- 1Centre d’Étude et de Valorisation de la Diversité Microbienne, Département de Biologie, Faculté des Sciences, Université de Sherbrooke, Sherbrooke, QC, Canada
- 2Département de Microbiologie et d’Infectiologie, Faculté de Médecine et de Sciences de la Santé, Université de Sherbrooke, Sherbrooke, QC, Canada
- 3Service de Pneumologie, Département de Médecine, Faculté de Médecine et des Sciences de la Santé, Université de Sherbrooke, Sherbrooke, QC, Canada
Staphylococcus aureus and Pseudomonas aeruginosa are prevalent lung pathogens in cystic fibrosis (CF). Whereas co-infection worsens the clinical outcome, prototypical strains are usually antagonistic in vitro. We sought to resolve the discrepancy between these in vitro and in vivo observations. In vitro, growth kinetics for co-cultures of co-isolates from CF patients showed that not all P. aeruginosa strains affected S. aureus viability. On solid media, S. aureus slow-growing colonies were visualized around some P. aeruginosa strains whether or not S. aureus viability was reduced in liquid co-cultures. The S. aureus–P. aeruginosa interactions were then characterized in a mouse lung infection model. Lung homogenates were plated on selective media allowing colony counts of either bacterium. Overall, 35 P. aeruginosa and 10 S. aureus strains (clinical, reference, and mutant strains), for a total of 200 co-infections, were evaluated. We observed that S. aureus colonization of lung tissues was promoted by P. aeruginosa and even by strains showing antagonism in vitro. Promotion was proportional to the extent of P. aeruginosa colonization, but no correlation was found with the degree of myeloperoxidase quantification (as marker of inflammation) or with specific virulence-associated factors using known mutant strains of S. aureus and P. aeruginosa. On the other hand, P. aeruginosa significantly increased the expression of two possible cell receptors for S. aureus, i.e., ICAM-1 and ITGA-5 (marker for integrin α5β1) in lung tissue, while mono-infections by S. aureus did not. This study provides insights on polymicrobial interactions that may influence the progression of CF-associated pulmonary infections.
Introduction
Cystic fibrosis (CF) is the most common recessive genetic disorder leading to chronic pulmonary infections, gastrointestinal disorders, diabetes, and other health complications. The most severe complications are associated with recurrent lung infections, which are responsible for high morbidity and mortality (Cystic Fibrosis Canada, 2018). A gene defect in the CF transmembrane conductance regulator (CFTR), which is a membrane protein and chloride channel, causes abnormally thick and viscous mucus production in the lung mucosa (Ratjen, 2009; Kreda et al., 2012). This defect affects muco-ciliary clearance, reduces bacterial killing via an impaired HCO3 excretion, and thus supports bacterial growth (Castellani and Assael, 2017). Infections lead to inflammation, and the host response stimulates further mucus production (Gómez and Prince, 2007; Ratjen, 2009). The mucus, rich in nutrients and not being efficiently cleared, promotes colonization of opportunistic pathogens (Palmer et al., 2005; Sriramulu et al., 2005). The establishment of this feedback loop results in frequent exacerbations and increasingly reduced lung functions, which eventually lead to death (Lyczak et al., 2002).
Throughout their lives, CF patients will be infected by many opportunistic environmental bacteria, the two most common being Staphylococcus aureus and Pseudomonas aeruginosa. The following chronological trend occurs in most patients: S. aureus typically colonizes younger patients, then its prevalence declines in adulthood. On the contrary, P. aeruginosa infections are infrequent in childhood but become predominant later when CF patients reach adulthood. Despite their seemingly sequential appearance, both pathogens remain highly prevalent through all stages of the lives of CF patients, with, respectively, 59.9 and 40.2% of patients infected by S. aureus and P. aeruginosa (Cystic Fibrosis Canada, 2018). While P. aeruginosa infections undoubtedly cause deterioration in patient health (Sadikot et al., 2005; Harun et al., 2016), the contribution of S. aureus infections to morbidity and mortality remains controversial with not all studies agreeing whether they can single-handedly worsen prognosis (Junge et al., 2016; Limoli et al., 2016). However, microbial interactions are possible. Cigana et al. (2018) investigated S. aureus–P. aeruginosa interactions in a murine chronic lung infection model. Following the natural course of infections in CF, mice were first infected with S. aureus, causing abscess-like wounds, then further infected by P. aeruginosa. P. aeruginosa was able to better chronically infect mice that had been pre-infected with S. aureus, reminiscent of that observed in CF-afflicted humans. Furthermore, many reports have associated S. aureus–P. aeruginosa co-infections with a worse clinical outcome for CF patients such as decreased pulmonary function, more frequent exacerbations, and increased mortality (Hubert et al., 2013; Limoli et al., 2016). Given these insights, it appears critical to further investigate the interactions between these microorganisms, to help prevent and treat deleterious co-infections.
Staphylococcus aureus small-colony variants (SCVs) are respiratory-deficient variants differing from their prototypical counterparts by their slow growth, alternative expression of virulence genes, and persistence in chronic infections (Moisan et al., 2006; Mitchell et al., 2013). SCVs are frequently associated with chronic infections, including CF lung infections (Kahl et al., 2016). Their ability to persist is mainly due to increased biofilm production and internalization into host cells, allowing them to evade the action of antibiotics and the immune system (Proctor et al., 2006). The alternative sigma factor B (SigB) is an important regulator of virulence in SCVs, and dominate over the quorum-sensing (QS) Agr system, which is responsible for exotoxins and hydrolytic enzyme expression (Novick and Geisinger, 2008; Mitchell et al., 2013). The presence of SCVs was directly associated with a worse respiratory outcome in children with CF (Wolter et al., 2013). Interestingly, P. aeruginosa can induce the SCV phenotype in S. aureus. P. aeruginosa produces a wide variety of QS molecules to coordinate the expression of its virulence factors, motility and extracellular matrix formation (Williams and Cámara, 2009). Among P. aeruginosa QS-controlled virulence factors, many such as the elastases, pyocyanin, pyoverdine, hydrogen cyanide, and alkyl quinolones were shown to negatively affect S. aureus growth in vitro (Machan et al., 1992; Hoffman et al., 2006; Goerke and Wolz, 2010). More specifically, P. aeruginosa 2-heptyl-4-hydroxy quinoline N-oxide (HQNO) induces the SCV phenotype by acting as a respiratory chain inhibitor for S. aureus (Lightbown and Jackson, 1956). HQNO-sensitized S. aureus are known to produce more biofilm, and there is a direct correlation between HQNO levels and biofilm production by S. aureus (Mitchell et al., 2010). Interactions between P. aeruginosa and S. aureus during a co-infection in CF patients are likely to occur and these may modulate virulence in unexpected ways.
On the other hand, we previously demonstrated that P. aeruginosa and S. aureus strains co-isolated from a same CF patient do not always interact as expected for prototypical strains (i.e., prototypical P. aeruginosa inducing biofilm production by S. aureus in vitro) (Fugère et al., 2014). For instance, a high HQNO production by some P. aeruginosa strains does not proportionally induce biofilm production by the co-isolated S. aureus strain. This suggests that co-isolates may adapt to each other in order to persist in the lung. Similarly, Limoli et al. (2017) recently demonstrated that P. aeruginosa isolates from long-term coinfected patients did not antagonize S. aureus in vitro. While such studies show that P. aeruginosa does not always antagonize S. aureus in vitro, data from co-infection animal models are needed to better understand clinical observations associated with P. aeruginosa and S. aureus co-infections.
To our knowledge, the impact of P. aeruginosa CF clinical strains on S. aureus colonization in vivo has never been systematically studied. The objective of the present study was to evaluate the circumstances allowing S. aureus to colonize and survive in the lung despite the presence of P. aeruginosa. We first used in vitro models to characterize clinical strains and the types of interactions between P. aeruginosa and S. aureus and then compared their ability to co-colonize in a murine lung infection model. Our findings show that S. aureus clearly profits from the presence of P. aeruginosa in a murine lung infection model, whether or not antagonism is seen in vitro.
Materials and Methods
Ethics Statement
The animal experiments were carried out according to the guidelines of the Canadian Council on Animal Care and the institutional ethics committee on animal experimentation of the Faculté des Sciences of Université de Sherbrooke, which specifically approved the protocols used for this study (FM2014-02 and FM2018-01B).
Bacterial Strains and Growth Conditions
Pseudomonas aeruginosa PA14 (Rahme et al., 1995) and S. aureus CF07-L (Moisan et al., 2006) were used as prototypical control strains. An additional 29 P. aeruginosa and 5 S. aureus clinical isolates were also used in this study. These isolates were previously characterized and obtained from 32 adult CF patients (Fugère et al., 2014). These included 16 P. aeruginosa that were co-isolated with S. aureus and 13 that were not (see additional information in Supplementary Table S1). Among these clinical strains, S. aureus strains CF6B-L, CF22A-L, CF39A-L, CF54A-L, and CF112A-L were more specifically selected and studied because their biofilm production was not stimulated by their co-isolated P. aeruginosa PAC6B, PAC22A, PAC39A, PAC54A, and PAC112A, respectively (Fugère et al., 2014).
To determine the impact of different bacterial components on the in vivo colonization of S. aureus in the presence of P. aeruginosa, different mutant strains from both species were also used in this study. Table 1 shows the relevant characteristics of those mutants and their origin. The S. aureus NRS strains were obtained from the Network on Antimicrobial Resistance in S. aureus (NARSA). TSA and TSB (BD, Mississauga, ON, Canada) were generally used as growth media. Cation-adjusted Mueller–Hinton broth (CAMHB; BD, Mississauga, ON, Canada) was used in growth kinetics experiments.
Growth Kinetics Experiments
The effect of P. aeruginosa isolates on S. aureus was investigated in growth kinetics experiments, similarly to those we previously described (Boulanger et al., 2015). Both S. aureus and P. aeruginosa were grown alone or in the presence of each other. Individual strains (105–106 CFU/ml) were used to inoculate CAMHB cultures. The cultures were incubated for 48 h with shaking (225 rpm) at 35°C. Samples were collected at 0, 2, 4, 6, 8, 24, and 48 h after the initial inoculation, serially diluted, and plated on TSA with 1 μg/ml of rifampicin (Clinical and Laboratory Standards Institute [CLSI], 2018) and on TSA with 10 μg/ml of polymyxin B (Clinical and Laboratory Standards Institute [CLSI], 2018) for selection of P. aeruginosa and S. aureus CFU, respectively. Bacterial counts were determined after a 24-h incubation at 35°C and confirmed after 48 h. Data were collected from at least three independent assays.
Co-culture Petri Model
To visualize colony morphology and apparition of slow-growing colonies of S. aureus in the presence of P. aeruginosa, a co-culture Petri model was established. Approximately 105CFU/ml of S. aureus was suspended in phosphate-buffered saline (PBS) and then spread on TSA plates. P. aeruginosa was suspended in PBS at a concentration of 107–108CFU/ml, then 10 μl of the suspension was spotted at the center of the S. aureus inoculated plates. Plates were incubated 24 h at 35°C, then areas of interest were photographed using a Leica M165 FC stereomicroscope (Leica, Concord, ON, Canada) with an objective of 0.63×. S. aureus showing a reduced colony size and a loss of pigmentation were considered slow-growing colonies and not strictly SCVs since they were not subcultured to see if they maintained their phenotype. Observations were collected from three independent experiments.
Mouse Lung Mono- and Co-infection Model
The mouse model of pulmonary infection has been described previously (Mitchell et al., 2013) and was used here to investigate the extent of colonization by S. aureus and P. aeruginosa in vivo during mono and coinfections. Briefly, overnight bacterial cultures were used to inoculate fresh TSB at an A600nm of 0.1. Cultures were grown at 35°C with shaking (225 rpm) until the A600nm reached 0.6–0.8. Bacterial cells were then collected by centrifugation, washed, and suspended in PBS. Strains were suspended in 50 μl to concentrations required for infection: 2 × 106 CFU for S. aureus and P. aeruginosa PA14, 2 × 107 CFU for all the other clinical strains of P. aeruginosa. Such inocula were chosen because they were found to be an appropriate bacterial load to induce reproducible infections. For mixed infections, the quantity of total bacteria was equivalent to the sum of each inoculum used in mono infection. A sterilized 250-μl glass syringe (Hamilton Company, Reno, NV, United States) equipped with a bent feeding needle (Fine Science Tools, North Vancouver, BC, Canada) was used to infect CD-1 female mice (22–24 g, Charles River, Sherbrooke, QC, Canada). Animals were anesthetized with ketamine and xylazine and then, using an otoscope equipped with a speculum (model 21700, Welch Allyn, Mississauga, ON, Canada), the trachea was located, and the tip of the feeding needle was inserted. While maintaining the otoscope in place, 50 μl of the inoculum was instilled. After 24 h of infection, the animals were anesthetized, sacrificed, and then the lungs were harvested and homogenized using a Kinematica Polytron homogenizer 10-35 GT (Kinematica, Bohemia, NY, United States) in 1.5 ml of PBS. CFU were enumerated by serially diluting homogenates in PBS and plating on TSA with 1 μg/ml of rifampicin and on TSA with 10 μg/ml of polymyxin B, allowing selective growth of P. aeruginosa and S. aureus, respectively. Part of the homogenates was kept at −80°C until used for measurement of myeloperoxidase (MPO) activity (see below).
MPO Activity
Assessment of inflammation and infiltration of neutrophils during mono or coinfections of mouse lung tissues were evaluated by quantification of MPO activity using the o-dianisidine-H2O2 method, as previously described (Côté-Gravel et al., 2016). Briefly, 10 μl of lung homogenate was mixed with a solution of o-dianisidine hydrochloride (167 μg/ml) (Sigma–Aldrich, Oakville, ON, Canada), 0.0005% H2O2 (Sigma–Aldrich, Oakville, ON, Canada), 50 mM hexade-cyltrimethylammonium bromide (CTAB) and 50 mM phosphate buffer at pH 6.0, in a 96-well plate. The A460nm was then measured at intervals of 15 s for 8 min and the maximum reaction rate was considered. One unit of MPO was defined as the quantity of enzyme degrading 1 μmol of H2O2/min at 25°C, with an absorption coefficient of 11.3 mM–1 cm–1 at 460 nm for o-dianisidine. MPO units were normalized according to the lung weight.
RNA Isolation and RT-qPCR
Non-infected and infected (mono and coinfections) mouse lungs were homogenized on ice in 1 ml Trizol (Thermo Fisher Scientific, Rochester, NY, United States) using a Dounce tissue grinder, according to the manufacturer’s indications. RNA was extracted from homogenates using the RNeasy Mini Kit (Qiagen, Toronto, ON, Canada), following the manufacturer’s protocol. RNA integrity was verified by migration on 1% agarose gels and absence of residual DNA was confirmed by PCR with glyceraldehyde-3-phosphate dehydrogenase (GAPDH) primers and subsequent migration on 1.5% agarose gels. cDNA was obtained by reverse transcription from RNA using the 5× All-In-One RT MasterMix kit (Applied Biological Materials, Richmond, BC, Canada). Two microliters of cDNA was amplified with the SYBR Green JumpStart Taq ReadyMix (Sigma–Aldrich, Oakville, ON, Canada) by qPCR. GAPDH was used as an internal control gene for all samples, to relativize intercellular adhesion molecule 1 (ICAM-1) and integrin alpha-5 (ITGA-5) expression. The following primer DNA sequences were used:
ICAM-1 FWD 5′-GTTCCAGTATGACTCCACTCACGG-3′;
ICAM-1 REV 5′-CGGCCTCACCCCATTTGATGTTAG-3′;
ITGA-5 FWD 5′-TGTTTCAGGCTGCGCTGTGA-3′;
ITGA-5 REV 5′-CTGGCGGCTCAGTATCTCCTC-3′;
GAPDH FWD 5′-GTTCCAGTATGACTCCACTCACGG′3’;
GAPDH REV 5′-CGGCCTCACCCCATTTGATGTTAG-3′.
Results
Clinical Co-isolates Show Different Levels of Antagonism in vitro
Using a collection of bacterial isolates from CF patients, Fugère et al. (2014) showed that co-isolated strains of P. aeruginosa and S. aureus interact differently in vitro than prototypical P. aeruginosa and S. aureus strains. Notably, when compared to prototypical strains, much less stimulation of S. aureus biofilm production was seen in presence of P. aeruginosa culture supernatants when co-isolates from CF patients were studied (Fugère et al., 2014). Interactions of co-isolates were thus further examined in the present work. Growth kinetics and viability of both species in co-cultures in vitro were measured. The prototypical strains PA14 and CF07-L, both extensively characterized in the scientific literature (Rahme et al., 1995; Mitchell et al., 2010), were considered as a suitable control pair for typical antagonistic interactions between these organisms. Figure 1 reports the types of interactions we observed. First, Figure 1A confirms the strong antagonism of PA14 over S. aureus CF07-L. Viability of S. aureus CF07-L was lowered by P. aeruginosa PA14 after 8 h of co-culture with viable counts dropping by 2.1 and 3.8 log10 CFU/mL at 24 and 48 h, respectively, compared to the counts of S. aureus in the mono-culture. Furthermore, co-culture on agar plates revealed formation of slow-growing colonies of S. aureus CF07-L around the large central colony of P. aeruginosa PA14 (Figure 1B), which is also typical of P. aeruginosa antagonism on S. aureus through the production of HQNO (Hoffman et al., 2006). We observed a similar antagonism by P. aeruginosa on S. aureus for the CF patient co-isolates PAC6B and CF6B-L, PAC39A and CF39A-L, and PAC112A and CF112A-L, respectively (Supplementary Figure S1), although the reduction of S. aureus CFU/mL counts at 48 h was less than that observed for CF07-L co-cultured with PA14 (i.e., 1.9, 2.1, and 3.6 log10, respectively). On the other hand, CF patient P. aeruginosa PAC54A did not affect the viability of its co-isolate S. aureus CF54A-L (Figure 1C) and no slow-growing colony of S. aureus and no growth inhibition was seen around P. aeruginosa on the agar plate (Figure 1D). When substituting P. aeruginosa PAC54A by PA14, a very strong antagonism toward S. aureus CF54A-L was observed (Figures 1E,F) showing that the lack of antagonism in Figures 1C,D was linked to P. aeruginosa PAC54A and not an insensitivity of S. aureus CF54A-L to P. aeruginosa. Interestingly, a different scenario was observed with the CF patient co-isolates P. aeruginosa PAC22A and S. aureus CF22A-L, where no effect on S. aureus viability was observed (Figure 1G) although slow-growing colonies of S. aureus appeared around the P. aeruginosa central colony (Figure 1H). In short, while P. aeruginosa PA14 displayed a strong antagonism on S. aureus CF07-L (or CF54A-L), the CF patient co-isolated P. aeruginosa–S. aureus pairs (i.e., PAC54A–CF54A-L, PAC22A–CF22A-L, PAC6B–CF6B-L, PAC39A–CF39A-L, PAC112A–CF112A-L) that we tested showed less or no antagonism in the co-culture in vitro models (broth and agar).
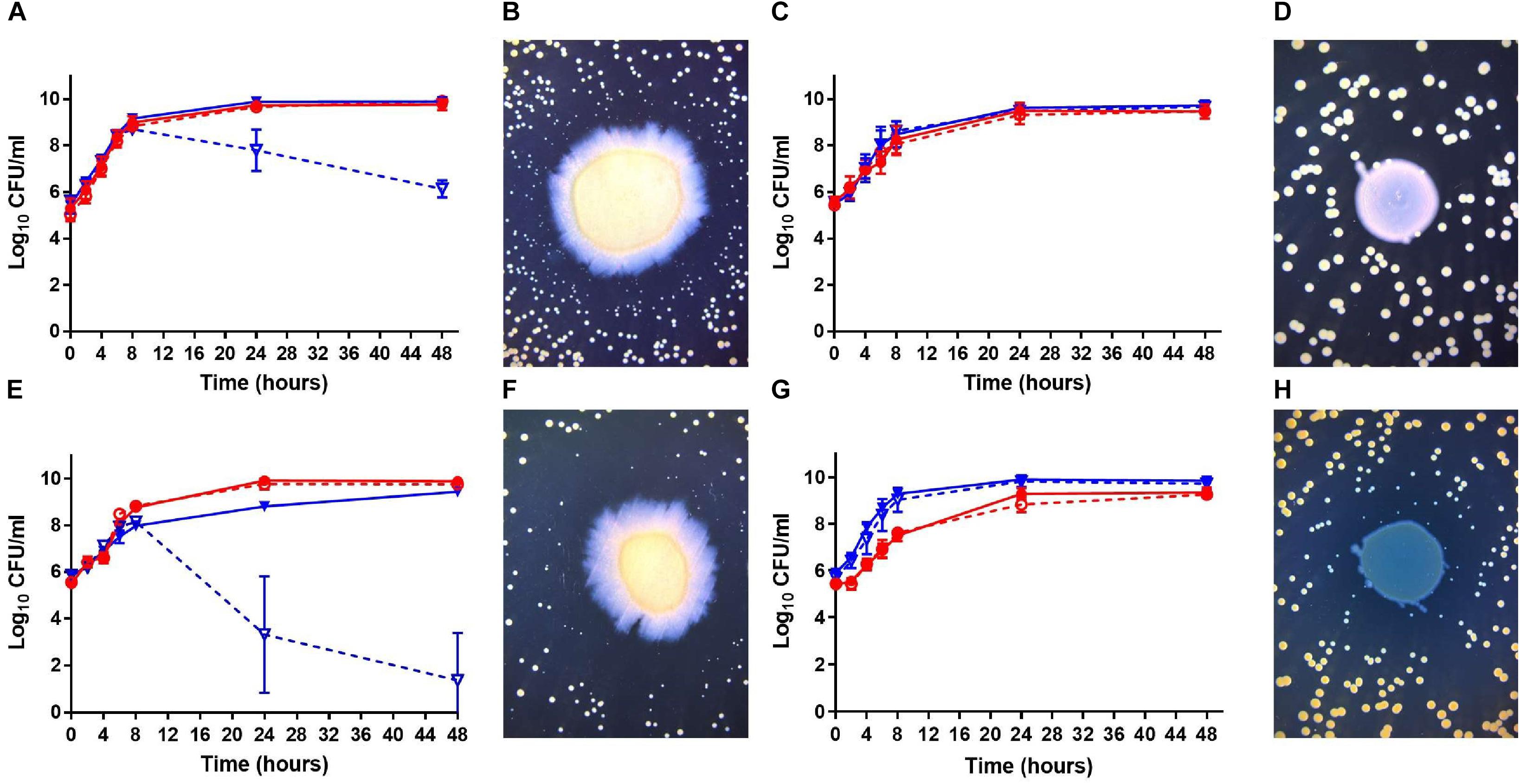
Figure 1. Growth kinetics and bacterial viability of S. aureus and P. aeruginosa in mono or co-cultures. (A,C,E,G) Bacteria were grown in broth cultures and viability is expressed in log10 CFU/ml. The CFUs were determined by plating sample dilutions on TSA supplemented with polymyxin B (S. aureus) or with rifampicin (P. aeruginosa). The solid dots (∙) and full red lines represent counts in P. aeruginosa mono-cultures; the open dots (°) and dashed red lines, P. aeruginosa counts in co-cultures; the solid triangles (▼) and full blue lines, counts of S. aureus in mono-cultures; the open triangles (▽) and dashed blue lines, S. aureus counts in co-cultures. (B,D,F,H) S. aureus was plated on TSA and then a spot of P. aeruginosa was deposited in the center of the plate. The plates were photographed after 24 h of incubation. The P. aeruginosa and S. aureus pairs tested were PA14 and CF07-L (A,B), PAC54A and CF54A-L (C,D), PA14 and CF54A-L (E,F), and PAC22A and CF22A-L (G,H), respectively. The data shown were collected from at least three independent assays.
P. aeruginosa Increases S. aureus Colonization in a Mouse Lung Infection Model, Regardless of Their Type of Interactions in vitro
Considering that we have observed that some S. aureus–P. aeruginosa clinical co-isolates do not necessarily interact as prototypical strains, namely by a lack or a lesser degree of antagonism in vitro, we wanted to examine if such in vitro observations correlated with the outcome of co-infections in vivo using a mouse pulmonary infection model. The level of bacterial colonization of the lungs following a mono-infection (either by S. aureus or by P. aeruginosa) was compared to the level of colonization obtained after a 24-h co-infection by both pathogens by measuring species-specific CFU counts. Several S. aureus–P. aeruginosa pairs were tested. P. aeruginosa PAC54A, a clinical strain showing no antagonism in vitro toward its co-isolate S. aureus CF54A-L (Figures 1C,D), promoted S. aureus lung colonization by a median increase of 0.8 log10 (Figure 2A) in comparison to the mono-infection of CF54A-L. Besides, strains P. aeruginosa PA14 and S. aureus CF07-L displayed antagonism in the in vitro models described earlier (Figures 1A,B) and were considered again as a typical control pair. Surprisingly, the antagonism observed in vitro did not translate in vivo and, on the contrary, cooperation was observed as P. aeruginosa PA14 increased S. aureus CF07-L CFU counts compared to the mono-infection (a median increase of 1.7 log10, Supplementary Figure S2). Due to this unexpected in vivo cooperation from a bacterial pair showing antagonism in vitro, PA14 co-infection was tested again, but this time with S. aureus CF54A-L, which was even more strongly affected by P. aeruginosa PA14 in vitro (Figures 1E,F). Once again, S. aureus CF54A-L colonization was enhanced by the presence of P. aeruginosa PA14 despite strong antagonism in vitro (2.0 log10 median increase compared to the mono-infection, Figure 2B). Noteworthy, in all cases, P. aeruginosa colonization was never promoted by the presence of S. aureus (comparison of P. aeruginosa mono and co-infections, not statistically significant, Figures 2A,B and Supplementary Figure S2). In summary, for all the tested strains, P. aeruginosa promotes S. aureus lung colonization, even if it is antagonistic in vitro.
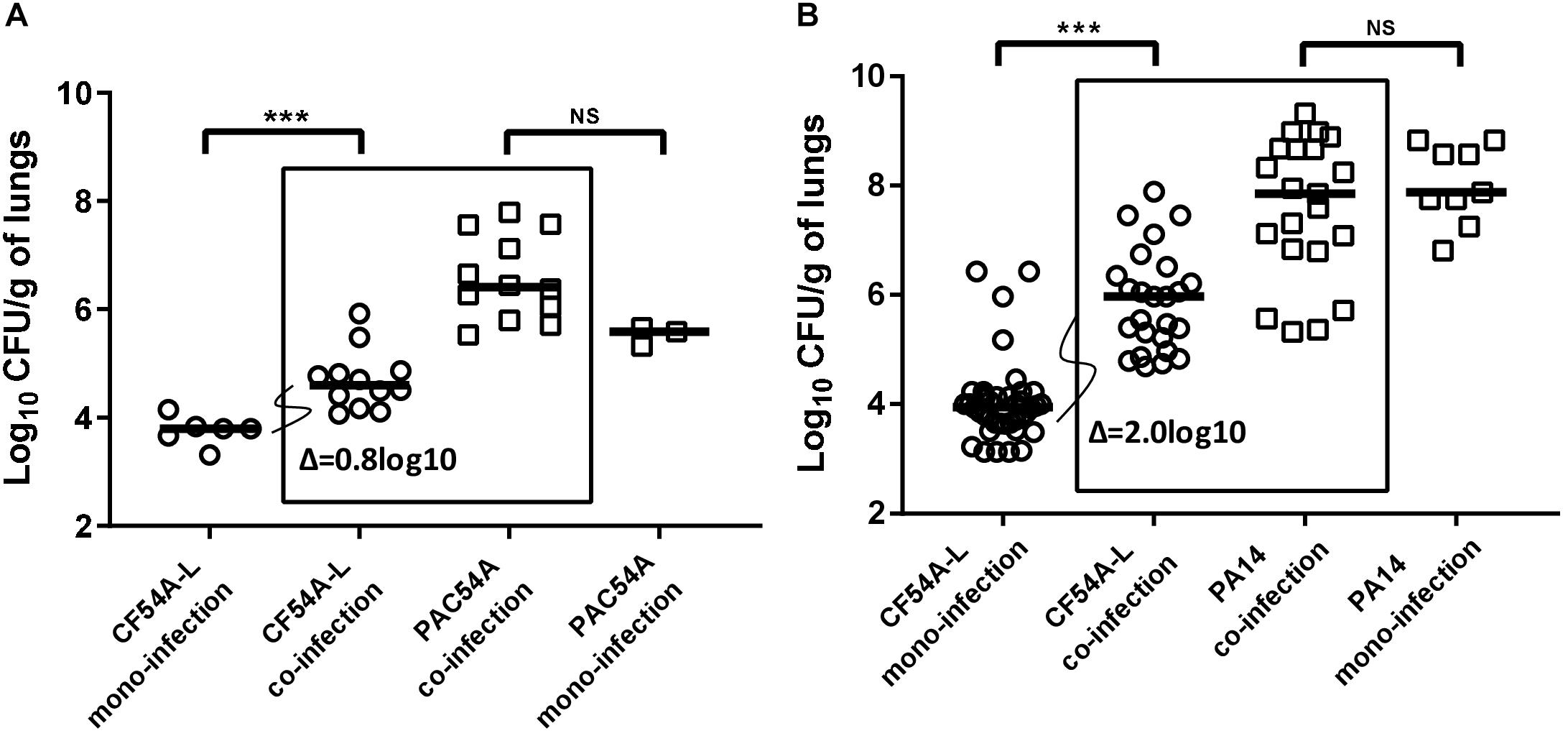
Figure 2. Mouse pulmonary mono or co-infections with S. aureus and P. aeruginosa. CFUs were determined 24 h post-infection by plating lung homogenates on TSA supplemented with polymyxin B (S. aureus) or with rifampicin (P. aeruginosa). CFUs obtained from co-infections are presented in boxes. For co-infections, the starting inoculum was equivalent to the sum of each inoculum used in mono-infections. The pairs tested were P. aeruginosa PAC54A and S. aureus CF54A-L (A), and P. aeruginosa PA14 and S. aureus CF54A-L (B). Each symbol represents the lungs of one mouse. The median for each group is indicated by the horizontal bar. Statistical differences between the median log10 CFU per gram of lungs for mono and co-infections for both P. aeruginosa and S. aureus were determined with a Mann–Whitney test: NS, not statistically significant, p > 0.05; ∗∗∗p < 0.001.
Searching for P. aeruginosa Virulence-Associated Factors Helping S. aureus Colonization
In an attempt to determine if a specific P. aeruginosa virulence-associated factor was responsible for the promotion of S. aureus lung colonization, PA14 mutants displaying different alterations, ranging from global virulence regulators to specific virulence factors (Table 1), were tested in the co-infection assay (all used at an inoculum of ∼2 × 106 CFU). S. aureus colonization was still improved when co-infecting with any of the PA14 mutants tested (PA14ΔrhlRΔlasR, 2.1 log10 median increase; PA14ΔlasA, 1.7 log10 increase; PA14ΔpqsA, 1.8 log10 increase) but the promotion was less than that observed with wild-type PA14 (2.5 log10 increase) (Figure 3). Therefore, none of the specific P. aeruginosa factors tested here could be identified as crucial for helping S. aureus colonization using this approach.
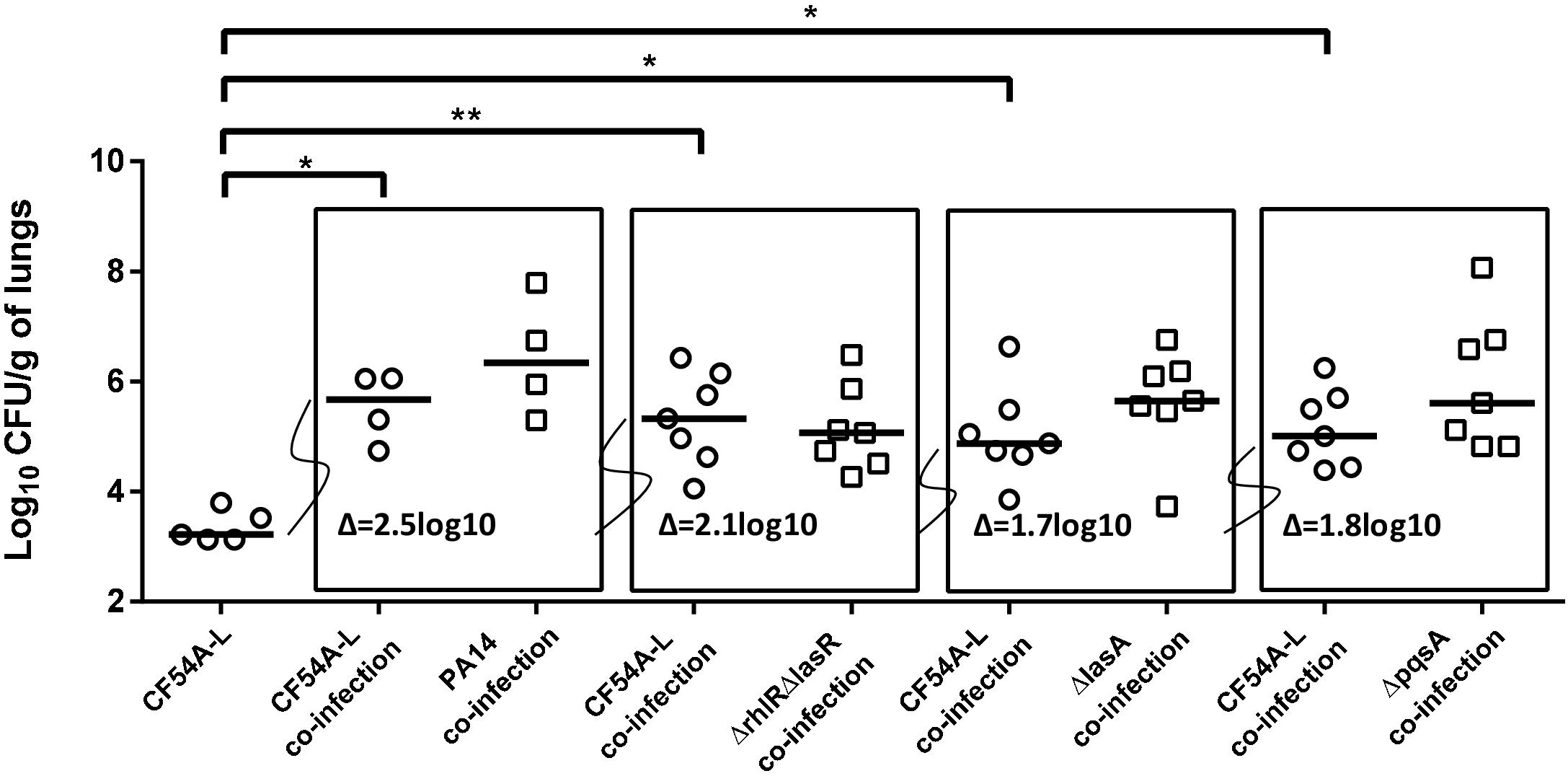
Figure 3. Mouse pulmonary mono or co-infections with S. aureus CF54A-L and P. aeruginosa PA14 and mutants. CFUs were determined 24 h post-infection by plating lung homogenates on TSA supplemented with polymyxin B (S. aureus) or with rifampicin (P. aeruginosa). CFUs obtained from co-infections are presented in boxes. For co-infections, the starting inoculum was equivalent to the sum of each inoculum used in mono-infection. The pairs tested were S. aureus CF54A-L and P. aeruginosa PA14; CF54A-L and PA14ΔrhlRΔlasR; CF54A-L and PA14ΔlasA; CF54A-L and PA14ΔpqsA. Each symbol represents the lungs of one mouse. The median for each group is indicated by the horizontal bar. Statistical differences between the median log10 CFU per gram of lungs for S. aureus CF54A-L mono and co-infections were determined with a Mann–Whitney test: NS, not statistically significant, p > 0.05; ∗p < 0.05; ∗∗p < 0.01.
Searching for S. aureus Virulence-Associated Factors Promoting Its Own Colonization During Co-infection
Since it was determined that P. aeruginosa mutants showing some attenuation in virulence still improved S. aureus colonization proportionally to their own colonization, the contribution of S. aureus in this phenomenon was also examined using a similar approach. Co-infections were performed using P. aeruginosa PA14 and various S. aureus mutants (all used at an inoculum of 2 × 106 CFU). The contribution of the global regulators SigB and Agr was investigated since they both are major virulence regulators in SCVs and wild-type strains (Novick and Geisinger, 2008; Mitchell et al., 2013). Therefore, given their large influence over S. aureus virulence, it seemed plausible they could be implicated in the improved colonization of S. aureus in presence of P. aeruginosa and could eventually lead to the identification of a precise responsible S. aureus factor. However, when tested in the lung co-infection model, NewbouldΔsigB colonization was increased by the presence of PA14, in a similar manner to that observed for the S. aureus wild-type counterpart Newbould (Figure 4). Similarly, 8325-4 is a S. aureus strain naturally deficient in SigB activity because of its defective rsbU alleles. Strain 8325-4 was thus compared to SH1000, an isogenic strain with a restored rsbU allele (O’Neill, 2010). Again, PA14 positively affected colonization of either S. aureus strains (8325-4 and SH1000) compared to S. aureus mono-infections. Next, using the same strain background (8325-4) we investigated a mutant for the fibronectin-binding proteins A and B, which are known multi-purpose adhesins for S. aureus (Josse et al., 2017). Despite the absence of FnbAB, Figure 4 shows that P. aeruginosa still helps S. aureus lung colonization. The role of the S. aureus Agr system was then examined. Agr is a global regulator of S. aureus virulence that influences expression of exoproducts and surface proteins. Nevertheless, NRS155, an agr-null derivative of NRS149, was still significantly helped in its lung colonization by P. aeruginosa PA14. In conclusion, neither the alternative transcription factor SigB, the adhesins FnbAB, or the Agr regulator seem to contribute to the mechanism by which P. aeruginosa helps the colonization of the lungs by S. aureus.
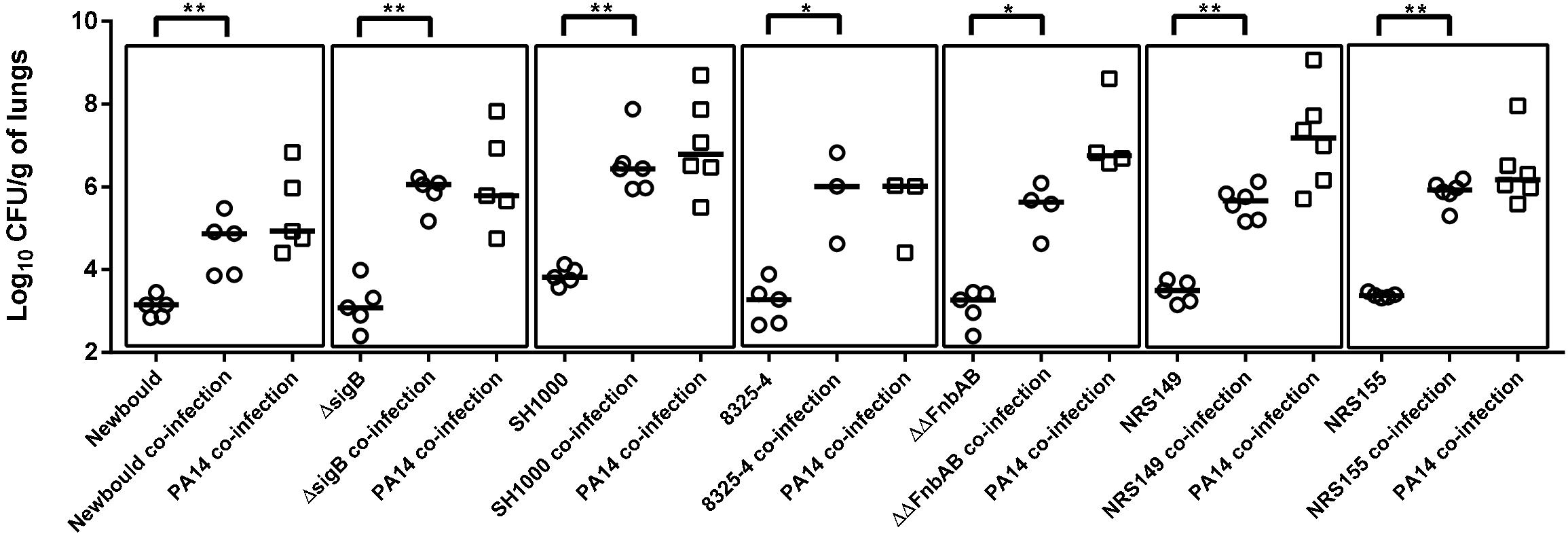
Figure 4. Mono or mixed mouse pulmonary infections with S. aureus virulence mutants and P. aeruginosa. CFUs were determined 24 h post-infection by plating lung homogenates on TSA supplemented with polymyxin B (S. aureus) or with rifampicin (P. aeruginosa). CFUs obtained from co-infections are presented in boxes. For co-infections, the starting inoculum was equivalent to the sum of each inoculum used in mono-infections. The pairs tested were S. aureus Newbould and P. aeruginosa PA14; NewbouldΔsigB and PA14; SH1000 and PA14; 8325-4 and PA14; 8325-4ΔΔfnbAB and PA14; NRS149 and PA14; NRS155 and PA14. Each symbol represents the lungs of one mouse. The median for each group is indicated by the horizontal bar. Statistical differences between the median log10 CFU per gram of lungs for every S. aureus mono and co-infection were determined with a Mann–Whitney test: NS, not statistically significant, p > 0.05; ∗p < 0.05; ∗∗p < 0.01.
P. aeruginosa Improves S. aureus Colonization in a Dose-Dependent Manner
Following the previous results, we formulated the hypothesis that during a co-infection, the higher the titer of P. aeruginosa in the lungs, the more S. aureus colonization would be enhanced. To test this hypothesis, the pulmonary co-infection model was employed using a collection of clinical and mutant strains. We used 35 P. aeruginosa strains in combination with 10 S. aureus strains and different inoculum sizes were used for some strains for a total of 200 co-infections. For each experimental co-infection, the CFU counts in the lungs for P. aeruginosa and for S. aureus were determined after 24 h and plotted in Figure 5A, which includes results using the P. aeruginosa PA14 mutants and S. aureus mutants of Figures 3, 4, respectively. Based on Figure 5A, it was clear that higher CFU counts of P. aeruginosa increased the colonization of S. aureus in a dose-dependent manner (Figure 5A, right panel, linear regression R2 = 0.6116, p < 0.0001). Figure 5A also shows that most of the S. aureus strains used in this model as mono-infections do not colonize the lungs very efficiently (median log10 CFU/g of lung of 3.8, Figure 5A, left panel). This shows once again that higher titers of P. aeruginosa in infected lungs drive upward and not downward the colonization potential of S. aureus.
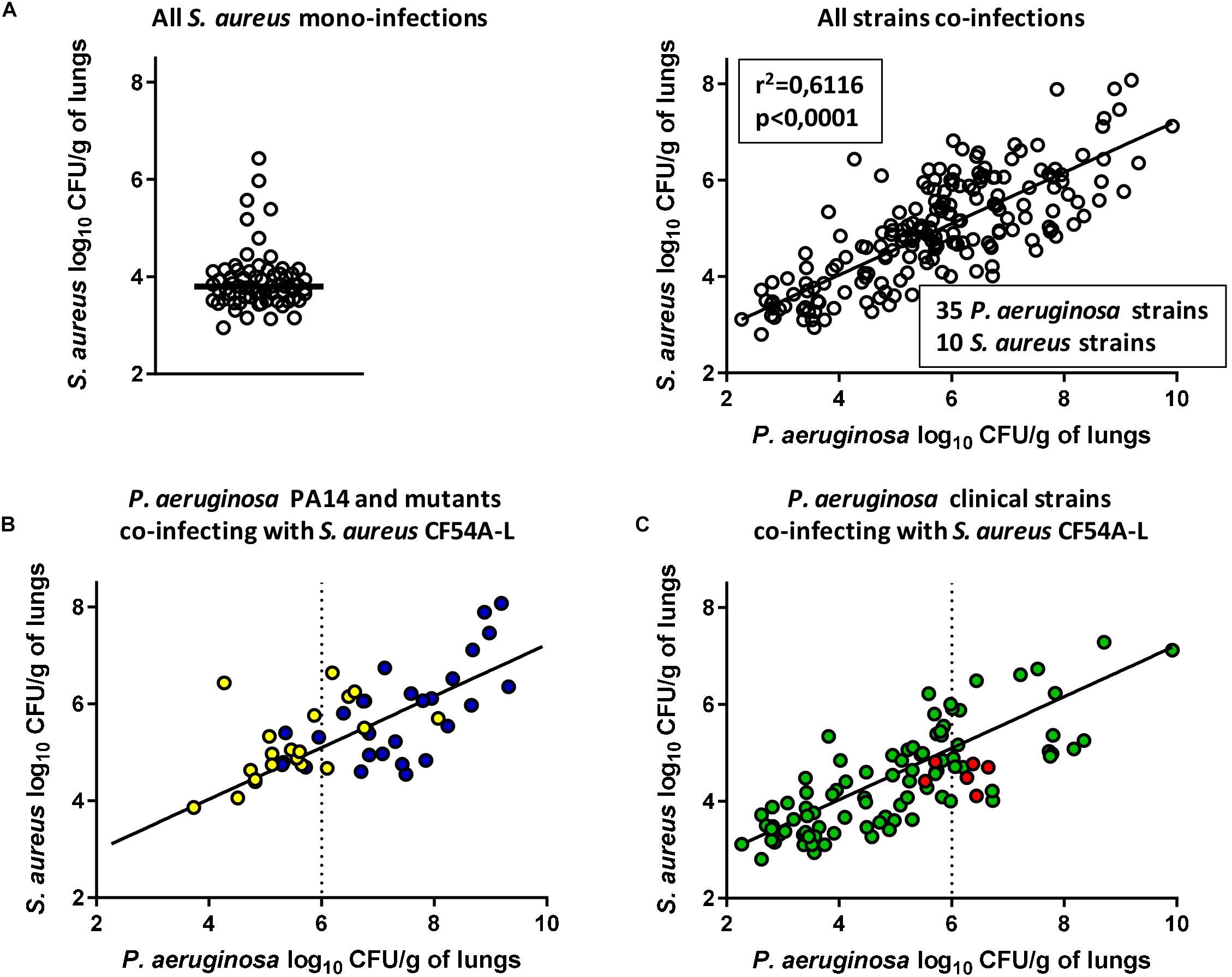
Figure 5. Compilation of mixed mouse pulmonary infections with S. aureus and P. aeruginosa. CFUs were determined 24 h post-infection by plating lung homogenates on TSA supplemented with polymyxin B (S. aureus) or with rifampicin (P. aeruginosa). For co-infections, the starting inoculum was equivalent to the sum of each inoculum used in mono-infections. The strains tested in “All S. aureus mono-infections” were S. aureus CF07-L, CF54A-L, and CF112A-L at an inoculum of 2 × 106CFU (A, left). In “All strains co-infections,” the strains tested were CF07-L, CF54A-L, CF112A-L, and every S. aureus strain indicated in Table 1, co-infecting with P. aeruginosa PA14, PAC54A, and PAC112A, all PA14 mutants indicated in Table 1 and 29 clinical isolates indicated in Supplementary Table S1 (A, right). In “P. aeruginosa PA14 and mutants co-infecting with S. aureus CF54A-L,” the strains tested were CF54A-L co-infecting with PA14 and its associated virulence mutants at an inoculum of 2 × 106 CFU (B). In “P. aeruginosa clinical strains co-infecting with S. aureus CF54A-L,” the strains tested were CF54A-L co-infecting with every P. aeruginosa clinical strain, including PAC54A, at an inoculum of 2 × 107 CFU (C). Each symbol represents the lungs of one mouse. Statistical significance of the trendline of all strain co-infections was determined with a linear regression test. Blue dots represent co-infections with PA14; yellow dots represent co-infections with PA14 virulence mutants; green dots represent co-infections with clinical P. aeruginosa strains; and red dots represent co-infections with PAC54A.
To better understand the correlation between P. aeruginosa and S. aureus colonization, the data from Figure 5A were analyzed separately for different P. aeruginosa subgroups. First, results for P. aeruginosa PA14 and its mutants (all used at an inoculum of ∼2.0 × 106 CFU) in co-infection with S. aureus strain CF54A-L are shown in Figure 5B. While infection by the mutant strains was less productive than that parent PA14 infections (generally yielding less than 107 CFU/g of lungs), colonization of S. aureus was still proportional to that of P. aeruginosa.
Data for P. aeruginosa clinical strains (all used at an inoculum of ∼2 × 107 CFU) in co-infection with S. aureus strain CF54A-L (shown to be susceptible to P. aeruginosa antagonism in vitro, Figures 1E,F) demonstrated once again the same correlation (Figure 5C). P. aeruginosa strain PAC54A (the non-antagonistic strain in vitro, Figures 1C,D) also fitted the linear regression (Figure 5C, red symbols) although this P. aeruginosa strain was less productive and accordingly, S. aureus colonization was less abundant. Similar to the P. aeruginosa PA14 mutants deficient in a variety of virulence-associated products (Figures 3, 5B), most P. aeruginosa clinical strains yielded less than 107 CFU/g of lungs (Figure 5C). Data for specific S. aureus strains and mutants, all in co-infection with P. aeruginosa PA14, are plotted in Supplementary Figure S3. No particular S. aureus mutant seemed to diverge from the trend described above.
Overall, each P. aeruginosa strain tested enhanced S. aureus colonization proportionally to their own ability to infect the lungs. P. aeruginosa clinical strains generally yielded lower levels of lung colonization compared to the prototypic strain PA14.
The Contribution of P. aeruginosa to S. aureus Colonization Is Independent of MPO Induction
Since no P. aeruginosa or S. aureus virulence factors could be identified as an essential determinant in the mechanism by which P. aeruginosa stimulates S. aureus colonization, other possible causes possibly involved in the beneficial effect of P. aeruginosa on the colonization of S. aureus were investigated. It was thus hypothesized that P. aeruginosa could exacerbate a pro-inflammatory response, which could help S. aureus colonization. MPO activity was therefore measured for a series of co-infections in the mouse since it was recently found to be a good indicator of inflammation (Côté-Gravel et al., 2016). S. aureus CF54A-L was used in all co-infections together with a variety of P. aeruginosa strains showing different levels of virulence and colonization. All P. aeruginosa strains were compared, using an inoculum of ∼2 × 106 CFU for PA14 and mutants and an inoculum of ∼2 × 107 CFU for PAC54A and P. aeruginosa clinical strains. Interestingly, only the co-infection of P. aeruginosa PAC54A and S. aureus CF54A-L resulted in a significantly higher MPO score (vs. the PBS control) even though the level of colonization for both species in that co-infection was much less than that achieved by the PA14–CF54A-L pair (Figure 6). This clearly indicates that a high colonization of P. aeruginosa does not necessarily translate into a high MPO score and that the level of MPO does not correlate with the ability of P. aeruginosa to promote S. aureus colonization. Also inversely, low MPO induction (as seen with the PA14 co-infections) does not better promote S. aureus colonization. Hence, the level of inflammation, as inferred by MPO production elicited by P. aeruginosa or the co-infection, does not seem to be involved in the mechanism by which P. aeruginosa helps S. aureus colonization.
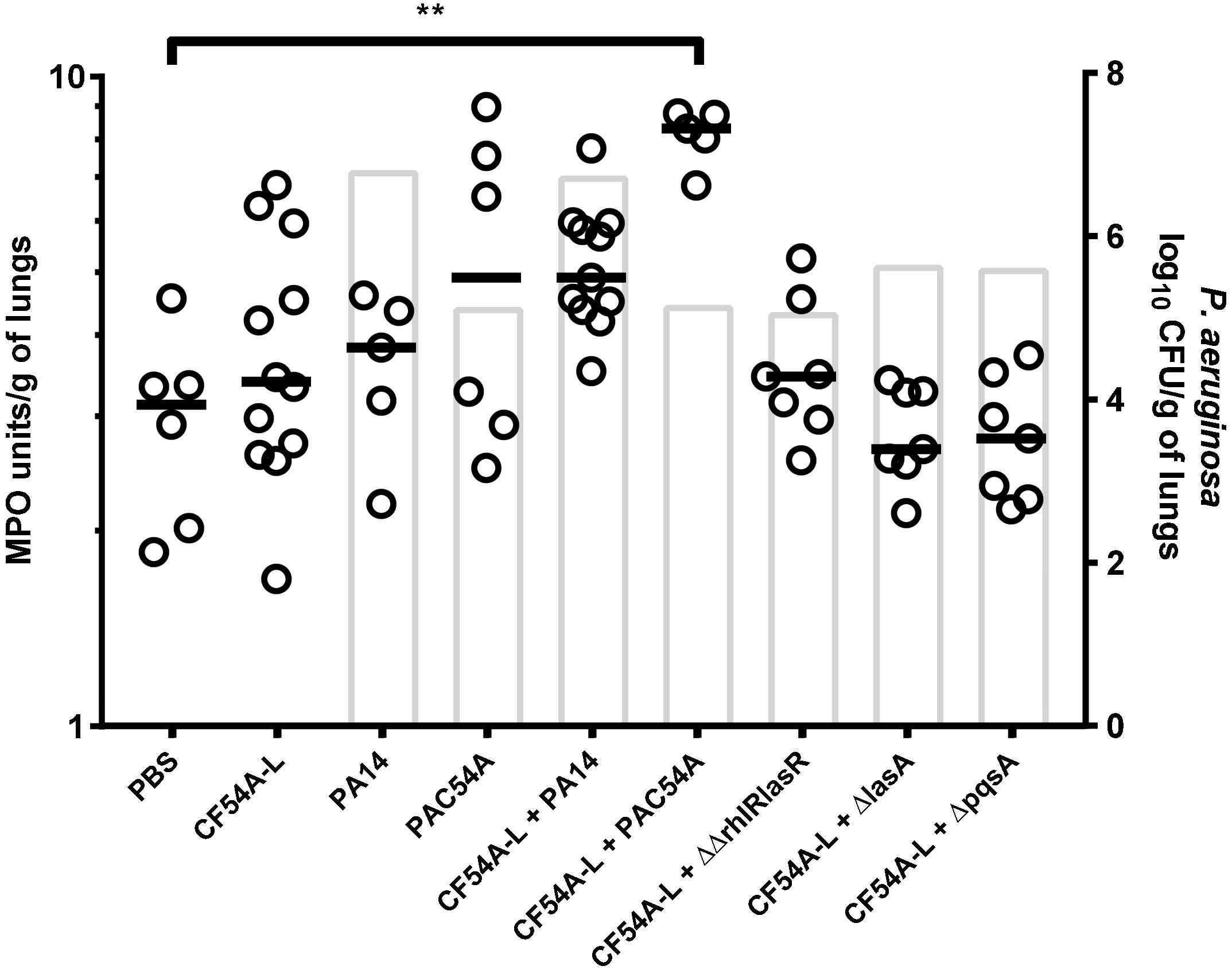
Figure 6. MPO activity of lungs mono or co-infected with S. aureus and P. aeruginosa. MPO units were determined using an enzymatic assay. CFUs were determined 24 h post-infection by plating lung homogenates on TSA supplemented with polymyxin B (S. aureus) or with rifampicin (P. aeruginosa). For co-infections, the starting inoculum was equivalent to the sum of each inoculum used in mono-infections. The strains tested were S. aureus CF54A-L; P. aeruginosa PA14, PA14ΔrhlRΔlasR, PA14ΔlasA, PA14ΔpqsA, and PAC54A. The median for MPO activity in each group is indicated by the horizontal bar, while open dots represent the MPO activity for an individual lung. Columns indicate the median P. aeruginosa colonization for each group. Statistical differences between the median MPO activity for PBS control and the other groups were determined with a Kruskal–Wallis test: ∗∗p < 0.01
P. aeruginosa Induces Overexpression of Known S. aureus Cell Surface Receptors
Since neither virulence-associated factors nor the level of inflammation could explain the promotion of S. aureus colonization by P. aeruginosa, we looked for other possibilities inspired by knowledge surrounding bacterial and viral co-infections. Indeed, it has been shown that rhinoviruses can promote cellular ICAM-1 and integrin α5β1 expression (Passariello et al., 2006), which have been previously described as cell surface receptors for S. aureus. Therefore, we verified by RT-qPCR if P. aeruginosa could induce a similar effect on lung tissue during infection. To this end, PA14 effect was investigated, as it was shown to be a strong inducer of S. aureus colonization. Figure 7A shows that a P. aeruginosa PA14 mono- or co-infection with S. aureus CF54A-L induced a significant increase in the expression of ICAM-1 in comparison to the PBS control. In addition, the expression of ITGA-5, which associates with the β1 subunit to form the α5β1 integrin, was also enhanced by the co-infection (Figure 7B), while on the other hand, a mono-infection by S. aureus CF54A-L did not change the level of expression of either ICAM-1 or ITGA-5. For each biological sample tested, a GAPDH internal control was added to ensure adequate RNA integrity and for relative quantification of gene expression levels. At least five biological replicates were produced for each infection group. Hence, PA14, during both mono- and co-infections, appeared to significantly stimulate the expression of these two cellular genes, which might in turn contribute to S. aureus colonization.
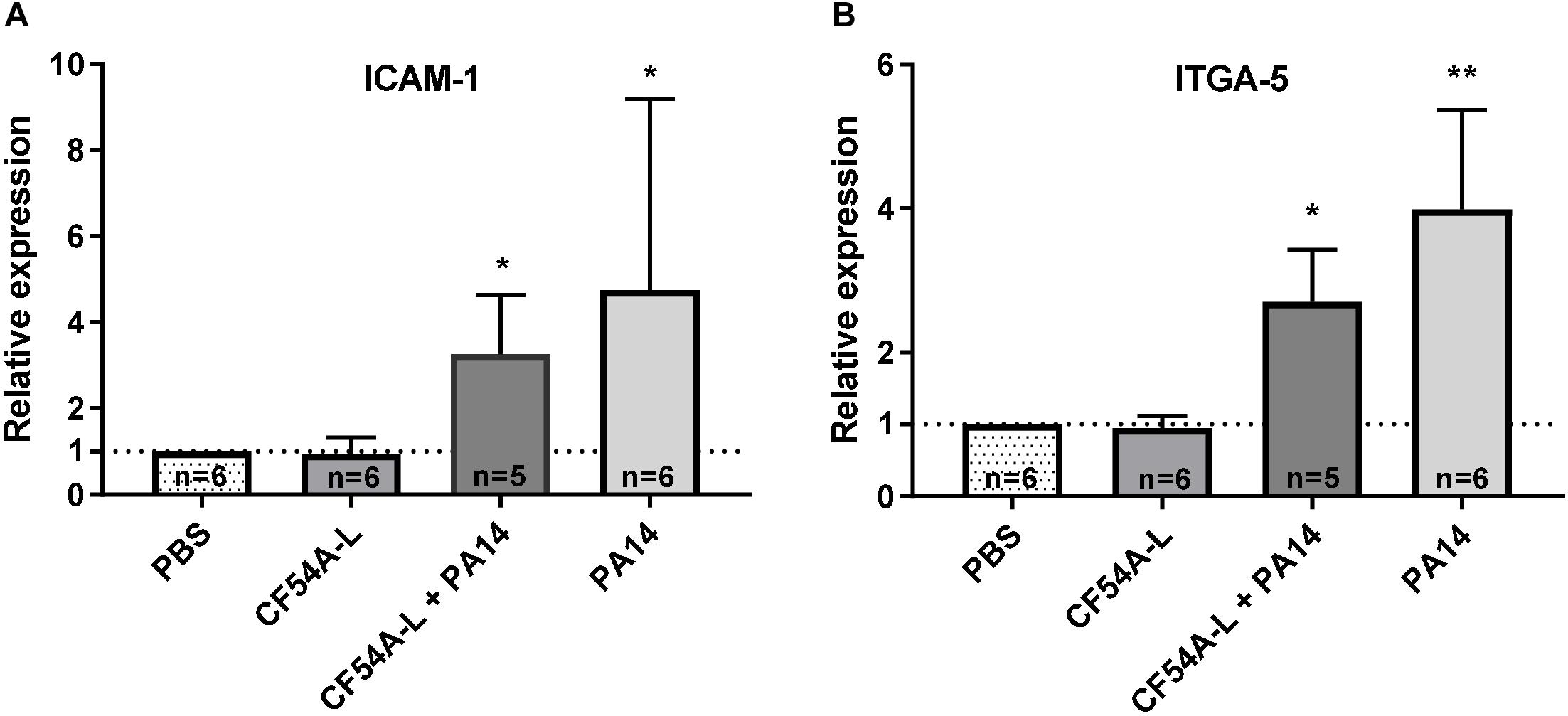
Figure 7. Relative expression of cellular ICAM-1 (A) and ITGA-5 (B) genes in lung tissues during mono or co-infections with S. aureus CF54A-L and P. aeruginosa PA14. Total RNA was extracted from lung tissues 24 h post-infection. Expression was quantified by RT-qPCR and normalized to the PBS control. GAPDH expression was used as an internal control for each individual sample. Quantity of biological replicates (n) is indicated for each group. Statistical differences between the relative expression of both genes in the infection groups compared to the PBS control group were determined with a Kruskal–Wallis test. ∗p < 0.05; ∗∗p < 0.01.
Discussion
This study aimed to gain a better understanding of the interactions between P. aeruginosa and S. aureus, which are allegedly antagonistic to each other in vitro, although they are commonly co-isolated from chronically infected CF patients. Also, we previously demonstrated that clinical P. aeruginosa and S. aureus co-isolates from adult CF patients do not necessarily display the same properties as prototypical antagonistic strains in vitro. One such a discrepancy between prototypic and clinical isolates properties was the relative ability of P. aeruginosa to induce biofilm production by S. aureus in vitro, a phenomenon strongly correlated to the quantity of HQNO produced by the clinical isolates of P. aeruginosa (Fugère et al., 2014). Studying further P. aeruginosa–S. aureus co-isolates should provide the necessary data to close the gap between the seemingly opposite in vitro and in vivo behaviors. Coherently with data displayed in our previous study, we demonstrated here that P. aeruginosa and S. aureus co-isolates do not always antagonize each other in vitro. Such results indicate that P. aeruginosa may become less aggressive toward S. aureus in the CF lung environment. This may either be the result of an adaptation toward S. aureus or to the hostile host environment and inflammatory response. By reducing its production of alkyl-quinolones and other QS factors, P. aeruginosa is less likely to trigger an intense immune response. At the same time, with a reduced production of HQNO and alkyl-quinolones, P. aeruginosa dampens its inhibitory effect against S. aureus (Hoffman et al., 2006). This could be the case for P. aeruginosa PAC54A, which produces very little HQNO and other QS factors compared to PA14 (Fugère et al., 2014; Supplementary Material), and which displays no antagonism toward its S. aureus co-isolate CF54A-L (Figures 1C,D). Besides, PAC22A, which produces a similar amount of HQNO compared to that of PA14 (Fugère et al., 2014; Supplementary Material), also did not reduce the viability of its co-isolate CF22A-L although it still stimulated the formation of slow-growing colonies (Figures 1G,H). This shows the complex interactions that can exist between these bacterial species. Overall, our findings establish that contrary to the long-held belief, P. aeruginosa does not always antagonize S. aureus in vitro and the effect of P. aeruginosa on S. aureus viability and phenotypes can vary.
To our knowledge, very few studies have specifically examined the interaction between S. aureus and P. aeruginosa strains co-isolated from CF patients. Since co-isolates do not necessarily interact as prototypical strains in vitro, we hypothesized that co-isolates could also behave differently in vivo, which in turn could provide some explanation on why S. aureus and P. aeruginosa are frequently co-isolated from the CF lung. According to our findings, it seems that the nature of the interaction between P. aeruginosa and S. aureus in vitro is not a good indicator of the outcome of a co-infection in vivo. Unexpectedly, the success of S. aureus colonization during co-infection correlated with the extent of P. aeruginosa colonization in the lungs, irrespective of the type of interactions (indifference or antagonism) between these two microorganisms in vitro. Also, using a series of P. aeruginosa PA14 mutants, none of the tested virulence-associated factor in this current study was specifically identified as responsible for the promotion of S. aureus colonization during co-infection. Still, as the ability of the P. aeruginosa clinical strains to infect and promote S. aureus co-colonization ranged from low to high, it would be important to identify P. aeruginosa properties that may be conserved among the best colonizers, and therefore the best inducer of S. aureus colonization. Whole-genome sequencing is currently underway to compare P. aeruginosa isolates that are “low” and “high” inducers of S. aureus colonization to identify P. aeruginosa factors or mutations that best profit S. aureus in vivo.
The contribution of S. aureus key virulence regulators or effectors to the outcome of P. aeruginosa–S. aureus co-infections was also investigated in this study. It is known that S. aureus can adopt the SCV phenotype in the presence of prototypic P. aeruginosa strains which produce HQNO (Hoffman et al., 2006). SCVs are proficient in the invasion of non-professional phagocytic cells, which in turn helps them to evade the immune system (Mitchell et al., 2011). It was therefore possible that P. aeruginosa enhances S. aureus colonization in vivo by inducing SCV-like properties. Since the alternative transcription factor SigB is a dominant regulator of virulence in SCVs (Mitchell et al., 2013), we tested the colonization ability of two SigB deleted or altered mutants in the presence of P. aeruginosa. Using such an approach, we were not able to demonstrate a contribution of S. aureus SigB to the outcome of co-infection in mice. However, since the murine infection model was acute and not chronic, it is possible that in such conditions, induction of SCVs, cellular invasion, and intracellular replication might have been less significant. Alternatively, another hypothesis was that P. aeruginosa might affect and positively upregulate virulence in S. aureus but again, an agr mutant was not altered in its ability to co-colonize the lung with P. aeruginosa even though Agr is an important virulence activator in prototypic S. aureus strains (Novick and Geisinger, 2008).
These results indicate that P. aeruginosa is probably not directly affecting S. aureus virulence. Since P. aeruginosa inhibits or is at best indifferent toward S. aureus in vitro, we then can only infer that the environment must be modified by P. aeruginosa in a way that it promotes S. aureus colonization in vivo. P. aeruginosa can induce inflammation with a panel of different virulence factors (Wieland et al., 2002; Lin and Kazmierczak, 2017). While inflammation is necessary for controlling bacterial infections, an over-stimulated inflammatory response can provoke host tissue damage and alter bacterial clearance (Lin and Kazmierczak, 2017). Also, we have shown that activation of NF-κB by LPS and TNF-α increases S. aureus invasion of pulmonary cells cultured in vitro (Mitchell et al., 2011). It is therefore possible that inflammation provoked by P. aeruginosa can contribute to S. aureus colonization. We investigated this possibility by quantifying MPO, as a marker for inflammation. However, we showed here that the P. aeruginosa strain inducing the most MPO production in the mice lungs was PAC54A although it was not as efficient as the prototypic strain PA14 at promoting S. aureus infection. Therefore, it was not possible to associate the level of inflammation (based on MPO activity) with the ability of P. aeruginosa to enhance S. aureus colonization.
It is now well-recognized that viral infections of the respiratory tract can enhance the possibility of bacterial superinfections and this research topic has been reviewed (Fedy Morgene et al., 2018). Rhinoviruses have already been identified as microorganisms able to promote S. aureus colonization in vivo. Explicitly, rhinoviruses increase S. aureus colonization by a mechanism involving the release of IL-6, IL-8, and the overexpression of ICAM-1 (Passariello et al., 2006). Moreover, rhinoviruses also upregulate integrin α5β1 transcription (Kim et al., 2015). This integrin is one of the main pathways by which S. aureus can invade non-professional phagocytic cells (Josse et al., 2017). Hence, based on such a precedent for in vivo cooperation between two microorganisms, we investigated the expression of ICAM-1 and ITGA-5 (a marker for integrin α5β1) in mouse lung tissue infected by P. aeruginosa and S. aureus. Interestingly, P. aeruginosa mono- or co-infections could indeed increase expression of both host cell components, whereas a mono-infection with S. aureus did not (Figure 7). ICAM-1 is responsible for the transmigration of leukocytes during an infection, which occurs through the endothelium to the site of the lesions (Springer, 1990). P. aeruginosa induces its overexpression, possibly through tissue damage and the inflammatory process. Likewise, Gram negative LPS was shown to induce expression of ITGA-5 (Roman et al., 2004; Sampaio et al., 2010). It is thus plausible that S. aureus might benefit from these transcriptional changes to adhere to host cells and increase its colonization of the lung tissue when P. aeruginosa is present. Moreover, while we found that ITGA-5 and ICAM-1 expression was upregulated in lung cells during P. aeruginosa infection, many other transcriptional changes could definitely occur. Conducting a dual RNAseq on either co-infected lungs (or on a mixed infection in a cell culture model) would lead to a better understanding of the changes of the host cells occurring in presence of P. aeruginosa and S. aureus (Westermann et al., 2017).
Staphylococcus aureus possesses a wide variety of adhesins (Fedy Morgene et al., 2018; Foster, 2019). S. aureus FnBPs are bacterial adhesins known to interact with the integrin α5β1 through fibronectin (Mitchell et al., 2008). Since we showed that colonization of S. aureus lacking FnBPs is still promoted by co-infection with P. aeruginosa, such a S. aureus mutant must have other means to interact with the host cells. For example, the S. aureus protein EAP, which also binds fibronectin (Sinha and Fraunholz, 2010), can perhaps compensate for a lack of FnBPs. Also, S. aureus teichoic acids were shown to contribute to binding to endothelial cells (Weidenmaier et al., 2005). Consequently, a S. aureus strain lacking FnBPs should still adhere to host cells and colonize tissues (Josse et al., 2017). Furthermore, ICAM-1 and integrin α5β1 can both individually allow S. aureus host cell binding (Sinha et al., 1999; Passariello et al., 2006), and according to the mouse ENCODE transcriptome data, ICAM-1 is up to 10 times more prevalent than ITGA-5 in mice lungs (Yue et al., 2014). Likewise, ICAM-1 is overexpressed in comparison to ITGA-5 in the human lungs (Fagerberg et al., 2014). Hence, prototypic S. aureus and the FnBPs mutant may both have predominantly interacted with ICAM-1 to permit colonization in the murine lung infection model.
By using an acute (24 h) lung infection model, we were able to examine a large panel of bacterial strains, including mutants and clinical isolates. The model rapidly granted us robust results and increased statistical power. Not only was it possible to establish a model that helped us to gain some insights that could possibly explain the often worse clinical prognosis of S. aureus–P. aeruginosa coinfections, but we could also test many different hypotheses regarding the mechanism by which P. aeruginosa improves S. aureus colonization. However, infections afflicting CF patients are mostly chronic, therefore a chronic lung infection model would probably better mimic the real clinical conditions. Future experiments should be conducted using a chronic infection model to confirm results obtained in the present study.
Overall, we showed that P. aeruginosa promotes S. aureus colonization in a dose-dependent manner in vivo. The mechanism could involve inflammation and induction of ICAM-1 and ITGA-5, which would allow a better adhesion and colonization of S. aureus. Further experiments are required to identify precisely how S. aureus colonization benefits from P. aeruginosa impact on the lungs.
Data Availability Statement
All datasets generated for this study are included in the article/Supplementary Material.
Ethics Statement
The animal study was reviewed and approved by the institutional ethics committee on animal experimentation of the Faculté des Sciences of Université de Sherbrooke.
Author Contributions
GM did the growth kinetics experiments in vitro, co-cultured petri dish assays, lung infections in mice, MPO extraction and quantification, verified ICAM-1 and ITGA-5 expression by qPCR, and wrote the article. J-PL contributed to the growth kinetics experiments. EB worked to develop the lung infection model. EF supervised the bacteria strains isolation. AC supervised the bacteria strains isolation and clinical evaluation of CF patients. FM contributed to the working plan and manuscript writing, and is the corresponding author.
Funding
This study was supported by grant 3010 from the Cystic Fibrosis Canada and by grant 2015-05916 from the Natural Sciences and Engineering Research Council of Canada (NSERC). GM received studentships from the NSERC and from the Fonds de Recherche du Québec – Nature et Technologies.
Conflict of Interest
The authors declare that the research was conducted in the absence of any commercial or financial relationships that could be construed as a potential conflict of interest.
Acknowledgments
We thank E. Deziel (INRS – Institut Armand Frappier, Laval, QC, Canada) for providing the series of P. aeruginosa PA14 mutants.
Supplementary Material
The Supplementary Material for this article can be found online at: https://www.frontiersin.org/articles/10.3389/fmicb.2019.02880/full#supplementary-material
References
Boulanger, S., Mitchell, G., Bouarab, K., Marsault, É, Cantin, A., Frost, E. H., et al. (2015). Bactericidal effect of tomatidine-tobramycin combination against methicillin-resistant Staphylococcus aureus and Pseudomonas aeruginosa is enhanced by interspecific small-molecule interactions. Antimicrob. Agents Chemother. 59, 7458–7464. doi: 10.1128/AAC.01711-1715
Castellani, C., and Assael, B. M. (2017). Cystic fibrosis: a clinical view. Cell. Mol. Life Sci. 74, 129–140. doi: 10.1007/s00018-016-2393-2399
Cigana, C., Bianconi, I., Baldan, R., De Simone, M., Riva, C., Sipione, B., et al. (2018). Staphylococcus aureus impacts Pseudomonas aeruginosa chronic respiratory disease in murine models. J. Infect. Dis. 217, 933–942. doi: 10.1093/infdis/jix621
Clinical and Laboratory Standards Institute [CLSI] (2018). Methods for Dilution Antimicrobial Susceptibility tests for Bacteria that Grow Aerobically, 11th Edn. West Valley Road, PA: CLSI.
Côté-Gravel, J., Brouillette, E., Obradović, N., Ster, C., Talbot, B. G., and Malouin, F. (2016). Characterization of a vraG mutant in a genetically stable staphylococcus aureus small-colony variant and preliminary assessment for use as a live-attenuated vaccine against intrammamary infections. PLoS One 11:e0166621. doi: 10.1371/journal.pone.0166621
Cystic Fibrosis Canada (2018). The Canadian Cystic Fibrosis Registry Annual Report. Available at: www.cysticfibrosis.ca. (accessed December 11, 2018).
Dekimpe, V., and Déziel, E. (2009). Revisiting the quorum-sensing hierarchy in Pseudomonas aeruginosa: the transcriptional regulator RhlR regulates LasR-specific factors. Microbiology 155, 712–723. doi: 10.1099/mic.0.022764-22760
Déziel, E., Lépine, F., Milot, S., He, J., Mindrinos, M. N., Tompkins, R. G., et al. (2004). Analysis of Pseudomonas aeruginosa 4-hydroxy-2-alkylquinolines (HAQs) reveals a role for 4-hydroxy-2-heptylquinoline in cell-to-cell communication. Proc. Natl. Acad. Sci. U.S.A. 101, 1339–1344. doi: 10.1073/pnas.0307694100
Fagerberg, L., Hallström, B. M., Oksvold, P., Kampf, C., Djureinovic, D., Odeberg, J., et al. (2014). Analysis of the human tissue-specific expression by genome-wide integration of transcriptomics and antibody-based proteomics. Mol. Cell. Proteomics 13, 397–406. doi: 10.1074/mcp.M113.035600
Fedy Morgene, M., Botelho-Nevers, E., Grattard, F., Pillet, S., Berthelot, P., Pozzetto, B., et al. (2018). Staphylococcus aureus colonization and non-influenza respiratory viruses: interactions and synergism mechanisms. Virulence 9, 1354–1363. doi: 10.1080/21505594.2018.1504561
Foster, T. J. (2019). The MSCRAMM family of cell-wall-anchored surface proteins of gram-positive cocci. Trends Microbiol. 27, 927–941. doi: 10.1016/j.tim.2019.06.007
Fugère, A., Lalonde Séguin, D., Mitchell, G., Déziel, E., Dekimpe, V., Cantin, A. M., et al. (2014). Interspecific small molecule interactions between clinical isolates of Pseudomonas aeruginosa and Staphylococcus aureus from adult cystic fibrosis patients. PLoS One 9:e86705. doi: 10.1371/journal.pone.0086705
Goerke, C., and Wolz, C. (2010). Adaptation of Staphylococcus aureus to the cystic fibrosis lung. Int. J. Med. Microbiol. 300, 520–525. doi: 10.1016/j.ijmm.2010.08.003
Gómez, M. I., and Prince, A. (2007). Opportunistic infections in lung disease: Pseudomonas infections in cystic fibrosis. Curr. Opin. Pharmacol. 7, 244–251. doi: 10.1016/j.coph.2006.12.005
Harun, S. N., Wainwright, C., Klein, K., and Hennig, S. (2016). A systematic review of studies examining the rate of lung function decline in patients with cystic fibrosis. Paediatr. Respir. Rev. 20, 55–66. doi: 10.1016/j.prrv.2016.03.002
Hoffman, L. R., Déziel, E., D’Argenio, D. A., Lépine, F., Emerson, J., McNamara, S., et al. (2006). Selection for Staphylococcus aureus small-colony variants due to growth in the presence of Pseudomonas aeruginosa. Proc. Natl. Acad. Sci. U..S..A. 103, 19890–19895. doi: 10.1073/pnas.0606756104
Hubert, D., Réglier-Poupet, H., Sermet-Gaudelus, I., Ferroni, A., Le Bourgeois, M., Burgel, P.-R., et al. (2013). Association between Staphylococcus aureus alone or combined with Pseudomonas aeruginosa and the clinical condition of patients with cystic fibrosis. J. Cyst. Fibros. 12, 497–503. doi: 10.1016/j.jcf.2012.12.003
Ji, G., Beavis, R., and Novick, R. P. (1997). Bacterial interference caused by autoinducing peptide variants. Science 276, 2027–2030. doi: 10.1126/science.276.5321.2027
Josse, J., Laurent, F., and Diot, A. (2017). Staphylococcal adhesion and host cell invasion: fibronectin-binding and other mechanisms. Front. Microbiol. 8:1–8. doi: 10.3389/fmicb.2017.02433
Junge, S., Görlich, D., den Reijer, M., Wiedemann, B., Tümmler, B., Ellemunter, H., et al. (2016). Factors associated with worse lung function in cystic fibrosis patients with persistent Staphylococcus aureus. PLoS One 11:e0166220. doi: 10.1371/journal.pone.0166220
Kahl, B. C., Becker, K., and Löffler, B. (2016). Clinical significance and pathogenesis of staphylococcal small colony variants in persistent infections. Clin. Microbiol. Rev. 29, 401–427. doi: 10.1128/CMR.00069-15
Kim, T.-K., Bheda-Malge, A., Lin, Y., Sreekrishna, K., Adams, R., Robinson, M. K., et al. (2015). A systems approach to understanding human rhinovirus and influenza virus infection. Virology 486, 146–157. doi: 10.1016/J.VIROL.2015.08.014
Kreda, S. M., Davis, C. W., and Rose, M. C. (2012). CFTR, mucins, and mucus obstruction in cystic fibrosis. Cold Spring Harb. Perspect. Med. 2:a009589. doi: 10.1101/cshperspect.a009589
Liberati, N. T., Urbach, J. M., Miyata, S., Lee, D. G., Drenkard, E., Wu, G., et al. (2006). An ordered, nonredundant library of Pseudomonas aeruginosa strain PA14 transposon insertion mutants. Proc. Natl. Acad. Sci. U.S.A. 103, 2833–2838. doi: 10.1073/pnas.0511100103
Lightbown, J. W., and Jackson, F. L. (1956). Inhibition of cytochrome systems of heart muscle and certain bacteria by the antagonists of dihydrostreptomycin: 2-alkyl-4-hydroxyquinoline N-oxides. Biochem. J. 63, 130–137. doi: 10.1042/bj0630130
Limoli, D. H., Whitfield, G. B., Kitao, T., Ivey, M. L., Davis, M. R., Grahl, N., et al. (2017). Pseudomonas aeruginosa alginate overproduction promotes coexistence with Staphylococcus aureus in a model of cystic fibrosis respiratory infection. MBio 8:e00186-17. doi: 10.1128/mBio.00186-117
Limoli, D. H., Yang, J., Khansaheb, M. K., Helfman, B., Peng, L., Stecenko, A. A., et al. (2016). Staphylococcus aureus and Pseudomonas aeruginosa co-infection is associated with cystic fibrosis-related diabetes and poor clinical outcomes. Eur. J. Clin. Microbiol. Infect. Dis. 35, 947–953. doi: 10.1007/s10096-016-2621-2620
Lin, C. K., and Kazmierczak, B. I. (2017). Inflammation: a double-edged sword in the response to Pseudomonas aeruginosa infection. J. Innate Immun. 9, 250–261. doi: 10.1159/000455857
Lyczak, J. B., Cannon, C. L., and Pier, G. B. (2002). Lung infections associated with cystic fibrosis. Clin. Microbiol. Rev. 15, 194–222. doi: 10.1128/CMR.15.2.194-222.2002
Lyon, G. J., Mayville, P., Muir, T. W., and Novick, R. P. (2000). Rational design of a global inhibitor of the virulence response in Staphylococcus aureus, based in part on localization of the site of inhibition to the receptor-histidine kinase, AgrC. Proc. Natl. Acad. Sci. U S A. 97, 13330–13335. doi: 10.1073/pnas.97.24.13330
Machan, Z. A., Taylor, G. W., Pitt, T. L., Cole, P. J., and Wilson, R. (1992). 2-Heptyl-4-hydroxyquinoline N-oxide, an antistaphylococcal agent produced by Pseudomonas aeruginosa. J. Antimicrob. Chemother. 30, 615–623. doi: 10.1093/jac/30.5.615
Mitchell, G., Fugère, A., Pépin Gaudreau, K., Brouillette, E., Frost, E. H., Cantin, A. M., et al. (2013). SigB Is a dominant regulator of virulence in staphylococcus aureus small-colony variants. PLoS One 8:1–14. doi: 10.1371/journal.pone.0065018
Mitchell, G., Grondin, G., Bilodeau, G., Cantin, A. M., and Malouin, F. (2011). Infection of polarized airway epithelial cells by normal and small-colony variant strains of Staphylococcus aureus is increased in cells with abnormal cystic fibrosis transmembrane conductance regulator function and is influenced by NF-κB. Infect. Immun. 79, 3541–3551. doi: 10.1128/IAI.00078-11
Mitchell, G., Lafrance, M., Boulanger, S., Séguin, D. L., Guay, I., Gattuso, M., et al. (2012). Tomatidine acts in synergy with aminoglycoside antibiotics against multiresistant Staphylococcus aureus and prevents virulence gene expression. J. Antimicrob. Chemother. 67, 559–568. doi: 10.1093/jac/dkr510
Mitchell, G., Lamontagne, C. A., Brouillette, E., Grondin, G., Talbot, B. G., Grandbois, M., et al. (2008). Staphylococcus aureus SigB activity promotes a strong fibronectin-bacterium interaction which may sustain host tissue colonization by small-colony variants isolated from cystic fibrosis patients. Mol. Microbiol. 70, 1540–1555. doi: 10.1111/j.1365-2958.2008.06511.x
Mitchell, G., Séguin, D. L., Asselin, A.-E., Déziel, E., Cantin, A. M., Frost, E. H., et al. (2010). Staphylococcus aureus sigma B-dependent emergence of small-colony variants and biofilm production following exposure to Pseudomonas aeruginosa 4-hydroxy-2-heptylquinoline-N-oxide. BMC Microbiol. 10:33. doi: 10.1186/1471-2180-10-33
Moisan, H., Brouillette, E., Jacob, C. L., Langlois-Bégin, P., Michaud, S., and Malouin, F. (2006). Transcription of virulence factors in Staphylococcus aureus small-colony variants isolated from cystic fibrosis patients is influenced by SigB. J. Bacteriol. 188, 64–76. doi: 10.1128/JB.188.1.64-76.2006
Novick, R. P., and Geisinger, E. (2008). Quorum Sensing in Staphylococci. Annu. Rev. Genet. 42, 541–564. doi: 10.1146/annurev.genet.42.110807.091640
O’Neill, A. J. (2010). Staphylococcus aureus SH1000 and 8325-4: comparative genome sequences of key laboratory strains in staphylococcal research. Lett. Appl. Microbiol. 51, 358–361. doi: 10.1111/j.1472-765X.2010.02885.x
Palmer, K. L., Mashburn, L. M., Singh, P. K., and Whiteley, M. (2005). Cystic fibrosis sputum supports growth and cues key aspects of Pseudomonas aeruginosa physiology. J. Bacteriol. 187, 5267–5277. doi: 10.1128/JB.187.15.5267-5277.2005
Passariello, C., Schippa, S., Conti, C., Russo, P., Poggiali, F., Garaci, E., et al. (2006). Rhinoviruses promote internalisation of Staphylococcus aureus into non-fully permissive cultured pneumocytes. Microbes Infect. 8, 758–766. doi: 10.1016/j.micinf.2005.09.013
Proctor, R. A., von Eiff, C., Kahl, B. C., Becker, K., McNamara, P., Herrmann, M., et al. (2006). Small colony variants: a pathogenic form of bacteria that facilitates persistent and recurrent infections. Nat. Rev. Microbiol. 4, 295–305. doi: 10.1038/nrmicro1384
Rahme, L. G., Stevens, E. J., Wolfort, S. F., Shao, J., Tompkins, R. G., and Ausubel, F. M. (1995). Common virulence factors for bacterial pathogenicity in plants and animals. Science 268, 1899–1902. doi: 10.1126/science.7604262
Ratjen, F. A. (2009). Cystic fibrosis: pathogenesis and future treatment strategies. Respir. Care 54, 595–605. doi: 10.4187/aarc0427
Roman, J., Ritzenthaler, J. D., Boles, B., Lois, M., and Roser-Page, S. (2004). Lipopolysaccharide induces expression of fibronectin α 5 β 1 -integrin receptors in human monocytic cells in a protein kinase C-dependent fashion. Am. J. Physiol. Cell. Mol. Physiol. 287, L239–L249. doi: 10.1152/ajplung.00244.2003
Sadikot, R. T., Blackwell, T. S., Christman, J. W., and Prince, A. S. (2005). Pathogen-host interactions in Pseudomonas aeruginosa pneumonia. Am. J. Respir. Crit. Care Med. 171, 1209–1223. doi: 10.1164/rccm.200408-1044SO
Sampaio, A. L. F., Zahn, G., Leoni, G., Vossmeyer, D., Christner, C., Marshall, J. F., et al. (2010). Inflammation-dependent α5β1 (very late antigen-5) expression on leukocytes reveals a functional role for this integrin in acute peritonitis. J. Leukoc. Biol. 87, 877–884. doi: 10.1189/jlb.1009670
Sinha, B., François, P. P., Nüsse, O., Foti, M., Hartford, O. M., Vaudaux, P., et al. (1999). Fibronectin-binding protein acts as Staphylococcus aureus invasin via fibronectin bridging to integrin alpha5beta1. Cell. Microbiol. 1, 101–117. doi: 10.1046/j.1462-5822.1999.00011.x
Sinha, B., and Fraunholz, M. (2010). Staphylococcus aureus host cell invasion and post-invasion events. Int. J. Med. Microbiol. 300, 170–175. doi: 10.1016/J.IJMM.2009.08.019
Springer, T. A. (1990). Adhesion receptors of the immune system. Nature 346, 425–434. doi: 10.1038/346425a0
Sriramulu, D. D., Lünsdorf, H., Lam, J. S., and Römling, U. (2005). Microcolony formation: a novel biofilm model of Pseudomonas aeruginosa for the cystic fibrosis lung. J. Med. Microbiol. 54, 667–676. doi: 10.1099/jmm.0.45969-0
Weidenmaier, C., Peschel, A., Xiong, Y.-Q., Kristian, S. A., Dietz, K., Yeaman, M. R., et al. (2005). Lack of wall teichoic acids in Staphylococcus aureus leads to reduced interactions with endothelial cells and to attenuated virulence in a rabbit model of endocarditis. J. Infect. Dis. 191, 1771–1777. doi: 10.1086/429692
Westermann, A. J., Barquist, L., and Vogel, J. (2017). Resolving host–pathogen interactions by dual RNA-seq. PLoS Pathog. 13:e1006033. doi: 10.1371/journal.ppat.1006033
Wieland, C. W., Siegmund, B., Senaldi, G., Vasil, M. L., Dinarello, C. A., and Fantuzzi, G. (2002). Pulmonary inflammation induced by Pseudomonas aeruginosa lipopolysaccharide, phospholipase C, and exotoxin A: role of interferon regulatory Factor 1. Infect. Immun. 70, 1352–1358. doi: 10.1128/IAI.70.3.1352
Williams, P., and Cámara, M. (2009). Quorum sensing and environmental adaptation in Pseudomonas aeruginosa: a tale of regulatory networks and multifunctional signal molecules. Curr. Opin. Microbiol. 12, 182–191. doi: 10.1016/j.mib.2009.01.005
Wolter, D. J., Emerson, J. C., McNamara, S., Buccat, A. M., Qin, X., Cochrane, E., et al. (2013). Staphylococcus aureus small-colony variants are independently associated with worse lung disease in children with cystic fibrosis. Clin. Infect. Dis. 57, 384–391. doi: 10.1093/cid/cit270
Keywords: microbial interactions, oral microbiology, Pseudomonas, Staphylococcus, quorum sensing, sociomicrobiology, microbiome, genomics
Citation: Millette G, Langlois J-P, Brouillette E, Frost EH, Cantin AM and Malouin F (2019) Despite Antagonism in vitro, Pseudomonas aeruginosa Enhances Staphylococcus aureus Colonization in a Murine Lung Infection Model. Front. Microbiol. 10:2880. doi: 10.3389/fmicb.2019.02880
Received: 17 July 2019; Accepted: 29 November 2019;
Published: 13 December 2019.
Edited by:
Giovanni Di Bonaventura, Università degli Studi “G. d’Annunzio” Chieti–Pescara, ItalyReviewed by:
Dinesh Sriramulu, Independent Researcher, Chennai, IndiaAlessandra Bragonzi, San Raffaele Scientific Institute (IRCCS), Italy
Copyright © 2019 Millette, Langlois, Brouillette, Frost, Cantin and Malouin. This is an open-access article distributed under the terms of the Creative Commons Attribution License (CC BY). The use, distribution or reproduction in other forums is permitted, provided the original author(s) and the copyright owner(s) are credited and that the original publication in this journal is cited, in accordance with accepted academic practice. No use, distribution or reproduction is permitted which does not comply with these terms.
*Correspondence: François Malouin, RnJhbmNvaXMuTWFsb3VpbkBVU2hlcmJyb29rZS5jYQ==