although this is beyond the scope of this study, future studies should examine this potential mechanism.
In summary, this study demonstrated increased activity of colistin and tigecycline combination against E. coli harboring blaNDM–5 and mcr-1. Importantly, a potentiation effect occurred at the clinically relevant concentrations of colistin and tigecycline, and was efficacious in the murine thigh infection model. In addition, we demonstrated for the first time that colistin permeabilization of the bacterial outer-membrane facilitates the uptake of tigecycline, contributing to increased activity of the combination.
The raw data supporting the conclusions of this article will be made available by the authors, without undue reservation, to any qualified researcher.
This study was carried out in accordance with the recommendations of ethical guidelines of South China Agricultural University. All animal experimental protocols and isolation procedures for E. coli strains were reviewed and approved by the South China Agricultural University Institutional Animal Ethics Committee (2019B161 and 2018B095). Individual written informed consent for the use of isolates was obtained.
Y-HL and Y-FZ designed the study and wrote the manuscript. Y-FZ, PL, and C-JZ carried out the experiments. JS and X-PL analyzed the data. All authors read and approved the final manuscript.
This study was supported by the National Key Research and Development Program of China (2016YFD0501300), the National Natural Science Foundation of China (31730097 and 31772793), the Program of Changjiang Scholars and Innovative Research Team in University of Ministry of Education of China (IRT_17R39), and the Foundation for Innovation and Strengthening School Project of Guangdong, China (2016KCXTD010).
The authors declare that the research was conducted in the absence of any commercial or financial relationships that could be construed as a potential conflict of interest.
The Supplementary Material for this article can be found online at: https://www.frontiersin.org/articles/10.3389/fmicb.2019.02957/full#supplementary-material
Albur, M., Noel, A., Bowker, K., and Macgowan, A. (2012). Bactericidal activity of multiple combinations of tigecycline and colistin against NDM-1-producing Enterobacteriaceae. Antimicrob. Agents Chemother. 56, 3441–3443. doi: 10.1128/AAC.05682-11
Bauer, G., Berens, C., Projan, S. J., and Hillen, W. (2004). Comparison of tetracycline and tigecycline binding to ribosomes mapped by dimethylsulphate and drug-directed Fe2+ cleavage of 16S rRNA. J. Antimicrob. Chemother. 53, 592–599. doi: 10.1093/jac/dkh125
Bercot, B., Poirel, L., Dortet, L., and Nordmann, P. (2011). In vitro evaluation of antibiotic synergy for NDM-1-producing Enterobacteriaceae. J. Antimicrob. Chemother. 66, 2295–2297. doi: 10.1093/jac/dkr296
Biswas, S., Brunel, J. M., Dubus, J. C., Reynaud-Gaubert, M., and Rolain, J. M. (2012). Colistin: an update on the antibiotic of the 21st century. Expert Rev. Anti Infect. Ther. 10, 917–934. doi: 10.1586/eri.12.78
Buyck, J. M., Plesiat, P., Traore, H., Vanderbist, F., Tulkens, P. M., and Van Bambeke, F. (2012). Increased susceptibility of Pseudomonas aeruginosa to macrolides and ketolides in eukaryotic cell culture media and biological fluids due to decreased expression of oprM and increased outer-membrane permeability. Clin. Infect. Dis. 55, 534–542. doi: 10.1093/cid/cis473
Cai, X., Yang, Z., Dai, J., Chen, K., Zhang, L., Ni, W., et al. (2017). Pharmacodynamics of tigecycline alone and in combination with colistin against clinical isolates of multidrug-resistant Acinetobacter baumannii in an in vitro pharmacodynamic model. Int. J. Antimicrob. Agents 49, 609–616. doi: 10.1016/j.ijantimicag.2017.01.007
Cheah, S. E., Wang, J., Nguyen, V. T., Turnidge, J. D., Li, J., and Nation, R. L. (2015). New pharmacokinetic/pharmacodynamic studies of systemically administered colistin against Pseudomonas aeruginosa and Acinetobacter baumannii in mouse thigh and lung infection models: smaller response in lung infection. J. Antimicrob. Chemother. 70, 3291–3297. doi: 10.1093/jac/dkv267
Chen, Y., Hu, D., Zhang, Q., Liao, X. P., Liu, Y. H., and Sun, J. (2017). Efflux Pump overexpression contributes to tigecycline heteroresistance in Salmonella enterica serovar Typhimurium. Front. Cell Infect. Microbiol. 7:37. doi: 10.3389/fcimb.2017.00037
Chopra, I., and Roberts, M. (2001). Tetracycline antibiotics: mode of action, applications, molecular biology, and epidemiology of bacterial resistance. Microbiol. Mol. Biol. Rev. 65, 232–260. doi: 10.1128/mmbr.65.2.232-260.2001
Cobo, J., Morosini, M. I., Pintado, V., Tato, M., Samaranch, N., Baquero, F., et al. (2008). Use of tigecycline for the treatment of prolonged bacteremia due to a multiresistant VIM-1 and SHV-12 beta–lactamase-producing Klebsiella pneumoniae epidemic clone. Diagn. Microbiol. Infect. Dis. 60, 319–322. doi: 10.1016/j.diagmicrobio.2007.09.017
De Pascale, G., Montini, L., Pennisi, M., Bernini, V., Maviglia, R., Bello, G., et al. (2014). High dose tigecycline in critically ill patients with severe infections due to multidrug-resistant bacteria. Crit. Care 18:R90. doi: 10.1186/cc13858
Deshpande, D., Srivastava, S., Nuermberger, E., Pasipanodya, J. G., Swaminathan, S., and Gumbo, T. (2016). Concentration-dependent synergy and antagonism of linezolid and moxifloxacin in the treatment of childhood tuberculosis: the dynamic duo. Clin. Infect. Dis. 63, s88–s94. doi: 10.1093/cid/ciw473
Docobo-Perez, F., Nordmann, P., Dominguez-Herrera, J., Lopez-Rojas, R., Smani, Y., Poirel, L., et al. (2012). Efficacies of colistin and tigecycline in mice with experimental pneumonia due to NDM-1-producing strains of Klebsiella pneumoniae and Escherichia coli. Int. J. Antimicrob. Agents 39, 251–254. doi: 10.1016/j.ijantimicag.2011.10.012
Du, H., Chen, L., Tang, Y. W., and Kreiswirth, B. N. (2016). Emergence of the mcr-1 colistin resistance gene in carbapenem-resistant Enterobacteriaceae. Lancet Infect. Dis. 16, 287–288. doi: 10.1016/s1473-3099(16)00056-6
Falagas, M. E., Lourida, P., Poulikakos, P., Rafailidis, P. I., and Tansarli, G. S. (2014). Antibiotic treatment of infections due to carbapenem-resistant Enterobacteriaceae: systematic evaluation of the available evidence. Antimicrob. Agents Chemother. 58, 654–663. doi: 10.1128/AAC.01222-13
Falagas, M. E., Rafailidis, P. I., Ioannidou, E., Alexiou, V. G., Matthaiou, D. K., Karageorgopoulos, D. E., et al. (2010). Colistin therapy for microbiologically documented multidrug-resistant Gram-negative bacterial infections: a retrospective cohort study of 258 patients. Int. J. Antimicrob. Agents 35, 194–199. doi: 10.1016/j.ijantimicag.2009.10.005
Falagas, M. E., Rafailidis, P. I., Kasiakou, S. K., Hatzopoulou, P., and Michalopoulos, A. (2006). Effectiveness and nephrotoxicity of colistin monotherapy vs. colistin-meropenem combination therapy for multidrug-resistant Gram-negative bacterial infections. Clin. Microbiol. Infect. 12, 1227–1230. doi: 10.1111/j.1469-0691.2006.01559.x
Hornsey, M., Phee, L., and Wareham, D. W. (2011). A novel variant, NDM-5, of the New Delhi metallo-beta-lactamase in a multidrug-resistant Escherichia coli ST648 isolate recovered from a patient in the United Kingdom. Antimicrob. Agents Chemother. 55, 5952–5954. doi: 10.1128/AAC.05108-11
Karaoglan, I., Zer, Y., Bosnak, V. K., Mete, A. O., and Namiduru, M. (2013). In vitro synergistic activity of colistin with tigecycline or beta-lactam antibiotic/beta-lactamase inhibitor combinations against carbapenem-resistant Acinetobacter baumannii. J. Int. Med. Res. 41, 1830–1837. doi: 10.1177/0300060513496172
Karnik, N. D., Sridharan, K., Jadhav, S. P., Kadam, P. P., Naidu, R. K., Namjoshi, R. D., et al. (2013). Pharmacokinetics of colistin in critically ill patients with multidrug-resistant Gram-negative bacilli infection. Eur. J. Clin. Pharmacol. 69, 1429–1436. doi: 10.1007/s00228-013-1493-9
Ku, Y. H., Chen, C. C., Lee, M. F., Chuang, Y. C., Tang, H. J., and Yu, W. L. (2017). Comparison of synergism between colistin, fosfomycin and tigecycline against extended-spectrum beta-lactamase-producing Klebsiella pneumoniae isolates or with carbapenem resistance. J. Microbiol. Immunol. Infect. 50, 931–939. doi: 10.1016/j.jmii.2016.12.008
Lagerback, P., Khine, W. W., Giske, C. G., and Tangden, T. (2016). Evaluation of antibacterial activities of colistin, rifampicin and meropenem combinations against NDM-1-producing Klebsiella pneumoniae in 24 h in vitro time-kill experiments. J. Antimicrob. Chemother. 71, 2321–2325. doi: 10.1093/jac/dkw213
Lai, Y. W., Campbell, L. T., Wilkins, M. R., Pang, C. N., Chen, S., and Carter, D. A. (2016). Synergy and antagonism between iron chelators and antifungal drugs in Cryptococcus. Int. J. Antimicrob. Agents 48, 388–394. doi: 10.1016/j.ijantimicag.2016.06.012
Liang, W., Liu, X. F., Huang, J., Zhu, D. M., Li, J., and Zhang, J. (2011). Activities of colistin- and minocycline-based combinations against extensive drug resistant Acinetobacter baumannii isolates from intensive care unit patients. BMC Infect. Dis. 11:109. doi: 10.1186/1471-2334-11-109
Liu, S., Zhang, J., Zhou, Y., Hu, N., Li, J., Wang, Y., et al. (2019). Pterostilbene restores carbapenem susceptibility in NDM-producing isolates via inhibiting the activity of NDM enzymes. Br. J. Pharmacol. [Epub ahead of print].
Liu, X., Zhao, M., Chen, Y., Bian, X., Li, Y., Shi, J., et al. (2016). Synergistic killing by meropenem and colistin combination of carbapenem-resistant Acinetobacter baumannii isolates from Chinese patients in an in vitro pharmacokinetic/pharmacodynamic model. Int. J. Antimicrob. Agents 48, 559–563. doi: 10.1016/j.ijantimicag.2016.07.018
Liu, Y. Y., Wang, Y., Walsh, T. R., Yi, L. X., Zhang, R., Spencer, J., et al. (2016). Emergence of plasmid-mediated colistin resistance mechanism MCR-1 in animals and human beings in China: a microbiological and molecular biological study. Lancet Infect. Dis. 16, 161–168. doi: 10.1016/S1473-3099(15)00424-7
Macnair, C. R., Stokes, J. M., Carfrae, L. A., Fiebig-Comyn, A. A., Coombes, B. K., Mulvey, M. R., et al. (2018). Overcoming mcr-1 mediated colistin resistance with colistin in combination with other antibiotics. Nat. Commun. 9:458. doi: 10.1038/s41467-018-02875-z
Meagher, A. K., Ambrose, P. G., Grasela, T. H., and Ellis-Grosse, E. J. (2005). The pharmacokinetic and pharmacodynamic profile of tigecycline. Clin. Infect. Dis. 41(Suppl. 5), S333–S340.
Nation, R. L., Garonzik, S. M., Thamlikitkul, V., Giamarellos-Bourboulis, E. J., Forrest, A., Paterson, D. L., et al. (2017). Dosing guidance for intravenous colistin in critically-ill patients. Clin. Infect. Dis. 64, 565–571. doi: 10.1093/cid/ciw839
Perez, F., and Bonomo, R. A. (2018). Evidence to improve the treatment of infections caused by carbapenem-resistant Gram-negative bacteria. Lancet Infect. Dis. 18, 358–360. doi: 10.1016/s1473-3099(18)30112-9
Pournaras, S., Vrioni, G., Neou, E., Dendrinos, J., Dimitroulia, E., Poulou, A., et al. (2011). Activity of tigecycline alone and in combination with colistin and meropenem against Klebsiella pneumoniae carbapenemase (KPC)-producing Enterobacteriaceae strains by time-kill assay. Int. J. Antimicrob. Agents 37, 244–247. doi: 10.1016/j.ijantimicag.2010.10.031
Prichard, M. N., and Shipman, C. Jr. (1990). A three-dimensional model to analyze drug-drug interactions. Antiviral Res. 14, 181–205. doi: 10.1016/0166-3542(90)90001-n
Rao, G. G., Ly, N. S., Diep, J., Forrest, A., Bulitta, J. B., Holden, P. N., et al. (2016). Combinatorial pharmacodynamics of polymyxin B and tigecycline against heteroresistant Acinetobacter baumannii. Int. J. Antimicrob. Agents 48, 331–336. doi: 10.1016/j.ijantimicag.2016.06.006
Roberts, K. D., Azad, M. A., Wang, J., Horne, A. S., Thompson, P. E., Nation, R. L., et al. (2015). Antimicrobial activity and toxicity of the major lipopeptide components of polymyxin b and colistin: last-line antibiotics against multidrug-resistant gram-negative bacteria. ACS Infect. Dis. 1, 568–575. doi: 10.1021/acsinfecdis.5b00085
Roberts, M. C. (2003). Tetracycline therapy: update. Clin. Infect. Dis. 36, 462–467. doi: 10.1086/367622
Schnappinger, D., and Hillen, W. (1996). Tetracyclines: antibiotic action, uptake, and resistance mechanisms. Arch. Microbiol. 165, 359–369. doi: 10.1007/s002030050339
Sun, J., Chen, C., Cui, C. Y., Zhang, Y., Liu, X., Cui, Z. H., et al. (2019). Plasmid-encoded tet(X) genes that confer high-level tigecycline resistance in Escherichia coli. Nat. Microbiol. 4, 1457–1464. doi: 10.1038/s41564-019-0496-4
Sun, J., Li, X. P., Yang, R. S., Fang, L. X., Huo, W., Li, S. M., et al. (2016a). Complete nucleotide sequence of an IncI2 plasmid coharboring blaCTX-M-55 and mcr-1. Antimicrob. Agents Chemother. 60, 5014–5017. doi: 10.1128/AAC.00774-16
Sun, J., Yang, R. S., Zhang, Q., Feng, Y., Fang, L. X., Xia, J., et al. (2016b). Co-transfer of blaNDM-5 and mcr-1 by an IncX3-X4 hybrid plasmid in Escherichia coli. Nat. Microbiol. 1:16176. doi: 10.1038/nmicrobiol.2016.176
Sun, J., Zhang, H., Liu, Y. H., and Feng, Y. (2018). Towards understanding MCR-like colistin resistance. Trends Microbiol. 26, 794–808. doi: 10.1016/j.tim.2018.02.006
Tran, T. B., Velkov, T., Nation, R. L., Forrest, A., Tsuji, B. T., Bergen, P. J., et al. (2016). Pharmacokinetics/pharmacodynamics of colistin and polymyxin B: are we there yet? Int. J. Antimicrob. Agents 48, 592–597. doi: 10.1016/j.ijantimicag.2016.09.010
Van Wart, S. A., Owen, J. S., Ludwig, E. A., Meagher, A. K., Korth-Bradley, J. M., and Cirincione, B. B. (2006). Population pharmacokinetics of tigecycline in patients with complicated intra-abdominal or skin and skin structure infections. Antimicrob. Agents Chemother. 50, 3701–3707. doi: 10.1128/aac.01636-05
Wiegand, I., Hilpert, K., and Hancock, R. E. (2008). Agar and broth dilution methods to determine the minimal inhibitory concentration (MIC) of antimicrobial substances. Nat. Protoc. 3, 163–175. doi: 10.1038/nprot.2007.521
Yahav, D., Lador, A., Paul, M., and Leibovici, L. (2011). Efficacy and safety of tigecycline: a systematic review and meta-analysis. J. Antimicrob. Chemother. 66, 1963–1971. doi: 10.1093/jac/dkr242
Yao, X., Doi, Y., Zeng, L., Lv, L., and Liu, J. H. (2016). Carbapenem-resistant and colistin-resistant Escherichia coli co-producing NDM-9 and MCR-1. Lancet Infect. Dis. 16, 288–289. doi: 10.1016/s1473-3099(16)00057-8
Zhang, H. Z., Zhang, J. S., and Qiao, L. (2013). The Acinetobacter baumannii group: a systemic review. World J. Emerg. Med. 4, 169–174. doi: 10.5847/wjem.j.1920-8642.2013.03.002
Zhao, M., Wu, X. J., Fan, Y. X., Zhang, Y. Y., Guo, B. N., Yu, J. C., et al. (2018). Pharmacokinetics of colistin methanesulfonate (CMS) in healthy Chinese subjects after single and multiple intravenous doses. Int. J. Antimicrob. Agents 51, 714–720. doi: 10.1016/j.ijantimicag.2017.12.025
Zhou, Y., Wang, J., Guo, Y., Liu, X., Liu, S., Niu, X., et al. (2019). Discovery of a potential MCR-1 inhibitor that reverses polymyxin activity against clinical mcr-1-positive Enterobacteriaceae. J. Infect. 78, 364–372. doi: 10.1016/j.jinf.2019.03.004
Zhou, Y. F., Tao, M. T., Feng, Y., Yang, R. S., Liao, X. P., Liu, Y. H., et al. (2017). Increased activity of colistin in combination with amikacin against Escherichia coli co-producing NDM-5 and MCR-1. J. Antimicrob. Chemother. 72, 1723–1730. doi: 10.1093/jac/dkx038
Zhou, Y. F., Tao, M. T., He, Y. Z., Sun, J., Liu, Y. H., and Liao, X. P. (2018). In Vivo bioluminescent monitoring of therapeutic efficacy and pharmacodynamic target assessment of antofloxacin against Escherichia coli in a neutropenic murine thigh infection model. Antimicrob. Agents Chemother. 62:e01281-17. doi: 10.1128/AAC.01281-17
Keywords: carbapenem-resistant Enterobacteriaceae, carbapenem-resistance, colistin-resistance, combination therapy, MCR-1, New Delhi metallo-β-lactamases-5
Citation: Zhou Y-F, Liu P, Zhang C-J, Liao X-P, Sun J and Liu Y-H (2020) Colistin Combined With Tigecycline: A Promising Alternative Strategy to Combat Escherichia coli Harboring blaNDM–5 and mcr-1. Front. Microbiol. 10:2957. doi: 10.3389/fmicb.2019.02957
Received: 12 September 2019; Accepted: 09 December 2019;
Published: 08 January 2020.
Edited by:
Gian Maria Rossolini, University of Florence, ItalyReviewed by:
Jianfeng Wang, Jilin University, ChinaCopyright © 2020 Zhou, Liu, Zhang, Liao, Sun and Liu. This is an open-access article distributed under the terms of the Creative Commons Attribution License (CC BY). The use, distribution or reproduction in other forums is permitted, provided the original author(s) and the copyright owner(s) are credited and that the original publication in this journal is cited, in accordance with accepted academic practice. No use, distribution or reproduction is permitted which does not comply with these terms.
*Correspondence: Ya-Hong Liu, bHloQHNjYXUuZWR1LmNu
Disclaimer: All claims expressed in this article are solely those of the authors and do not necessarily represent those of their affiliated organizations, or those of the publisher, the editors and the reviewers. Any product that may be evaluated in this article or claim that may be made by its manufacturer is not guaranteed or endorsed by the publisher.
Research integrity at Frontiers
Learn more about the work of our research integrity team to safeguard the quality of each article we publish.