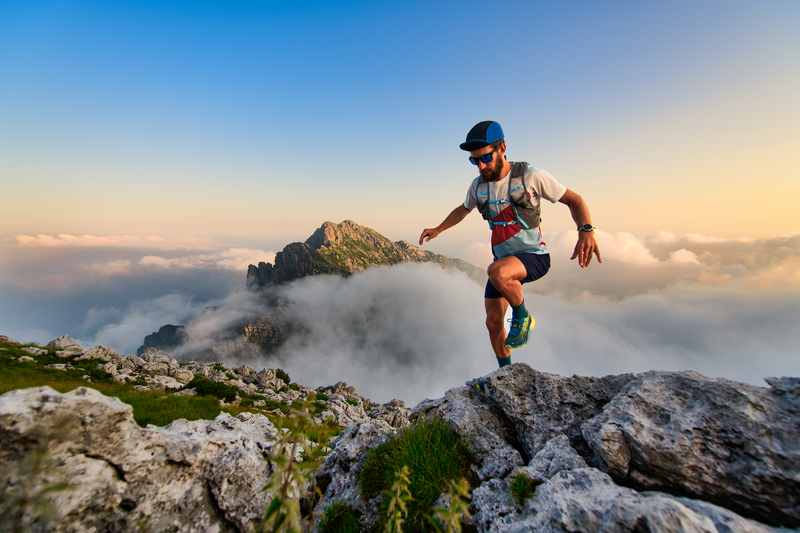
95% of researchers rate our articles as excellent or good
Learn more about the work of our research integrity team to safeguard the quality of each article we publish.
Find out more
ORIGINAL RESEARCH article
Front. Microbiol. , 09 October 2019
Sec. Microbial Physiology and Metabolism
Volume 10 - 2019 | https://doi.org/10.3389/fmicb.2019.02305
Microbial metabolites, including B complex vitamins contribute to diverse aspects of human health. Folate, or vitamin B9, refers to a broad category of biomolecules that include pterin, para-aminobenzoic acid (pABA), and glutamate subunits. Folates are required for DNA synthesis and epigenetic regulation. In addition to dietary nutrients, the gut microbiota has been recognized as a source of B complex vitamins, including folate. This study evaluated the predicted folate synthesis capabilities in the genomes of human commensal microbes identified in the Human Microbiome Project and folate production by representative strains of six human intestinal bacterial phyla. Bacterial folate synthesis genes were ubiquitous across 512 gastrointestinal reference genomes with 13% of the genomes containing all genes required for complete de novo folate synthesis. An additional 39% of the genomes had the genetic capacity to synthesize folates in the presence of pABA, an upstream intermediate that can be obtained through diet or from other intestinal microbes. Bacterial folate synthesis was assessed during exponential and stationary phase growth through the evaluation of expression of select folate synthesis genes, quantification of total folate production, and analysis of folate polyglutamylation. Increased expression of key folate synthesis genes was apparent in exponential phase, and increased folate polyglutamylation occurred during late stationary phase. Of the folate producers, we focused on the commensal Lactobacillus reuteri to examine host–microbe interactions in relation to folate and examined folate receptors in the physiologically relevant human enteroid model. RNAseq data revealed segment-specific folate receptor distribution. Treatment of human colonoid monolayers with conditioned media (CM) from wild-type L. reuteri did not influence the expression of key folate transporters proton-coupled folate transporter (PCFT) or reduced folate carrier (RFC). However, CM from L. reuteri containing a site-specific inactivation of the folC gene, which prevents the bacteria from synthesizing a polyglutamate tail on folate, significantly upregulated RFC expression. No effects were observed using L. reuteri with a site inactivation of folC2, which results in no folate production. This work sheds light on the contributions of microbial folate to overall folate status and mammalian host metabolism.
The human gut microbiota harbors a complex and dynamic microbial community that is dominated by Firmicutes and Bacteroidetes, with a lesser proportion of Actinobacteria, Proteobacteria, Fusobacteria, and Verrucomicrobia (D’Argenio and Salvatore, 2015). Collectively the intestinal microbiota plays a pivotal role in nutrient digestion and production of host-modulating metabolites (O’Connor, 2013; Berding and Donovan, 2016; Maruvada et al., 2017). The fermentation and metabolic conversion of dietary components by intestinal bacteria results in diverse metabolites such as SCFAs, long chain fatty acids, lactic acid, and biogenic amines (Cummings and Macfarlane, 1997; Kimura et al., 2002; Hayakawa et al., 2004; Izquierdo Canas et al., 2009; Tabanelli et al., 2012; Thomas et al., 2012; Frei et al., 2013; Ferstl et al., 2014; Hollister et al., 2014; Kibe et al., 2014; Luk et al., 2018; Shimizu et al., 2018). These metabolites may have an impact on the intestinal milieu and mammalian health status. Vitamins are another such end-product (Birnbaum et al., 1967; Friend et al., 1983; Cummings and Macfarlane, 1997; Hugenholtz and Smid, 2002; Crittenden et al., 2003; Streit and Entcheva, 2003; Burgess et al., 2006; Jayashree et al., 2010; LeBlanc et al., 2011, 2013b). Vitamins cannot be synthesized by mammals and therefore must be obtained through intestinal absorption from exogenous sources, including dietary sources and the gut microbiota (Rossi et al., 2011). This point is clearly demonstrated by the fact that germ-free animals lacking a microbiota require supplementation of vitamin K and certain B vitamins, while their conventionalized complete microbiota counterparts do not require these vitamins (Rossi et al., 2011). Bacterial genera common in the distal intestine, including Bacteroides, Bifidobacterium, and Enterococcus, are known to synthesize vitamins (Morowitz et al., 2011). Previous studies have identified individual species capable of producing vitamins (Birnbaum et al., 1967; Friend et al., 1983; Cummings and Macfarlane, 1997; Hugenholtz and Smid, 2002; Crittenden et al., 2003; Streit and Entcheva, 2003; Burgess et al., 2006; Jayashree et al., 2010; LeBlanc et al., 2011, 2013b). Additionally, several studies have performed in-depth analyses of vitamin production by microbes from the human intestine (Magnusdottir et al., 2015; Rowland et al., 2018; Das et al., 2019) These studies have highlighted the need for an evaluation of the folate synthesis capabilities of a broad cohort of bacterial strains, in conjunction with a detailed evaluation of the folates synthesized, as well as the specific effects of those folates on the human intestine.
Microbes produce B complex vitamins, including B1, B6, B12, and vitamin B9. Folate, or vitamin B9, is a broad category of biomolecules characterized by containing a complex of pterin, pABA, and glutamate subunits (Blakley and Benkovic, 1984). Folate biosynthesis requires a complex pathway involving 16 enzymatic processes (KEGG, 2017; Kanehisa and Goto, 2000). First, chorismate is produced from the glycolysis product, phosphoenolpyruvate which combines with pABA. GTP is converted to dihydropterin pyrophosphate prior to combining pABA and dihydropterin pyrophosphate intermediates. Finally, glutamylation and reduction yield THF, the simplest form of bioavailable folate (Scott, 1999; Rossi et al., 2011). The majority of naturally occurring folates are THF, 5-methyl THF, and 10-formyl THF, which are polyglutamylated. Bacteria synthesize mono- and poly-glutamylated folate (also known as 5,10-methenyl- THF or FPG), folate forms that are easily processed and absorbed by mammalian cells (Kim T. H. et al., 2004; Thomas et al., 2016). Microbe-generated vitamins, including folate, are primarily absorbed in the colon, while dietary vitamins are largely absorbed in the small intestine (Ichihashi et al., 1992; Said and Mohammed, 2006). Several bacterial strains within the human microbiome synthesize and secrete THFs which can be absorbed by the intestinal epithelium (Camilo et al., 1996; Asrar and O’Connor, 2005; Maynard et al., 2018). Previous studies have evaluated potential probiotic strains such as Bifidobacterium and Lactobacillus as a source of folate compounds (LeBlanc et al., 2011, 2013b). However, few studies have examined the capacity of the gut microbiome to generate folate. Moreover, a paucity of data have been published defining microbe-mediated folate modifications, such as polyglutamylation.
On the host side, dietary and microbe-produced folate uptake occurs through four proteins: folate receptor alpha (FOLR1), folate receptor beta (FOLR2), PCFT, or RFC (Zhao and Goldman, 2007; Visentin et al., 2014). FOLR1 and FOLR2 are localized to tissue outside the intestine and neither receptor is believed to contribute significantly to intestinal folate absorption (Hague et al., 1995; Barber et al., 1999; Kim, 1999, 2006; Kamen and Smith, 2004; Kim D. H. et al., 2004; Blom et al., 2006; Pitkin, 2007; Vollset et al., 2013; Wibowo et al., 2013; Zhao and Goldman, 2013; Visentin et al., 2014; Shen et al., 2015; Balashova et al., 2018). In contrast PCFT and RFC folate transporters are postulated to play a significant role in intestinal folate transport (Visentin et al., 2014). To the best of our knowledge, no study has examined folate transporter distribution along the length of the human intestine or identified folate transport/assimilation mechanisms in different regions of the gastrointestinal tract. Existing studies examining folate receptor expression and function have relied on cancer-derived cell lines. However, intestinal cancer cells exhibit a hallmark upregulation of FOLR1, which is not normally present in the healthy colon and this upregulation may affect other folate transporter responses. Another major gap in knowledge is the lack of studies describing folates produced by microbes and mammalian folate transporters. A more accurate model of the intestine is needed to identify folate-related functions in response to microbial folate compounds.
Folate is essential for several metabolic processes including one-carbon transfer, methylation metabolism through the synthesis of SAM, and the synthesis of thymidylate, purines, and several amino acids (Baggott and Tamura, 2015; Brosnan et al., 2015). Once absorbed, folate also participates in nucleotide synthesis and replication, repair, and methylation of DNA. Given the important role of folates in cell maintenance, deficiency in folate has been linked to a large spectrum of health disorders including coronary heart disease, osteoporosis, Alzheimer’s disease, and colorectal cancer (LeBlanc et al., 2011, 2013a). Bacterial folate has been put forward as a potential nutrition source for treating or preventing low-folate conditions (Pompei et al., 2007b; Rossi et al., 2011). However, this effect has been hindered by the lack of characterization of folate-secreting intestinal bacterial strains.
In this study, the predicted folate synthesis capabilities of representative members of the human intestinal microbiome were evaluated to determine the patterns and prevalence of bacterial folate metabolism genes. Bacterial folate production was examined in a phylogenetically diverse set of intestinal microbes representing six key phyla of the human intestinal microbiome. Additionally, a more in-depth characterization of folate glutamylation by two key commensal microbes was examined by mass spectrometry. To connect microbe-derived folate metabolism with uptake by the host, we assessed folate-associated processes in human intestinal enteroids by RNAseq and identified the potential for microbial folate compounds and FPGs to alter the expression of folate transporters in human enterocytes.
All bacterial strains and growth conditions used in this study are described in Supplementary Table S1. Bifunctional dihydrofolate synthase/folylpolyglutamyl synthase type 2 (folC2) and bifunctional dihydrofolate synthase/folylpolyglutamyl synthase (folC) genes (GenBank NZ_ACGX02000001−007, HMPREF0536_11260, and HMPREF0536_10555, respectively) were inactivated in Lactobacillus reuteri ATCC PTA 6475 (L. reuteri 6475) by site-specific integration of plasmid pORI28 into the L. reuteri 6475 chromosome as described previously (Walter et al., 2005; Thomas et al., 2016). Dideoxy DNA sequencing was used to confirm site-specific insertional mutagenesis. L. reuteri 6475, L. reuteri 6475 folC2:pORI28 (referred to as folC2), and L. reuteri 6475 folC:pORI28 (referred to as folC) were cultured in MRS medium. Insertion mutants were cultured in the presence of 10 μg/mL erythromycin. Bifidobacterium longum subspecies infantis ATCC 15697 was also cultured in MRS medium. Prevotella copri CB7 DSM 18205 and Clostridium sporogenes DSM 795, ATCC 3584 were grown in brain–heart-infusion (BHI) broth supplemented with 2% yeast extract and 0.2% cysteine. Akkermansia muciniphila ATCC BAA-835 was cultured in BHI supplemented with 0.4% pig stomach mucin (Sigma Aldrich). Escherichia coli Nissle 1917 was cultured in Luria-Bertani (LB) broth. All cultures were grown in an Anaerobe Systems AS-580 anaerobic chamber supplied with a mixture of 10% CO2, 5% H2, and 85% N2. Following initial growth, cultures were subcultured into the appropriate fully defined medium. Bacterial media recipes and reagents for the fully defined media are labeled as LDM4 and CDMM and compiled in Supplementary Tables S2, S3, respectively.
For growth curves, cultures of L. reuteri 6475, L. reuteri 6475:folC2, and L. reuteri 6475:folC grown at exponential phase in MRS were used to inoculate the fully-defined medium LDM4 (OD600nm adjusted to 0.1) and incubated anaerobically at 37°C. Growth was examined by optical density (OD600nm) at time 0, 1, 3, 6, 9, 12, 24, and 48 h. Optical densities were examined at OD600nm on a Smartspec Plus Spectrophotometer (Bio-Rad Laboratories Inc.) and colony forming unit (CFU) counts were performed at 6, 24, and 48 h on MRS or MRS-ERM agar plates to examine growth.
To generate bacterial Conditioned media (CM) for cell treatment, L. reuteri 6475, L. reuteri 6475:folC2, and L. reuteri 6475:folC were grown at exponential phase in MRS and used to inoculate LDM4 (OD600nm adjusted to 0.1). Cultures were grown anaerobically at 37°C for 24 h (stationary phase). Following incubation, cells were pelleted by centrifugation (5,000 × g for 5 min) and the supernatant was treated with DTT (Sigma 10708984001) to release protein-bound folates, then filtered using Amicon Ultra-15 centrifugal filter units using the ultracel-3 membrane (Millipore UFC900324, Millipore, Bedford, MA, United States). The filtrate (<3 kDa) was lyophilized and resuspended in enteroid media to generate 25% CM in the appropriate cell culture medium. All CM was pH-adjusted to pH = 7.4.
Bacterial cultures were collected at exponential or stationary phases (strain-specific media and incubation duration in Supplementary Table S1). Cultures were centrifuged at 3,000 × g for 15 min at 4°C. Bacterial culture supernatants were reserved for folate quantification. The cell pellet was resuspended in STE buffer (100 mM NaCl, 10 mM Tris-HCl, pH 8.0, 1 mM Ethylenediaminetetraacetic acid (EDTA), pH 8.0) and centrifuged at 7,500 × g for 5 min at 4°C, and supernatants were removed. Bacterial cell pellets were resuspended in 100 μl of “Fol diluent,” included with the ARCHITECT Folate Assay (Abbott Laboratories, Abbott Park, IL, United States). One hundred microliters of culture supernatants was diluted in 100 μl of “Fol diluent.” The concentration of folate present in each sample was determined using the ARCHITECT Folate assay, a competitive immunoassay using direct chemiluminescence. Samples were treated with 0.1 M DTT for 5 min to release folate conjugates from binding proteins in the sample. Folate quantification was determined through a competitive binding assay utilizing an acridinium–folate-bound biotin–folate-binding protein. Binding was detected by the ARCHITECT i System using avidin-conjugated paramagnetic solid phase particles. The inter- and intra-assay variation of the assay was <10% and recovery of added folate was >90%.
Bacterial cultures were collected at exponential or stationary phases (strain-specific media and incubation duration in Supplementary Table S1). The cultures were centrifuged at 4,500 × g for 10 min at 4°C, and bacterial supernatants were removed. Bacterial cell pellets were washed with water and centrifuged at 6,000 × g for 10 min at room temperature. Folates were extracted from cell pellets by adding extraction buffer (0.1% TFA, 50% acetonitrile in water), vortexing, and centrifuging at 16,000 × g for 10 min at room temperature. The folate-containing supernatants were spotted in CHCA matrix (ProteoChem P9100, Hurricane, UT, United States) onto a MALDI target (SimuTOF Systems, Marlborough, MA, United States). A standard consisting of eight peptides ranging from 8 to 28 amino acids was spotted onto the MALDI target as calibrant. Spectra were acquired on a SimulTOF Combo 200 Mass Spectrometer (Virgin Instruments, Marlborough, MA, United States) in reflector mode and annotated with MoverZ software (Proteometrics, LLC, New York, NY, United States).
Distribution of the folate synthesis genes in the gastrointestinal reference genomes of the Human Microbiome Project (HMP) was determined using the Integrated Microbial Genomes and Metagenomes (IMG/M) (Markowitz et al., 2012) available through the Joint Genomes Institute (JGI). Version 5.0 (updated August of 2018) was accessed through https://img.jgi.doe.gov/ on November 14, 2018. The Profile and Alignment Tool (in JGI) was used to determine identities of folate biosynthesis genes present in the 512 gastrointestinal bacterial reference genomes of the HMP. Genes encoding enzymes involved in folate synthesis were designated by the enzyme commission (E.C.) number. The E.C. number for each enzyme involved in folate synthesis was queried against each genome using the Profile and Alignment Tool in JGI. Folate metabolic capacity was established on the basis of genes present in each genome.
Bacterial cells were fixed by adding an equal volume of cold methanol to exponential or stationary phase bacterial cultures. Fixed cells were pelleted at 16,000 × g for 1 min. RNA was isolated from bacterial cell pellets using the QuickRNA kit (Zymo Research, Irvine, CA, United States) according to the manufacturer’s instructions with modifications. Briefly, bacterial pellets were resuspended in 100 μl STE buffer (100 mM NaCl, 10 mM Tris–HCl, pH 8.0, 1 mM EDTA), and transferred to tubes containing 0.1 mm glass beads. Samples were disrupted on a FastPrep bead homogenizer (MP Biologicals) for 20 s at 4.0 m/s. Following disruption, Zymo Quick RNA lysis buffer was added to each glass bead containing tube, and the samples were disrupted on the FastPrep homogenizer for a second time. Cell debris and beads were removed by centrifugation at 10,000 × g for 1 min, and the supernatant was processed for RNA according to the manufacturer’s protocol. Genomic DNA removal was performed using the Ambion Turbo DNA-free kit following manufacturer instructions (ThermoFisher, Waltham, MA, United States). Quantitation and quality control (A260/A280 and 260/230 ratios) were performed with a Nanodrop OneC (ThermoFisher Scientific, Waltham, MA, United States) Spectrophotometer. Synthesis of cDNA from RNA was completed by reverse transcription using the SensiFast cDNA synthesis kit (Bioline, London, United Kingdom). For quantitative real-time PCR (qRT-PCR) analysis of bacterial folate genes, bacterial cDNA was examined using FAST SYBR green and primers (Supplementary Table S4) on a Stratagene 3005p qPCR System (ThermoFisher, Waltham, MA, United States). For relative quantitation, the ΔΔCt method was used and microbial mRNA quantities were normalized to amounts of 16S rRNA.
Differentiated human colonoid monolayers were generated as previously described (Zou et al., 2017; Rajan et al., 2018). The colonoid lines AsC109 and C103 were established from colonic tissue sampled via endoscopic-guided biopsy procedure from the ascending colon (Baylor College of Medicine, IRB # H-35094). Three-dimensional colonoids were cultured in complete medium with growth factors (CMGF+) as previously described (Sato et al., 2011; Saxena et al., 2016). Briefly, CMGF+ consisted of advanced Dulbecco’s modified Eagle medium (DMEM)/F-12 medium (Invitrogen) supplemented with 1× GlutaMAX (Invitrogen), 10 mM HEPES buffer (Invitrogen), and 100 U/mL penicillin–streptomycin (Invitrogen), with 50% (v/v) Wnt3A-conditioned medium, 20% (v/v) R-spondin conditioned medium, 10% (v/v) Noggin-conditioned medium, 1× N-2 supplement (Invitrogen), 1× B-27 supplement (Invitrogen), 1 mM N-acetylcysteine (Sigma–Aldrich), 50 ng/mL mouse epidermal growth factor (EGF) (Invitrogen), 10 nM Leu-Gastrin I (Sigma–Aldrich), 500 nM A-83-01 (Tocris Bioscience), 10 nM SB202190 (Sigma–Aldrich), and 10 mM nicotinamide (Sigma–Aldrich, St. Louis, MO, United States). Colonoids were passaged in phenol red-free, growth factor-reduced Matrigel (Corning).
Colonoids at passage 6 were seeded into flat 96-well plates as described previously (VanDussen et al., 2015; Ettayebi et al., 2016) to generate monolayers. Briefly, 96-well plates were pre-treated with Matrigel diluted in 1× phosphate-buffered saline (PBS) (1:40) and incubated at 37°C. The Matrigel was removed from 3D colonoids and the colonoids were washed with an ice-cold solution of 0.5 mM EDTA in 1× PBS and dissociated with 0.05% trypsin/0.5 mM EDTA at 37°C for 4 min. Following the incubation, the trypsin was inactivated with advanced DMEM/F12, 1× Glutamax, 1× HEPES + 10% fetal bovine serum. The cell solution was pipetted vigorously and filtered via a 40 μm nylon cell strainer (Falcon, Cat. No. 352340). The resulting single cells were centrifuged at 400 × g for 5 min, resuspended with CMGF+ and 10 μM Y-27632 Rock inhibitor, and plated into Matrigel-coated wells. After 48 h, the medium was changed to differentiation medium, which contains the same components as CMGF+ without Wnt3A conditioned medium, R-spondin conditioned medium, SB202190, and nicotinamide and only 5% (v/v) Noggin conditioned medium, with the addition of 10 μM Y-27632 Rock inhibitor. Differentiation medium with Y-27632 was changed daily for 4–5 days to differentiate cells.
For bacterial supernatant treatment, the differentiation media on colonoid monolayers on 96-well plates was changed to DMEM without folate supplemented with 1× HEPES, 1× Glutamax, and 1× pyruvate. Colonoids were treated for 16 h with either media alone, 25% CM from uninoculated LDM4, wild-type L. reuteri 6475, L. reuteri 6475:folC (gene required for pteroate glutamylation), or L. reuteri 6475:folC2 (gene required for THF synthesis) (Thomas et al., 2016) in the minimal medium. Following treatment, 60 μM resazurin was added to the media and metabolic activity was examined by a change in fluorescence (excitation 560 nm: emission: 600 nm) after 2 h of incubation. Next the monolayers were gently dissociated with PBS (Ca2+, Mg2+ free) containing 3 mM EDTA, 10 mM glucose, and 10 μl cell solution was mixed with 10 μl trypan blue to assess viability. Cell counts were determined using a Countess Automated Cell Counter (Invitrogen, Carlsbad, CA, United States) [n = 2 biological replicates (enteroid lines), three technical replicates].
To assess the transcriptional profile of human intestinal enteroids, sterile monolayers were prepared on transwells and differentiated as previously described (Zou et al., 2017). RNA was extracted via TRIZOL according to the manufacturer’s instructions and rRNA integrity was checked on a 2100 Bioanalyzer (Agilent, Santa Clara, CA, United States) from two independent biological replicates per segment. Using mRNA enriched samples by Novogene, paired-end Illumina sequencing was performed following the standard work-flow procedure. Raw sequence reads were mapped to human genome hg19 using STAR software to generate the number of fragments per kilobase per million mapped reads (FPKM) for each gene (n = 2 biological replicates).
Colonoid RNA was extracted via TRIZOL according to the manufacturer’s instructions and genomic DNA removal was performed using the Ambion Turbo DNA-free kit (ThermoFisher, Waltham, MA, United States). Quantitation and quality control (A260/A280 and 260/230 ratios) were performed with a Nanodrop OneC (ThermoFisher Scientific, Waltham, MA, United States) Spectrophotometer. cDNA was synthesized from RNA by reverse transcription using the SensiFast cDNA synthesis kit (Bioline, London, United Kingdom). Expression of key folate-related genes was analyzed using qRT-PCR. The reaction was performed with colonoid cDNA and FAST SYBR green master mix (Applied Biosystems, Foster City, CA, United States) per manufacturer instructions. The following amplification conditions were used: after an initial denaturation at 95°C for 20 s, 40 amplification cycles were performed at 95°C for 3 s and 60°C for 30 s on a Stratagene 3005p qPCR System (ThermoFisher, Waltham, MA, United States). Primers used in this study are listed in Supplementary Table S4. The ΔΔCt method was used for relative quantification and mRNA levels were normalized to human 18S.
Statistical analyses were performed using GraphPad Prism version 198 5.04 (GraphPad Software, San Diego, CA, United States). All data were normally distributed and examined using non-parametric tests. All data were analyzed by one-way ANOVA with a Bonferroni post hoc test. Statistical test results are presented as mean ± standard deviation (SD). P-values are indicated by asterisks as follows: ∗p < 0.05.
The folate metabolic capacity of 512 well-defined, phylogenetically diverse reference bacterial genomes from the human gastrointestinal tract was evaluated. Bacterial genomes were binned as potential contributors of folate biosynthesis if the genome contained at least one set of genes representing folate biosynthetic metabolic pathway. Pathways include genes required for synthesis of (1) chorismate from phosphoenolpyruvate (seven enzymatic functions), (2) pABA from chorismate (two enzymatic functions), (3) dihydropterin pyrophosphate from GTP (four enzymatic functions), and (4) THF from pABA and dihydropterin pyrophosphate intermediates (four enzymatic functions) (Figure 1A). A summary of the relative capacity of these gut microbes to synthesize specific folate intermediates is illustrated in a stacked graph stratified by phylum (Figure 1B).
Figure 1. Distribution of folate synthesis genes in the gastrointestinal human microbiome. (A) Schematic of de novo folate synthesis divided into four modules: (1) chorismate synthesis, (2) pABA synthesis, (3) pterin synthesis, and (4) folate synthesis. Each protein is listed by name and enzyme commission (E.C.) number. (B) Distribution of genomic folate synthesis capabilities within the Human Microbiome Project’s Gastrointestinal Reference Genomes stratified by phylum.
Chorismate acts as an intermediate for diverse metabolic pathways including folate and amino acid biosynthesis and the genes required to synthesize chorismate from the glycolysis product, phosphoenolpyruvate, were found in 256 genomes (Figure 1B). These genomes represented all key phyla except Bacteroidetes. We found 134 genomes encode the capacity to convert chorismate to pABA including microbes from the phyla Proteobacteria, Firmicutes, Actinobacteria, and Fusobacteria. A total of 368 genomes, 72% of the reference genomes, representing six key intestinal phyla encode the capacity of synthesizing dihydropterin pyrophosphate from GTP (Pterin Synthesis). Genes involved in the synthesis of THF from pABA and dihydropterin pyrophosphate (folate synthesis) were found in 291 (57%) of the bacterial genomes representing six key phyla of the human intestinal microbiome, Firmicutes, Bacteroidetes, Actinobacteria, Fusobacteria, Proteobacteria, and Verrucomicrobia (Figure 1B). A total of 392 (77%) bacterial genomes contain genes capable of synthesizing THF if pABA is present as a precursor (Figure 1B). We also found that 267 genomes had the capacity for both pterin and folate synthesis. Finally, we found that 68 genomes were capable of using chorismate to synthesize folates completely de novo (Figure 1B). The phyla Firmicutes and Proteobacteria exhibited the highest number of genomes capable of de novo folate synthesis. This dataset indicates that the majority of microbes must obtain precursors in the form of dietary nutrients or microbial cross-feeding in order to produce luminal folate in the intestine.
The biochemical functionality of bacterial folate biosynthesis genes identified in situ was verified in a cohort of 10 bacterial strains representing six key phyla of the human intestinal microbiome. These microbes included three strains which are currently available as probiotics (L. reuteri ATCC PTA 6475, Enterococcus faecalis Symbioflor DSM 16431, and E. coli Nissle 1917) (Gronbach et al., 2010; Mu et al., 2018; Baccouri et al., 2019). Figure 2 depicts the genetic capacity of 10 bacterial strains to synthesize folate de novo. Circle shading corresponds to genetic capacity; with completely shaded circles representing complete gene sets for the indicated pathways and empty circle indicating no genes present (Figure 2). From this representative cohort, only two strains (B. longum subsp. infantis ATCC 15697 and E. coli Nissle 1917) representing two phyla (Actinobacteria and Proteobacteria) contain complete pathways for generating folate de novo. In the phylum Firmicutes, three of four strains (C. sporogenes DSM 795, E. faecalis Symbioflor DSM 16431, and L. reuteri ATCC PTA 6475) are completely devoid of genes necessary to synthesize pABA and hence their ability to synthesize folate depends on the presence of pABA in their environmental milieu. This is consistent with previous work demonstrating the requirement of pABA for L. reuteri folate-production (Spinler et al., 2014; Thomas et al., 2016).
Figure 2. In silico analysis of folate synthesis gene distribution in an intestinal microbe cohort. The Joint Genomes Institute (JGI) Profile and Alignment Tool was used to predict chorismate synthesis genes, pABA synthesis genes, pterin synthesis genes, and folate synthesis genes in a cohort of 10 strains representing six phyla of the human intestinal microbiome. The circles are filled in proportion to the number of those genes contained in the genome of each bacterial strain. Circles 90% filled represent one missing gene while circle 75% filled represent two missing genes.
To verify our in situ findings, we characterized folate production by one strain representing each of the six major intestinal phyla: B. longum subsp. infantis, C. sporogenes, L. reuteri, E. coli Nissle, A. muciniphila, and P. copri. We cultured each strain to exponential and stationary phase growth since bacterial metabolism differs significantly between growth phases (Rolfe et al., 2012; Robador et al., 2018; Supplementary Table S1). We collected supernatants and cell pellets and analyzed folate concentrations by competitive immunoassay. The intermediate pABA was included in all defined medias (LDM4 and CDMM). We found that in exponential growth phase, B. longum subsp. infantis (123.3 ± 11.2 ng/ml), L. reuteri (62.6 ± 1.8 ng/ml), and A. muciniphila (64.4 ± 0.15 ng/ml) produced net amounts of folate, while C. sporogenes, E. coli Nissle, and P. copri were folate consumers (Table 1). However, in stationary phase the majority of bacteria were net folate producers, with B. longum infantis generating the highest concentration (261.1 ± 0.15 ng/ml). Based on net production or consumption of folate, representative intestinal microbes can be divided into three functional groups: folate consumers, folate producers, and conditional producer/consumer. Regardless of growth phase, P. copri DSM 18205 is a net folate consumer and B. longum subsp. infantis ATCC 15697 is a net folate producer. E. coli Nissle 1917, on the other hand, is a conditional producer, consuming folate in exponential phase and producing folate during stationary phase (Table 1). This in vitro data indicate that several intestinal species are capable of generating folate.
We examined two members of the gut microbiota, E. coli and L. reuteri, to assess expression of folate genes and the ability to polyglutamylate folate. Consistent with folate production (Table 1), qPCR analysis of key folate genes indicated increased expression in the exponential phase for E. coli Nissle 1917 (Figure 3A). Genes such as folK, the final enzyme required for pterin synthesis, were upregulated in E. coli Nissle 1917 exponential phase compared to stationary phase. Similar to E. coli Nissle 1917, folK was also upregulated in L. reuteri ATCC 6475 during exponential phase. Additionally folP, the enzyme which combines the pterin and pABA intermediates was significantly upregulated in exponential phase in L. reuteri ATCC 6475. Next, we examined glutamylation profiles produced by these strains. The folates synthesized by E. coli Nissle 1917 contain relatively constant glutamate tails (3–5 glutamate residues) and do not lengthen with increased time of incubation (Figure 3C). In contrast, folates synthesized by L. reuteri 6475 produce relatively long glutamate tails (FPGs; chain length 7–9 glutamate residues), which increase in length by adding glutamate residues over time (Figure 3D). In addition, to the simple 5,10-methenyl THF (tetrahydrofolic acid) polyglutamate polymers produced by E. coli Nissle 1917, L. reuteri 6475 produces both 5,10-methenyl THF polyglutamates and a unique 5,10−ethenyl THF with a covalently linked γ-polyglutamate tail (Roth et al., 2019). 5,10-Ethenyl THF carries two carbons at the “business end” of THF, between N5 and N10. It contributes to a two-carbon variant of the folate cycle that leads to the production of ethionine instead of methionine (Roth et al., 2019). These data are among the first to identify commensal gut microbes with unique patterns of glutamylated folate, which may have an impact on host folate processes.
Figure 3. Folate synthesis during exponential and stationary growth phase by qPCR and MALDI-TOF mass spectrometry. Expression of folate synthesis genes at exponential and stationary phase growth in (A) Escherichia coli Nissle 1917 and (B) Lactobacillus reuteri ATCC PTA 6475 as determined by qPCR. aroC is involved in chorismate synthesis; pabB in pABA synthesis; folP, folC, and folA in pterin and folate synthesis. Expression is normalized to 16S rRNA. One-way ANOVA with Bonferroni post hoc test. ∗p < 0.05 (n = 3 biological replicates). Differential glutamylation profiles of folates determined by MALDI-TOF mass spectrometry for (C) E. coli Nissle 1917 and (D) L. reuteri ATCC PTA 6475. L. reuteri produces both the 5,10-methenyl THF as well as the 5,10-ethenyl THF form with + 14 glutamate (GLU) residues. Blue boxes highlight the peaks which represents MTHF + nGLU.
The novel human enteroid model has transformed the study of intestinal epithelial cells. Enteroids derived from intestinal stem cells represent non-transformed, multicellular epithelial cultures that can express all the native differentiated intestinal cell types (Sato et al., 2009, 2011; Clevers, 2016; Saxena et al., 2016; Zou et al., 2017). Enteroids appear to recapitulate human intestinal physiology, making them an ideal model to examine folate-associated processes (Foulke-Abel et al., 2016; Saxena et al., 2016; Zou et al., 2017). Moreover, human enteroids grown as 2D monolayers represent an optimal tool for examining host–microbe interactions. Using RNAseq, we identified several folate-related genes in human enteroids derived from duodenum, jejunum, ileum, and colon (Figures 4A–E). High expression was observed in genes associated with nucleotide synthesis, methylation, one-carbon cycle, and folate transporters (Figures 4B–E). The most highly expressed genes included methylenetetrahydrofolate reductase (MTHFR), folylpolyglutamate synthase (FPGS), 5-methyltetrahydrofolate-homocysteine methyltransferase reductase (MTRR), PCFT (SLC46A1) and methylenetetrahydrofolate dehydrogenase (NADP + Dependent) 2 (MTHFD2), methenyltetrahydrofolate cyclohydrolase, and folate hydrolysis (FOLH) (Figure 4A). These genes represent unique host pathways involved in folate homeostasis. Interestingly, of these highly expressed genes, robust expression was observed in the small intestine (duodenum, jejunum, and ileum) and to a slightly lesser degree in the colon. FOLH is responsible for converting FPG to mono-glutamate which can then be absorbed by mammalian cells and FOLH1 expression was high throughout all intestinal segments, including the colon (35.7 ± 12.5 FPKM). Since the colon is the primary site of microbe-produced folate absorption (Pompei et al., 2007a; Aufreiter et al., 2009; Lakoff et al., 2014), we chose to focus our attention on the colon.
Figure 4. RNAseq expression of folate-associated genes in human enteroids. Enteroids derived from diverse intestinal segments were examined by RNAseq. (A) Heat map of the expression of folate-associated genes in duplicate biological samples from human duodenum, jejunum, ileum, and colon enteroids. Expression is indicated from high (dark blue) to low (white). The data were divided into four groups based on gene expression: nucleotide synthesis (B), methylation (C), one-carbon cycle (D), and folate transporters (E) (n = 2 biological replicates).
We modeled host–microbe interactions by examining folate receptor/transporter gene expression in response to folate generated by L. reuteri 6475. We also included two L. reuteri 6475 mutants which harbored insertions in either the folC gene (6475:folC) or the folC2 gene (6475:folC2). Our previous study showed that 6475:folC lacks the ability to produce a polyglutmate tail on 5,10-methenyl THF and that 6475:folC2 does not produce any 5,10-methenyl THF (Thomas et al., 2016). We confirmed that these mutants exhibit normal growth in LDM4 by optical density (OD600nm) and CFU over 48 h (Supplementary Figures S1A,B). The CFU counts of all L. reuteri strains (WT, 6475:folC, or 6475:folC2) after 24 h of growth mirror the concentrations of L. reuteri observed in patients (108) (Million et al., 2013) and concentrations that are well tolerated and safe in humans (Mu et al., 2018). As a result, 24 h was selected for further analysis. By mass spectrometry, we quantified the folate produced by our L. reuteri strains after 24 h growth (Supplementary Figure S1C). Consistent with our previous work, we demonstrate that the 6475:folC mutant is capable of producing glutamylated folate (170 ± 25.6 ng/ml), while the 6475:folC2 mutant is unable to produce folate above the LDM4 media control (16.7 ± 1.2 ng/ml) (Supplementary Figures S1D,E).
Using wild-type L. reuteri 6475 and insertion mutants 6475:folC and 6475:folC2 as a model for microbe folate production, the relative abilities of microbial-derived folates to modulate human folate receptor gene expression could be examined. Two human colonoid lines were used for all analyses: Asc109 and C103. Colonoid monolayers were treated with DMEM containing no folate in the presence of 25% LDM4 or 25% L. reuteri (WT, 6475:folC, or 6475:folC2) LDM4 CM overnight (16 h). After treatment, the colonoid monolayers maintained their intestinal architecture as observed by light microscopy (data not shown). To confirm the viability of the enteroid monolayers following treatment, we assessed viability by trypan blue staining (Figures 5A,E) and metabolic activity by resazurin assay (Figures 5B,F). Consistent with the microscopic images, we observed no changes in viability or metabolic activity in colonoids treated with either L. reuteri strain. Analysis of mRNA levels of the PCFT (Figures 5C,G) and reduced folate transporter (RFC) (Figures 5D,H) by qPCR revealed unique patterns in response to our bacterial supernatant. Application of bacterial supernatants had no effect on the levels of the PCFT in either colonoid line (Figures 5C,G). However, the application of bacterial supernatants containing a non-glutamylated folate (L. reuteri 6475:folC-mutant strain) to human colonoids yielded enhanced expression of the folate transporter RFC (Figures 5D,H). This effect was not observed with the L. reuteri 6475:folC2-mutant strain which is unable to synthesize folate, pointing to the potential role of non-glutamylated folate in modulating RFC levels. In addition to receptor levels, other folate-related genes were queried (Supplementary Figure S2). No changes were observed in the GCPII (folh1), which is required to deconjugate polyglutamylated folates, with any of the treatment conditions (Supplementary Figures S2A,B). Likewise, no significant changes were observed in any MTHFD genes (Supplementary Figures S2C–H) or serine hydroxymethyltransferase 2 (SMTH2) (data not shown). Together these data demonstrated that bacteria in the human gastrointestinal microbiome produce bioavailable folates and that the glutamylation status of microbial folate can influence folate transporter expression in the human intestine (Figure 6).
Figure 5. Expression of folate transporters in human colonoids treated with L. reuteri conditioned media. Human colon monolayers (lines Asc109 and C103) were incubated with 25% uninoculated bacterial medium (LDM4) or 25% L. reuteri ATCC 6475 conditioned media for 16 h. In addition to WT L. reuteri, the 6475:folC mutant, which can produce THF, but can’t synthesize a polyglutamate tail, and the 6475:folC2 mutant, which can’t synthesis any THF, were included in the analysis. Viability assessment of colonoids following 16 h incubation as determined by (A,E) trypan blue staining and (B,F) resazurin assay. Expression of proton-coupled folate transporter, PCFT (C,G) and reduced folate carrier, RFC (D,H) determined by qPCR normalized to 18S. One-way ANOVA with Bonferroni post hoc test. ∗p < 0.05 (n = 2 biological replicates, three technical replicates).
Figure 6. Model of microbial folate production and microbe:host interactions. Polyglutamylated tetrahydrofolates are synthesized by the human intestinal microbiome. Human gamma-glutamylhydrolase, GGH, and gamma-glutamyltransferase, GGT, remove glutamate moieties making monoglutamylated folates available for uptake by other luminal bacteria or human colonocytes. Folate can activate folate receptors (Frα or Frβ) or be taken up into enterocytes by folate transporters (RFC or PCFT).
Our study has demonstrated that folate biosynthesis is a major biochemical feature of the human intestinal microbiome. Four basic metabolic modules in folate biosynthesis have been defined, and described in terms of prevalence of these separate modules in the reference set of 512 microbial genomes attributed to the human gastrointestinal tract. These modules include chorismate, pABA, pterin, and folate synthesis. Our data demonstrate that these genes are distributed among the six phyla of the human microbiome (Actinobacteria, Bacteroidetes, Firmicutes, Proteobacteria, Fusobacteria, and Verrucomicrobia). However, only strains from Proteobacteria and to a lesser degree Firmicutes, Actinobacteria, and Verrucomicrobia (68 strains in total) are able to completely generate folate de novo. This study provides insight into the genetics of the human intestinal microbiome and suggests a cooperative role of microbial communities to generate B complex vitamins in the human host. Our studies revealed different glutamylation profiles for commensal gut microbes that may affect folate bioavailability in the intestine. We specifically showed that L. reuteri and E. coli Nissle generate moderate amounts of folate (49–77 ng/μl) and differ in the extent of polyglutamylation. Finally, our data showed that in the absence of bacterial THFs, folate transporter expression in human colonic enterocytes is significantly increased. This work has advanced the scientific understanding of bacterial folate metabolism and expanded the potential role for the microbiome in treating vitamin deficiencies.
Folate deficiency contributes to serious health problems and can result from insufficient dietary intake, poor absorption of ingested folate, and altered folate metabolism due to genetic defects or drug interactions (Rajagopalan et al., 2002; Hiraoka and Kagawa, 2017). In the absence of a genetic inability to absorb folate, folate deficiency can be treated through dietary changes, increasing consumption of dark green vegetables, beans, liver, and folate-enriched foods. Long-term correction of low folate through the diet has yielded mixed results (Menon et al., 2005; Kemperman et al., 2006; Nair and Iyengar, 2009; Kaehler et al., 2012). Folate deficiency can also be treated by supplementation with folic acid, a synthetic, fully oxidized, mono-glutamylated form of folate. In contrast to the synthetic folic acid, naturally occurring dietary and microbe-produced folates are polyglutamylated, fully reduced THFs with methyl, methylene, or formyl groups. THF is the form of folate that can be processed through the one-carbon cycle, making this form a more desirable format for absorption. As a result, we speculate that the delivery of microbial folates may be amenable for the treatment of low folate. Microbe-derived folate is particularly attractive for patients with inactivating single-nucleotide polymorphisms (SNPs) in dihydrofolate reductase (DHFR), which is required to convert folic acid to THF. These patients are unable to efficiently absorb synthetic folates (Askari and Krajinovic, 2010; Hiraoka and Kagawa, 2017) and thus need alternative sources of THFs. By delineating the mechanisms regulating the production and secretion of folate by the microbiome, manufacturers, physicians, and researchers can make informed decisions regarding probiotic recommendations and nutritional counseling based on human microbiome science.
We found that 13.3% of 512 bacterial reference genomes from the human intestinal microbiome contain the genes spanning all four metabolic modules involved in de novo synthesis of THF. This sizeable fraction of intestinal microbes containing complete sets of folate biosynthetic genes suggests that the microbiome serves as an important folate-generating “organ.” The remaining genomes (>86%) require folate or folate intermediates from other bacteria of the human microbiome or the human diet. Therefore, when proposing a potential probiotic source of folate, either complete capacity to generate folates or effective combinations of folate producing strains (combining a pABA-producing strain with a pABA-dependent strain) should be considered. Our data are consistent with previous genomic studies that predicted that 26% of Actinobacteria, 79% of Fusobacteria, 71% of Proteobacteria, and 15% of Firmicutes in the human intestinal microbiome are fully capable of synthesizing folate de novo (Magnusdottir et al., 2015). Magnusdottir et al. (2015) suggested that the human microbiome was potentially capable of synthesizing 37% of the daily recommended intake of folate for non-pregnant, non-breastfeeding adults. This recommendation highlights the potential importance of the gut microbiome as a source of folate production. We have identified strains including B. ovatus ATCC 8483 and A. muciniphila ATCC BAA 835 that contain the complete complement of 16 enzyme functions needed to synthesize folates. These strains have not historically been considered probiotics and as a result remain uncharacterized and may have undesired effects. Therefore, it is advantageous to utilize a well-characterized probiotic strains for folate delivery. The human breast milk-derived strain L. reuteri ATCC PTA 6475 has been used in clinical trials (NCT02422082, NCT00774163, NCT03360253) and is known to be safe to animals and humans (Adams and Marteau, 1995; McCabe et al., 2013; Britton et al., 2014; Fatheree et al., 2017; Galofre et al., 2018; McCabe and Parameswaran, 2018; Schepper et al., 2019). The genome of L. reuteri ATCC PTA 6475 lacks genes needed to synthesize chorismate or pABA, and depends on folate precursors. As a result, we propose addition of L. reuteri and pABA to harness the full folate producing potential of L. reuteri.
Knowledge of folate-related genes in the intestine has been limited by the lack of clinically relevant systems. The majority of existing work has been performed in rodent models and cancer-derived cell lines. Work using PCFT-deficient mice has identified that the presence of PCFT is a requirement for the transport of folates across the apical brush-border membrane of the proximal small intestine (Wang et al., 2013; Visentin et al., 2014). In immortalized rat small intestinal epithelial IEC-6 cells, PCFT was also found to facilitate entry of folate at low pH, while RFC enabled folate absorption at neutral pH (Rajgopal et al., 2001). Both these studies focused on the small intestine and did not examine the colon, which is the primary site for microbe-produced folate absorption (Pompei et al., 2007a; Aufreiter et al., 2009; Lakoff et al., 2014). Although PCFT is present in the colonic mucosa, the extent of colonic folate absorption in vivo is not known. Rodents are the experimental tool of choice for the majority of intestinal absorption studies and these types of studies have yielded tremendous insight into intestinal homeostasis. Nevertheless, significant differences between mice and humans may affect our knowledge of folate transport (Mestas and Hughes, 2004). Lakoff et al. (2014) verified that humans can absorb folates through the colon using a pH-sensitive capsule for targeted delivery. However, this study did not evaluate the composition of the human microbiome in their subjects or the cumulative effects of microbial metabolites on folate absorption as it used a single defined monoglutamylated folate. Our work is the first to identify folate-related processes in epithelial cells within different intestinal regions based on human intestinal enteroids. Human intestinal enteroids yield a model of intestinal epithelium exhibiting a similar cellular composition and function as the intact epithelium of the human intestine (Sato et al., 2011; Sato and Clevers, 2013; Foulke-Abel et al., 2014, 2016; Middendorp et al., 2014; Saxena et al., 2016; Zachos et al., 2016). Additionally, enteroids exhibit region-specific attributes of the human gastrointestinal epithelium (Middendorp et al., 2014; Saxena et al., 2016) and are non-transformed cells derived from intestinal stem cells. Enteroids have been used to examine host–microbe and host–viral interactions (Finkbeiner et al., 2012; Engevik et al., 2013; Yin et al., 2015; Saxena et al., 2016, 2017; Zou et al., 2017; Blutt et al., 2018; Rajan et al., 2018; Yin and Zhou, 2018). To the best of our knowledge, we are the first to identify folate genes in enteroids from diverse regions of the intestine. This work suggests that although the colon expresses lower levels of the major genes involved in nucleotide synthesis, methylation, one-carbon cycle, and folate transporters compared to the small intestine, it still expresses high levels of several genes including MTHFR, DHRF, MTRR, FPGS, PCFT, and RFC. Dietary FPGs are hydrolyzed to folylmonoglutamate derivatives prior to absorption by the intestinal epithelial cells. Of particular interest, the colonoids express high levels of folate hydrolase (FOLH) and γ-glutamyl hydrolase (GGH), enzymes found of the brush border and in the lysosome of the intestinal epithelial cells (Chave et al., 2003). These enzymes participate in the dietary FOLH. Additionally, only the colon expressed folate hydrolase 2 (FOLH1B), another hydrolase known to convert FPGs to folylmonoglutamates. These findings indicate that folate absorption by the human colon may significantly contribute to net folate absorption.
This study extends our knowledge of intestinal folate metabolism by using human enteroids to examine microbial folate–host interactions. Our work indicates that bacterial supernatants containing non-polyglutamylated THFs (L. reuteri 6475:folC-mutant strain) results in upregulation of the RFC transporter. Both PCFT and RFC are highly specific for the monoglutamate form of folate (Visentin et al., 2014). We speculate that in our enteroid model, the GCPII (folh1) cleaves the glutamate moieties to provide entrance of the L. reuteri folate polyglutamates into the cell. Interesting, we did not observe any changes in folh1 expression in our colonoids following bacterial supernatant treatment. Our data indicate that WT L. reuteri, producing a glutamylated THF and L. reuteri 6475:folC2, which produces no folate, had no effect on RFC levels in human colonoids. However, we do demonstrate that L. reuteri 6475:folC, which makes a non-glutamylated folate upregulates RFC. We reason that WT L. reuteri’s glutamylated folate requires extensive deconjugation by Folh1 before absorption occurs. This enzymatic deconjugation might limit the bioavailability of L. reuteri’s folate, thereby limiting the concentration of folate available to influence RFC levels. Since THF can be absorbed by intestinal cells (Bhandari and Gregory, 1992), we predict that the non-glutamylated folate produced by L. reuteri 6475:folC is taken up more rapidly and may signal to the host to upregulate the RFC receptor. Consistent with this hypothesis, the L. reuteri 6475:folC2 mutant, which is unable to produce any folate, has no effect on RFC levels. This finding indicates that L. reuteri does not secrete folate-independent factors that upregulate RFC levels. Based on previously published microarray data (GSE32971), 47 of the 13,580 genes examined were downregulated in the 6475:folC mutant compared to WT L. reuteri (Thomas et al., 2016). These genes included several downstream folate-related genes, including folK and folB. Additionally 64 genes were upregulated in 6475:folC-mutant compared to WT L. reuteri (Thomas et al., 2016). Overall, 0.08% (111/13,580) of the L. reuteri genes were altered with the site-specific insertion in folC. We believe that this good evidence that loss of folC is not significantly influencing L. reuteri’s metabolism or metabolite profile. Additional experiments using a complemented folC in L. reuteri in the future would help address these questions. While we hypothesize that the glutamate status of folate influences RFC levels, it is also possible that increased RFC levels may reflect an attempt by the host to increase intracellular folate due to the lack of folate glutamylation and perhaps uptake. Currently we do not have data on microbial folate entry into the enteroid cultures. However, we believe that in the future, this enteroid culture system may serve as a valuable tool to address folate uptake questions.
Of note, cancer-derived Caco-2 cells and immortalized human colon cell line NCM 460 have demonstrated that PCFT is the primary transporter involved in intestinal folate absorption and this absorption occurs at low pH (Kumar et al., 1997; Qiu et al., 2006). While the pH of the distal colon systemically may not be favorable to folate absorption at a pH ∼7 (Evans et al., 1988; McDougall et al., 1993; Engevik et al., 2015), L. reuteri is known to adhere to the intestinal mucus layer directly above the epithelium and produce lactic acid which can lower the local pH (Mackenzie et al., 2010; Jensen et al., 2014). In this way, L. reuteri may drive additionally folate absorption in a local manner. Additionally, the cecum has a reduced pH due to the relative abundance of lactic acid bacteria and may also serve as a fertile area of folate assimilation. In our colonoid experiments, we neutralized our L. reuteri CM (pH = 7.0) to ensure that we did not affect our epithelial viability. In the future, varying pH parameters should be considered using the enteroid culture system.
Collectively our data indicate that the intestinal microbiome can participate in luminal folate production and subsequent modulation of folate transporter biology in the mammalian host. Selection of “smart” combinations of probiotics may provide supplemental sources of B complex vitamins such as folates, and future nutritional counseling may combine microbiome data and dietary information to guide individuals so that vitamin deficiency states are prevented. Optimizing microbial metabolism and vitamin sources in the gut microbiome may enhance human health by enhancing cellular functions and intestinal homeostasis.
The raw data supporting the conclusions of this manuscript will be made available by the authors upon request.
This study (protocol H-35094) was carried out in accordance with the policies of, and approved by, the Baylor College of Medicine Institutional Review Board with written informed consent from all subjects.
MAE, CM, and JV conceived and designed the research, and drafted the manuscript. MAE, CM, DR, KE, SD, JS, and SC performed the experiments. MAE, CM, DR, KE, and JS analyzed the data. MAE, CM, and DR prepared the figures. MAE, CM, DR, KE, SD, JS, SC, MKE, MK, and JV edited and revised the manuscript. JV approved the final version of the manuscript.
This study was supported by grants from the National Institute of Diabetes and Digestive and Kidney Diseases (Grant P30-DK-56338 to Texas Medical Center – Digestive Disease Center, Gastrointestinal Experimental Model Systems), the National Cancer Institute (Grant U01-CA-170930 to JV), and unrestricted research support from the BioGaia AB (to JV). The Mass Spectrometry & Proteomics Core Facility of the City of Hope was supported in parts by the National Cancer Institute of the National Institutes of Health under award number P30CA033572.
JV serves on the scientific advisory boards of Biomica, Plexus Worldwide, and Seed Health, and he also receives unrestricted research support from BioGaia, AB.
The remaining authors declare that the research was conducted in the absence of any commercial or financial relationships that could be construed as a potential conflict of interest.
We would like to thank Dr. Xi-Lei Zeng for expertise and preparation of the enteroid monolayers used in this study.
The Supplementary Material for this article can be found online at: https://www.frontiersin.org/articles/10.3389/fmicb.2019.02305/full#supplementary-material
FIGURE S1 | Lactobacillus reuteri strains (wild-type, 6475:folC, and 6475:folC2) exhibit normal growth, but varying degrees of folate production. (A) Diagram of the genes required to convert 7,8-dihydropteroate to 5,10-methenyl-THF (tetrahydrofolate). Key genes include folC (purple) and folC2 (light blue). (B) Growth of WT L. reuteri and two L. reuteri mutants, 6475:folC which can produce folate, but cannot add a glutamate tail, and 6475:folC2, which cannot produce folate, in LDM4 over 48 h. Samples were collected at 1, 3, 6, 12, 16, 24, and 48 h. (C) Colony forming units (CFUs) of L. reuteri strains at time 6, 24, and 48 h post-inoculation in LDM4. (D) Folate quantification in uninoculated LDM4 or WT L. reuteri, 6475:folC and 6475:folC2 LDM4 conditioned media after 24 h incubation by competitive immunoassay. (E) Glutamylation profile of folates produced by L. reuteri 6475:folC determined by MALDI-TOF mass spectrometry. The diagram depicts no clear glutamate peaks for 6475:folC folate, indicative of no polyglutamate residues. ∗p < 0.05 (n = 3 biological replicates, 3 technical replicates).
FIGURE S2 | Expression of downstream folate targets in human colonoids treated with L. reuteri conditioned media. Human colon monolayers (lines Asc109 and C103) were incubated with 25% uninoculated bacterial medium (LDM4) or 25% L. reuteri ATCC 6475 conditioned media for 16 h. In addition to WT L. reuteri, the 6475:folC mutant, which can produce THF, but can’t synthesize a polyglutamate tail, and the 6475:folC2 mutant, which can’t synthesis any THF, were included in the analysis. Examination of mRNA levels (A,E) folh1, required for removing folate glutamates and (B–H) methylenetetrahydrofolate dehydrogenase, required for intracellular folate processing, by qPCR revealed no changes between groups. All qPCR data were normalized to 18S. One-way ANOVA with Bonferroni post hoc test (n = 2 biological replicates, 3 technical replicates).
TABLE S1 | Bacterial strains, growth media, and exponential and stationary phase time points. aLDM4 supplement: 0.1 mg/L vitamin K and 5 mg/L hemin.
TABLE S2 | Lactobacillus defined medium 4 (LDM4) recipe.
TABLE S3 | Chemically defined minimal media (CDMM) recipe.
TABLE S4 | Bacterial folate synthesis qRT-PCR primers.
CHCA, α-cyano -4-hydroxycinnamic acid; CM, conditioned media; DTT, dithiothreitol; folC2, folylpolyglutamate 2 gene; FPG, folylpolyglutamates; GCPII, glutamate carboxypeptidase II; GTP, guanosine triphosphate; pABA, para-aminobenzoic acid; PCFT, proton-coupled folate transporter; RFC, reduced folate carrier; SAM, S-adenosylmethionine; SCFAs, short chain fatty acids; THF, tetrahydrofolate.
Adams, M. R., and Marteau, P. (1995). On the safety of lactic acid bacteria from food. Int. J. Food Microbiol. 27, 263–264.
Askari, B. S., and Krajinovic, M. (2010). Dihydrofolate reductase gene variations in susceptibility to disease and treatment outcomes. Curr. Genomics 11, 578–583. doi: 10.2174/138920210793360925
Asrar, F. M., and O’Connor, D. L. (2005). Bacterially synthesized folate and supplemental folic acid are absorbed across the large intestine of piglets. J. Nutr. Biochem. 16, 587–593. doi: 10.1016/j.jnutbio.2005.02.006
Aufreiter, S., Gregory, J. F. III, Pfeiffer, C. M., Fazili, Z., Kim, Y. I., Marcon, N., et al. (2009). Folate is absorbed across the colon of adults: evidence from cecal infusion of (13)C-labeled [6S]-5-formyltetrahydrofolic acid. Am. J. Clin. Nutr. 90, 116–123. doi: 10.3945/ajcn.2008.27345
Baccouri, O., Boukerb, A. M., Farhat, L. B., Zebre, A., Zimmermann, K., Domann, E., et al. (2019). Probiotic potential and safety evaluation of Enterococcus faecalis OB14 and OB15, isolated from traditional tunisian testouri cheese and rigouta, using physiological and genomic analysis. Front. Microbiol. 10:881. doi: 10.3389/fmicb.2019.00881
Baggott, J. E., and Tamura, T. (2015). Folate-dependent purine nucleotide biosynthesis in humans. Adv. Nutr. 6, 564–571. doi: 10.3945/an.115.008300
Balashova, O. A., Visina, O., and Borodinsky, L. N. (2018). Folate action in nervous system development and disease. Dev. Neurobiol. 78, 391–402. doi: 10.1002/dneu.22579
Barber, R. C., Lammer, E. J., Shaw, G. M., Greer, K. A., and Finnell, R. H. (1999). The role of folate transport and metabolism in neural tube defect risk. Mol. Genet. Metab. 66, 1–9. doi: 10.1006/mgme.1998.2787
Berding, K., and Donovan, S. M. (2016). Microbiome and nutrition in autism spectrum disorder: current knowledge and research needs. Nutr. Rev. 74, 723–736. doi: 10.1093/nutrit/nuw048
Bhandari, S. D., and Gregory, J. F. III (1992). Folic acid, 5-methyl-tetrahydrofolate and 5-formyl-tetrahydrofolate exhibit equivalent intestinal absorption, metabolism and in vivo kinetics in rats. J. Nutr. 122, 1847–1854. doi: 10.1093/jn/122.9.1847
Birnbaum, J., Pai, C. H., and Lichstein, H. C. (1967). Biosynthesis of biotin in microorganisms. V. Control of vitamer production. J. Bacteriol. 94, 1846–1853.
Blom, H. J., Shaw, G. M., den Heijer, M., and Finnell, R. H. (2006). Neural tube defects and folate: case far from closed. Nat. Rev. Neurosci. 7, 724–731. doi: 10.1038/nrn1986
Blutt, S. E., Crawford, S. E., Ramani, S., Zou, W. Y., and Estes, M. K. (2018). Engineered human gastrointestinal cultures to study the microbiome and infectious diseases. Cell. Mol. Gastroenterol. Hepatol. 5, 241–251. doi: 10.1016/j.jcmgh.2017.12.001
Britton, R. A., Irwin, R., Quach, D., Schaefer, L., Zhang, J., Lee, T., et al. (2014). Probiotic L. reuteri treatment prevents bone loss in a menopausal ovariectomized mouse model. J. Cell Physiol. 229, 1822–1830. doi: 10.1002/jcp.24636
Brosnan, M. E., MacMillan, L., Stevens, J. R., and Brosnan, J. T. (2015). Division of labour: how does folate metabolism partition between one-carbon metabolism and amino acid oxidation? Biochem. J. 472, 135–146. doi: 10.1042/BJ20150837
Burgess, C. M., Smid, E. J., Rutten, G., and van Sinderen, D. (2006). A general method for selection of riboflavin-overproducing food grade micro-organisms. Microb. Cell Fact. 5:24. doi: 10.1186/1475-2859-5-24
Camilo, E., Zimmerman, J., Mason, J. B., Golner, B., Russell, R., Selhub, J., et al. (1996). Folate synthesized by bacteria in the human upper small intestine is assimilated by the host. Gastroenterology 110, 991–998.
Chave, K. J., Ryan, T. J., Chmura, S. E., and Galivan, J. (2003). Identification of single nucleotide polymorphisms in the human gamma-glutamyl hydrolase gene and characterization of promoter polymorphisms. Gene 319, 167–175.
Clevers, H. (2016). Modeling development and disease with organoids. Cell 165, 1586–1597. doi: 10.1016/j.cell.2016.05.082
Crittenden, R. G., Martinez, N. R., and Playne, M. J. (2003). Synthesis and utilisation of folate by yoghurt starter cultures and probiotic bacteria. Int. J. Food Microbiol. 80, 217–222.
Cummings, J. H., and Macfarlane, G. T. (1997). Role of intestinal bacteria in nutrient metabolism. JPEN J. Parenter. Enteral Nutr. 21, 357–365. doi: 10.1177/0148607197021006357
D’Argenio, V., and Salvatore, F. (2015). The role of the gut microbiome in the healthy adult status. Clin. Chim. Acta 451(Pt A), 97–102. doi: 10.1016/j.cca.2015.01.003
Das, P., Babaei, P., and Nielsen, J. (2019). Metagenomic analysis of microbe-mediated vitamin metabolism in the human gut microbiome. BCM Genomics 20, 208–219. doi: 10.1186/s12864-019-5591-7
Engevik, M. A., Aihara, E., Montrose, M. H., Shull, G. E., Hassett, D. J., and Worrell, R. T. (2013). Loss of NHE3 alters gut microbiota composition and influences Bacteroides thetaiotaomicron growth. Am. J. Physiol. Gastrointest. Liver Physiol. 305, G697–G711. doi: 10.1152/ajpgi.00184.2013
Engevik, M. A., Engevik, K. A., Yacyshyn, M. B., Wang, J., Hassett, D. J., Darien, B., et al. (2015). Human Clostridium difficile infection: inhibition of NHE3 and microbiota profile. Am. J. Physiol. Gastrointest. Liver Physiol. 308, G497–G509. doi: 10.1152/ajpgi.00090.2014
Ettayebi, K., Crawford, S. E., Murakami, K., Broughman, J. R., Karandikar, U., Tenge, V. R., et al. (2016). Replication of human noroviruses in stem cell-derived human enteroids. Science 353, 1387–1393. doi: 10.1126/science.aaf5211
Evans, D. F., Pye, G., Bramley, R., Clark, A. G., Dyson, T. J., and Hardcastle, J. D. (1988). Measurement of gastrointestinal pH profiles in normal ambulant human subjects. Gut 29, 1035–1041.
Fatheree, N. Y., Liu, Y., Taylor, C. M., Hoang, T. K., Cai, C., Rahbar, M. H., et al. (2017). Lactobacillus reuteri for infants with colic: a double-blind, placebo-controlled, randomized clinical trial. J. Pediatr. 191, 170.e2–178.e2. doi: 10.1016/j.jpeds.2017.07.036
Ferstl, R., Frei, R., Schiavi, E., Konieczna, P., Barcik, W., Ziegler, M., et al. (2014). Histamine receptor 2 is a key influence in immune responses to intestinal histamine-secreting microbes. J. Allergy Clin. Immunol. 134, 744–746e743. doi: 10.1016/j.jaci.2014.04.034
Finkbeiner, S. R., Zeng, X. L., Utama, B., Atmar, R. L., Shroyer, N. F., and Estes, M. K. (2012). Stem cell-derived human intestinal organoids as an infection model for rotaviruses. mBio 3:e00159-12. doi: 10.1128/mBio.00159-12
Foulke-Abel, J., In, J., Kovbasnjuk, O., Zachos, N. C., Ettayebi, K., Blutt, S. E., et al. (2014). Human enteroids as an ex-vivo model of host-pathogen interactions in the gastrointestinal tract. Exp. Biol. Med. 239, 1124–1134. doi: 10.1177/1535370214529398
Foulke-Abel, J., In, J., Yin, J., Zachos, N. C., Kovbasnjuk, O., Estes, M. K., et al. (2016). Human enteroids as a model of upper small intestinal ion transport physiology and pathophysiology. Gastroenterology 150, 638.e8–649.e8. doi: 10.1053/j.gastro.2015.11.047
Frei, R., Ferstl, R., Konieczna, P., Ziegler, M., Simon, T., Rugeles, T. M., et al. (2013). Histamine receptor 2 modifies dendritic cell responses to microbial ligands. J. Allergy Clin. Immunol. 132, 194–204. doi: 10.1016/j.jaci.2013.01.013
Friend, B. A., Fiedler, J. M., and Shahani, K. M. (1983). Influence of culture selection on the flavor, antimicrobial activity, β-galactosidase and B-vitamins of yoghurt. Milchwissenschaft 38, 133–136.
Galofre, M., Palao, D., Vicario, M., Nart, J., and Violant, D. (2018). Clinical and microbiological evaluation of the effect of Lactobacillus reuteri in the treatment of mucositis and peri-implantitis: a triple-blind randomized clinical trial. J. Periodontal Res. 53, 378–390. doi: 10.1111/jre.12523
Gronbach, K., Eberle, U., Muller, M., Olschlager, T. A., Dobrindt, U., Leithauser, F., et al. (2010). Safety of probiotic Escherichia coli strain Nissle 1917 depends on intestinal microbiota and adaptive immunity of the host. Infect. Immun. 78, 3036–3046. doi: 10.1128/IAI.00218-0
Hague, A., Elder, D. J., Hicks, D. J., and Paraskeva, C. (1995). Apoptosis in colorectal tumour cells: induction by the short chain fatty acids butyrate, propionate and acetate and by the bile salt deoxycholate. Int. J. Cancer 60, 400–406.
Hayakawa, K., Kimura, M., Kasaha, K., Matsumoto, K., Sansawa, H., and Yamori, Y. (2004). Effect of a gamma-aminobutyric acid-enriched dairy product on the blood pressure of spontaneously hypertensive and normotensive Wistar-Kyoto rats. Br. J. Nutr. 92, 411–417.
Hiraoka, M., and Kagawa, Y. (2017). Genetic polymorphisms and folate status. Cong. Anom. 57, 142–149. doi: 10.1111/cga.12232
Hollister, E. B., Gao, C., and Versalovic, J. (2014). Compositional and functional features of the gastrointestinal microbiome and their effects on human health. Gastroenterology 146, 1449–1458. doi: 10.1053/j.gastro.2014.01.052
Hugenholtz, J., and Smid, E. J. (2002). Nutraceutical production with food-grade microorganisms. Curr. Opin. Biotechnol. 13, 497–507.
Ichihashi, T., Takagishi, Y., Uchida, K., and Yamada, H. (1992). Colonic absorption of menaquinone-4 and menaquinone-9 in rats. J. Nutr. 122, 506–512. doi: 10.1093/jn/122.3.506
Izquierdo Canas, P. M., Gomez Alonso, S., Ruiz Perez, P., Sesena Prieto, S., Garcia Romero, E., and Palop Herreros, M. L. (2009). Biogenic amine production by Oenococcus oeni isolates from malolactic fermentation of Tempranillo wine. J. Food Prot. 72, 907–910.
Jayashree, S., Jayaraman, K., and Kalaichelvan, G. (2010). Isolation, screening and characterization of riboflavin producing lactic acid bacteria from Katpadi, Vellore district. Recent Res. Sci. Technol. 2, 83–88.
Jensen, H., Roos, S., Jonsson, H., Rud, I., Grimmer, S., van Pijkeren, J. P., et al. (2014). Role of Lactobacillus reuteri cell and mucus-binding protein A (CmbA) in adhesion to intestinal epithelial cells and mucus in vitro. Microbiology 160(Pt 4), 671–681. doi: 10.1099/mic.0.073551-0
Kaehler, S. T., Baumgartner, H., Jeske, M., Anliker, M., Schennach, H., Marschang, P., et al. (2012). Prevalence of hypovitaminosis D and folate deficiency in healthy young female Austrian students in a health care profession. Eur. J. Nutr. 51, 1021–1031. doi: 10.1007/s00394-011-0281-5
Kamen, B. A., and Smith, A. K. (2004). A review of folate receptor alpha cycling and 5-methyltetrahydrofolate accumulation with an emphasis on cell models in vitro. Adv. Drug Deliv. Rev. 56, 1085–1097. doi: 10.1016/j.addr.2004.01.002
Kanehisa, M., and Goto, S. (2000). KEGG: kyoto encyclopedia of genes and genomes. Nucleic Acids Res. 28, 27–30.
Kemperman, R. F., Veurink, M., van der Wal, T., Knegtering, H., Bruggeman, R., Fokkema, M. R., et al. (2006). Low essential fatty acid and B-vitamin status in a subgroup of patients with schizophrenia and its response to dietary supplementation. Prostaglandins Leukot. Essent. Fatty Acids 74, 75–85. doi: 10.1016/j.plefa.2005.11.004
Kibe, R., Kurihara, S., Sakai, Y., Suzuki, H., Ooga, T., Sawaki, E., et al. (2014). Upregulation of colonic luminal polyamines produced by intestinal microbiota delays senescence in mice. Sci. Rep. 4:4548. doi: 10.1038/srep04548
Kim, D. H., Ahn, Y. O., Lee, B. H., Tsuji, E., Kiyohara, C., and Kono, S. (2004). Methylenetetrahydrofolate reductase polymorphism, alcohol intake, and risks of colon and rectal cancers in Korea. Cancer Lett. 216, 199–205. doi: 10.1016/j.canlet.2004.08.014
Kim, T. H., Yang, J., Darling, P. B., and O’Connor, D. L. (2004). A large pool of available folate exists in the large intestine of human infants and piglets. J. Nutr. 134, 1389–1394. doi: 10.1093/jn/134.6.1389
Kim, Y. I. (1999). Folate and cancer prevention: a new medical application of folate beyond hyperhomocysteinemia and neural tube defects. Nutr. Rev. 57, 314–321.
Kim, Y. I. (2006). Does a high folate intake increase the risk of breast cancer? Nutr. Rev. 64(10 Pt 1), 468–475.
Kimura, M., Hayakawa, K., and Sansawa, H. (2002). Involvement of gamma-aminobutyric acid (GABA) B receptors in the hypotensive effect of systemically administered GABA in spontaneously hypertensive rats. Jpn. J. Pharmacol. 89, 388–394.
Kumar, C. K., Moyer, M. P., Dudeja, P. K., and Said, H. M. (1997). A protein-tyrosine kinase-regulated, pH-dependent, carrier-mediated uptake system for folate in human normal colonic epithelial cell line NCM460. J. Biol. Chem. 272, 6226–6231.
Lakoff, A., Fazili, Z., Aufreiter, S., Pfeiffer, C. M., Connolly, B., Gregory, J. F., et al. (2014). Folate is absorbed across the human colon: evidence by using enteric-coated caplets containing 13C-labeled [6S]-5-formyltetrahydrofolate. Am. J. Clin. Nutr. 100, 1278–1286. doi: 10.3945/ajcn.114.091785
LeBlanc, J. G., Aubry, C., Cortes-Perez, N. G., de Moreno de LeBlanc, A., Vergnolle, N., Langella, P., et al. (2013a). Mucosal targeting of therapeutic molecules using genetically modified lactic acid bacteria: an update. FEMS Microbiol. Lett. 344, 1–9. doi: 10.1111/1574-6968.12159
LeBlanc, J. G., Milani, C., de Giori, G. S., Sesma, F., van Sinderen, D., and Ventura, M. (2013b). Bacteria as vitamin suppliers to their host: a gut microbiota perspective. Curr. Opin. Biotechnol. 24, 160–168. doi: 10.1016/j.copbio.2012.08.005
LeBlanc, J. G., Laino, J. E., del Valle, M. J., Vannini, V., van Sinderen, D., Taranto, M. P., et al. (2011). B-group vitamin production by lactic acid bacteria–current knowledge and potential applications. J. Appl. Microbiol. 111, 1297–1309. doi: 10.1111/j.1365-2672.2011.05157.x
Luk, B., Veeraragavan, S., Engevik, M., Balderas, M., Major, A., Runge, J., et al. (2018). Postnatal colonization with human “infant-type” Bifidobacterium species alters behavior of adult gnotobiotic mice. PLoS One 13:e0196510. doi: 10.1371/journal.pone.0196510
Mackenzie, D. A., Jeffers, F., Parker, M. L., Vibert-Vallet, A., Bongaerts, R. J., Roos, S., et al. (2010). Strain-specific diversity of mucus-binding proteins in the adhesion and aggregation properties of Lactobacillus reuteri. Microbiology 156(Pt 11), 3368–3378. doi: 10.1099/mic.0.043265-0
Magnusdottir, S., Ravcheev, D., de Crecy-Lagard, V., and Thiele, I. (2015). Systematic genome assessment of B-vitamin biosynthesis suggests co-operation among gut microbes. Front. Genet. 6:148. doi: 10.3389/fgene.2015.00148
Markowitz, V. M., Chen, I. M., Palaniappan, K., Chu, K., Szeto, E., Grechkin, Y., et al. (2012). IMG: the Integrated Microbial Genomes database and comparative analysis system. Nucleic Acids Res. 40, D115–D122. doi: 10.1093/nar/gkr1044
Maruvada, P., Leone, V., Kaplan, L. M., and Chang, E. B. (2017). The human microbiome and obesity: moving beyond associations. Cell Host Microbe 22, 589–599. doi: 10.1016/j.chom.2017.10.005
Maynard, C., Cummins, I., Green, J., and Weinkove, D. (2018). A bacterial route for folic acid supplementation. BMC Biol. 16:67. doi: 10.1186/s12915-018-0534-3
McCabe, L. R., Irwin, R., Schaefer, L., and Britton, R. A. (2013). Probiotic use decreases intestinal inflammation and increases bone density in healthy male but not female mice. J. Cell Physiol. 228, 1793–1798. doi: 10.1002/jcp.24340
McCabe, L. R., and Parameswaran, N. (2018). Advances in probiotic regulation of bone and mineral metabolism. Calcif. Tissue Int. 102, 480–488. doi: 10.1007/s00223-018-0403-7
McDougall, C. J., Wong, R., Scudera, P., Lesser, M., and DeCosse, J. J. (1993). Colonic mucosal pH in humans. Dig. Dis. Sci. 38, 542–545.
Menon, V., Wang, X., Greene, T., Beck, G. J., Kusek, J. W., Selhub, J., et al. (2005). Homocysteine in chronic kidney disease: effect of low protein diet and repletion with B vitamins. Kidney Int. 67, 1539–1546. doi: 10.1111/j.1523-1755.2005.00234.x
Mestas, J., and Hughes, C. C. (2004). Of mice and not men: differences between mouse and human immunology. J. Immunol. 172, 2731–2738.
Middendorp, S., Schneeberger, K., Wiegerinck, C. L., Mokry, M., Akkerman, R. D., van Wijngaarden, S., et al. (2014). Adult stem cells in the small intestine are intrinsically programmed with their location-specific function. Stem Cells 32, 1083–1091. doi: 10.1002/stem.1655
Million, M., Angelakis, E., Maraninchi, M., Henry, M., Giorgi, R., Valero, R., et al. (2013). Correlation between body mass index and gut concentrations of Lactobacillus reuteri, Bifidobacterium animalis, Methanobrevibacter smithii and Escherichia coli. Int. J. Obes. 37, 1460–1466. doi: 10.1038/ijo.2013.20
Morowitz, M. J., Carlisle, E. M., and Alverdy, J. C. (2011). Contributions of intestinal bacteria to nutrition and metabolism in the critically ill. Surg. Clin. North Am. 91, 771–785,viii. doi: 10.1016/j.suc.2011.05.001
Mu, Q., Tavella, V. J., and Luo, X. M. (2018). Role of Lactobacillus reuteri in human health and diseases. Front. Microbiol. 9:757. doi: 10.3389/fmicb.2018.00757
Nair, K. M., and Iyengar, V. (2009). Iron content, bioavailability & factors affecting iron status of Indians. Indian J. Med. Res. 130, 634–645.
O’Connor, E. M. (2013). The role of gut microbiota in nutritional status. Curr. Opin. Clin. Nutr. Metab. Care 16, 509–516. doi: 10.1097/MCO.0b013e3283638eb3
Pitkin, R. M. (2007). Folate and neural tube defects. Am. J. Clin. Nutr. 85, 285S–288S. doi: 10.1093/ajcn/85.1.285S
Pompei, A., Cordisco, L., Amaretti, A., Zanoni, S., Matteuzzi, D., and Rossi, M. (2007a). Folate production by bifidobacteria as a potential probiotic property. Appl. Environ. Microbiol. 73, 179–185. doi: 10.1128/AEM.01763-6
Pompei, A., Cordisco, L., Amaretti, A., Zanoni, S., Raimondi, S., Matteuzzi, D., et al. (2007b). Administration of folate-producing bifidobacteria enhances folate status in Wistar rats. J. Nutr. 137, 2742–2746. doi: 10.1093/jn/137.12.2742
Qiu, A., Jansen, M., Sakaris, A., Min, S. H., Chattopadhyay, S., Tsai, E., et al. (2006). Identification of an intestinal folate transporter and the molecular basis for hereditary folate malabsorption. Cell 127, 917–928. doi: 10.1016/j.cell.2006.09.041
Rajagopalan, P. T., Zhang, Z., McCourt, L., Dwyer, M., Benkovic, S. J., and Hammes, G. G. (2002). Interaction of dihydrofolate reductase with methotrexate: ensemble and single-molecule kinetics. Proc. Natl. Acad. Sci. U.S.A. 99, 13481–13486. doi: 10.1073/pnas.172501499
Rajan, A., Vela, L., Zeng, X. L., Yu, X., Shroyer, N., Blutt, S. E., et al. (2018). Novel segment- and host-specific patterns of enteroaggregative Escherichia coli adherence to human intestinal enteroids. mBio 9:e02419-17. doi: 10.1128/mBio.02419-7
Rajgopal, A., Sierra, E. E., Zhao, R., and Goldman, I. D. (2001). Expression of the reduced folate carrier SLC19A1 in IEC-6 cells results in two distinct transport activities. Am. J. Physiol. Cell Physiol. 281, C1579–C1586. doi: 10.1152/ajpcell.2001.281.5.C1579
Robador, A., LaRowe, D. E., Finkel, S. E., Amend, J. P., and Nealson, K. H. (2018). Changes in microbial energy metabolism measured by nanocalorimetry during growth phase transitions. Front. Microbiol. 9:109. doi: 10.3389/fmicb.2018.00109
Rolfe, M. D., Rice, C. J., Lucchini, S., Pin, C., Thompson, A., Cameron, A. D., et al. (2012). Lag phase is a distinct growth phase that prepares bacteria for exponential growth and involves transient metal accumulation. J. Bacteriol. 194, 686–701. doi: 10.1128/JB.06112-1
Rossi, M., Amaretti, A., and Raimondi, S. (2011). Folate production by probiotic bacteria. Nutrients 3, 118–134. doi: 10.3390/nu3010118
Roth, D., Chiang, A. J., Hu, W., Gugiu, G. B., Morra, C. N., Versalovic, J., et al. (2019). Two-carbon folate cycle of commensal Lactobacillus reuteri 6475 gives rise to immunomodulatory ethionine, a source for histone ethylation. FASEB J. 33, 3536–3548. doi: 10.1096/fj.201801848R
Rowland, I., Gibson, G., Heinken, A., Scott, K., Swann, J., Thiele, I., et al. (2018). Gut microbiota functions: metabolism of nutrients and other food components. Eur. J. Nutr. 1, 1–24. doi: 10.1007/s00394-017-1445-8
Said, H. M., and Mohammed, Z. M. (2006). Intestinal absorption of water-soluble vitamins: an update. Curr. Opin. Gastroenterol. 22, 140–146. doi: 10.1097/01.mog.0000203870.22706.52
Sato, T., and Clevers, H. (2013). Growing self-organizing mini-guts from a single intestinal stem cell: mechanism and applications. Science 340, 1190–1194. doi: 10.1126/science.1234852
Sato, T., Stange, D. E., Ferrante, M., Vries, R. G., Van Es, J. H., Van den Brink, S., et al. (2011). Long-term expansion of epithelial organoids from human colon, adenoma, adenocarcinoma, and Barrett’s epithelium. Gastroenterology 141, 1762–1772. doi: 10.1053/j.gastro.2011.07.050
Sato, T., Vries, R. G., Snippert, H. J., van de Wetering, M., Barker, N., Stange, D. E., et al. (2009). Single Lgr5 stem cells build crypt-villus structures in vitro without a mesenchymal niche. Nature 459, 262–265. doi: 10.1038/nature07935
Saxena, K., Blutt, S. E., Ettayebi, K., Zeng, X. L., Broughman, J. R., Crawford, S. E., et al. (2016). Human intestinal enteroids: a new model to study human rotavirus infection, host restriction, and pathophysiology. J. Virol. 90, 43–56. doi: 10.1128/JVI.01930-15
Saxena, K., Simon, L. M., Zeng, X. L., Blutt, S. E., Crawford, S. E., Sastri, N. P., et al. (2017). A paradox of transcriptional and functional innate interferon responses of human intestinal enteroids to enteric virus infection. Proc. Natl. Acad. Sci. U.S.A. 114, E570–E579. doi: 10.1073/pnas.1615422114
Schepper, J. D., Collins, F. L., Rios-Arce, N. D., Raehtz, S., Schaefer, L., Gardinier, J. D., et al. (2019). Probiotic Lactobacillus reuteri prevents postantibiotic bone loss by reducing intestinal dysbiosis and preventing barrier disruption. J. Bone Miner. Res. 34, 681–698. doi: 10.1002/jbmr.3635
Shen, J., Putt, K. S., Visscher, D. W., Murphy, L., Cohen, C., Singhal, S., et al. (2015). Assessment of folate receptor-beta expression in human neoplastic tissues. Oncotarget 6, 14700–14709. doi: 10.18632/oncotarget.3739
Shimizu, J., Kubota, T., Takada, E., Takai, K., Fujiwara, N., Arimitsu, N., et al. (2018). Propionate-producing bacteria in the intestine may associate with skewed responses of IL10-producing regulatory T cells in patients with relapsing polychondritis. PLoS One 13:e0203657. doi: 10.1371/journal.pone.0203657
Spinler, J. K., Sontakke, A., Hollister, E. B., Venable, S. F., Oh, P. L., Balderas, M. A., et al. (2014). From prediction to function using evolutionary genomics: human-specific ecotypes of Lactobacillus reuteri have diverse probiotic functions. Genome Biol. Evol. 6, 1772–1789. doi: 10.1093/gbe/evu137
Streit, W. R., and Entcheva, P. (2003). Biotin in microbes, the genes involved in its biosynthesis, its biochemical role and perspectives for biotechnological production. Appl. Microbiol. Biotechnol. 61, 21–31. doi: 10.1007/s00253-002-1186-2
Tabanelli, G., Torriani, S., Rossi, F., Rizzotti, L., and Gardini, F. (2012). Effect of chemico-physical parameters on the histidine decarboxylase (HdcA) enzymatic activity in Streptococcus thermophilus PRI60. J. Food Sci. 77, M231–M237. doi: 10.1111/j.1750-3841.2012.02628.x
Thomas, C. M., Hong, T., van Pijkeren, J. P., Hemarajata, P., Trinh, D. V., Hu, W., et al. (2012). Histamine derived from probiotic Lactobacillus reuteri suppresses TNF via modulation of PKA and ERK signaling. PLoS One 7:e31951. doi: 10.1371/journal.pone.0031951
Thomas, C. M., Saulnier, D. M., Spinler, J. K., Hemarajata, P., Gao, C., Jones, S. E., et al. (2016). FolC2-mediated folate metabolism contributes to suppression of inflammation by probiotic Lactobacillus reuteri. Microbiologyopen 5, 802–818. doi: 10.1002/mbo3.371
VanDussen, K. L., Marinshaw, J. M., Shaikh, N., Miyoshi, H., Moon, C., Tarr, P. I., et al. (2015). Development of an enhanced human gastrointestinal epithelial culture system to facilitate patient-based assays. Gut 64, 911–920. doi: 10.1136/gutjnl-2013-306651
Visentin, M., Diop-Bove, N., Zhao, R., and Goldman, I. D. (2014). The intestinal absorption of folates. Annu. Rev. Physiol. 76, 251–274. doi: 10.1146/annurev-physiol-020911-153251
Vollset, S. E., Clarke, R., Lewington, S., Ebbing, M., Halsey, J., Lonn, E., et al. (2013). Effects of folic acid supplementation on overall and site-specific cancer incidence during the randomised trials: meta-analyses of data on 50,000 individuals. Lancet 381, 1029–1036. doi: 10.1016/S0140-6736(12)62001-7
Walter, J., Chagnaud, P., Tannock, G. W., Loach, D. M., Dal Bello, F., Jenkinson, H. F., et al. (2005). A high-molecular-mass surface protein (Lsp) and methionine sulfoxide reductase B (MsrB) contribute to the ecological performance of Lactobacillus reuteri in the murine gut. Appl. Environ. Microbiol. 71, 979–986. doi: 10.1128/AEM.71.2.979-986.2005
Wang, X., Cabrera, R. M., Li, Y., Miller, D. S., and Finnell, R. H. (2013). Functional regulation of P-glycoprotein at the blood-brain barrier in proton-coupled folate transporter (PCFT) mutant mice. FASEB J. 27, 1167–1175. doi: 10.1096/fj.12-218495
Wibowo, A. S., Singh, M., Reeder, K. M., Carter, J. J., Kovach, A. R., Meng, W., et al. (2013). Structures of human folate receptors reveal biological trafficking states and diversity in folate and antifolate recognition. Proc. Natl. Acad. Sci. U.S.A. 110, 15180–15188. doi: 10.1073/pnas.1308827110
Yin, Y., Bijvelds, M., Dang, W., Xu, L., van der Eijk, A. A., Knipping, K., et al. (2015). Modeling rotavirus infection and antiviral therapy using primary intestinal organoids. Antiviral Res. 123, 120–131. doi: 10.1016/j.antiviral.2015.09.010
Yin, Y., and Zhou, D. (2018). Organoid and enteroid modeling of Salmonella infection. Front. Cell Infect. Microbiol. 8:102. doi: 10.3389/fcimb.2018.00102
Zachos, N. C., Kovbasnjuk, O., Foulke-Abel, J., In, J., Blutt, S. E., de Jonge, H. R., et al. (2016). Human enteroids/colonoids and intestinal organoids functionally recapitulate normal intestinal physiology and pathophysiology. J. Biol. Chem. 291, 3759–3766. doi: 10.1074/jbc.R114.635995
Zhao, R., and Goldman, I. D. (2007). The molecular identity and characterization of a proton-coupled folate transporter–PCFT; biological ramifications and impact on the activity of pemetrexed. Cancer Metastasis Rev. 26, 129–139. doi: 10.1007/s10555-007-9047-1
Zhao, R., and Goldman, I. D. (2013). Folate and thiamine transporters mediated by facilitative carriers (SLC19A1-3 and SLC46A1) and folate receptors. Mol. Aspects Med. 34, 373–385. doi: 10.1016/j.mam.2012.07.006
Keywords: B vitamin, enteroids, folate transporters, folylpolyglutamate, Lactobacillus reuteri, Lactobacilli, microbiome
Citation: Engevik MA, Morra CN, Röth D, Engevik K, Spinler JK, Devaraj S, Crawford SE, Estes MK, Kalkum M and Versalovic J (2019) Microbial Metabolic Capacity for Intestinal Folate Production and Modulation of Host Folate Receptors. Front. Microbiol. 10:2305. doi: 10.3389/fmicb.2019.02305
Received: 16 March 2019; Accepted: 20 September 2019;
Published: 09 October 2019.
Edited by:
Biswarup Mukhopadhyay, Virginia Tech, United StatesReviewed by:
Christina Bourne, University of Oklahoma, United StatesCopyright © 2019 Engevik, Morra, Röth, Engevik, Spinler, Devaraj, Crawford, Estes, Kalkum and Versalovic. This is an open-access article distributed under the terms of the Creative Commons Attribution License (CC BY). The use, distribution or reproduction in other forums is permitted, provided the original author(s) and the copyright owner(s) are credited and that the original publication in this journal is cited, in accordance with accepted academic practice. No use, distribution or reproduction is permitted which does not comply with these terms.
*Correspondence: James Versalovic, amFtZXN2QGJjbS5lZHU=
Disclaimer: All claims expressed in this article are solely those of the authors and do not necessarily represent those of their affiliated organizations, or those of the publisher, the editors and the reviewers. Any product that may be evaluated in this article or claim that may be made by its manufacturer is not guaranteed or endorsed by the publisher.
Research integrity at Frontiers
Learn more about the work of our research integrity team to safeguard the quality of each article we publish.