- 1Advanced Light and Electron Microscopy (ZBS-4), Robert Koch Institute, Berlin, Germany
- 2Institute of Microbiology and Epizootics, Centre for Infection Medicine, Freie Universität Berlin, Berlin, Germany
- 3Microbial Genomics (NG1), Robert Koch Institute, Berlin, Germany
- 4Nosocomial Pathogens and Antibiotic Resistances, Robert Koch Institute, Wernigerode, Germany
- 5Institute for Veterinary Epidemiology and Biostatistics, Freie Universität Berlin, Berlin, Germany
- 6Robert Koch Institute, Berlin, Germany
Strategies to reduce economic losses associated with post-weaning diarrhea in pig farming include high-level dietary zinc oxide supplementation. However, excessive usage of zinc oxide in the pig production sector was found to be associated with accumulation of multidrug resistant bacteria in these animals, presenting an environmental burden through contaminated manure. Here we report on zinc tolerance among a random selection of intestinal Escherichia coli comprising of different antibiotic resistance phenotypes and sampling sites isolated during a controlled feeding trial from 16 weaned piglets: In total, 179 isolates from “pigs fed with high zinc concentrations” (high zinc group, [HZG]: n = 99) and a corresponding “control group” ([CG]: n = 80) were investigated with regard to zinc tolerance, antimicrobial- and biocide susceptibilities by determining minimum inhibitory concentrations (MICs). In addition, in silico whole genome screening (WGSc) for antibiotic resistance genes (ARGs) as well as biocide- and heavy metal tolerance genes was performed using an in-house BLAST-based pipeline. Overall, porcine E. coli isolates showed three different ZnCl2 MICs: 128 μg/ml (HZG, 2%; CG, 6%), 256 μg/ml (HZG, 64%; CG, 91%) and 512 μg/ml ZnCl2 (HZG, 34%, CG, 3%), a unimodal distribution most likely reflecting natural differences in zinc tolerance associated with different genetic lineages. However, a selective impact of the zinc-rich supplemented diet seems to be reasonable, since the linear mixed regression model revealed a statistically significant association between “higher” ZnCl2 MICs and isolates representing the HZG as well as “lower ZnCl2 MICs” with isolates of the CG (p = 0.005). None of the zinc chloride MICs was associated with a particular antibiotic-, heavy metal- or biocide- tolerance/resistance phenotype. Isolates expressing the 512 μg/ml MIC were either positive for ARGs conferring resistance to aminoglycosides, tetracycline and sulfamethoxazole-trimethoprim, or harbored no ARGs at all. Moreover, WGSc revealed a ubiquitous presence of zinc homeostasis and – detoxification genes, including zitB, zntA, and pit. In conclusion, we provide evidence that zinc-rich supplementation of pig feed selects for more zinc tolerant E. coli, including isolates harboring ARGs and biocide- and heavy metal tolerance genes – a putative selective advantage considering substances and antibiotics currently used in industrial pork production systems.
Introduction
Enterotoxigenic Escherichia coli (ETEC) are commonly associated with post-weaning diarrhea (PWD) in piglets, a disease causing serious losses in the pig industry worldwide (Fairbrother et al., 2005; Rhouma et al., 2017a). Currently, different strategies are utilized to reduce ETEC-associated economic costs in pig farming, including oral colistin sulfate treatment in some regions of the world (Rhouma et al., 2017b), vaccination (Moon and Bunn, 1993; Blázquez et al., 2018) and probiotics (Li et al., 2018; Yan et al., 2018). In addition, high-level dietary zinc oxide supplementation is used against PWD in the pig production sector (Fairbrother et al., 2005; Vahjen et al., 2011; Bednorz et al., 2013; Starke et al., 2014; Pieper et al., 2015; Kloubert et al., 2018).
However, the effects of zinc-rich diets on porcine intestinal bacterial populations, especially E. coli, are not fully understood yet. Zinc is the second most abundant transition metal in most phyla and generally considered as essential for life. Together with copper, it is an important trace element required for hormone function, reproduction, vitamin synthesis, enzyme formation and it promotes a strong immune system function (Yu et al., 2017). Both metals are usually added to animal feed in amounts necessary for physiological body function (Yazdankhah et al., 2014; Yu et al., 2017).
As a divalent cation (Zn2+), zinc plays an important role as a catalytic and structural cofactor in virtually all aspects of cell metabolism (Vallee and Auld, 1990). Keeping a balanced intracellular zinc homeostasis is a prerequisite for mammals and most bacterial species (Nies and Grass, 2009). Therefore, zinc quantities within cells are highly regulated, as zinc deprivation hinders bacterial growth, while an excess of zinc could be toxic (Gielda and DiRita, 2012). Factors reported to increase zinc tolerance levels in E. coli described so far include the cation diffusion facilitator (CDF) ZitB, the P1b-type ATPase ZntA and the low-affinity inorganic phosphate transporter Pit (Beard et al., 2000; Grass et al., 2005; Deus et al., 2017; Hoegler and Hecht, 2018).
So far, an increased tolerance toward (trace) metals, including zinc, is clearly linked to genes conferring antibiotic resistance in different bacterial species (Cavaco et al., 2011; Agga et al., 2014; Medardus et al., 2014; Becerra-Castro et al., 2015; Song et al., 2017; van Alen et al., 2018), possibly indicating a worrisome co-selective effect of zinc oxide mass utilization (Seiler and Berendonk, 2012; Bednorz et al., 2013; Yazdankhah et al., 2014; Ciesinski et al., 2018). Consequently, the current anthropogenic contamination of the environment with heavy metals is regarded as a serious problem (Seiler and Berendonk, 2012).
In E. coli, an extensive, finely tuned network of efflux pumps, ligands and transcription factors is involved in intracellular osmoadaption and heavy metal detoxification, also warranting maintenance of cellular zinc homeostasis (Hantke, 2005; Nies and Grass, 2009; Porcheron et al., 2013; Watly et al., 2016). Recent studies revealed that zinc tolerance levels differ not only between bacterial species but also within particular species, including E. coli of human and avian origin (Deus et al., 2017; Stocks et al., 2019).
The aim of this work is to study the effects of zinc-rich diets on a representatively selected collection of intestinal E. coli obtained from post-weaning piglets, considering a putative association of a nutritive zinc oxide excess and (i) phenotypic zinc tolerance levels, (ii) antibiotic- and biocide susceptibility profiles, and (iii) genes involved in antimicrobial resistance, zinc (heavy metal)- and biocide tolerance.
Materials and Methods
Sample Size and Isolate Selection
The representative set of E. coli isolates investigated here was selected based on a previous feeding trial (Ciesinski et al., 2018) carried out in accordance with the principles of the Basel Declaration following the institutional and national guidelines for the care and use of animals. The protocol was approved by the local state office of occupational health and technical safety “Landesamt für Gesundheit und Soziales, Berlin” (LaGeSo Reg. Nr. 0296/13) as described before (Ciesinski et al., 2018).
Briefly, 32 landrace piglets weaned at day 25 ± 1 were separated in two groups for 4 weeks: the first group of piglets, denoted here as the high-zinc group (HZG) was fed with a diet supplemented with a comparatively high amount of zinc oxide (2,103 mg zinc/kg diet), while the second group served as control. This control group (CG) received a common piglet diet containing a physiologic concentration of zinc oxide (72 mg zinc/kg diet) to avoid trace metal malnutrition (Ciesinski et al., 2018). The trial started with 32 piglets, which were sacrificed mid-trial (38 ± 2 days, n = 16) and at the end (52 ± 2 days, n = 16).
Here we focus on E. coli obtained from samples of pigs sacrificed at the end (n = 16; 52 ± 2 days) of the feeding trial only. Altogether, 817 E. coli collected from the feces, digesta- and mucosa samples obtained from these final 16 pigs were stored in glycerol stocks at −80° (Ciesinski et al., 2018). Using meta data such as sampling site, feeding group and evaluation of growth on plates containing different antibiotics which were available for all the 817 E. coli obtained from this initial approach (Ciesinski et al., 2018) we selected a stratified random sample comprising 179 isolates (Table 1 and Supplementary Table S1).
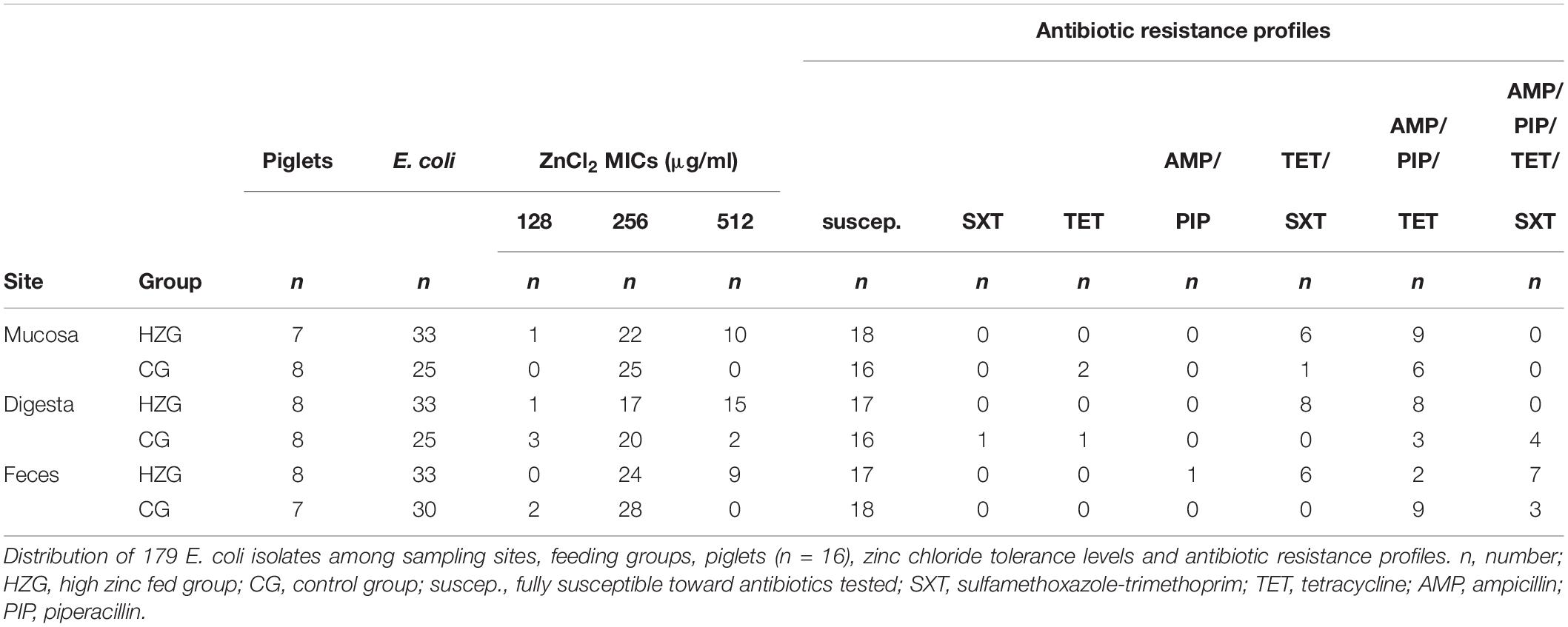
Table 1. Distribution of E. coli among sampling sites, feeding groups, pigs, ZnCl2 MICs and antibiotic resistance profiles.
Phenotype Characterization of Porcine E. coli
A broth microdilution assay was carried out for biocides and heavy metal salts using microtiter-plates (Merlin, Bornheim-Hersel, Germany) as described before (Deus et al., 2017) including alkyl diaminoethyl glycin hydrochloride [ADH], benzethonium chloride [BEN], benzalkonium chloride [BKC], guanidine chlorhexidine [CHX], acridine compound acriflavine [ACR], copper sulfate [COP], silver nitrate [SIL] and zinc chloride [ZKC]. E. coli strains ATCC25922 and ATCC10536 were used for internal quality control. In addition, E. coli strain RKI6122 was used as a reference for growth in the presence of 1024 μg ZnCl2/ml (Deus et al., 2017).
Antimicrobial susceptibility testing (AST) using the VITEK® 2 system (BioMérieux, Germany; AST card GN38) was performed including amikacin, amoxicillin/clavulanic acid, ampicillin, cephalexin, chloramphenicol, enrofloxacin, gentamicin, marbofloxacin, piperacillin, tetracycline, tobramycin and trimethoprim/sulfamethoxazole according to the standards given by the CLSI VET01-A4 and M100-S21) (Clinical and Laboratory Standards Institute, 2012, 2013).
Molecular Characterization of E. coli
Hundred and seventy nine E. coli were sequenced using Illumina MiSeq® 300 bp paired-end whole genome sequencing (WGS) with an obtained coverage of >90X. Plasmid DNA of E. coli RKI3099, an isolate representing a frequently occurring genomic background associated with increased zinc tolerance together with antimicrobial resistance (Supplementary Table S1), was isolated using Qiagen Plasmid Mini Kit according to manufacturer’s instructions. The purified plasmid DNA was sequenced using the Pacific Biosciences RS II platform with the P6C4 chemistry in a single flowcell. Pacific Biosciences sequencing was completed by generating a sequencing library from 5 to 20 kb using standard methods. PacBio raw data and the Illumina short reads were hybrid assembled using unicycler v4.4 (Wick et al., 2017). Adapter-trimmed reads were used for de novo assembly into contiguous sequences (contigs) and subsequently into scaffolds using SPAdes v3.11. All draft genomes were annotated using Prokka (Seemann, 2014).
In previous studies, factors have been described as being capable to confer elevated zinc tolerance levels in E. coli (Grass et al., 2005; Deus et al., 2017; Vidhyaparkavi et al., 2017; Stocks et al., 2019). Consequently, we analyzed the presence or absence of a broad set of genes involved in zinc homeostasis (n = 35) including zinc-binding metalloenzymes (n = 69) (Supplementary Table S2).
Since co-selection of antibiotic- and metal resistance is an issue of utmost importance and metal resistance genes are often co-located on mobile genetic elements (MGEs) alongside antibiotic resistance genes (ARGs) (Baker-Austin et al., 2006; Fard et al., 2011; Holzel et al., 2012; Fang et al., 2016; Song et al., 2017; Argudín et al., 2019), we further investigated the occurrence of genes known to be associated with either antibiotic- or metal resistance on mobile genetic elements among our isolate collection (Supplementary Table S1).
Further investigation included screening of 203 genes described by Pal et al. (2013), particularly known to be associated with increased tolerance or even resistance toward different biocides, for example acridine compound acriflavine [ACR], benzalkonium chloride [BKC] and benzethonium chloride [BEN]. The screening procedure included among others qacE, its variant qacE(Δ1), qacL, sugE, ygiW, ymgB (Supplementary Table S3) and further operons known to be involved in heavy metal detoxification (arsABCD, cusABCF, merRT, pcoABCDE, pcoRS, silABCEFP, silRS, terBCDWZ, ygiW) alongside their regulatory genes (Supplementary Table S3).
Consequently, in silico whole-genome screening for all these genes associated with antibiotic resistance (ARGs) [n = 2570 included variants of ARGs], biocide resistance or heavy metal tolerance was performed using an in-house BLAST-pipeline with the general identity threshold 95% ID and 90% minimum length based on ResFinder 3.1 (Zankari et al., 2012), CARD (The Comprehensive Antibiotic Resistance Database, Jia et al., 2017) and BacMet (Antibacterial Biocide & Metal Resistance Genes Database; Pal et al., 2013).
Whole genome screening data were used for further genotype characterization including determination of multilocus sequence type (ST) using MLSTFinder 2.0 (Larsen et al., 2012), serotype prediction (SerotypeFinder 2.0, Joensen et al., 2015) and occurrence of plasmid incompatibility groups with PlasmidFinder 2.0 with a threshold of 95% ID (Carattoli et al., 2014). A detailed overview on all isolates and characteristics is provided in Supplementary Table S1.
Whole genome sequences for all 179 E. coli are deposited into NCBI-Genbank and accession numbers are provided in Supplementary Table S4.
Statistical Analysis
Data were analyzed using SPSS software version 25.0 (IBM, New York, NY, United States). P-values < 0.05 were considered statistically significant.
A linear mixed-model regression approach was used to test whether feeding group and sampling sites (mucosa, digesta and feces) had an effect on E. coli ZnCl2 MICs, with the individual pig as a random factor. The logarithm (basis 2) of ZnCl2 MIC values was the dependent variable in all analyses. All two-way interactions were included in the models. Non-significant interactions were removed one by one. Variance components were used to determine the proportion of variance that accounted for differences between individual animals. Model diagnostics included visual inspection of residuals for normality and homoscedasticity.
Further, mixed linear regression models were developed to investigate the influence of
• occurrence of resistance toward one or more antibiotic classes (yes or no),
• the MIC levels of acridine compound acriflavine (logarithmic to the basis 2),
• silver nitrate (logarithmic to the basis 2), or
• chlorhexidine (logarithmic to the basis 2), respectively,
on lg2 ZnCl2 MIC values including sample origin and feeding group as fixed factors and the individual pig as a random factor.
Results
Zinc Tolerance Levels of Porcine Intestinal E. coli
Here we present results based on a stratified random sample comprising 179 E. coli (Supplementary Table S1) representing different AST phenotypes, three different sampling sites (digesta, mucosa, feces) and two feeding groups (HZG and CG). For this collection three different levels of tolerance toward zinc chloride (ZnCl2) were detected (Table 1). The lowest level of tolerance toward ZnCl2 (128 μg/ml) was recorded for 2% of the HZG and 6% of the CG isolates, respectively. Sixty four percent of the HZG and 91% of the CG isolates were associated with a ZnCl2 MIC of 256 μg/ml. Considering the maximum ZnCl2 MIC of 512 μg/ml exhibited by isolates in this study, a clear difference between isolates belonging to the distinct feeding groups was obvious: while 34% of the E. coli from samples of the HZG clustered here, only 3% of those obtained from the CG reached this particular tolerance level as well (Figure 1).
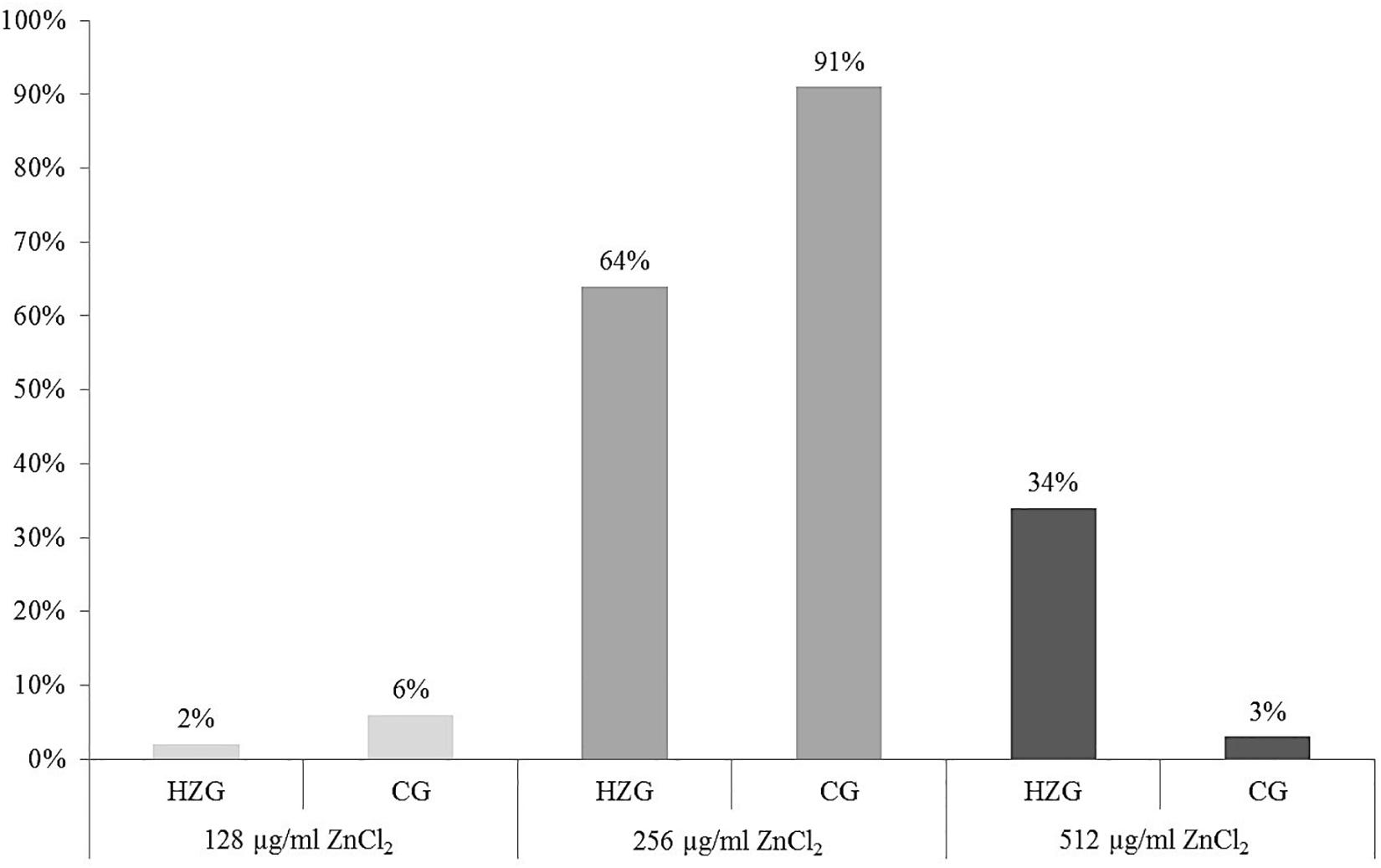
Figure 1. Distribution of zinc chloride MICs among 179 intestinal E. coli from piglets. Relative (%) distribution of three different ZnCl2 MICs among isolates from the high-zinc group (HZG, n = 99) and the control group (CG, n = 80).
Further analysis using a linear mixed regression model taking inter-individual host (n = 16 pigs) differences (inter-host variance: 16.7% of total variance) into account revealed a statistically significant association between ZnCl2 MICs and isolates representing the HZG as well as ZnCl2 MICs with isolates of the CG (p = 0.005) with a regression coefficient of −0.332 (Table 2). Considering E. coli from the three sampling sites (mucosa, digesta from colon ascendence and feces from the ampulla recti), ZnCl2 MICs lacked significant differences (p = 0.636).
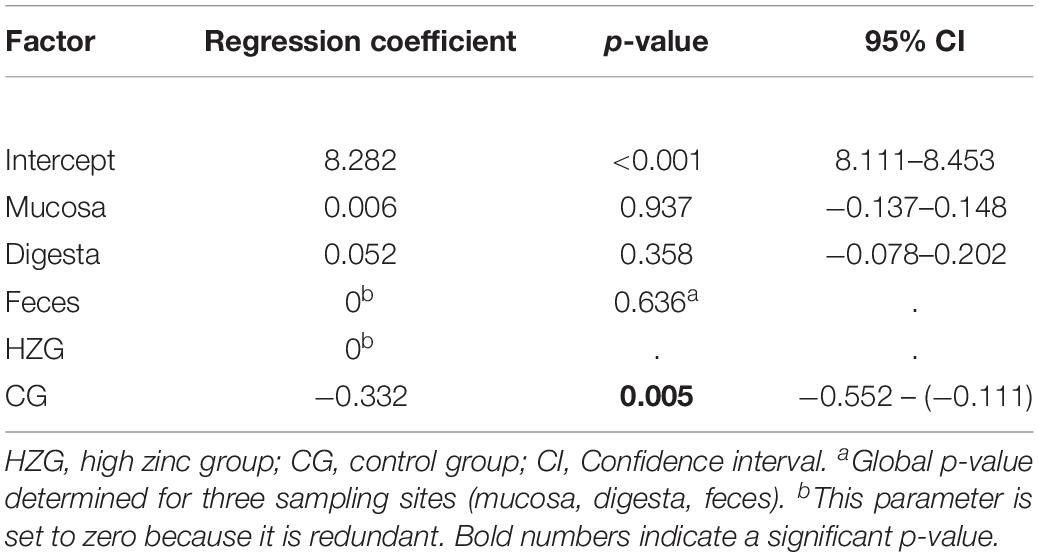
Table 2. Results of mixed linear regression model examining the influence of feeding group and sample site on lg2 ZnCl2 (dependent factor) for 179 E. coli with pig as random variable.
Zinc Tolerance, Antibiotic and Biocide Susceptibility Profiles
To answer the question whether a particular level of zinc tolerance is associated with a certain antibiotic resistance phenotype, all E. coli were grouped according to their individual resistance profile and zinc tolerance level (Figure 2). Non-susceptibilities detected for the 179 E. coli included those to sulfamethoxazole-trimethoprim, tetracycline, ampicillin and piperacillin only (Table 1 and Figure 2). Isolates expressing the “highest” ZnCl2 MIC (512 μg/ml) showed either a susceptible phenotype toward the panel of antibiotics tested here or yielded non-susceptibility for tetracycline and sulfamethoxazole-trimethoprim. Overall, MICs of antibiotic-resistant (resistance toward one or more antibiotic classes) and susceptible isolates showed no significant difference using mixed linear regression (p = 0.119) (Table 3), but drug resistant isolates had slightly higher ZnCl2 MIC values than susceptible isolates (regression coefficient 0.105). Overall, zinc tolerance levels of E. coli investigated in this study lacked a particular association with the occurrence of any antibiotic resistance phenotype concerning the antimicrobial substances included.
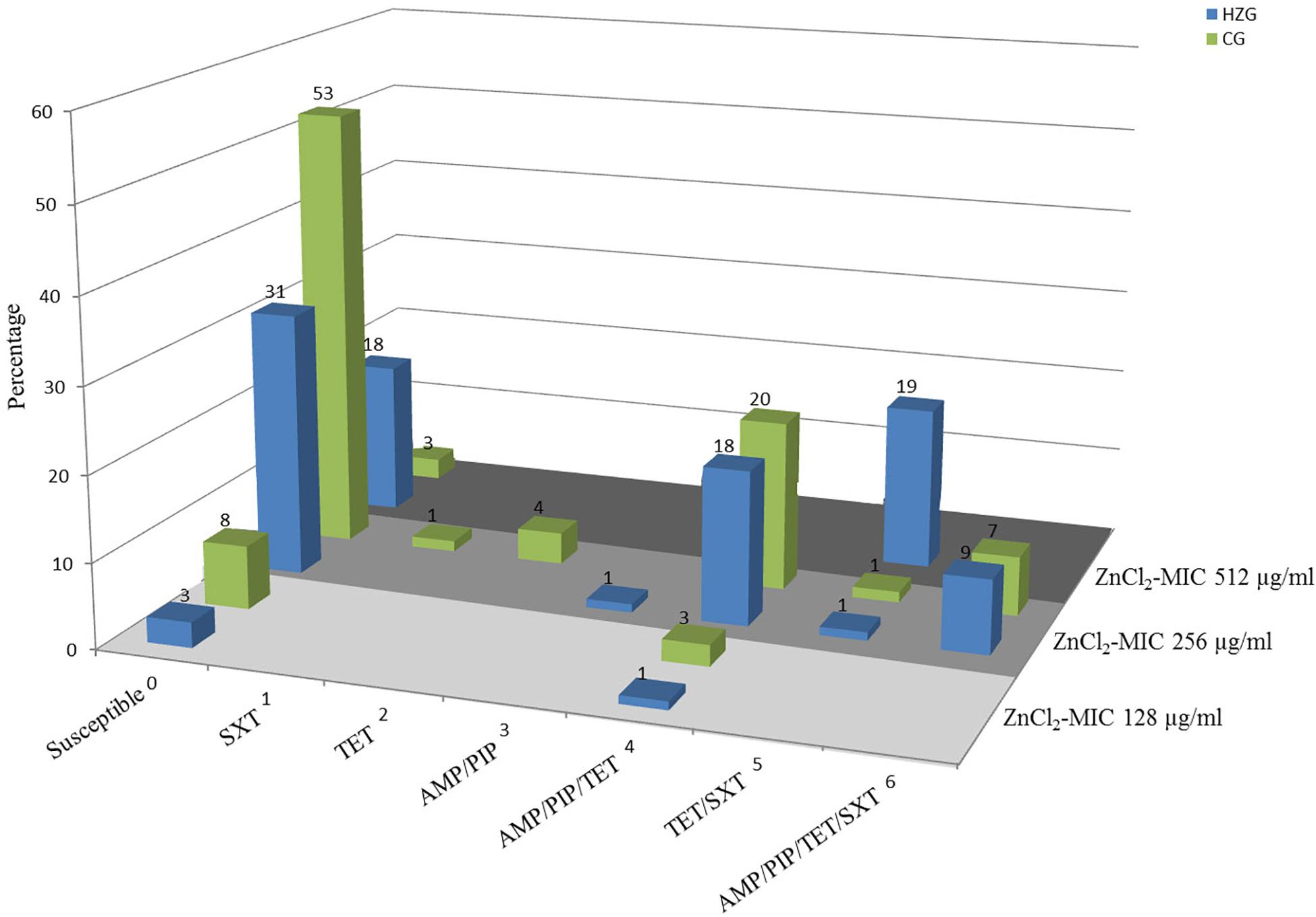
Figure 2. Antibiotic resistance pattern and zinc chloride MICs of porcine intestinal E. coli. Relative (%) distribution of resistance pattern compared with zinc chloride MICs (light gray = ZnCl2 MIC of 128 μg/ml; gray = ZnCl2 MIC of 256 μg/ml; dark gray = ZnCl2 MIC of 512 μg/ml) in high-zinc group (blue) [HZG] and control group (green) [CG]. SXT, sulfamethoxazole-trimethoprim; TET, tetracycline; AMP, ampicillin; PIP, piperacillin.
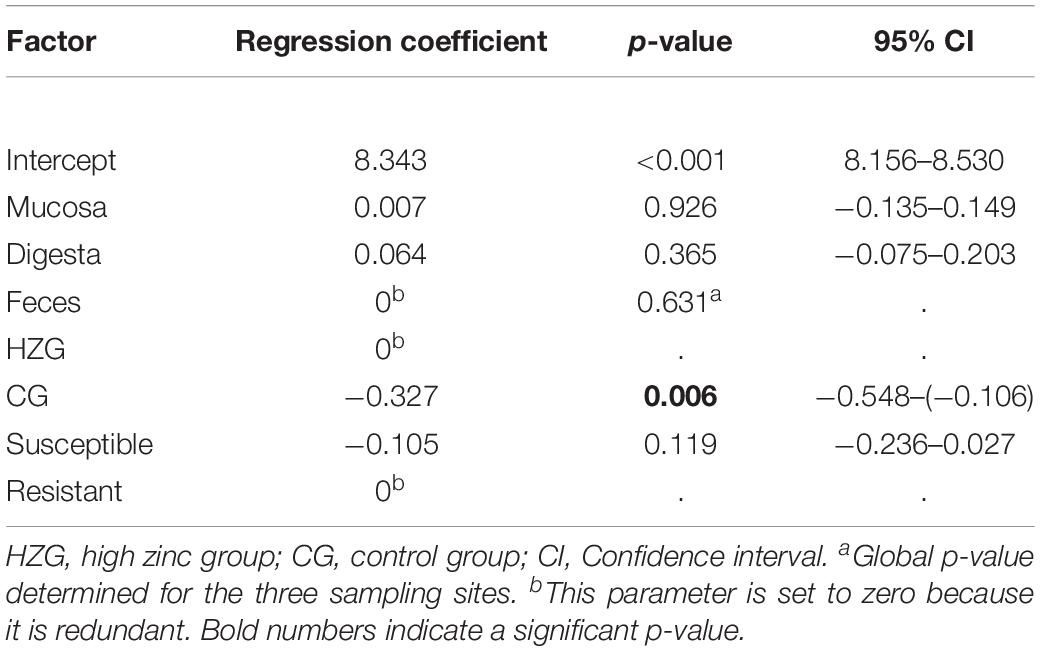
Table 3. Results of mixed linear regression model examining the influence of antimicrobial resistance, feeding group and sampling site on lg2 ZnCl2 MIC (dependent factor) for 179 E. coli with pig as random variable.
Minimum inhibitory concentrations detected for biocides and further inorganic metal compounds showed a unimodal value distribution (Table 4), possibly indicating the lack of a distinct non-wild type E. coli subpopulation for any of the substances tested here. The broadest distribution of MICs was recorded for acridine compound acriflavine, silver nitrate and chlorhexidine, comprising four dilution steps each (Table 4).
We have set up mixed linear regression models to investigate a putative association between ZnCl2 MICs and tolerance levels toward a specific biocide or inorganic metal. The model including lg2 acridine MICs as an influence factor beside the feeding group and the three sampling sites once again showed a significant association between ZnCl2 MICs and feeding group (p = 0.011), but not with either sampling site (p = 0.640) or lg2 acridine MICs (p = 0.746) (Supplementary Table 5A).
The silver nitrate model (dependent variable lg2 ZnCl2 MIC, independent variables lg2 silver nitrate MIC, feeding group and sampling site) also showed a significant association with the feeding group (p = 0.010), but not for lg2 silver nitrate (p = 0.979) nor the sampling site (p = 0.643, Supplementary Table 5B).
The model build to test the influence of chlorhexidine showed a significant interaction between the feeding group and the lg2 chlorhexidine MICs (p = 0.047). While the lg2 ZnCl2 MICs decreased with increasing lg2 chlorhexidine MICs in the HZG, they increased slightly in the CG with increasing lg2 chlorhexidine MICs. The parameters sampling site (p = 0.338) and feeding group (p = 0.137) did not have significant effects, but lg2 chlorhexidine MIC (p = 0.022) did (Supplementary Table 5C). 15.6% of the variance was due to variance between animals.
Genomic Background and Genes Involved in Zinc Tolerance
Whole genome screening data were used to assign all E. coli ST and to predict serotypes among the isolates representing the HZG (n = 99) and the CG (n = 80). As shown in Figure 2, these 179 isolates representing the two feeding groups were assigned to 15 STs each. Overall, isolates belonging to the sequence type complex (STC) 10 (ST10 and ST34) were most common among the representative isolates of both groups (HZG, 50.5%; CG, 40%).
Considering the corresponding predicted serotypes, E. coli O62:H30 (ST34) was common in the representative samples of both feeding groups (20% [HZG]/19% [CG]), followed by the ST10 O88:H12 (9% [HZG]/7% [CG]) (Figure 2). In addition, most E. coli representing both feeding groups (141 of 179) showed a ZnCl2 MIC of 256 μg/ml. These isolates were assigned to 17 STs and 27 serotypes. The isolates with ZnCl2 MIC 128 μg/ml belonged to three different STs (4 serotypes) and the ZnCl2 MIC of 512 μg/ml included isolates of seven STs and seven corresponding serotypes (Figure 3). Both groups of E. coli representing the two different feeding groups included predicted serotypes which were unique to it, for instance CG isolates belonging to O92:H2 and O157:H43 or O89:H38 and O182:H19 in the HZG (Figure 3).
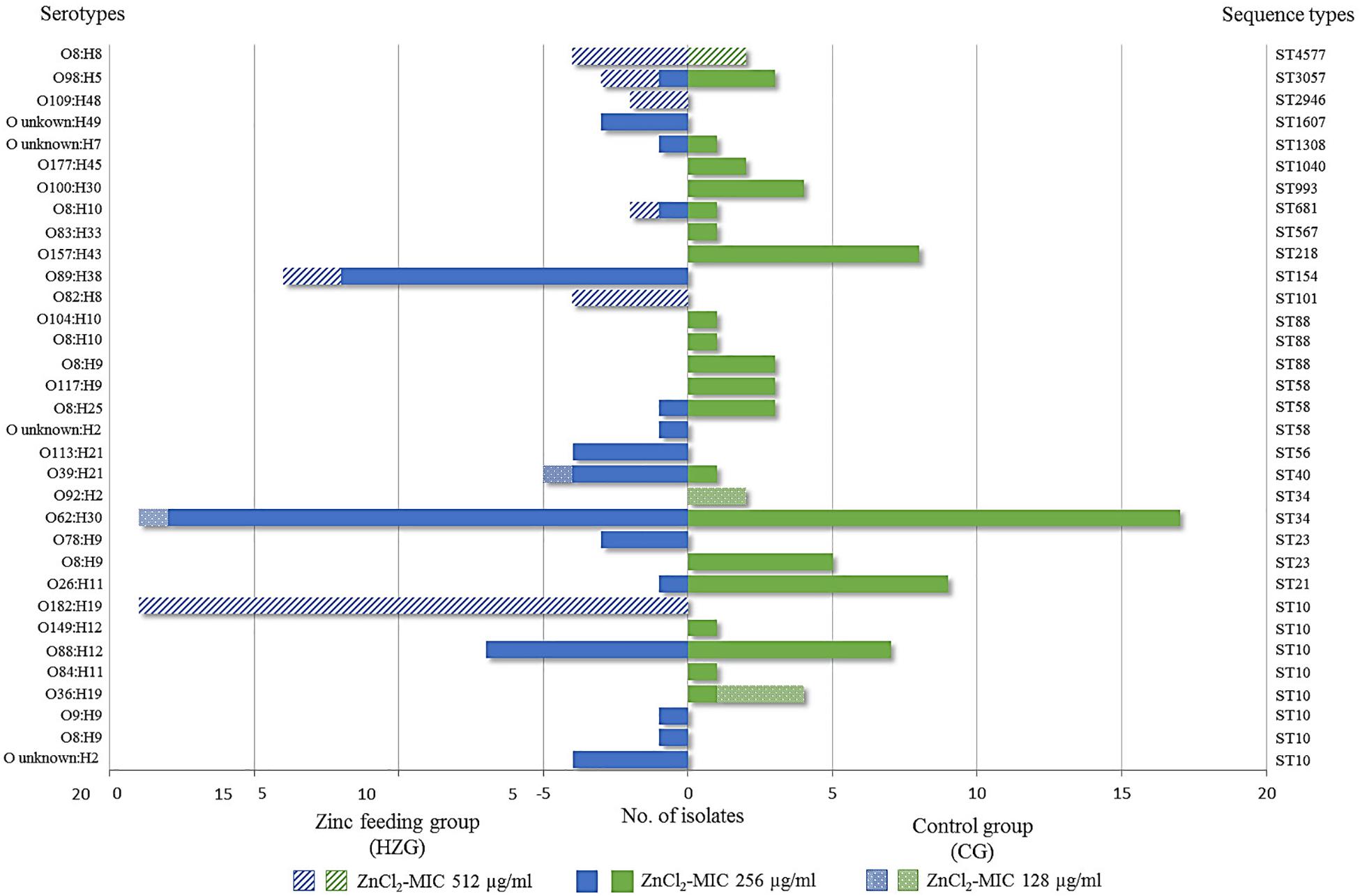
Figure 3. Sequence types and serotypes of 179 with E. coli showing three different zinc chloride MICs. Sequence- and (predicted) serotype distribution and frequencies of 179 E. coli isolates representing both feeding groups together with zinc chloride tolerance MICs. Most isolates showed the ZnCl2 MIC of 256 μg/ml, represented by 17 STs in both feeding groups. The broadest heterogeneity seems to be associated with sequence type complex (STC)10 isolates, which were obtained from both feeding groups (high-zinc group [HZG] and control group [CG]), including representatives for each of the three zinc chloride tolerance values.
In particular factors have been described as being capable to confer elevated zinc tolerance levels in E. coli (Grass et al., 2005; Deus et al., 2017; Vidhyaparkavi et al., 2017; Stocks et al., 2019). Consequently, we analyzed the presence or absence of a broad set of genes involved in zinc homeostasis (n = 35) (Table 5) including zinc-binding metalloenzymes (n = 69) (Supplementary Table S2). All 179 isolates harbored genes associated with zinc detoxification such as zitB, zntA and pit (Table 5). Also, factors involved in zinc uptake like ZupT (metal uptake protein, preference for zinc), the ABC transporter ZnuABC and major regulators such as Zur were identified in each of the genomes. Only the Rac-prophage zinc-binding chaperone protein YdaE (Blindauer et al., 2002) and the Zn(II)-responsive ribosomal proteins YkgM (Hensley et al., 2012) was not ubiquitously distributed among isolates of the three distinct ZnCl2 MICs (Table 5). In addition, almost all genomes were found positive for genes encoding zinc-binding metalloenzymes involved in a highly diverse net of cell functions (Supplementary Table S2). A detailed overview on gene presence or absence, query protein coverage and number of predicted protein variants for these 104 factors investigated here is provided in Table 5 and Supplementary Table S2. Accordingly, sheer presence or absence of particular factors or even a particular amino acid sequence variant does not explain the different levels of zinc tolerance among the analyzed E. coli population.
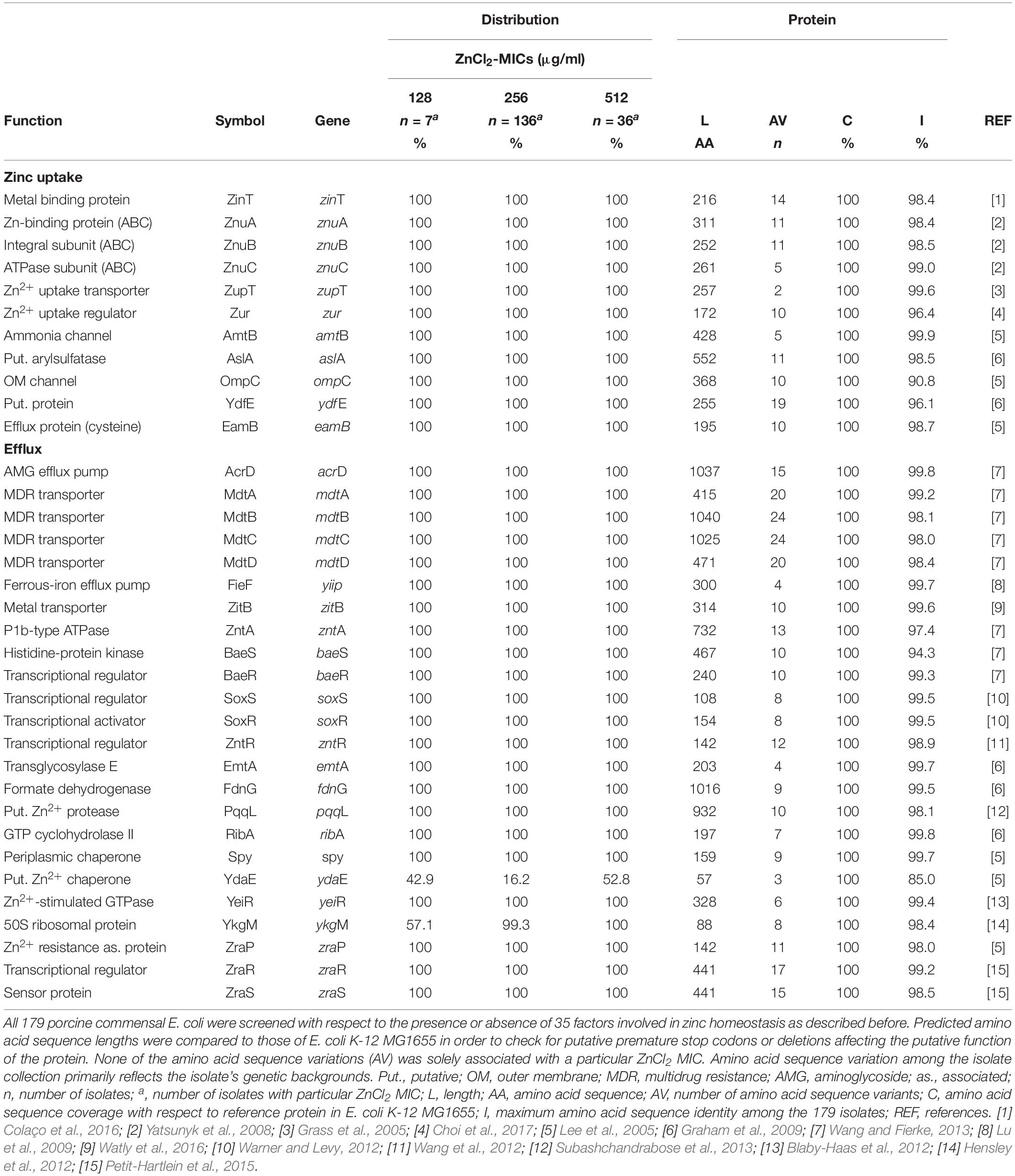
Table 5. Screening results of factors involved in zinc homeostasis for 179 porcine commensal E. coli.
Antibiotic Resistance – Biocide – And Heavy Metal Tolerance Genes
In total, 87/179 E. coli comprising isolates of both feeding groups and each zinc tolerance level lack ARGs, which is in strict concordance with their resistance phenotype (Figure 2 and Table 6). However, genes known to confer antibiotic resistance have been identified on MGEs within the isolate collection. While the ZnCl2 MIC 128 μg/ml is associated with the occurrence of 0–4 ARGs and three STs, the ZnCl2 MIC 256 μg/ml shows a range from 0 to7 ARGs and ZnCl2 MIC 512 μg/ml harbors either 0 or 5 ARGs (Supplementary Table S1).
Seven isolates expressing a ZnCl2 MIC of 128 μg/ml belonged to ST10 O36:H19 (n = 3), ST34 O62:H30 (n = 1), O92:H2 (n = 2), and ST40 O39:H21 (n = 1). ARGs conferring resistance to aminoglycosides, beta-lactams and tetracycline (aadA1, blaTEM–1b, strA, strB, and tetB) were solely associated with both serotypes belonging to ST34.
Sixty nine of 136 E. coli with the 256 μg/ml ZnCl2 MIC harbored a different ARG combinations, e.g., isolates belonging to ST56 O113:H21 (n = 4) were positive for aadA1 and sul1 and ST993 O100:H30 (n = 4) isolates harbored tetB. ST10 O88:H12 (n = 13) harbored aadA1, dfrA1, blaTEM–1b, strA, strB, sul3, and tetA.
Considering the 36 isolates with the 512 μg/ml ZnCl2 MIC, ARGs (aadA1, dfrA1, sul1, sul3 and tetA) conferring resistance to aminoglycosides, trimethoprim, sulfonamides and tetracycline were exclusively associated with 19 isolates belonging to ST10 O182:H19. The remaining 17 isolates completely lack ARGs and belong to six different STs (101, 154, 681, 2946, 3057, 4577) (Supplementary Table S1). WGSc indicated the presence of more than one plasmid harboring the 5 ARGs and further genes conferring tolerance toward biocides and heavy metals (Figure 4) among the ST10 O182:H19 isolates. Subsequently, PacBio sequencing was employed to further investigate the composition of these structures extracted from a representative isolate (RKI3099).
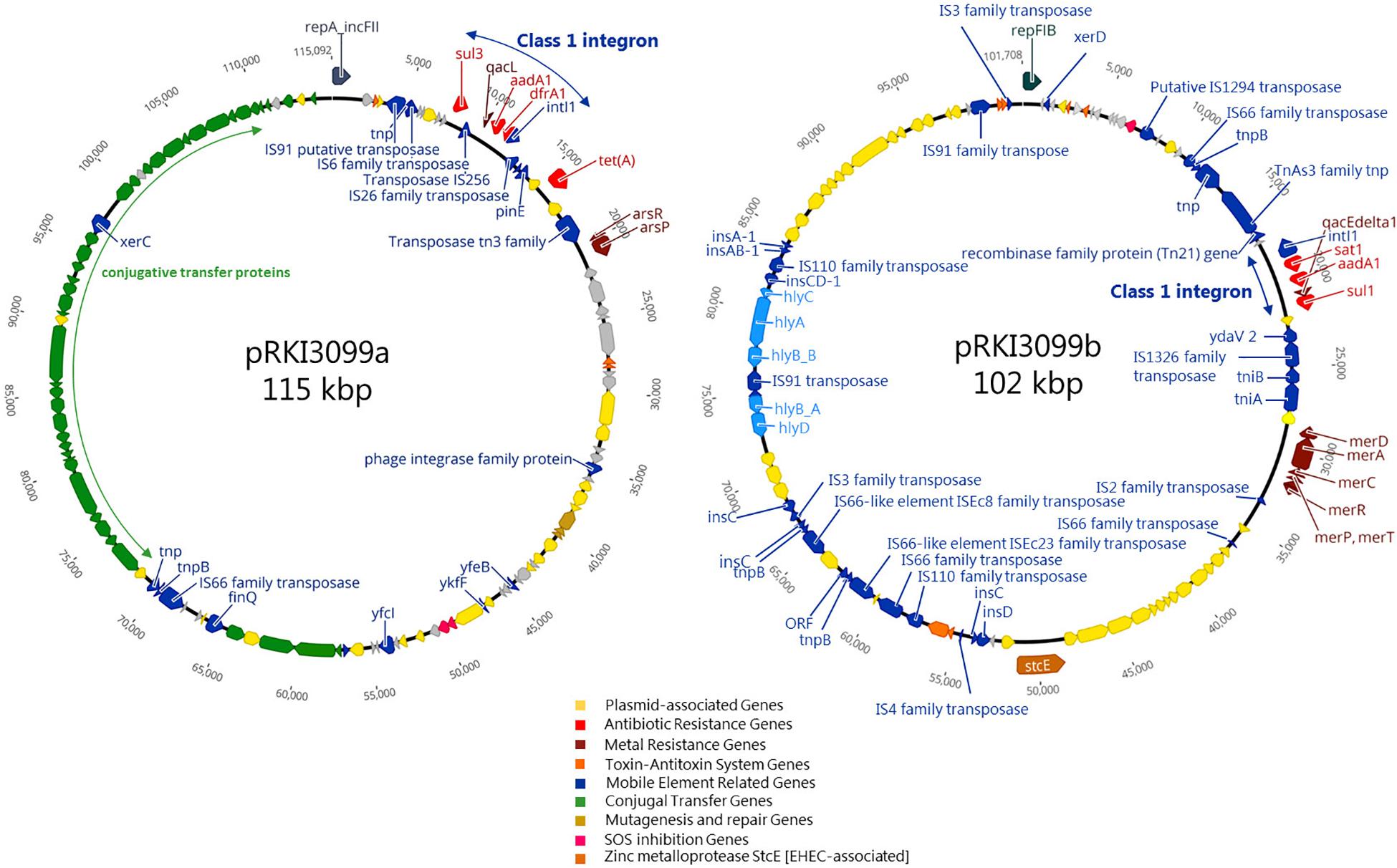
Figure 4. Schematic circular representation of plasmids pRKI3099a and pRKI3099b from E. coli isolate RKI3099. RKI3099 belonged to ST10 O182:H19 expressing ZnCl2 MIC of 512 μg/ml. The predicted protein function are indicated by color code as follows: plasmid-association (yellow), antibiotic resistance (red), metal resistance (dark red), toxin-antitoxin system (orange), mobile genetic elements (blue), conjugal transfer (green), mutagenesis and repair (gold), SOS inhibition (pink) and the zinc metalloprotease StcE (brown). Plasmid pRKI3099a (115 kpb; IncFII) and pRKI3099b (102 kbp; IncFIB) carry class 1 integron variants by integrase IntI1 (small blue arrows), and a disrupted form of the biocide resistance gene qacE (qacEΔ1) or the biocide resistance gene qacL, as well as the sulfonamide resistance genes sul1 or sul3.
As a result, two novel plasmids (Figure 4) with a size of 115 kb (pRKI3099a; accession number MN124285) and 102 kb (pRKI3099b; accession number MN124286) were identified. Both plasmids carry class I integron variants characterized by integrase IntI1, and either a disrupted form of the biocide resistance gene qacE (qacEΔ1) (Paulsen et al., 1996) or the biocide resistance gene qacL, as well as the sulfonamide resistance genes sul1 (RKI3099b) or sul3 (pRKI3099a). The plasmid pRKI3099a is a IncFII-type plasmid that also carries the tetracycline resistance gene tetA and the nearly 40 kb transfer operon (tra) which is essential for F-plasmid transfer (Frost et al., 1994). The IncFIB plasmid RKI3099b harbors the mercury resistance operon merRTPCDAB, diverse insertion sequences and a hly-operon disrupted by IS91. Additionally, both plasmids include typical plasmid partitioning genes such as toxin-antitoxin systems (e.g., vapB/C and hicA/B) and further mobile element related genes (Figure 4).
Taken together, the occurrence of certain ARGs is more associated with the genomic background and serotype of the E. coli investigated here than with a particular zinc tolerance level or feeding group. Consequently, we decided to abstain from further statistic calculation for individual resistance genes clearly mirroring frequencies of certain genomic lineages only.
Genes known to confer resistance/tolerance toward biocides and heavy metals located on plasmids seem to have a strong association (p ≤ 0.05) with isolates of the HZG, comprising different STs and serotypes (Supplementary Table S1). However, considering the ZnCl2 MIC of 512 μg/ml within the HZG, only isolates belonging to ST10 O182:H19 carried the above mentioned plasmids harboring biocide resistance (e.g., quaternary ammonium compound efflux transporters encoded by qacL or qacEΔ1) and heavy metal tolerance genes (e.g., mercury resistance operon merRTPCDAB) alongside further ARGs (Figure 4).
Discussion
Former studies revealed an increase in antibiotic resistance among E. coli (Holzel et al., 2012; Bednorz et al., 2013) and other bacteria (Aarestrup et al., 2010; Cavaco et al., 2010) obtained from pig manure which were previously exposed to high zinc supplemented diets. Although a general correlation between heavy metal – and antibiotic resistance seems to exist (Pal et al., 2015; Yu et al., 2017), so far only a few factors conferring heavy metal resistance in different bacterial species have been identified, mostly located alongside ARGs on mobile genetic elements such as insertion sequences and composite transposons (Rice and Carias, 1998), unit transposons (Rubens et al., 1979; Liebert et al., 1999), gene cassettes and integrons (Kholodii et al., 2003; Petrova et al., 2011) and plasmids (Carattoli, 2013; Fernandez-Lopez et al., 2016). A prominent example might be the zinc-resistance factor Crz identified on the staphylococcal chromosomal cassette conferring methicillin resistance (SCCmec type V) in S. aureus from livestock (Cavaco et al., 2011).
Zinc Tolerance Levels, Antibiotic- and Biocide Susceptibility of Porcine Intestinal E. coli
Here we report about phenotypic and genotypic characteristics of 179 E. coli selected from a collection obtained from piglets fed with either a zinc-rich diet (n = 8) or a common piglet diet (n = 8). Considering the ZnCl2 MICs in this study, our isolate collection seems to reflect the naturally occurring unimodal distribution of zinc tolerance levels most likely lacking non-wildtype phenotypes, as previously reported for E. coli of avian and human origin (Deus et al., 2017). So far, an association between a ZnCl2 MIC and a particular antibiotic resistance phenotype has not been detected.
Nonetheless, the linear mixed regression model revealed a statistically significant association between “higher” ZnCl2 MICs and isolates representing the HZG as well as “lower ZnCl2 MICs” with isolates of the CG (p = 0.005), indicating a selective advantage of distinct commensal E. coli lineages in the presence of high amounts of zinc in the piglets’ diet. One exception is E. coli belonging to ST4577 (O8:H8). This genotype shows a 512 μg/ml zinc MIC, and while it occurs in samples from both feeding groups, it is nonetheless only rarely found among CG isolates (2.5%) (Figure 3).
A recent study showed that zinc inhibits virulence expression of diarrheagenic E. coli by inducing the bacterial envelope stress response while inhibiting the SOS response (Bunnell et al., 2017). Moreover, zinc salts were found to be capable of reducing SOS-induced hypermutation through error-prone polymerases in the presence of antibiotics (Bunnell et al., 2017). Consequently, isolates exhibiting comparatively higher levels of zinc tolerance might reflect a more inert SOS-system activation, gaining a selective advantage by preventing early SOS-induced hypermutation, even at sub-inhibitory concentrations of antibiotics. Clearly, further studies on that particular subject are needed, as our comprehensive in silico analysis did not give any explanation about which mechanism might be responsible for the variety of zinc tolerance measured.
The distribution of MICs obtained for further heavy metals and different biocides suggested the lack of a non-wild type population among the isolate collection, as assumed before (Deus et al., 2017). The mixed linear regression model set-up for lg2 chlorhexidine MICs indicated a putative association of lg2 chlorhexidine MICs and lg2 ZnCl2 MICs in the different feeding groups. However, the complex nature of interactions between biocides and heavy metals and their possible effects on bacterial populations needs to be further investigated.
Genomic Background and Genes Involved in Zinc (Heavy Metal)- and Biocide Tolerance
Escherichia coli belonging to the STC10 (ST10 and ST34) were most common among the isolates of both groups (HZG, 50.5%; CG, 40%). This finding is in accordance with a recent study which summarizes that in Germany, Denmark, Ireland, and Spain STC10 is the dominant genomic lineage among commensal E. coli from pigs (Cortés et al., 2010; Bednorz et al., 2013; Herrero-Fresno et al., 2015; Wang et al., 2016; Ahmed et al., 2017) and reported this lineage as being predominant among intestinal E. coli from Australian pigs’ as well (Reid et al., 2017). Moreover, E. coli belonging to STC10 have been characterized as opportunistic, frequently associated with multidrug resistance and widely distributed among a broad host range (Alcalá et al., 2016; Cordoni et al., 2016; Guenther et al., 2017).
Based on former studies, several genes have been emphasized with respect to their capability to confer increased zinc tolerance or even -resistance in E. coli. In this context, the P1b-type ATPase ZntA and the CDF ZitB have been frequently nominated (Hantke, 2005; Ding et al., 2012; Porcheron et al., 2013; Watly et al., 2016; Deus et al., 2017; Ojer-Usoz et al., 2017). In addition, the protein ZraP associated with zinc-resistance, the transcriptional regulatory protein ZraR (synonym: HydG), the serine acetyltransferase CysE and the low-affinity inorganic phosphate transporter PitA have been linked to zinc tolerance (Casewell et al., 2003; Hoegler and Hecht, 2018; Stocks et al., 2019). We were able to provide evidence that genes encoding these and many other factors involved in bacterial zinc hemostasis were present in almost all 179 isolates investigated, indicating that the sheer presence of these factors or even certain combinations do not confer zinc tolerance MICs deviating from the unimodal distribution.
As was described for ZupT, point mutations are able to change the kinetics of metal uptake systems toward an increase zinc tolerance (Taudte and Grass, 2010). However, isolates investigated here lack any ZupT amino acid sequence variation (Table 5). Considering the predicted amino acid variations of other zinc-associated proteins, differences seem to be lineage-specific and lack particular associations with the distinct zinc MICs (Table 5).
Accumulations of metals such as copper and zinc have also been linked to antibiotic resistance development in environmental bacteria, as thoroughly reviewed by Poole (2017). However, molecular mechanisms responsible for the presence as well as absence of heavy metal-, antibiotic-, and biocide co-resistances are not fully understood yet (Yu et al., 2017). A putative effect of overexpressing non-specific efflux pumps conferring phenotypic resistance to antibiotics, biocides and heavy metals, as described for Listeria monocytogenes (Mata et al., 2000) to the phenotypes reported here cannot be ruled out completely. Therefore, studying the transcriptomic response of distinct genomic lineages with respect to different ZnCl2 MICs might reveal the most relevant factors explaining our results. However, for each of the antibiotic resistance phenotypes detected in our isolates at least one corresponding ARG was identified (Supplementary Table S1), clearly reasoning against a primary role of any non-specific efflux pumps.
In the past, there has been an extensive and substantial discussion about fitness cost(s) of ARGs for bacteria, especially E. coli (Melnyk et al., 2015), which is beyond the scope of this project. However, the ZnCl2 MIC 512 μg/ml value is associated with either ST10 O182:H19 and the ARGs aadA1, dfrA1, sul1, sul3, and tetA (Figure 4) conferring resistance to aminoglycosides, trimethoprim, sulfonamides and tetracycline or no ARG at all (6 STs; 6 serotypes) (Supplementary Table S1 and Figure 2). Notably, most of these ARGs in ST10 O182:H19 are associated with class I integrons, which are commonly regarded as “low-cost structures,” known to promote selection while confronted with sub-inhibitory antibiotic concentrations (Lacotte et al., 2017). Thus, picturing a co-selective pressure above the minimal selective pressure induced by zinc excess together with any of the antibiotics mentioned, a clear selective advantage, even if associated with cost(s), seems reasonable for this serotype, as it was discussed for appearance and elimination of resistance genes before (Yu et al., 2017). Moreover, a former study from Australia revealed commensal E. coli from pigs as a reservoir for class 1 integrons, frequently associated with three or more ARGs as well as genes conferring heavy metal tolerance (Reid et al., 2017). As proposed, biocides may promote dissemination of mobile genetic elements and hence resistance genes (Gillings et al., 2008). Moreover, biocides may have driven the fixation and spread of class 1 integrons, responsible for a major part of antibiotic resistance (Gillings et al., 2008).
Almost all 179 E. coli described here harbored the chromosomally encoded AcrAB-TolC-system which is known to decrease susceptibility toward a wide variety of antibiotics and biocides including acriflavine (Buffet-Bataillon et al., 2012). While detoxification by overexpression of AcrAB-TolC and other efflux pumps (e.g., MdtEF-TolC) has been reported before (Novoa and Conroy-Ben, 2019), this study aimed to evaluate the genes whose presence were described as responsible or involved in increasing bacterial zinc tolerance (Table 5), an assumption we have clearly rejected. Nonetheless, regulatory proteins such as SoxS for AcrAB are sensitive for oxidative stress (Harrison et al., 2009) induced by different metal ions, subsequently leading to an increased expression of the corresponding efflux system while mediating tolerance toward a broad range of antibiotics (Seiler and Berendonk, 2012). Consequently, further research on differences in transcription patterns during zinc-induced stress might reveal the factors essential for increased zinc tolerance in particular E. coli lineages.
Conclusion
Using comprehensive phenotypical and in silico analyses, this study sheds light on the effects of high-zinc oxide diets on intestinal E. coli populations in weaned piglets: An association of the isolates’ ZnCl2 MIC with the feeding group was obvious, while neither the presence nor the rare absence of a specific gene or gene combination involved in cellular zinc homeostasis could be identified to be associated with a particular degree of zinc tolerance. Thus, a simple model of co-selection does not account for the different levels of zinc tolerance reported here.
Data Availability Statement
The datasets generated for this study can be found in the NCBI database. A full list for all 179 entries is provided in Supplementary Table S4.
Author Contributions
LW, AL-B, and AB designed the project. VJ and BW conceived and designed the experiments. IE sequenced the isolates. VJ performed the laboratory analysis. VJ, BW, LE, TS, FG, RM, and YP analyzed the data. VJ, BW, and LW wrote the manuscript. All authors have read and approved the final draft of the manuscript.
Funding
This work was funded by the Deutsche Forschungsgemeinschaft (DFG no. WI 1436/12-1). AL-B, AB, and BW were supported by the project #1Health-PREVENT (Grants 01KI1727F and 01KI1727D) from the German Federal Ministry of Education and Research (BMBF). LE was supported by the project PAC-CAMPY (Grant 01KI1725F) within the German Research Network of Zoonotic Diseases. The funders had no role in the study design, data collection and analysis, decision to publish, or preparation of the manuscript.
Conflict of Interest
The authors declare that the research was conducted in the absence of any commercial or financial relationships that could be construed as a potential conflict of interest.
Acknowledgments
We thank Julia Assmann, Charlotte Huber, Anne Kauter, and the colleagues from the Advanced Light and Electron Microscopy (ZBS-4) department of the Robert Koch Institute for their individual contribution and support of this project. We also thank Petra Krienke for her excellent technical assistance in the sequence lab. We also thank Esther-Maria Antao for language assistance with the manuscript and Carina Jahnke for figure converting.
Supplementary Material
The Supplementary Material for this article can be found online at: https://www.frontiersin.org/articles/10.3389/fmicb.2019.02734/full#supplementary-material
References
Aarestrup, F. M., Cavaco, L., and Hasman, H. (2010). Decreased susceptibility to zinc chloride is associated with methicillin resistant Staphylococcus aureus CC398 in Danish swine. Vet. Microbiol. 142, 455–457. doi: 10.1016/j.vetmic.2009.10.021
Agga, G. E., Scott, H. M., Amachawadi, R. G., Nagaraja, T. G., Vinasco, J., Bai, J., et al. (2014). Effects of chlortetracycline and copper supplementation on antimicrobial resistance of fecal Escherichia coli from weaned pigs. Prev. Vet. Med. 114, 231–246. doi: 10.1016/j.prevetmed.2014.02.010
Ahmed, S., Olsen, J. E., and Herrero-Fresno, A. (2017). The genetic diversity of commensal Escherichia coli strains isolated from non-antimicrobial treated pigs varies according to age group. PLoS One 12:e0178623. doi: 10.1371/journal.pone.0178623
Alcalá, L., Alonso, C. A., Simón, C., González-Esteban, C., Orós, J., Rezusta, A., et al. (2016). Wild birds, frequent carriers of extended-spectrum β-lactamase (ESBL) producing Escherichia coli of CTX-M and SHV-12 Types. Microb. Ecol. 72, 861–869. doi: 10.1007/s00248-015-0718-0
Aldema, M. L., Mcmurry, L. M., Walmsley, A. R., and Levy, S. B. (1996). Purification of the Tn 10-specified tetracycline efflux antiporter TetA in a native state as a polyhistidine fusion protein. Mol. Microbiol. 19, 187–195. doi: 10.1046/j.1365-2958.1996.359886.x
Antunes, P., Machado, J., Sousa, J. C., and Peixe, L. (2005). Dissemination of sulfonamide resistance genes (sul1, sul2, and sul3) in portuguese Salmonella enterica strains and relation with integrons. Antimicrob. Agents Chemother. 49, 836–839.
Argudín, M. A., Hoefer, A., and Butaye, P. (2019). Heavy metal resistance in bacteria from animals. Res. Vet. Sci. 122, 132–147. doi: 10.1016/j.rvsc.2018.11.007
Baker-Austin, C., Wright, M. S., Stepanauskas, R., and Mcarthur, J. V. (2006). Co-selection of antibiotic and metal resistance. Trends Microbiol. 14, 176–182. doi: 10.1016/j.tim.2006.02.006
Beard, S. J., Hashim, R., Wu, G., Binet, M. R. B., Hughes, M. N., and Poole, R. K. (2000). Evidence for the transport of zinc(II) ions via the Pit inorganic phosphate transport system in Escherichia coli. FEMS Microbiol. Lett. 184, 231–235. doi: 10.1016/s0378-1097(00)00055-0
Becerra-Castro, C., Machado, R. A., Vaz-Moreira, I., and Manaia, C. M. (2015). Assessment of copper and zinc salts as selectors of antibiotic resistance in Gram-negative bacteria. Sci. Total Environ. 53, 367–372. doi: 10.1016/j.scitotenv.2015.05.102
Bednorz, C., Oelgeschläger, K., Kinnemann, B., Hartmann, S., Neumann, K., Pieper, R., et al. (2013). The broader context of antibiotic resistance: zinc feed supplementation of piglets increases the proportion of multi-resistant Escherichia coli in vivo. Int. J. Med. Microbiol. 303, 396–403. doi: 10.1016/j.ijmm.2013.06.004
Blaby-Haas, C. E., Flood, J. A., Crecy-Lagard, V. D., and Zamble, D. B. (2012). YeiR: a metal-binding GTPase from Escherichia coli involved in metal homeostasis. Metallomics 4, 488–497. doi: 10.1039/c2mt20012k
Blázquez, E., Rodríguez, C., Ródenas, J., Pérez De Rozas, A., Campbell, J. M., Segalés, J., et al. (2018). Evaluation of ultraviolet-C and spray-drying processes as two independent inactivation steps on enterotoxigenic Escherichia coli K88 and K99 strains inoculated in fresh unconcentrated porcine plasma. Lett. Appl. Microbiol. 67, 442–448. doi: 10.1111/lam.13068
Blindauer, C. A., Harrison, M. D., Robinson, A. K., Parkinson, J. A., Bowness, P. W., Sadler, P. J., et al. (2002). Multiple bacteria encode metallothioneins and SmtA-like zinc fingers. Mol. Microbiol. 45, 1421–1432. doi: 10.1046/j.1365-2958.2002.03109.x
Buffet-Bataillon, S., Le Jeune, A., Le Gall-David, S., Bonnaure-Mallet, M., and Jolivet-Gougeon, A. (2012). Molecular mechanisms of higher MICs of antibiotics and quaternary ammonium compounds for Escherichia coli isolated from bacteraemia. J. Antimicrob. Chemother. 67, 2837–2842. doi: 10.1093/jac/dks321
Bunnell, B. E., Escobar, J. F., Bair, K. L., Sutton, M. D., and Crane, J. K. (2017). Zinc blocks SOS-induced antibiotic resistance via inhibition of RecA in Escherichia coli. PLoS One 12:e0178303. doi: 10.1371/journal.pone.0178303
Carattoli, A. (2013). Plasmids and the spread of resistance. Int. J. Med. Microbiol. 303, 298–304. doi: 10.1016/j.ijmm.2013.02.001
Carattoli, A., Zankari, E., García-Fernández, A., Voldby Larsen, M., Lund, O., Villa, L., et al. (2014). In silico detection and typing of plasmids using plasmidfinder and plasmid multilocus sequence typing. Antimicrob. Agents Chemother. 58, 3895–3903. doi: 10.1128/AAC.02412-14
Casewell, M., Friis, C., Marco, E., Mcmullin, P., and Phillips, I. (2003). The European ban on growth-promoting antibiotics and emerging consequences for human and animal health. J. Antimicrob. Chemother. 52, 159–161. doi: 10.1093/jac/dkg313
Cavaco, L. M., Hasman, H., and Aarestrup, F. M. (2011). Zinc resistance of Staphylococcus aureus of animal origin is strongly associated with methicillin resistance. Vet. Microbiol. 150, 344–348. doi: 10.1016/j.vetmic.2011.02.014
Cavaco, L. M., Hasman, H., Stegger, M., Andersen, P. S., Skov, R., Fluit, A. C., et al. (2010). Cloning and occurrence of czrC, a gene conferring cadmium and zinc resistance in methicillin-resistant Staphylococcus aureus CC398 isolates. Antimicrob. Agents Chemother. 54, 3605–3608. doi: 10.1128/AAC.00058-10
Choi, S.-H., Lee, K.-L., Shin, J.-H., Cho, Y.-B., Cha, S.-S., and Roe, J.-H. (2017). Zinc-dependent regulation of zinc import and export genes by Zur. Nat. Commun. 8:15812. doi: 10.1038/ncomms15812
Ciesinski, L., Guenther, S., Pieper, R., Kalisch, M., Bednorz, C., and Wieler, L. H. (2018). High dietary zinc feeding promotes persistence of multi-resistant E. coli in the swine gut. PLoS One 13:e0191660. doi: 10.1371/journal.pone.0191660
Clinical and Laboratory Standards Institute (2012). Performance Standards for Antimicrobial Susceptibility Testing; Twenty-Second Informational Supplement M100-S21. Wayne, PA: Clinical and Laboratory Standards Institute.
Clinical and Laboratory Standards Institute (2013). Vet01-S2 Performance Standards for Antimicrobial Disk and Dilution Susceptibility for Bacteria Isolated from Animals. Second International Supplement. Wayne, PA: Clinical and Laboratory Standards Institute.
Colaço, H. G., Santo, P. E., Matias, P. M., Bandeiras, T. M., and Vicente, J. B. (2016). Roles of Escherichia coli ZinT in cobalt, mercury and cadmium resistance and structural insights into the metal binding mechanism. Metallomics 8, 327–336. doi: 10.1039/c5mt00291e
Cordoni, G., Woodward, M. J., Wu, H., Alanazi, M., Wallis, T., and La Ragione, R. M. (2016). Comparative genomics of European avian pathogenic E. coli (APEC). BMC Genomics 17:960–960.
Cortés, P., Blanc, V., Mora, A., Dahbi, G., Blanco, J. E., Blanco, M., et al. (2010). Isolation and characterization of potentially pathogenic antimicrobial-resistant Escherichia coli strains from chicken and pig farms in Spain. Appl. Environ. Microbiol. 76, 2799–2805. doi: 10.1128/AEM.02421-09
Deus, D., Krischek, C., Pfeifer, Y., Sharifi, A. R., Fiegen, U., Reich, F., et al. (2017). Comparative analysis of the susceptibility to biocides and heavy metals of extended-spectrum β-lactamase-producing Escherichia coli isolates of human and avian origin, Germany. Diagn. Microbiol. Infect. Dis. 88, 88–92. doi: 10.1016/j.diagmicrobio.2017.01.023
Ding, Y., Tang, X., Lu, P., Wu, B., Xu, Z., Liu, W., et al. (2012). Clonal analysis and virulent traits of pathogenic extraintestinal Escherichia coli isolates from swine in China. BMC Vet. Res. 8:140. doi: 10.1186/1746-6148-8-140
Fairbrother, J. M., Nadeau, É, and Gyles, C. L. (2005). Escherichia coli in postweaning diarrhea in pigs: an update on bacterial types, pathogenesis, and prevention strategies. Anim. Health Res. Rev. 6, 17–39. doi: 10.1079/ahr2005105
Fang, L., Li, X., Li, L., Li, S., Liao, X., Sun, J., et al. (2016). Co-spread of metal and antibiotic resistance within ST3-IncHI2 plasmids from E. coli isolates of food-producing animals. Sci. Rep. 6:25312. doi: 10.1038/srep25312
Fard, R. M. N., Heuzenroeder, M. W., and Barton, M. D. (2011). Antimicrobial and heavy metal resistance in commensal enterococci isolated from pigs. Vet. Microbiol. 148, 276–282. doi: 10.1016/j.vetmic.2010.09.002
Fernandez-Lopez, R., De Toro, M., Moncalian, G., Garcillan-Barcia, M. P., and De La Cruz, F. (2016). Comparative genomics of the conjugation region of f-like plasmids: five shades of F. Front. Mol. Biosci. 3:71. doi: 10.3389/fmolb.2016.00071
Frost, L. S., Ippen-Ihler, K., and Skurray, R. A. (1994). Analysis of the sequence and gene products of the transfer region of the F sex factor. Microbiol. Rev. 58, 162–210.
Gielda, L. M., and DiRita, V. J. (2012). Zinc competition among the intestinal microbiota. mBio 3:e171-12. doi: 10.1128/mBio.00171-12
Gillings, M. R., Xuejun, D., Hardwick, S. A., Holley, M. P., and Stokes, H. W. (2008). Gene cassettes encoding resistance to quaternary ammonium compounds: a role in the origin of clinical class 1 integrons? ISME J. 3:209. doi: 10.1038/ismej.2008.98
Graham, A. I., Hunt, S., Stokes, S. L., Bramall, N., Bunch, J., Cox, A. G., et al. (2009). Severe zinc depletion of Escherichia coli: roles for high affinity zinc binding by ZinT, zinc transport and zinc-independent proteins. J. Biol. Chem. 284, 18377–18389. doi: 10.1074/jbc.M109.001503
Grass, G., Franke, S., Taudte, N., Nies, D. H., Kucharski, L. M., Maguire, M. E., et al. (2005). The metal permease zupT from Escherichia coli is a transporter with a broad substrate spectrum. J. Bacteriol. 187, 1604–1611. doi: 10.1128/jb.187.5.1604-1611.2005
Guenther, S., Falgenhauer, L., Semmler, T., Imirzalioglu, C., Chakraborty, T., Roesler, U., et al. (2017). Environmental emission of multiresistant Escherichia coli carrying the colistin resistance gene mcr-1 from German swine farms. J. Antimicrob. Chemother. 72, 1289–1292. doi: 10.1093/jac/dkw585
Hantke, K. (2005). Bacterial zinc uptake and regulators. Curr. Opin. Microbiol. 8, 196–202. doi: 10.1016/j.mib.2005.02.001
Harrison, J. J., Tremaroli, V., Stan, M. A., Chan, C. S., Vacchi-Suzzi, C., Heyne, B. J., et al. (2009). Chromosomal antioxidant genes have metal ion-specific roles as determinants of bacterial metal tolerance. Environ. Microbiol. 11, 2491–2509. doi: 10.1111/j.1462-2920.2009.01973.x
Hensley, M. P., Gunasekera, T. S., Easton, J. A., Sigdel, T. K., Sugarbaker, S. A., Klingbeil, L., et al. (2012). Characterization of Zn(II)-responsive ribosomal proteins YkgM and L31 in E. coli. J. Inorg. Biochem. 111, 164–172. doi: 10.1016/j.jinorgbio.2011.11.022
Herrero-Fresno, A., Larsen, I., and Olsen, J. E. (2015). Genetic relatedness of commensal Escherichia coli from nursery pigs in intensive pig production in Denmark and molecular characterization of genetically different strains. J. Appl. Microbiol. 119, 342–353. doi: 10.1111/jam.12840
Hoegler, K. J., and Hecht, M. H. (2018). Artificial gene amplification in Escherichia coli reveals numerous determinants for resistance to metal toxicity. J. Mol. Evol. 86, 103–110. doi: 10.1007/s00239-018-9830-3
Hollingshead, S., and Vapnek, D. (1985). Nucleotide sequence analysis of a gene encoding a streptomycin/spectinomycin adenylyltransferase. Plasmid 13, 17–30. doi: 10.1016/0147-619x(85)90052-6
Holzel, C. S., Muller, C., Harms, K. S., Mikolajewski, S., Schafer, S., Schwaiger, K., et al. (2012). Heavy metals in liquid pig manure in light of bacterial antimicrobial resistance. Environ. Res. 113, 21–27. doi: 10.1016/j.envres.2012.01.002
Jia, B., Raphenya, A. R., Alcock, B., Waglechner, N., Guo, P., Tsang, K. K., et al. (2017). CARD 2017: expansion and model-centric curation of the comprehensive antibiotic resistance database. Nucleic Acids Res. 45, D566–D573. doi: 10.1093/nar/gkw1004
Joensen, K. G., Tetzschner, A. M. M., Iguchi, A., Aarestrup, F. M., and Scheutz, F. (2015). Rapid and easy in silico serotyping of Escherichia coli isolates by use of whole-genome sequencing data. J. Clin. Microbiol. 53, 2410–2426. doi: 10.1128/JCM.00008-15
Kholodii, G., Mindlin, S., Petrova, M., and Minakhina, S. (2003). Tn5060 from the Siberian permafrost is most closely related to the ancestor of Tn21 prior to integron acquisition. FEMS Microbiol. Lett. 226, 251–255. doi: 10.1016/s0378-1097(03)00559-7
Kloubert, V., Blaabjerg, K., Dalgaard, T. S., Poulsen, H. D., Rink, L., and Wessels, I. (2018). Influence of zinc supplementation on immune parameters in weaned pigs. J. Trace Elem. Med. Biol. 49, 231–240. doi: 10.1016/j.jtemb.2018.01.006
Lacotte, Y., Ploy, M.-C., and Raherison, S. (2017). Class 1 integrons are low-cost structures in Escherichia coli. ISME J. 11, 1535–1544. doi: 10.1038/ismej.2017.38
Larsen, M. V., Cosentino, S., Rasmussen, S., Friis, C., Hasman, H., Marvig, R. L., et al. (2012). Multilocus sequence typing of total-genome-sequenced bacteria. J. Clin. Microbiol. 50, 1355–1361. doi: 10.1128/JCM.06094-11
Lee, L. J., Barrett, J. A., and Poole, R. K. (2005). Genome-wide transcriptional response of chemostat-cultured Escherichia coli to zinc. J. Bacteriol. 187, 1124–1134. doi: 10.1128/jb.187.3.1124-1134.2005
Li, H. H., Li, Y. P., Zhu, Q., Qiao, J. Y., and Wang, W. J. (2018). Dietary supplementation with Clostridium butyricum helps to improve the intestinal barrier function of weaned piglets challenged with enterotoxigenic Escherichia coli K88. J. Appl. Microbiol. 125, 964–975. doi: 10.1111/jam.13936
Liebert, C. A., Hall, R. M., and Summers, A. O. (1999). Transposon Tn21, flagship of the floating genome. Microbiol. Mol. Biol. Rev. 63, 507–522.
Lu, M., Chai, J., and Fu, D. (2009). Structural basis for autoregulation of the zinc transporter YiiP. Nat. Struct. Mol. Biol. 16, 1063–1067. doi: 10.1038/nsmb.1662
Mata, M. T., Baquero, F., and Pérez-Díaz, J. C. (2000). A multidrug efflux transporter in Listeria monocytogenes. FEMS Microbiol. Lett. 187, 185–188. doi: 10.1016/s0378-1097(00)00199-3
Medardus, J. J., Molla, B. Z., Nicol, M., Morrow, W. M., Rajala-Schultz, P. J., Kazwala, R., et al. (2014). In-feed use of heavy metal micronutrients in U.S. swine production systems and its role in persistence of multidrug-resistant salmonellae. Appl. Environ. Microbiol. 80, 2317–2325. doi: 10.1128/AEM.04283-13
Melnyk, A. H., Wong, A., and Kassen, R. (2015). The fitness costs of antibiotic resistance mutations. Evol. Appl. 8, 273–283. doi: 10.1111/eva.12196
Moon, H. W., and Bunn, T. O. (1993). Vaccines for preventing enterotoxigenic Escherichia coli infections in farm animals. Vaccine 11, 213–220. doi: 10.1016/0264-410x(93)90020-x
Novoa, D., and Conroy-Ben, O. (2019). The anaerobic efflux pump MdtEF-TolC confers resistance to cationic biocides. bioRxiv [Preprint]. doi: 10.1101/570408
Ojer-Usoz, E., González, D., and Vitas, A. I. (2017). Clonal diversity of ESBL-producing Escherichia coli isolated from environmental, human and food samples. Int. J. Environ. Res. Public Health 14:676. doi: 10.3390/ijerph14070676
Pal, C., Bengtsson-Palme, J., Kristiansson, E., and Larsson, D. G. J. (2015). Co-occurrence of resistance genes to antibiotics, biocides and metals reveals novel insights into their co-selection potential. BMC Genomics 16:964. doi: 10.1186/s12864-015-2153-5
Pal, C., Bengtsson-Palme, J., Rensing, C., Kristiansson, E., and Larsson, D. G. J. (2013). BacMet: antibacterial biocide and metal resistance genes database. Nucleic Acids Res. 42, D737–D743. doi: 10.1093/nar/gkt1252
Paulsen, I. T., Brown, M. H., and Skurray, R. A. (1996). Proton-dependent multidrug efflux systems. Microbiol. Rev. 60, 575–608.
Pawlowski, A. C., Stogios, P. J., Koteva, K., Skarina, T., Evdokimova, E., Savchenko, A., et al. (2018). The evolution of substrate discrimination in macrolide antibiotic resistance enzymes. Nat. Commun. 9:112. doi: 10.1038/s41467-017-02680-0
Petit-Hartlein, I., Rome, K., De Rosny, E., Molton, F., Duboc, C., Gueguen, E., et al. (2015). Biophysical and physiological characterization of ZraP from Escherichia coli, the periplasmic accessory protein of the atypical ZraSR two-component system. Biochem. J. 472, 205–216. doi: 10.1042/BJ20150827
Petrova, M., Gorlenko, Z., and Mindlin, S. (2011). Tn5045, a novel integron-containing antibiotic and chromate resistance transposon isolated from a permafrost bacterium. Res. Microbiol. 162, 337–345. doi: 10.1016/j.resmic.2011.01.003
Pieper, R., Martin, L., Schunter, N., Villodre Tudela, C., Weise, C., Klopfleisch, R., et al. (2015). Impact of high dietary zinc on zinc accumulation, enzyme activity and proteomic profiles in the pancreas of piglets. J. Trace Elem. Med. Biol. 30, 30–36. doi: 10.1016/j.jtemb.2015.01.008
Poole, K. (2017). At the nexus of antibiotics and metals: the impact of cu and zn on antibiotic activity and resistance. Trends Microbiol. 25, 820–832. doi: 10.1016/j.tim.2017.04.010
Porcheron, G., Garenaux, A., Proulx, J., Sabri, M., and Dozois, C. M. (2013). Iron, copper, zinc, and manganese transport and regulation in pathogenic Enterobacteria: correlations between strains, site of infection and the relative importance of the different metal transport systems for virulence. Front. Cell Infect. Microbiol. 3:90. doi: 10.3389/fcimb.2013.00090
Reid, C. J., Wyrsch, E. R., Roy Chowdhury, P., Zingali, T., Liu, M., Darling, A. E., et al. (2017). Porcine commensal Escherichia coli: a reservoir for class 1 integrons associated with IS26. Microb. Genom. 3, doi: 10.1099/mgen.0.000143
Rhouma, M., Fairbrother, J. M., Beaudry, F., and Letellier, A. (2017a). Post weaning diarrhea in pigs: risk factors and non-colistin-based control strategies. Acta Vet. Scand. 59, 31–31. doi: 10.1186/s13028-017-0299-7
Rhouma, M., Fairbrother, J. M., Thériault, W., Beaudry, F., Bergeron, N., Laurent-Lewandowski, S., et al. (2017b). The fecal presence of enterotoxin and F4 genes as an indicator of efficacy of treatment with colistin sulfate in pigs. BMC Microbiol. 17:6–6. doi: 10.1186/s12866-016-0915-0
Rice, L. B., and Carias, L. L. (1998). Transfer of Tn5385, a composite, multiresistance chromosomal element from Enterococcus faecalis. J. Bacteriol. 180, 714–721.
Roberts, M. C. (2005). Update on acquired tetracycline resistance genes. FEMS Microbiol. Lett. 245, 195–203. doi: 10.1016/j.femsle.2005.02.034
Rubens, C. E., Mcneill, W. F., and Farrar, W. E. (1979). Transposable plasmid deoxyribonucleic acid sequence in Pseudomonas aeruginosa which mediates resistance to gentamicin and four other antimicrobial agents. J. Bacteriol. 139:877.
Salverda, M. L., De Visser, J. A., and Barlow, M. (2010). Natural evolution of TEM-1 β-lactamase: experimental reconstruction and clinical relevance. FEMS Microbiol. Rev. 34, 1015–1036. doi: 10.1111/j.1574-6976.2010.00222.x
Scholz, P., Haring, V., Wittmann-Liebold, B., Ashman, K., Bagdasarian, M., and Scherzinger, E. (1989). Complete nucleotide sequence and gene organization of the broad-host-range plasmid RSF1010. Gene 75, 271–288. doi: 10.1016/0378-1119(89)90273-4
Seemann, T. (2014). Prokka: rapid prokaryotic genome annotation. Bioinformatics 30, 2068–2069. doi: 10.1093/bioinformatics/btu153
Seiler, C., and Berendonk, T. U. (2012). Heavy metal driven co-selection of antibiotic resistance in soil and water bodies impacted by agriculture and aquaculture. Front. Microbiol. 3:399–399. doi: 10.3389/fmicb.2012.00399
Sköld, O. (2001). Resistance to trimethoprim and sulfonamides. Vet. Res. 32, 261–273. doi: 10.1051/vetres:2001123
Song, J., Rensing, C., Holm, P. E., Virta, M., and Brandt, K. K. (2017). Comparison of metals and tetracycline as selective agents for development of tetracycline resistant bacterial communities in agricultural soil. Environ. Sci. Technol. 51, 3040–3047. doi: 10.1021/acs.est.6b05342
Starke, I. C., Pieper, R., Neumann, K., Zentek, J., and Vahjen, W. (2014). The impact of high dietary zinc oxide on the development of the intestinal microbiota in weaned piglets. FEMS Microbiol. Ecol. 87, 416–427. doi: 10.1111/1574-6941.12233
Stocks, C. J., Phan, M.-D., Achard, M. E. S., Nhu, N. T. K., Condon, N. D., Gawthorne, J. A., et al. (2019). Uropathogenic Escherichia coli employs both evasion and resistance to subvert innate immune-mediated zinc toxicity for dissemination. Proc. Natl. Acad. Sci. U.S.A. 116, 6341–6350. doi: 10.1073/pnas.1820870116
Subashchandrabose, S., Smith, S. N., Spurbeck, R. R., Kole, M. M., and Mobley, H. L. (2013). Genome-wide detection of fitness genes in uropathogenic Escherichia coli during systemic infection. PLoS Pathog. 9:e1003788. doi: 10.1371/journal.ppat.1003788
Taudte, N., and Grass, G. (2010). Point mutations change specificity and kinetics of metal uptake by ZupT from Escherichia coli. Biometals 23, 643–656. doi: 10.1007/s10534-010-9319-z
Turnidge, J., Kahlmeter, G., and Kronvall, G. (2006). Statistical characterisation of bacterial wild-type MIC value distributions and the determination of epidemiological cut-off values. Clin. Microbiol. Infect. 12, 418–425. doi: 10.1111/j.1469-0691.2006.01377.x
Vahjen, W., Pieper, R., and Zentek, J. (2011). Increased dietary zinc oxide changes the bacterial core and enterobacterial composition in the ileum of piglets. J. Anim. Sci. 89, 2430–2439. doi: 10.2527/jas.2010-3270
Vallee, B. L., and Auld, D. S. (1990). Zinc coordination, function, and structure of zinc enzymes and other proteins. Biochemistry 29, 5647–5659. doi: 10.1021/bi00476a001
van Alen, S., Kaspar, U., Idelevich, E. A., Köck, R., and Becker, K. (2018). Increase of zinc resistance in German human derived livestock-associated MRSA between 2000 and 2014. Vet. Microbiol. 214, 7–12. doi: 10.1016/j.vetmic.2017.11.032
Vidhyaparkavi, A., Osborne, J., and Babu, S. (2017). Analysis of zntA gene in environmental Escherichia coli and additional implications on its role in zinc translocation. Biotech 7:9. doi: 10.1007/s13205-017-0613-0
Wang, D., and Fierke, C. A. (2013). The BaeSR regulon is involved in defense against zinc toxicity in E. coli. Metallomics 5, 372–383. doi: 10.1039/c3mt20217h
Wang, D., Hosteen, O., and Fierke, C. A. (2012). ZntR-mediated transcription of zntA responds to nanomolar intracellular free zinc. J. Inorg. Biochem. 111, 173–181. doi: 10.1016/j.jinorgbio.2012.02.008
Wang, J., Gibbons, J. F., Mcgrath, K., Bai, L., Li, F., Leonard, F. C., et al. (2016). Molecular characterization of blaESBL-producing Escherichia coli cultured from pig farms in Ireland. J. Antimicrob. Chemother. 71, 3062–3065.
Warner, D. M., and Levy, S. B. (2012). SoxS increases the expression of the zinc uptake system ZnuACB in an Escherichia coli murine pyelonephritis model. J. Bacteriol. 194, 1177–1185. doi: 10.1128/JB.05451-11
Watly, J., Potocki, S., and Rowinska-Zyrek, M. (2016). Zinc homeostasis at the bacteria/host interface-from coordination chemistry to nutritional immunity. Chemistry 22, 15992–16010. doi: 10.1002/chem.201602376
Wick, R. R., Judd, L. M., Gorrie, C. L., and Holt, K. E. (2017). Unicycler: resolving bacterial genome assemblies from short and long sequencing reads. PLoS Comput. Biol. 13:e1005595. doi: 10.1371/journal.pcbi.1005595
Yan, T., Zhang, F., He, Y., Wang, X., Jin, X., Zhang, P., et al. (2018). Enterococcus faecium HDRsEf1 elevates the intestinal barrier defense against enterotoxigenic Escherichia coli and regulates occludin expression via activation of TLR-2 and PI3K signalling pathways. Lett. Appl. Microbiol. 67, 520–527. doi: 10.1111/lam.13067
Yatsunyk, L. A., Easton, J. A., Kim, L. R., Sugarbaker, S. A., Bennett, B., Breece, R. M., et al. (2008). Structure and metal binding properties of ZnuA, a periplasmic zinc transporter from Escherichia coli. J. Biol. Inorg. Chem. 13, 271–288. doi: 10.1007/s00775-007-0320-0
Yazdankhah, S., Rudi, K., and Bernhoft, A. (2014). Zinc and copper in animal feed - development of resistance and co-resistance to antimicrobial agents in bacteria of animal origin. Microb. Ecol. Health Dis. 25:25862. doi: 10.3402/mehd.v25.25862
Yu, Z., Gunn, L., Wall, P., and Fanning, S. (2017). Antimicrobial resistance and its association with tolerance to heavy metals in agriculture production. Food Microbiol. 64, 23–32. doi: 10.1016/j.fm.2016.12.009
Keywords: Escherichia coli, zinc, antimicrobial resistance, pig, heavy metal tolerance
Citation: Johanns VC, Ghazisaeedi F, Epping L, Semmler T, Lübke-Becker A, Pfeifer Y, Bethe A, Eichhorn I, Merle R, Walther B and Wieler LH (2019) Effects of a Four-Week High-Dosage Zinc Oxide Supplemented Diet on Commensal Escherichia coli of Weaned Pigs. Front. Microbiol. 10:2734. doi: 10.3389/fmicb.2019.02734
Received: 16 July 2019; Accepted: 11 November 2019;
Published: 28 November 2019.
Edited by:
Patrick Rik Butaye, Ross University School of Veterinary Medicine, Saint Kitts and NevisReviewed by:
Jeroen Dewulf, Ghent University, BelgiumCatherine M. Logue, University of Georgia, United States
Copyright © 2019 Johanns, Ghazisaeedi, Epping, Semmler, Lübke-Becker, Pfeifer, Bethe, Eichhorn, Merle, Walther and Wieler. This is an open-access article distributed under the terms of the Creative Commons Attribution License (CC BY). The use, distribution or reproduction in other forums is permitted, provided the original author(s) and the copyright owner(s) are credited and that the original publication in this journal is cited, in accordance with accepted academic practice. No use, distribution or reproduction is permitted which does not comply with these terms.
*Correspondence: Birgit Walther, d2FsdGhlcmJAcmtpLmRl
†These authors share senior authorship