- Nosopharm, Nîmes, France
A major issue currently facing medicine is antibiotic resistance. No new class of antibiotics for the treatment of Gram-negative infections has been introduced in more than 40 years. We screened a collection of Xenorhabdus and Photorhabdus strains in the quest to discover new structures that are active against the most problematic multidrug-resistant bacteria. These species are symbiotic bacteria of entomopathogenic nematodes and their life cycle, the richness of the bacteria’s genome in non-ribosomal peptide synthetase (NRPS) and polyketide synthase (PKS) genes, and their propensity to produce secondary metabolites with a large diversity of chemical structures make them a good starting point to begin an ambitious drug discovery program. Odilorhabdins (ODLs), a novel antibacterial class, were identified from this campaign. These compounds inhibit bacterial translation by binding to the small ribosomal subunit at a site not exploited by current antibiotics. Following the development of the total synthesis of this family of peptides, a medicinal chemistry program was started to optimize their pharmacological properties. NOSO-502, the first ODL preclinical candidate was selected. This compound is currently under preclinical development for the treatment of multidrug-resistant Gram-negative infections in hospitalized patients.
Introduction
The efforts of the pharmaceutical industry to generate new highly potent antibiotics with novel mechanisms of action have weakened dramatically over the last three decades for economic, scientific, or strategic reasons, resulting in the decline of the discovery of new classes of antibacterials. The rapid emergence of resistant bacteria combined with the absence of new drugs has led clinicians to a therapeutic impasse, especially in intensive care units. It is thus urgent to find new antibiotic chemical scaffolds to renew the current therapeutic arsenal. Natural products have historically been of crucial importance in the identification and development of antibacterial agents (Clardy et al., 2006). Indeed, most antibiotics in clinical use or advanced development come from secondary metabolites that were originally isolated from bacteria or fungi, such as penicillin, isolated from the fungus Penicillium, or tetracycline found in the soil-dwelling bacteria Streptomyces aureofaciens. Historically, Actinomycetes have been the most important source for the discovery of new antibiotics. Many drugs used today in clinical practice originate from metabolites produced by Actinomycetes, (Genilloud, 2017) such as chloramphenicol (producing strain: Streptomyces venezuelae), daptomycin (Streptomyces roseoporus), erythromycin (Saccharopolyspora erythraea), gentamicin (Micromonospora purpurea), lincomycin (Streptomyces lincolnensis), rifamycin (Amycolatopsis mediterranei), streptomycin (Streptomyces griseus), tetracycline (S. aureofaciens), and vancomycin (Amycolatopsis orientalis). Although Actinomycetes are still one of the most important sources for novel structures, (Genilloud, 2017) it is equally important to explore new underexploited microbial bioresources, as shown by the recent characterization of two highly promising antibiotics with novel mechanisms of action. Teixobactin was isolated from uncultured Eleftheria terrae, (Ling et al., 2015) and Odilorhabdins (ODLs) from Xenorhabdus nematophila (Pantel et al., 2018).
Xenorhabdus and Photorhabdus: Important Strains for the Discovery of New Antibacterials
The genera Xenorhabdus and Photorhabdus of the Enterobacteriaceae family are mutualistically associated with entomopathogenic nematodes. These bacteria have a fascinating life cycle (Figure 1) that requires the production of a great diversity of antibacterial and antifungal compounds (Dreyer et al., 2018). The bacteria-nematode pair infects and kills insects. Xenorhabdus or Photorhabdus bacteria then establish suitable conditions for the reproduction of the nematode by providing nutrients and protecting the insect corpse from environmental predators, such as bacteria and fungi. Xenorhabdus and Photorhabdus offer many advantages for anti-infective drug discovery. First, they produce novel and undescribed antimicrobial molecules with original chemical structures. This feature is genetically supported by the high content of non-ribosomal peptide synthetase (NRPS) and polyketide synthase (PKS) genes in their genomes (Tobias et al., 2017). NRPS and PKS enzymes or hybrids thereof are responsible for the biosynthesis of complex secondary metabolites via the combinatorial assembly of simple blocks, such as amino acids, acetate, or propionate. Then, antimicrobial compounds produced by Xenorhabdus and Photorhabdus interact with the biological matrices of the dead insect but are not toxic for the nematode. These properties represent a natural primary filter for compound drugability and safety in eukaryotic organisms. Finally, these genera are an underestimated and neglected source of novel bioactive compounds and thus constitute a promising source for undisclosed and unpatented molecules.
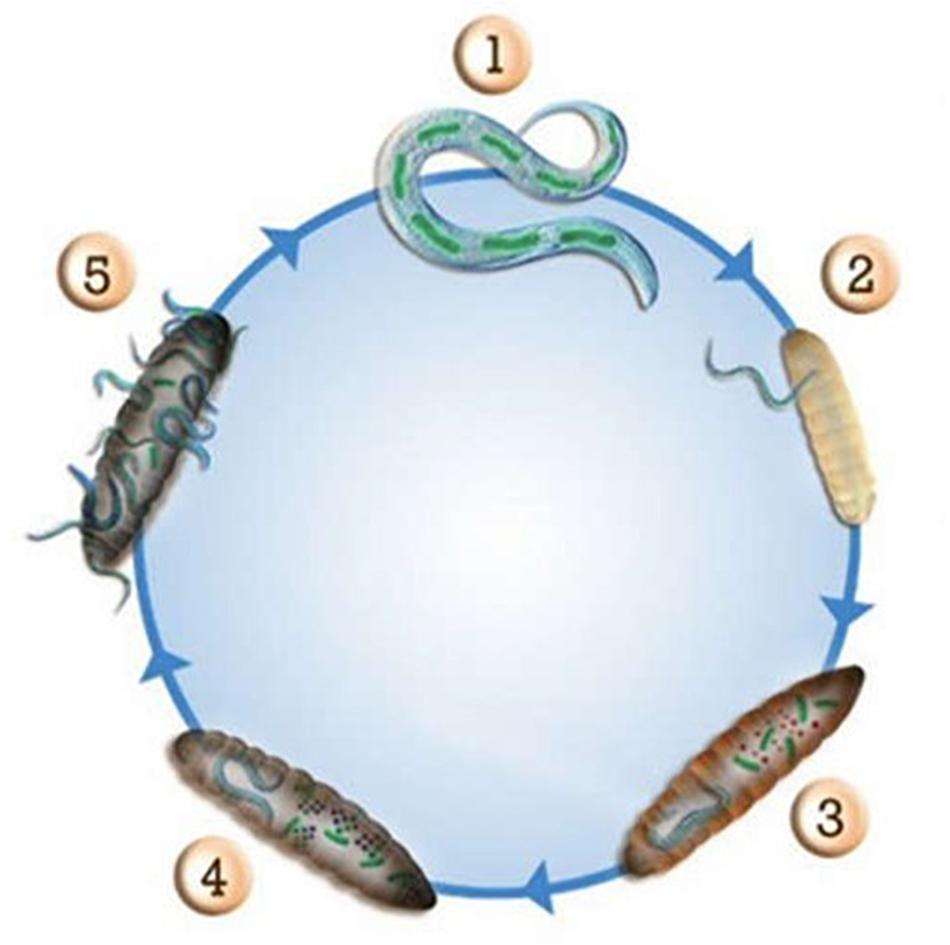
Figure 1. Life cycle of entomopathogenic nematodes and their symbiotic bacteria in insect larvae. (1) Photorhabdus or Xenorhabdus bacteria live in the intestine of the host nematode; (2) The host nematode infects an insect; (3) Photorhabdus or Xenorhabdus bacteria are released within the insect and produce compounds to kill it; (4) Photorhabdus or Xenorhabdus bacteria produce a set of antibiotic molecules to prevent microbial competitors of the environment to degrade the corpse of the insect; (5) The nematode and the bacteria use the biomass of the insect as nutrients to reproduce. Photorhabdus or Xenorhabdus bacteria recolonize the nematode that will emerge from the corpse of the insect.
Discovery of Odilorhabdins
Most bacteria do not express their full genomic potential under laboratory growth conditions or when using classic culture media and some secondary metabolites may not be produced. Multiple strategies to increase metabolite production have been described. A simple approach consists of culturing bacterial strains in various media and altering various cultivation parameters, such as temperature, salinity, flask shape, and aeration. A second approach is based on a published study showing that mutations in the RNA polymerase of various Actinomycetes strains are effective in enhancing the production of new antibiotics by activating biosynthetic gene clusters, which are “silent” or poorly expressed in wild type strains (Tanaka et al., 2013). This strategy has been applied to Xenorhabdus and Photorhabdus strains by the cultivation of selected clones resistant to rifamycins, an antibiotic family known to generate characteristic mutations on the RNA polymerase β subunit. Finally, a third approach, based on cocultivation of the strain of interest with multiple microbial strains (e.g., Streptomyces abikoensis, Streptomyces glomeroaurantiacus, Alteromonas macleodii, Micromonospora aurantiaca, Stenotrophomonas terrae, Bacillus subtilis, Staphylococcus aureus, and Sphingomonas aquatilis) has been used for antibiotic production and is well documented in the literature (Ueda and Beppu, 2017). For example, biphenomycin C was produced by Streptomyces griseorubiginosus and converted to the active antibiotic in a coculture with Pseudomonas maltophilia (Ezaki et al., 1993). In another example, alchivemycin A was only produced when Streptomyces lividans was in direct contact with bacteria producing mycolic acid (Onaka et al., 2011).
These three strategies were applied to Xenorhabdus and Photorhabdus bacteria and the supernatants of 150 cultured strains were screened for the presence of antibacterial activity. Active supernatants were fractionated by high-performance liquid chromatography (HPLC). Antibacterial, antifungal, and cytotoxic activities of the obtained fractions were measured to select those showing only antibacterial activity to avoid potentially cytotoxic compounds. The isolation and identification of ODLs from one of these fractions followed a traditional protocol, including isolation/purification by HPLC and molecular mass determination by mass spectrometry. A total of three antibacterial metabolites were characterized from the culture supernatant of X. nematophila strain CNCM I-4530. These compounds were named NOSO-95A (MW: 1,296 Da), NOSO-95B (MW: 1,280 Da), and NOSO-95C (MW: 1,264 Da) (Pantel et al., 2018). The molecular masses of ODLs were compared with those in the antibiotic mass bank to confirm their novelty. ODLs have a wide antibacterial activity spectrum that includes Gram-negative and Gram-positive bacteria. NOSO-95A was shown to have activity against many resistant strains with limited treatment options, such as carbapenem-resistant Enterobacteriaceae (CRE) or methicillin-resistant Staphylococcus aureus (MRSA) (Gualtieri et al., 2013; Pantel et al., 2018). At the same time, NOSO-95A did not show any cytotoxic effect on human HepG2 cell line, even at concentrations up to 128 μg/mL which exceeds the typical MICs for Escherichia coli, Klebsiella pneumoniae, and S. aureus by 16-, 32-, and 128-fold, respectively. NOSO-95A showed bactericidal activity against K. pneumoniae and S. aureus. These encouraging results prompted us to continue the characterization of NOSO-95A. Its in vivo efficacy was evaluated in a mouse septicemia model with S. aureus. All NOSO-95A-treated mice survived up to the end of the study (120 h) at a dose of 2.5 mg/kg. These data show the high potential of ODLs as a new class of antibiotic.
Structural Determination and Identification of the Biosynthetic Gene Cluster
The chemical structure of NOSO-95A, solved by nuclear magnetic resonance (NMR) and LC-MS/MS fragmentation analysis, revealed ODLs to be representative of a new chemical class of antibiotics (Figure 2) (Pantel et al., 2018). NOSO-95A is a 10-mer linear cationic peptide containing four proteinogenic and six non-standard amino acids: (2S,3S)-α,γ-diamino β-hydroxybutyric acid (Dab(βOH) at positions 2 and 3, D-ornithine (D-Orn) at position 5, Z-α,β–dehydroarginine (Dha) at position 9, (5S)-5-hydroxylysine (Dhl) at positions 8 and 10, and a functionalized secondary amide at the C-terminal position [α,δ–diamino butane (Dbt)]. NOSO-95B and C differ from NOSO-95A by the substitution of Dhl at position 10 (NOSO-95B) or at positions 8 and 10 (NOSO-95C) by a lysine (Figure 2). We developed the synthesis of all diastereoisomers of the three non-standard amino acids, Dab(βOH), Dha, and Dhl, and elucidated the stereochemistry of each chiral center of NOSO-95A by the advanced Marfey’s method (Bhushan and Brückner, 2004). Orn was found to be the only amino acid of R configuration while all other chiral centers were of S configuration. At the same time that the current study was being conducted, we identified the biosynthetic NRPS gene cluster (Pantel et al., 2018). This step was necessary for the production of ODLs and related analogs by engineering NRPS module enzymes. This strategy was used for daptomycin, a cyclic lipopeptide antibiotic used for the treatment of infections caused by S. aureus (Nguyen et al., 2010). We identified four large NRPS-coding genes in the genome of the producer X. nematophila as the putative biosynthetic gene cluster using anti-SMASH, a secondary metabolite gene cluster prediction software. Inactivation of the first gene of the cluster abolished production of all three ODLs, confirming that the cluster is responsible for their production (Pantel et al., 2018). Two of the non-standard amino acids, Dab(βOH) and Dha, were not commercially available and were required for the total synthesis of NOSO-95C. We developed their synthesis on a multi-gram scale and reported the first total synthesis of NOSO-95C (Sarciaux et al., 2018). The 1H NMR and LC-MS spectra and antibacterial activity of the natural and synthetic peptides were similar.
Structure-Activity Relationships and Selection of a Preclinical Candidate
Based on these encouraging results and with a validated synthetic method, we initiated a medicinal chemistry program on NOSO-95C to study the structure-activity relationships (SAR) of ODLs with the objective to better understand the role of each amino acid for the antibacterial activity and the inhibition of bacterial translation (Sarciaux et al., 2018). Before starting this study, the inhibition of bacterial translation was identified as the principal mode of action of ODLs. We evaluated the antibacterial activity of analogs against Enterobacteriaceae (E. coli and K. pneumoniae) and their potential to inhibit bacterial translation to help drive preliminary SAR studies. First, alanine scanning (Ala scan) was performed. This consists of replacing each amino acid by an alanine to evaluate the impact of lateral chains on biological activity. This strategy has been previously used to decipher the SAR of various antibacterial peptides, such as an analog of teixobactin, (Parmar et al., 2017) and feglymycin (Hänchen et al., 2013). Removal of the lateral chain of Lys1, His7, Lys8, Lys10, or Dbt11 had little or no impact on the antibacterial activity of NOSO-95C and inhibition of translation. Replacing Dab(βOH)3 by alanine resulted in a four-fold gain in antibacterial activity and in the same level of inhibition of bacterial translation than that of NOSO-95C. A better passage through the bacterial membrane could explain the improvement of antibacterial activity while conserving the same level of inhibition of translation (Figure 3). Significant decreases of both antibacterial activity and inhibition of the translation were observed when substituting Dab(βOH)2 and D-Orn5 (Figure 3). As shown later on using X-ray crystallography, the lateral chains of these two amino acids interact directly with the bacterial ribosome (Pantel et al., 2018). Substitution of Gly4, Pro6, and Dha9 by an alanine should have a strong effect on the secondary structure of the peptide. Indeed, the antibacterial activity of these analogs and the inhibition of translation were strongly reduced with the exception of the analog in which Pro6 was replaced by Ala, for which activity was conserved on E. coli while measuring a five-fold decrease of the inhibition of translation. This could be explained by a more flexible structure, making it easier for this analog to cross the bacterial membranes. We next investigated the effect of modifying the lateral chain of Dab(βOH)2 and Dha9. First, the hydroxyl group of Dab(βOH)2 was removed resulting in a strong decrease of both antibacterial activity and inhibition of translation. The same deleterious effect was observed when Dab(βOH)2 was replaced by allo-threonine (AlloThr) or Ser to study the influence of the amine function of the lateral chain. Influence of substitution of the lateral chain of Dha9 and removal of the double bond was next investigated by introducing dehydroamino butyric acid (DhAbu), L- or D-Arg. These substitutions had a limited impact, unlike substitution by alanine, meaning that either the double bond or the guanidine moiety is needed for efficient antibacterial activity and inhibition of translation. Finally, truncation of amino acids from the N- and C-terminal position of the peptide was tested to find the shortest active sequence. Removing amino acids from C-terminal part up to Lys10 (included) led to structures with almost identical in vitro antibacterial efficacy and slightly better inhibition of bacterial translation compare to parent compound (Figure 3). Importance of Dha9 was confirmed by the strong decrease of antibacterial activity and inhibition of the translation of the analog in which this amino acid was removed. Reduction of the peptidic chain from the N-terminal side was then investigated. Removal of the first amino acid or both Lys1 and Dab(βOH)2 was detrimental for both antibacterial efficacy and inhibition of translation. The key role of the N-terminal amine function for the binding to the ribosome was later confirmed by co-crystallization studies.
The combination of best modifications and of the shorter active sequence led to NOSO-95179 identified as a lead compound showing promising in vitro and in vivo antibacterial activity against Enterobacteriaceae (including CRE) and low toxicity (Pantel et al., 2018; Sarciaux et al., 2018). This compound was used as the starting point for a lead-optimization campaign. The object of this final drug discovery phase was to maintain the favorable properties of the lead compound while improving on any deficiencies. One important objective was to enhance in vitro antibacterial activity and in vivo efficacy. Five hundred analogs were synthesized and evaluated using assays similar to those performed during the previous stage (MIC determination against a panel of referenced strains, cytotoxicity against the HepG2 cell line, and inhibition of bacterial translation). None of the compounds displayed cytotoxic activity and the 10% with the best antibacterial activity and inhibition of bacterial translation were selected and examined for extended microbiological properties (MIC against a large panel of bacterial strains displaying different resistance profiles, bactericidal kinetics, and frequency of resistance), and ADME-tox properties (blood stability, acute toxicity in the mouse, and hemolytic properties). The pharmacokinetic (PK) properties, plasma protein binding, and efficacy in a murine sepsis infection model of 40 selected analogs were then assessed. Finally, the properties of the 10 best analogs were compared using a large panel of tests, including pharmacology, ADME, and safety toxicology studies. Analogs with modifications at both the Ala3 and His7 positions showed a combination of good in vitro activity and improved in vivo efficacy through favorable PK profiles, leading to increased exposure. Among these analogs, NOSO-502 showed the best profile and was selected as a promising candidate (Figure 2) (Racine et al., 2018). MIC of NOSO-502 ranges from 0.5 to 4 μg/ml against Enterobacteriaceae type strains and CRE strains expressing carbapenemase belonging to classes A, B, and D of the Ambler classification. This compound retains excellent activity against strains resistant to colistin, a last resort antibiotic used to treat multidrug-resistant bacterial infections, through expression of mobile colistin resistance (mcr) genes or mutations in mgrB, PmrAB, PhoPQ genes. In addition, NOSO-502 has a low potential for resistance development (Racine et al., 2018). It is effective in mouse models of serious hospital-acquired infections (sepsis, complicated urinary tract infection (UTI), lung infection) and provides significant protection against E. coli and K. pneumoniae, the highest-incidence hospital pathogens in complicated intra-abdominal infection (IAI) and UTI. Interestingly, NOSO-502 was effective against an E. coli strain that expresses the metallo-β-lactamase NDM-1 and is resistant to other major antibiotic classes. Although nephrotoxicity and cardiotoxicity are associated with many antibiotics, NOSO-502 exhibited a good safety profile based on data from in vitro nephrotoxicity, cardiotoxicity, genotoxicity, or cytotoxicity standard studies. AUC/MIC was selected as the PK/PD index that shows the highest correlation with the antibacterial effect of NOSO-502 in a murine thigh infection model (Zhao et al., 2018). These data combined with human PK exposure and MIC distribution will be helpful in determining an appropriate dosing-regimen for future clinical studies.
Mechanism of Action
Deciphering the mechanism of action of a new antibacterial compound is crucial for a drug discovery and development program. However, it is often a complicated process, for which various strategies have been described (Farha and Brown, 2016). We initially investigated the mode of action of ODLs by assessing the effect of the compound on the incorporation of radiolabeled precursors into four major biosynthetic pathways (protein, RNA, DNA, or peptidoglycan synthesis) of E. coli cells. These experiments demonstrated that bacterial protein synthesis is the main target of ODLs. In accordance with this conclusion, we showed that ODLs inhibit the production of a protein in an E. coli cell-free transcription-translation system with an IC50 in the same range as that of known ribosome-targeting antibiotics, such as chloramphenicol and spectinomycin (Pantel et al., 2018). Target identification was conducted by selecting resistant mutants carrying alterations in the drug-target site. As ODLs interfere with protein synthesis, one of the putative targets was the ribosome, which is the major target for antibacterials. The ribosome is a supramolecular enzyme which translates genetic information into proteins. In bacteria, it is composed of two subunits, a small 30S and large 50S subunit, which join to form a 70S ribosome. Each subunit is composed of proteins and ribosomal RNA (rRNA). In wild type E. coli, seven copies of the rRNA operon are present in the chromosome. An E. coli strain in which six of the seven rRNA operons had been deleted was used to increase the chances of selecting resistant strains with mutations in the rRNA. Whole-genome sequencing of the selected ODL-resistant clones showed that almost all the mutants carried mutations in the 16S rRNA gene of the small ribosomal subunit, clustered in the vicinity of the decoding center (DC) (Pantel et al., 2018). The DC of the ribosome performs messenger RNA (mRNA) translation and provides the fidelity of the codon/anticodon interactions, along with performing mRNA translocation during protein biosynthesis.
The crystal structure of a 70S ribosome associated with ODLs confirmed that this new class of antibiotic interacts with the small ribosomal subunit (30S) by forming multiple hydrogen bonds with 16S rRNA residues of helices 31, 32, and 34 (h31, h32, and h34) (Pantel et al., 2018). This binding site is at a site distinct from those of other inhibitors that target the 30S ribosomal subunit, such as aminoglycosides, tuberactinomycins (viomycin, capreomycin), edeine, pactamycin, tetracycline, and negamycin (Table 1). The tetracycline and negamycin sites are closest to the site of ODL binding but do not overlap with that of ODLs. Moreover, ODL-resistant clones are not resistant to other clinically used ribosomal inhibitors. These results were crucial for carrying the development of ODLs forward. In this binding site, ODLs simultaneously interact with the 16S rRNA and the anticodon loop of the transfer RNA (tRNA) in the A-site, suggesting that the drug may increase the affinity of the aminoacyl tRNA for the ribosome. The predictable consequence is constrained progression of the ribosome along the mRNA and decreased fidelity of translation. We analyzed the effect of ODLs on translocation using a toe-printing assay. This method allows the determination of interactions between ribosomes or ribosomal subunits and mRNA. At lower concentrations, ODLs induced amino acid misincorporation by reducing the accuracy of decoding, whereas at higher concentrations they interfered with translocation. This mode of translation inhibition that is dependent on the drug concentration is similar to that described for aminoglycosides and negamycin. However, these antibiotics achieve this effect via different mechanisms. Aminoglycosides interact exclusively with the 16S rRNA and increase tRNA affinity by stabilizing a conformation of the 16S rRNA that interacts with the tRNA anticodon (Demirci et al., 2013). In contrast, negamycin and ODLs establish direct contacts with the A-site tRNA, but they interact at different sites (Pantel et al., 2018). Antimicrobial peptides (AMPs) that target the ribosome are rare. Indeed, most AMPs are mostly known for their disruptive effects on bacterial membranes (e.g., polymyxin B, gramicidin, LL-37, and melittin). Only nine classes interact with the bacterial ribosome, of which five, including ODLs, interact with the small ribosomal subunit (Polikanov et al., 2018). The binding site on the 30S ribosomal and mode of action of ODLs are different from the four other classes (Figure 4). Edeine (EDE), a pentapeptide amide produced by Bacillus brevis, and GE81112, a tetrapeptide produced by some Streptomyces species, target the translation initiation phase. EDE inhibits the formation of the 30S pre-initiation complex and prevents the formation of a competent 70S initiation complex, whereas GE81112 induces conformational changes of the 30S subunit, preventing its joining with the 50S subunit to form the 70S initiation complex (Dinos et al., 2004; Brandi et al., 2006). Similarly to ODLs, dityromycin (DIT) and tuberactinomycins (TUBs) inhibit translocation at the elongation phase, but by different mechanisms. DIT, a cyclic peptide produced by Streptosporangium cinnabarum, interacts with the 30S ribosomal protein S12 and inhibits translocation by preventing EF-G from adopting the final state necessary for translocation of the tRNAs and mRNA on the small subunit (Bulkley et al., 2014). TUBs, cyclic pentapeptides produced by various Streptomyces species, bind at the interface between the 30S and 50S subunits and act by trapping the ribosome in an intermediate state on the translocation pathway (Ly et al., 2010). The targets of the four classes of AMPs that target the 50S ribosomal subunit are mostly clustered around the peptidyl transferase center (PTC), where the peptide bond is formed. Streptogramins, a class produced by Streptomyces species, prevent correct positioning of tRNAs into the PTC, making peptide-bond formation impossible (Schmeing et al., 2005). Klebsazolicin, produced by K. pneumoniae, obstructs the ribosomal exit tunnel and blocks elongation of the nascent peptide (Metelev et al., 2017). Proline-rich antimicrobial peptides (PrAMPs) have been found in insects, crustaceans, and mammals. Oncocins, the best-characterized type I PrAMPs, bind within the ribosomal exit tunnel in a reverse orientation relative to the nascent polypeptide chain, preventing transition into the elongation phase of translation (Roy et al., 2015). The apidaecin derivative Api137 blocks translation termination by trapping release factors (Florin et al., 2017). Finally, thiopeptide antibiotics GE2270A and thiostrepton inhibit translation by interacting with the translation factor EF-Tu or directly with the ribosome. Thiopeptides binding site on the large ribosomal subunit overlaps the IF2, EF-Tu, and EF-G translation factors’ binding site (Bagley et al., 2005).
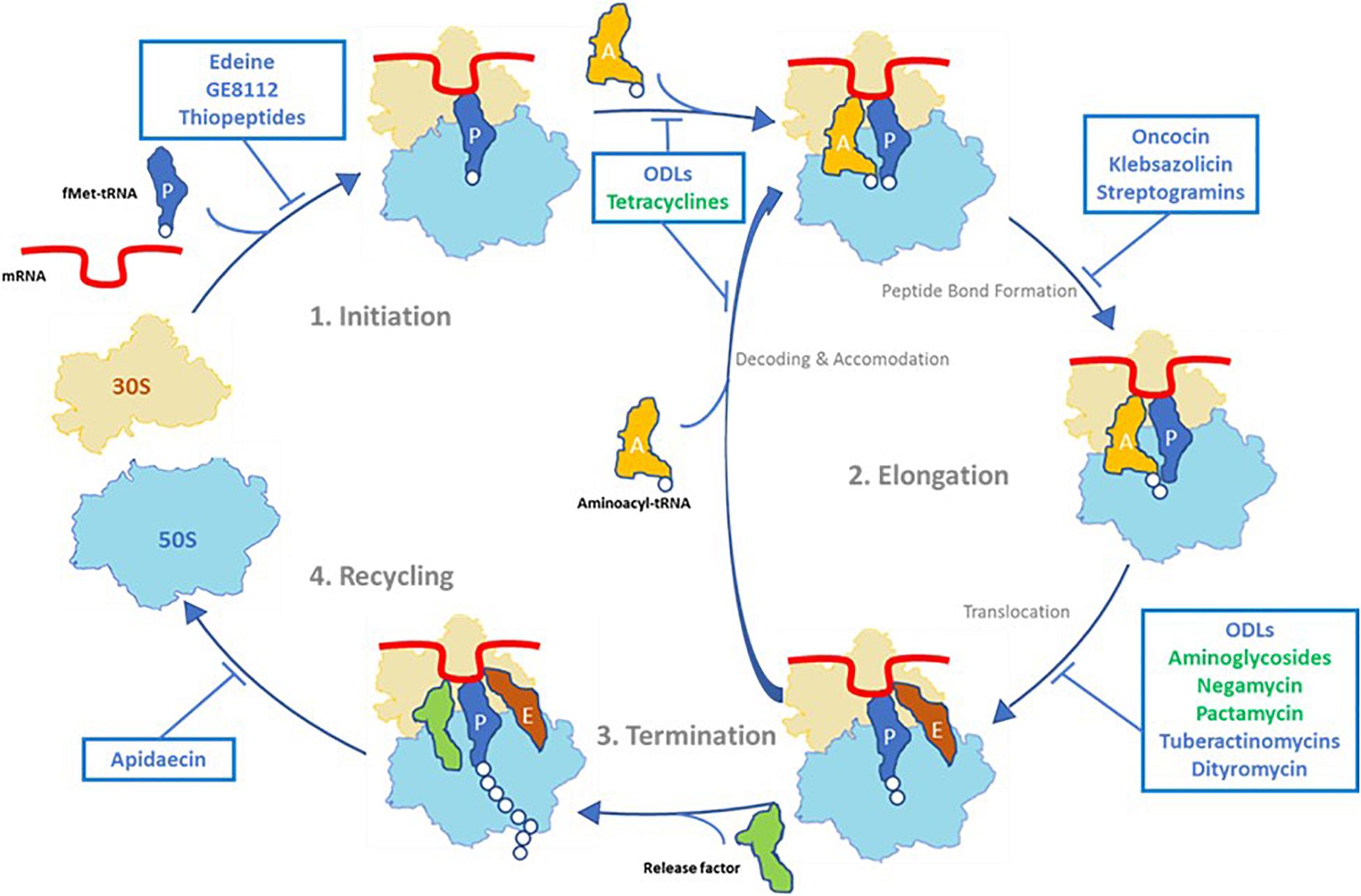
Figure 4. Overview of antibiotics that target the 30S ribosomal subunit (in green) and antibacterial peptides that inhibit the prokaryotic translation cycle (in blue).
Antibiotics That Are Active Against Carbapenem-Resistant Enterobacteriaceae (CRE) in Development or Recently Approved
In 2013, CRE, Clostridium difficile, and Neisseria gonorrhoeae bacteria were identified by the Centers for Disease Control and Prevention (CDC) as urgent drug-resistant threats, confirming the need to rapidly develop new antibiotic scaffolds active against these pathogens (Centers for Disease Control and Prevention, 2013). The urgent need to find new therapies against CRE was recently confirmed by the WHO (Tacconelli et al., 2018). Carbapenems are broad-spectrum β-lactam antibiotics considered to be the last treatment option for infections caused by multidrug-resistant pathogens. However, their use has been compromised due to the increasing prevalence of CRE infections in hospitals. CRE have become increasingly resistant to last-resort antibiotics and cause a range of healthcare-associated infection, such as pneumonia (HABP/VABP), UTI, IAI, and bloodstream infection (BSI) (Sheu et al., 2019). Numerous outbreaks of hospital acquired infections due to CRE were reported in different regions of the world (Tzouvelekis et al., 2012; van Duin and Doi, 2017). Recent reports show that the mortality of patients with CRE infections is three times higher than for patients with similar susceptible infections (Martin et al., 2018). In 2013, the CDC evaluated that CRE (E. coli and K. pneumoniae) were responsible of 9,300 nosocomial infections in the United States and caused the deaths of approximately 600 people (Centers for Disease Control and Prevention, 2013). The main mechanism responsible for carbapenem resistance among Enterobacteriaceae is the production of a carbapenemases, which are β-lactamase enzymes that efficiently break down carbapenem antibiotics. Many carbapenemases belonging to Ambler classes A, B, and D of β-lactamases have been described in Enterobacteriaceae. Optimal treatment for serious infections due to CRE is yet to be determined, but current treatment often involves the use of gentamicin, tigecycline, colistin, and amikacin, alone or in combination with each other antibiotics and carbapenems (Martin et al., 2018). In such infections, resistance to carbapenems is associated with high levels of resistance to other antibiotics [e.g., colistin (22.6%), gentamicin (43.5%), tigecycline (15.2%)] (Trecarichi and Tumbarello, 2017). Furthermore, certain therapies, such as colistin, gentamicin, or amikacin, are associated with an increased risk of nephrotoxicity. These observations highlight the importance of the development of new therapeutic options to obtain more positive outcomes in CRE infections, especially compounds from new classes of antibiotic with original mode of action to limit the risk of cross-resistance.
NOSO-502 or other ODL analogs could be one such novel therapy. Indeed, this new family of antibiotics is active against CRE isolates producing β-lactamases belonging to Ambler classes A, B, C, and D and also exhibiting resistance to gentamicin, amikacin, polymyxin B, or tigecycline. The vast majority of agents recently approved or still in development are the result of modifications of existing agents (Table 2). They cannot generally overcome multiple existing resistance mechanisms. Furthermore, none of the recently approved antimicrobials are effective against all classes of carbapenemases, unlike ODLs, with the exception of eravacycline, (Livermore et al., 2016) an antibiotic of the tetracycline class approved by the United States Food and Drug Administration (FDA) in 2018 for the treatment of complicated IAI. The combination of ceftazidime + avibactam (Avycaz®) was approved by the FDA in 2015 and by the European Medicines Agency in 2016 for the treatment of cUTI and cIAI. It is active against isolates producing class A and D carbapenemases but does not cover metallo-β-lactamases like NDM-1, VIM, or IMP (Falcone and Paterson, 2016). The combination of meropenem + vaborbactam (Vabomere®), approved by the FDA in 2017 for the treatment of cUTI (including acute pyelonephritis), cIAI, and HABP/VABP, is only active against class A carbapenemase-producing strains (KPC) within the CRE group (Castanheira et al., 2017). Plazomicin is an aminoglycoside that was approved in June 2018 for the treatment of cUTI (including pyelonephritis) caused by E. coli, K. pneumoniae, Enterobacter cloacae, and Proteus mirabilis. As other aminoglycosides, its activity is not affected by resistance mechanisms to other antibiotic classes, such as β-lactamases and carbapenemases, including metallo-β-lactamases. However, it has been shown that the class B metallo-β-lactamase NDM-1 is frequently co-expressed with 16S ribosomal RNA methyltransferases and plazomicin, like all aminoglycosides, is inactive against strains that express these enzymes (Serio et al., 2019). Other drug candidates to address these resistance issues are currently under clinical development but all belong to existing classes of antibiotics (β-lactams, β-lactamase inhibitors combined with β-lactams, tetracyclines, aminoglycosides, fosfomycins and polymyxins). Nevertheless cefiderocol, a siderophore cephalosporin, (Kohira et al., 2015) and the combinations aztreonam-avibactam or cefepim-zidebactam appear to be promising options to treat such infections (Sader et al., 2017a, b).
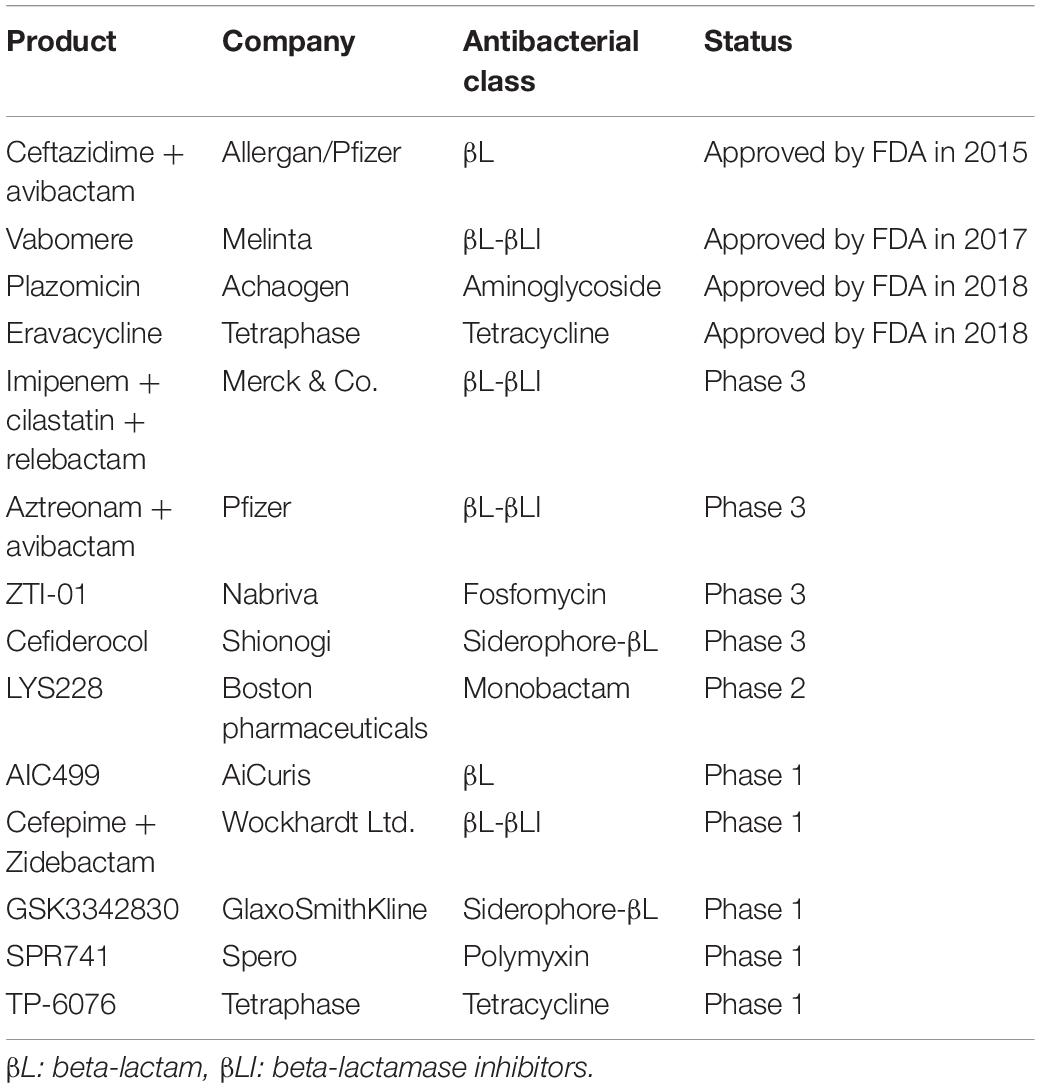
Table 2. Antibiotics active against carbapenem-resistant Enterobacteriaceae (CRE) in development or recently approved by the FDA.
Conclusion
The need for new antibiotic classes is exceedingly urgent. In this context, ODLs are a promising option to treat some of the most problematic multidrug-resistant Gram-negative infections. This is one more example of the importance of preserving biodiversity as a source of future drugs.
Author Contributions
Both authors listed have made a substantial, direct and intellectual contribution to the work, and approved it for publication.
Funding
This work received financial support from OSEO and the Languedoc-Roussillon region under grant agreement A1010014J and from the DGA under grant agreement 122906117. The research leading to these results also received support from the Innovative Medicines Initiative Joint Undertaking under grant agreement 115583, the resources of which are composed of financial contributions from the European Union Seventh Framework Program (FP7/2007-2013) and in-kind contributions of EFPIA companies.
Conflict of Interest
MG is a founder and shareholder of Nosopharm. ER and MG are employees of Nosopharm.
References
Bagley, M., Dale, J., Merritt, E., and Xiong, X. (2005). Thiopeptide antibiotics. Chem. Rev. 105, 685–714. doi: 10.1021/cr0300441
Bhushan, R., and Brückner, H. (2004). Marfey’s reagent for chiral amino acid analysis: a review. Amino Acids 27, 231–247. doi: 10.1007/s00726-004-0118-0
Brandi, L., Fabbretti, A., La Teana, A., Abbondi, M., Losi, D., Donadio, S., et al. (2006). Specific, efficient, and selective inhibition of prokaryotic translation initiation by a novel peptide antibiotic. Proc. Natl. Acad. Sci. U.S.A. 103, 39–44. doi: 10.1073/pnas.0507740102
Brodersen, D. E., Clemons, W. M., Carter, A. P., Morgan-Warren, R. J., Wimberly, B. T., and Ramakrishnan, V. (2000). The structural basis for the action of the antibiotics tetracycline, pactamycin, and hygromycin B on the 30S ribosomal subunit. Cell 103, 1143–1154. doi: 10.1016/s0092-8674(00)00216-6
Bulkley, D., Brandi, L., Polikanov, Y. S., Fabbretti, A., O’Connor, M., Gualerzi, C. O., et al. (2014). The antibiotics dityromycin and GE82832 bind protein S12 and block EF-G-catalyzed translocation. Cell Rep. 6, 357–365. doi: 10.1016/j.celrep.2013.12.024
Castanheira, M., Huband, M. D., Mendes, R. E., and Flamm, R. K. (2017). Effect of the β-Lactamase inhibitor vaborbactam combined with meropenem against serine carbapenemase-producing Enterobacteriaceae. Antimicrob. Agents Chemother. 61, 5454–5458. doi: 10.1128/AAC.00711-16
Centers for Disease Control and Prevention, (2013). Antibiotic Resistance Threats in the United States. Atlanta, GA: Centers for Disease Control and Prevention.
Clardy, J., Fischbach, M. A., and Walsh, C. T. (2006). New antibiotics from bacterial natural products. Nat. Biotechnol. 24, 1541–1550. doi: 10.1038/nbt1266
Demirci, H., Murphy, F. T., Murphy, E., Gregory, S. T., Dahlberg, A. E., and Jogl, G. (2013). A structural basis for streptomycin-induced misreading of the genetic code. Nat. Commun. 4, 1355–1362. doi: 10.1038/ncomms2346
Dinos, G., Wilson, D. N., Teraoka, Y., Szaflarski, W., Fucini, P., Kalpaxis, D., et al. (2004). Dissecting the ribosomal inhibition mechanisms of edeine and pactamycin: the universally conserved residues G693 and C795 regulate P-site RNA binding. Mol. Cell 13, 113–124. doi: 10.1016/s1097-2765(04)00002-4
Dreyer, J., Malan, A. P., and Dicks, L. M. T. (2018). Bacteria of the genus Xenorhabdus, a novel source of bioactive compounds. Front. Microbiol. 9:3177. doi: 10.3389/fmicb.2018.03177
Ezaki, M., Shigematsu, N., Yamashita, M., Komori, T., Umehara, K., and Imanaka, H. (1993). Biphenomycin C, a precursor of biphenomycin a in mixed culture. J. Antibiot. 46, 135–140. doi: 10.7164/antibiotics.46.135
Falcone, M., and Paterson, D. (2016). Spotlight on ceftazidime/avibactam: a new option for MDR Gram-negative infections. J. Antimicrob. Chemother. 71, 2713–2722. doi: 10.1093/jac/dkw239
Farha, M. A., and Brown, E. D. (2016). Strategies for target identification of antimicrobial natural products. Nat. Prod. Rep. 33:66880. doi: 10.1039/c5np00127g
Florin, T., Maracci, C., Graf, M., Karki, P., Klepacki, D., Berninghausen, O., et al. (2017). An antimicrobial peptide that inhibits translation by trapping release factors on the ribosome. Nat. Struct. Mol. Biol. 24, 752–757. doi: 10.1038/nsmb.3439
Genilloud, O. (2017). Actinomycetes: still a source of novel antibiotics. Nat. Prod. Rep. 34, 1203–1232. doi: 10.1039/c7np00026j
Gualtieri, M., Villain-Guillot, P., Givaudan, A., and Pages, S. (2013). Novel Peptide Derivates as Antibiotics. EP11183034.5 & US61/540.085. Patent WO20130 45600 (A1).
Hänchen, A., Rausch, S., Landmann, B., Toti, L., Nusser, A., and Süssmuth, R. D. (2013). Alanine scan of the peptide antibiotic feglymycin: assessment of amino acid side chains contributing to antimicrobial activity. Chembiochem 14, 625–632. doi: 10.1002/cbic.201300032
Kohira, N., West, J., Ito, A., Ito-Horiyama, T., Nakamura, R., Sato, T., et al. (2015). In Vitro Antimicrobial activity of a siderophore cephalosporin, S-649266, against Enterobacteriaceae clinical isolates, including carbapenem-resistant strains. Antimicrob. Agents Chemother. 60, 729–734. doi: 10.1128/AAC.01695-15
Ling, T. T., Schneider, T., Peoples, A. J., Spoering, L., Engels, I., and Conlon, B. P. (2015). A new antibiotic kills pathogen without detectable resistance. Nature 22, 455–459. doi: 10.1038/nature14098
Livermore, D. M., Mushtaq, S., Warner, M., and Woodford, N. (2016). In Vitro activity of eravacycline against carbapenem-resistant Enterobacteriaceae and Acinetobacter baumannii. Antimicrob. Agents Chemother. 60, 3840–3844. doi: 10.1128/AAC.00436-16
Ly, C. T., Altuntop, M. E., and Wang, Y. (2010). Single-molecule study of viomycin’s inhibition mechanism on ribosome translocation. Biochemistry 49, 9732–9738. doi: 10.1021/bi101029g
Martin, A., Fahrbach, K., Zhao, Q., and Lodise, T. (2018). Association between carbapenem resistance and mortality among adult, hospitalized patients with serious infections due to Enterobacteriaceae: results of a systematic literature review and meta-analysis. Open Forum Infect. Dis. 5:ofy150. doi: 10.1093/ofid/ofy150
Metelev, M., Osterman, I. A., Ghilarov, D., Khabibullina, N. F., Yakimov, A., Shabalin, K., et al. (2017). Klebsazolicin inhibits 70S ribosome by obstructing the peptide exit tunnel. Nat. Chem. Biol. 13, 1129–1136. doi: 10.1038/nchembio.2462
Nguyen, K. T., He, X., Alexander, D. C., Li, C., Gu, J. Q., Mascio, C., et al. (2010). Genetically engineered lipopeptide antibiotics related to A54145 and daptomycin with improved properties. Antimicrob. Agents Chemother. 54:140413. doi: 10.1128/AAC.01307-09
Onaka, H., Mori, Y., Igarashi, Y., and Furumai, T. (2011). Mycolic acid-containing bacteria induce natural-product biosynthesis in Streptomyces species. Appl. Environ. Microbiol. 77, 400–406. doi: 10.1128/AEM.01337-10
Pantel, L., Florin, T., Dobosz-Bartoszek, M., Racine, E., Sarciaux, M., Serri, M., et al. (2018). Odilorhabdins, antibacterial agents that cause miscoding by binding at a new ribosomal site. Mol Cell 70, 83–94. doi: 10.1016/j.molcel.2018.03.001
Parmar, A., Iyer, A., Prior, S. H., Lloyd, D. G., Tze Leng Goh, E., Vincent, C. S., et al. (2017). Teixobactin analogues reveal enduracididine to be non-essential for highly potent antibacterial activity and lipid II binding. Chem. Sci. 8:8183. doi: 10.1039/c7sc03241b
Pioletti, M., Schlünzen, F., Harms, J., Zarivach, R., Glühmann, M., Avila, H., et al. (2001). Crystal structures of complexes of the small ribosomal subunit with tetracycline, edeine and IF3. EMBO J. 2001:182939. doi: 10.1093/emboj/20.8.1829
Polikanov, Y. S., Aleksashin, N. A., Beckert, B., and Wilson, D. N. (2018). The mechanisms of action of ribosome-targeting peptide antibiotics. Front. Mol. Biosci. 5:48. doi: 10.3389/fmolb.2018.00048
Polikanov, Y. S., Szal, T., Jiang, F., Gupta, P., Matsuda, R., Shiozuka, M., et al. (2014). Negamycin interferes with decoding and translocation by simultaneous interaction with rRNA and tRNA. Mol. Cell 56, 541–550. doi: 10.1016/j.molcel.2014.09.021
Racine, E., Nordmann, P., Pantel, L., Sarciaux, M., Serri, M., Houard, J., et al. (2018). In Vitro and in Vivo characterization of NOSO-502, a novel inhibitor of bacterial translation. Antimicrob. Agents Chemother. 62:e1016-18. doi: 10.1128/AAC.01016-18
Roy, R. N., Lomakin, I. B., Gagnon, M. G., and Steitz, T. A. (2015). The mechanism of inhibition of protein synthesis by the proline-rich peptide oncocin. Nat. Struct. Mol. Biol. 22, 466–469. doi: 10.1038/nsmb.3031
Sader, H. S., Castanheira, M., Streit, J. M., Duncan, L. R., and Flamm, R. K. (2017a). Cefepime-Zidebactam (WCK 5222) activity tested against gram-negative organisms causing bloodstream infections worldwide. Open Forum Infect. Dis. 4, S374–S375.
Sader, H. S., Mendes, R. E., Pfaller, M. A., Shortridge, D., Flamm, R. K., and Castanheira, M. (2017b). Antimicrobial activities of aztreonam-avibactam and comparator agents against contemporary (2016) clinical Enterobacteriaceae isolates. Antimicrob. Agents Chemother. 62:e01856-17. doi: 10.1128/AAC.01856-17
Sarciaux, M., Pantel, L., Midrier, C., Serri, M., Gerber, C., Marcia de Figueiredo, R., et al. (2018). Total synthesis and structure-activity relationships study of odilorhabdins, a new class of peptides showing potent antibacterial activity. J. Med. Chem. 61, 7814–7826. doi: 10.1021/acs.jmedchem.8b00790
Schmeing, T. M., Huang, K. S., Strobel, S. A., and Steitz, T. A. (2005). An induced-fit mechanism to promote peptide bond formation and exclude hydrolysis of peptidyl-tRNA. Nature 438, 520–524. doi: 10.1038/nature04152
Serio, A. W., Keepers, T., and Krause, K. M. (2019). Plazomicin is active against metallo-β-lactamase-producing Enterobacteriaceae. Open Forum Infect. Dis. 6:ofz123. doi: 10.1093/ofid/ofz123
Sheu, C. C., Chang, Y. T., Lin, S. Y., Chen, Y. H., and Hsueh, P. R. (2019). Infections caused by carbapenem-resistant Enterobacteriaceae: an update on therapeutic options. Front. Microbiol. 10:80. doi: 10.3389/fmicb.2019.00080
Stanley, R. E., Blaha, G., Grodzicki, R. L., Strickler, M. D., and Steitz, T. A. (2010). The structures of the anti-tuberculosis antibiotics viomycin and capreomycin bound to the 70S ribosome. Nat. Struct. Mol. Biol. 17, 289–293. doi: 10.1038/nsmb.1755
Tacconelli, E., Carrara, E., Savoldi, A., Harbarth, S., Mendelson, M., Monnet, D. L., et al. (2018). Discovery, research, and development of new antibiotics: the WHO priority list of antibiotic-resistant bacteria and tuberculosis. Lancet Inf. Dis. 18, 318–327. doi: 10.1016/S1473-3099(17)30753-3
Tanaka, Y., Kasahara, K., Hirose, Y., Murakami, K., Kugimiya, R., and Ochi, K. (2013). Activation and products of the cryptic secondary metabolite biosynthetic gene clusters by rifampin resistance (rpoB) mutations in actinomycetes. J. Bacteriol. 195, 2959–2970. doi: 10.1128/JB.00147-13
Tobias, N. J., Wolff, H., Djahanschiri, B., Grundmann, F., Kronenwerth, M., Shi, Y. M., et al. (2017). Natural product diversity associated with the nematode symbionts Photorhabdus and Xenorhabdus. Nat. Microbiol. 2, 1676–1685. doi: 10.1038/s41564-017-0039-9
Trecarichi, E. M., and Tumbarello, M. (2017). Therapeutic options for carbapenem-resistant Enterobacteriaceae infections. Virulence 8, 470–484. doi: 10.1080/21505594.2017.1292196
Tzouvelekis, L. S., Markogiannakis, A., Psichogiou, M., Tassios, P. T., and Daikos, G. L. (2012). Carbapenemases in Klebsiella pneumoniae and other Enterobacteriaceae: an evolving crisis of global dimensions. Clin. Microbiol. Rev. 25, 682–707. doi: 10.1128/CMR.05035-11
Ueda, K., and Beppu, T. (2017). Antibiotics in microbial coculture. J. Antibiot. 70, 361–365. doi: 10.1038/ja.2016.127
van Duin, D., and Doi, Y. (2017). The global epidemiology of carbapenemase-producing Enterobacteriaceae. Virulence 8, 460–469. doi: 10.1080/21505594.2016.1222343
Wang, L., Pulk, A., Wasserman, M. R., Feldman, M. B., Altman, R. B., Cate, J. H., et al. (2012). Allosteric control of the ribosome by small-molecule antibiotics. Nat. Struct. Mol. Biol. 19, 957–963. doi: 10.1038/nsmb.2360
Wilson, D. N. (2009). The A-Z of bacterial translation inhibitors. Crit. Rev. Biochem. Mol. Biol. 44, 393–433. doi: 10.3109/10409230903307311
Zhao, M., Lepak, A. J., Marchillo, K., VanHecker, J., and Andes, D. (2018). In Vivo pharmacodynamic characterization of a novel odilorhabdin antibiotic, NOSO-502, against Escherichia coli and Klebsiella pneumoniae in a murine thigh infection model. Antimicrob. Agents Chemother. 62:e01067-18. doi: 10.1128/AAC.01067-18
Keywords: Odilorhabdins, antimicrobial agent, Xenorhabdus, translation inhibitor, cationic peptide
Citation: Racine E and Gualtieri M (2019) From Worms to Drug Candidate: The Story of Odilorhabdins, a New Class of Antimicrobial Agents. Front. Microbiol. 10:2893. doi: 10.3389/fmicb.2019.02893
Received: 07 October 2019; Accepted: 02 December 2019;
Published: 18 December 2019.
Edited by:
András Fodor, University of Szeged, HungaryReviewed by:
John D. Wade, The University of Melbourne, AustraliaAdler Ray Dillman, University of California, Riverside, United States
Eva Kondorosi, Biological Research Centre (MTA), Hungary
David Clarke, University College Cork, Ireland
Copyright © 2019 Racine and Gualtieri. This is an open-access article distributed under the terms of the Creative Commons Attribution License (CC BY). The use, distribution or reproduction in other forums is permitted, provided the original author(s) and the copyright owner(s) are credited and that the original publication in this journal is cited, in accordance with accepted academic practice. No use, distribution or reproduction is permitted which does not comply with these terms.
*Correspondence: Maxime Gualtieri, bS5ndWFsdGllcmlAbm9zb3BoYXJtLmNvbQ==