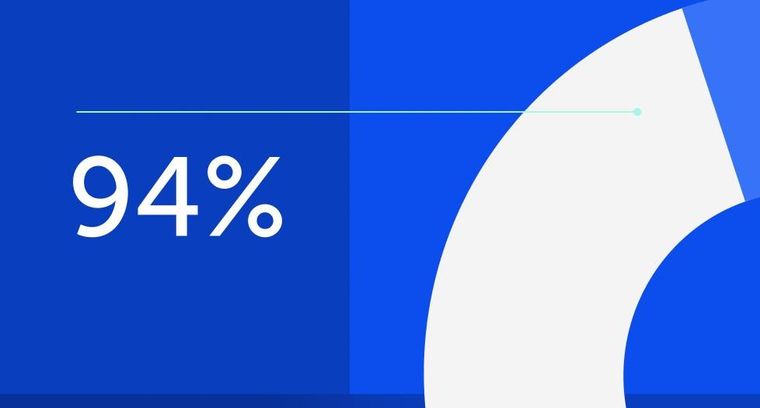
94% of researchers rate our articles as excellent or good
Learn more about the work of our research integrity team to safeguard the quality of each article we publish.
Find out more
ORIGINAL RESEARCH article
Front. Microbiol., 26 September 2019
Sec. Extreme Microbiology
Volume 10 - 2019 | https://doi.org/10.3389/fmicb.2019.02161
Exiguobacterium is a polyextremophile bacterial genus with a physiology that allows it to develop in different adverse environments. The Salar de Huasco is one of these environments due to its altitude, atmospheric pressure, solar radiation, temperature variations, pH, salinity, and the presence of toxic compounds such as arsenic. However, the physiological and/or molecular mechanisms that enable them to prosper in these environments have not yet been described. Our research group has isolated several strains of Exiguobacterium genus from different sites of Salar de Huasco, which show different resistance levels to As(III) and As(V). In this work, we compare the protein expression patterns of the three strains in response to arsenic by a proteomic approach; strains were grown in absence of the metalloid and in presence of As(III) and As(V) sublethal concentrations and the protein separation was carried out in 2D electrophoresis gels (2D-GE). In total, 999 spots were detected, between 77 and 173 of which showed significant changes for As(III) among the three strains, and between 90 and 143 for As(V), respectively, compared to the corresponding control condition. Twenty-seven of those were identified by mass spectrometry (MS). Among these identified proteins, the ArsA [ATPase from the As(III) efflux pump] was found to be up-regulated in response to both arsenic conditions in the three strains, as well as the Co-enzyme A disulfide reductase (Cdr) in the two more resistant strains. Interestingly, in this genus the gene that codifies for Cdr is found within the genic context of the ars operon. We suggest that this protein could be restoring antioxidants molecules, necessary for the As(V) reduction. Additionally, among the proteins that change their expression against As, we found several with functions relevant to stress response, e.g., Hpf, LuxS, GLpX, GlnE, and Fur. This study allowed us to shed light into the physiology necessary for these bacteria to be able to tolerate the toxicity and stress generated by the presence of arsenic in their niche.
Arsenic is a ubiquitous and abundant metalloid, frequently found in water bodies, accumulated in soil or as particulates in the air. There are two main oxidation states found in the environment: +5 [arsenate, As(V)] and +3 [arsenite, As(III)] (Mandal and Suzuki, 2002; Xiao et al., 2016). As(V) can interfere with important cell processes causing the substitution of PO4–2 inside the cell (Goyer and Clarkson, 2001; Andres and Bertin, 2016). On the other hand, As(III) is more toxic because it reacts with thiol groups, oxidizing cysteine residues inhibiting enzyme function, additionally it interferes with the maintenance of the redox state reacting with anti-oxidative molecules (Liu et al., 2001; Slyemi and Bonnefoy, 2012). Thus, cellular damage caused by arsenic is associated with its chemical nature, the generation of Reactive Oxygen Species (ROS) (Bhattacharjee and Rosen, 2007; Jomova et al., 2011), and the activation of the ROS-detoxifying machinery (Hrimpeng et al., 2006).
The presence of arsenic in its natural form occurs form geologic events (Bissen and Frimmel, 2003; Follmann and Brownson, 2009). Additionally, anthropogenic activities, like the mining industry, are the major causes of contamination and related health problems (Pacyna and Pacyna, 2001; Han et al., 2002). In northern Chile, the consumption of contaminated waters is the main cause of arsenic-related health problems (Smith et al., 1998; Flynn et al., 2002; Ferreccio and Sancha, 2006; Frazer, 2012). Specifically, in the Salar de Huasco there has been reports of toxic arsenic concentrations for humans with a temporal variation between 34 and 268 mg kg–1 in sediments (De Gregori et al., 2003; Herrera et al., 2009) and 70 mg L–1 in water (Herrera et al., 2014). However, the spatial variation in the concentration of the metalloid in the area is still relatively unknown.
There are four bacterial resistance mechanisms to arsenic described and the most studied are based on the toxicity reduction and expulsion of the arsenic species from the cell (Nies and Silver, 1995; Cánovas et al., 2003). The most common mechanism found in bacteria is the one mediated by the ars operon (Xiao et al., 2016). This operon mediates the reduction of As(V) to As(III) by the enzyme Arseniate reductase (ArsC) and the later expulsion from the cell trough the ArsB and/or ACR3 pumps (Slyemi and Bonnefoy, 2012). Another resistant mechanism is the dissimilatory reduction of As(V) through the genes in the arr operon, found mainly on bacteria grown in anoxic conditions, using this compound as the last electron acceptor (Malasarn et al., 2008; Ohtsuka et al., 2013). Additionally, successive methylations of As(III) by the arsenite methyltransferase ArsM for volatilization is another well-studied mechanism (Qin et al., 2006; Zhang et al., 2015). Finally, an oxidation-based mechanism to obtain energy while diminishing As(III) toxicity has been described in bacteria like Alcaligenes faecalis and Thermus aquaticus YT1 (Philips and Taylor, 1976; Gihring et al., 2001).
Exiguobacterium is a cosmopolitan genus composed by Gram-positive, facultative anaerobic, non-sporulating and motile rods that have been isolated from varied environments such as permafrost, salt lakes, deserts and even industrial wastes, suggesting a high plasticity, adaptation capacity, and tolerance to extreme environmental factors (Collins et al., 1983; Vishnivetskaya and Kathariou, 2005; Ordoñez et al., 2009; Vishnivetskaya et al., 2009). Strains from this genus have been reported to reduce and bio-accumulate arsenic (Anderson and Cook, 2004; Pandey and Bhatt, 2015), and show high resistance to oxidative stress (Takebe et al., 2007).
The Exiguobacterium sp. SH31 is a strain isolated from the chilean Altiplano area, in the Salar de Huasco sediments that has a high tolerance to As. The genome from this strain was sequenced and the genomic analyses revealed that it has a great variety of genes related to the response and tolerance to extreme environmental conditions (Castro-Severyn et al., 2017; Remonsellez et al., 2018). In regard to the arsenic resistance, it has the As(V) reduction arsRDAXB operon and also the acr3 gene. The presence of acr3 was reported for the first time in the Exiguobacterium genus in the genome of the S17 strain that was isolated from the Argentinian Altiplano (Belfiore et al., 2013), tolerates high concentrations of As(V) (150 mM) and As(III) (10 mM) (Ordoñez et al., 2013, 2015). Even though all Exiguobacterium strains have some version of the As(V) reduction operon, it is possible that the greater resistance presented by S17 and SH31 strains could be due the presence of ACR3 expulsion pump (Ordoñez et al., 2015). The presence of this gene has been previously reported in bacteria present on environment with high concentrations of arsenic (Cai et al., 2009).
The global molecular mechanisms in response to arsenic have been poorly described so far. Most of the studies are not comparable because they use different models, isolated in variable conditions and different As tolerance levels. Transcriptomic patterns of Enterobacteriaceae strain LSJC7 in response to As(V) and Herminiimonas arsenicoxydans in response to As(III) and As(V) revealed few constant elements, among these central metabolism and oxidative stress response (Cleiss-Arnold et al., 2010; Zhang et al., 2016). Comparative proteomic analyses of Exiguobacterium sp. S17 and Klebsiella pneumoniae exposed to arsenic have revealed that most of the proteins expression changes are related to general stress, oxidative stress, carbon metabolism and transport processes (Daware et al., 2012; Belfiore et al., 2013). These results show that the global response is not standard, and it does not depend exclusively on the described arsenic response systems, nevertheless, the level of arsenic tolerance is affected by the central metabolic response (Andres and Bertin, 2016). To better elucidate bacterial As resistance mechanisms at protein level, we aimed to study the stress response caused by the As(V) and As(III) in Exiguobacterium sp. For this, we compared the protein expression of three different strains of Exiguobacterium sp. isolated from different sites of the Salar de Huasco, where each of them had particular and different environmental conditions.
Exiguobacterium sp. SH31 was isolated from H4 site of the Salar de Huasco and its arsenic tolerance was described previously as [100 mM As(V) and 10 mM As(III)] (Castro-Severyn et al., 2017; Remonsellez et al., 2018). During February of 2017, another two Exiguobacterium strains SH0S7 and SH1S21 were isolated from sediment samples from H0 and H1 sites of the Salar de Huasco, respectively, (sites were described previously: Dorador et al., 2008). Strains were identified by 16S rRNA gene sequencing (ABI PRISM 3500xl Applied Biosystems – Centro de Secuenciación Automática de ADN, Pontificia Universidad Católica de Chile). Physicochemical parameters of sampling sites (temperature, salinity, pH) were recorded with a digital multi-parameter instrument (HI 98192 – HANNA Instruments) and total arsenic content was analyzed by IPC-MS (INQUISAL-CONICET, San Luis, Argentina). The arsenic tolerance of these two new strains was assessed by minimal inhibitory concentrations (MIC) assays. Briefly, bacterial cultures in Luria-Bertani broth (LB) were grown at 25°C with constant agitation (150 rpm) until 0.04 OD600. From this culture, we set up a micro plate with dilutions of As(III) and As(V) to a final concentration of 0.1–25 mM and 10–300 mM, respectively, for each strain. Finally, the plates were incubated at 25°C for 72 h with constant agitation, and OD600 was measured with a TECAN Infinite 200 PRO Nanoquant. Growth was monitored for 24 h at 25°C with OD600 measures every hour and curves were plotted using R package ggplot2 (Wickham, 2016).
The three Exiguobacterium strains were grown in LB at 25°C with constant agitation (150 rpm) overnight and this culture was used as inoculum to three flasks for each strain: 50 ml of LB as control, 50 ml of LB-As(III), and 50 ml of LB-As(V). Flasks were cultured at 25°C, 150 rpm up to mid-exponential growth phase (OD600 0.4). Arsenic conditions were particular to each strain, half of the MIC value was used as As(III) and As(V) treatment conditions. Bacterial cells were harvested by centrifugation (3,000 g, 10 min) and washed twice with tris–HCl buffer (50 mM, pH 8.5). Bacterial pellets were resuspended in 1 ml of the same buffer supplemented with 1 mM of PMSF (Phenylmethylsulfonyl Fluoride) and were sonicated at 40% amplitude (130 watts, 20 kHz) during 5 min (10 s on and 10 s off cycles) Ultrasonic Processor VCX-130, (Sonics, Inc.). The lysates were centrifuged at 24,000 g for 40 min at 4°C, the supernatants were recovered and stored at −80°C until use. Three independent assays for each strain and condition were performed. Protein concentrations were measured using the Coomassie (Bradford) Protein Assay (Thermo Fisher Scientific).
Two-hundred μg of protein for each sample were lyophilized, resuspended in 200 μL of lysis buffer (8 M urea, 2 M thiourea, 40 mM tris, 4% w/v CHAPS, 65 mM dithioerythritol (DTE), protease inhibitor (Roche Diagnostics) and incubated at room temperature on a rotating wheel for 2 h. Next, the samples were centrifuged 30 min at 20,000 g and the supernatants were collected and stored at −80°C until use. Protein quantification was performed using the RC DC Protein Assay (Bio-Rad).
Ten μg of protein extracts diluted in the IEF buffer (8 M urea, 2 M thiourea, 4% w/v CHAPS, 65 mM DTE, 0.0025% v/v bromophenol blue and 1% v/v IPG-buffer, pH 3-10 NL) were incubated for 1 h at room temperature on a rotating wheel. Precast IPG Strip (7 cm) with immobilized pH 4–7 gradient (Bio-Rad) were rehydrated with 125 μl of samples overnight. Isoelectric focusing (IEF) was carried out on an EttanTMIPGphorTM system (GE Healthcare) at 20°C using a gradient mode to a total amount of 8000 Vh. After IEF, proteins were reduced in equilibration buffer (65 mM DTT in 6 M urea, 1.5 M tris–HCl, pH 8.8, 30% v/v glycerol, 2% v/v SDS, and 0.001% v/v bromophenol blue) for 10 min, and then alkylated in equilibration buffer containing 135 mM iodoacetamide instead of DTT for 10 min. The proteins were separated in the second dimension on homemade 12% SDS-polyacrylamide gels using the Criterion Dodeca Cell system (Bio-Rad) at a constant voltage of 120 V during 80 min. Analytical gels were stained with silver nitrate. Three biological replicates per conditions and per strain were performed.
Gels were scanned (Epson perfection V750 Pro) and images were analyzed with the Progenesis Samespot® software v3.0 (Nonlinear Dynamics). After accurate gel alignment, the protein spots were detected automatically, and the quality of the automatic match was critically evaluated for each gel and, if necessary, corrections were made manually to eliminate any error or artifact in the protein spot assignment. Intensity values expressed as a percentage of normalized volumes are considered for further analysis.
Each selected protein spot was excised from preparative gels and washed successively with water, 50 mM triethylammonium bicarbonate (TEABC) and 100% acetonitrile. Dried protein spots were rehydrated in 50 mM TEABC containing 0.1 μg of Sequencing grade modified trypsin (Promega) and incubated at 37°C overnight. Digested peptides of each gel piece were extracted successively with 100% acetonitrile, 50 mM TEABC and 5% formic acid and the supernatant were recovered into a new tube. The pooled peptide solutions were stored at −20°C until use.
The protein digests were analyzed using a Q Exactive Plus mass spectrometer (Thermo Fisher Scientific) interfaced with a nano-flow HPLC (RSLC U3000, Thermo Fisher Scientific). Samples were loaded onto a 15 cm reverse phase column (Acclaim Pepmap 100® C18, Nano Viper, Thermo Fisher Scientific) and separated using a 30 min gradient of 2 to 40% gradient of buffer B (80% ACN, 0.1% formic acid) at a flow rate of 300 nl min–1. MS/MS analyses were performed in a data-dependent mode. Full scans (375–1,500 m/z) were acquired in the Orbitrap mass analyzer with a 70,000 resolution at 200 m/z. The twelve most intense ions (charge states ≥ 2) were sequentially isolated and fragmented by HCD (high-energy collisional dissociation) in the collision cell and detected at 17,500 resolution. The spectral data were analyzed via the Proteome DiscovererTM v1.4.1.12 software (Thermo Fisher Scientific). For protein identifications, databases used were: Exiguobacterium sp. (strain ATCC BAA-1283) (UniProt Proteome ID: UP000000716_EXISA-cano_2017_11.fasta) with the subsequent parameters: Trypsin as the enzyme, one missed cleavage allowed, carbamidomethylation of cysteine as fixed modification and oxidation as variable modification. The MS identification of all proteins follows some filters: Mascot significance Threshold (Significance threshold: 0.01) and peptides per protein (minimal number of peptides: 1, count only rank 1 peptide: true, count peptide only in top scored proteins: true). All the proteomics data have been deposited to the ProteomeXchange Consortium (Deutsch et al., 2016) via the PRIDE partner repository (Perez-Riverol et al., 2018) with the dataset identifier PXD01470.
To determine the relative expression of coding genes of identified proteins, we quantified transcripts levels by qRT-PCR. Bacterial strains were grown on the same conditions described previously and RNA extractions were carried out using the GeneJET RNA Purification Kit (Thermo Fisher Scientific) according to manufacturer’s instructions. RNA integrity, quality, and quantity were verified using 1% agarose electrophoresis, OD260/280 ratio and the QuantiFluor RNA System (Promega®). cDNA was synthesized using the M-MLV Reverse Transcriptase kit (Promega®) and random primer oligonucleotides hexamers (InvitrogenTM). The PCR reaction was carried out as follows: 10 min at 95°C followed by 40 amplification cycles (95°C × 30 s, 58°C × 30 s, and 72°C × 30 s), and a final step of 95°C × 15 s; 25°C × 1 s; 70°C × 15 s, and 95°C × 1 s) using specific primers for each gene (Supplementary Table S1). Transcript levels were quantified using the Brilliant II SYBR Green qPCR Master mix kit (Agilent Technologies) on a Stratagene Mx3000P thermal cycler. Gene expression levels were calculated according to Pfaffl (2001) using 16S rRNA gene as normalizator.
The arsenic resistance ars operon genetic context, including cdr gene was visualized using Genious® v7.1.9 software. Using SH31 strain as reference (UniProt: UP000177837; NCBI: LYTG01000000) for BLASTP (Altschul et al., 1990); we queried the studied strains plus others from GenBank (Supplementary Table S2) to compare sequence identity of the genes related to ars operon and arsenic resistance (presence and copy number was evaluated with E-value 1E–5 and 80% identity).
Western Blot analyses were performed to validate expression changes of the identified LuxS protein detected on the three strains 2D-GE in response to both arsenic conditions. Samples were prepared as described previously and 50 μg of protein supernatant were loaded for SDS-PAGE, followed by its blotting onto a nitrocellulose membrane (Mini-PROTEAN Tetra Cell/Blotting Module – Bio-Rad). LuxS protein was probed primary with a rabbit polyclonal anti-LuxS antibody (1:5000 dilution; MyBioSource: MBS1491336) and a mouse anti-rabbit AP-conjugated was used as secondary antibody (1:5000 dilution; Abcam: 99696). Membranes were digitalized and analyzed with ImageJ v1.52a software (Schneider et al., 2012) using the digital densitometry values to calculate the expression as fold change.
All statistics and figures were computed using the “R/Bioconductor” statistical open source software (Gentleman et al., 2004), Progenesis Samespot® software v3.0 (Nonlinear Dynamics) and GraphPad v5.0 (Prism®). The differential intensity levels of protein spots among the three conditions for each strain were analyzed using the R package Limma, an empirical Bayesian method for two group comparison similar to a two-sample t-test, except that a moderated t-statistic is calculated in which posterior residual SDs replace ordinary SDs (Ritchie et al., 2015). Differential relative gene expression for all comparison were analyzed using One-way ANOVA with post-hoc Tuckey HSD. A p-value less than 0.05 and fold change of more or less than 40% was considered statistically significant for all comparisons. Correlation analyses were performed using the R package Corrplot 0.84 (Wei and Simko, 2017) and plots were made with R package ggplot2 (Wickham, 2016). Heatmap and Hierarchical Ascendant Clustering (HAC) analysis was performed by using heatmap function of R package stats. HAC is a method of cluster analysis based on a pairwise distance matrix, which builds a hierarchy of clusters with sequentially agglomerative and divisive approaches. We used this method to organize the map and to group the statistically significant spots according to the nearest level of intensity. For this analysis, Euclidean distance and complete linkage were chosen as parameters.
Strains were isolated from different sites in the Salar de Huasco and those showed contrasting tolerance levels to As(III) and As(V). Each site had differential and specific environmental conditions (Figure 1A), it is mostly formed by shallow lagoons, permanent and non-permanent ponds and salt crusts (Uribe et al., 2015). The main differences among the three sampling points were salinity and arsenic concentrations. Salinity appears to increase gradually from north to south, as well as the As concentration with 9 mg kg–1 for H0 site, 16.3 mg kg–1 for H1 and 155 mg kg–1 for the H4 site, considering the distance between H0 and H1 is 1.6 km, between H1 and H4 is 2.4 km (the total distance from H0 to H4 is 4 km). Even though these samplings sites have been previously described (Dorador et al., 2008; Remonsellez et al., 2018), here we report for the first time the concentration and spatial variation of arsenic in the Salar de Huasco.
Figure 1. Sampling sites, strains characterization and As resistance. (A) Above: Salar de Huasco map showing the three sites locations (H0, H1, and H4), from which studied strains SH31, SH1S21, and SH0S7 were isolated (source: Google-Earth). Below: Parameters table of the three sites, sediment samples and isolated strains resistance levels. (B) Growth curves of the three studied strains, under the tested conditions: control, As(III) and As(V) at different concentration depending of the strains. OD600 readings were recorded during 24 h. Mean values (n = 3) are plotted.
Analysis of the 16S rRNA gene sequence of the three strains revealed that SH0S7 has 98% identity with AC-CS-C2 strain (FJ231171) and SH1S21 strain has 97% identity with SH31 strain (LYTG00000000.1). The Average Nucleotide Identity (97.4% SH31 – SH1S21; 99% SH31 – SH0S7; 97.4% SH1S21 – SH0S7 and an average of 94.6% between S17 and our three strains) evidenced that our three strains are very close and related, they would belong to the same species as well as the S17 strain (Ordoñez et al., 2013). These results imply that their phenotypes are not related to species classification or taxonomy.
We monitored the capacity of these strains to grow under the experimental conditions [As(III) and As(V) at concentrations corresponding to half of the MIC] (Figure 1B) and we found that in the three strains, As(III) generated a greater effect on the kinetic and growth patterns of the strains (Supplementary Table S3). It is worth mentioning that, under all conditions, the bacteria were able to grow efficiently and the presence of every phase of bacterial growth is clearly observed. In the strains SH31 and SH1S21, it can be observed that cultures reached OD600 of 0.4 in around 6 h. However, SH0S7 strains needed around 10 h to reach the same OD. This is probably due to a different requirements or ability to adapt to the culture conditions independently of the As concentrations.
To identify proteins that could be involved in the tolerance of bacteria to the toxicity and stress generated by the presence of arsenic, we analyzed by 2D-GE the proteomic profiles of each strain in the three conditions: control, As(III) and As(V) (examples of 2D-GE gels were showed in Figure 2). After image analysis, 999 protein spots were detected from proteins extracts with a wide range of molecular weight (20–200 kDa) and isoelectric points (4–7 pI). Various comparisons were performed to select informative differences in protein expression: comparison of control conditions, As(III) and As(V) for each strain, comparisons of control conditions separately, As(III) and As(V) for all strains.
Figure 2. Proteomic analysis of the three studied strains. (A) 2D-GE of proteins extracted from supernatant of bacteria culture in As(V) condition for each Exigobacterium strain showed as example. (B) Heat maps of the top 100 protein spots by expression levels, present in all 2D-GE samples (three strains in three conditions in triplicate). The intensity levels (percentage of normalized volumes) of the protein spots were visualized by a heat map, according to their statistical significance p of the Limma analysis, which were plotted using ggplot2. Each column represents the data from one 2D-GE experiment. Rows represent individual spots and graduated scale color codes from green (low level of intensity) to red (high level of intensity).
The comparison to the control condition to As(III) and As(V) conditions allowed to identify, respectively, 173 and 90 differential protein spots expressed in SH31 strain, 111 and 143 in SH1S21 strain and 77 and 120 for SH0S7. We observed more differential protein spots expressed between control vs. As(V) for the SH1S21 and SH0S7 strains than control vs. As(III) comparison. This was the opposite for the SH31 strain. It is not clear what are the reasons for these differences between the three strains response patterns.
In order to better visualize the protein expressions, the “100 best” protein spots, statistically different in each strain, were subjected to hierarchical clustering analysis generating the heat map shown in Figure 2. We observed that replicates are correctly grouped by conditions in each strain. Data distribution according to selection criteria is shown in the Supplementary Figure S1. Then we selected the most relevant protein spots (statistically and manually verified), from all comparisons for mass spectrophotometry identification. Several stringent criteria to select protein spots for MS identification were imposed: statistical significance (p ≤ 0.05) and fold change (FC ± 40%), differential protein spot expression should be change and in the same way in the triplicate samples and inter-strains comparison were also taken in consideration, meaning that we took into account, whether if the spot was present in one strain (regardless significative expression change) but absent in another. All selected spots were manually validated.
A total of 27 protein spots were selected and 25 different proteins were identified by MS (Table 1). Most of the identified proteins were assigned to four categories according to its function in the cell: metabolism (GcvPB, PdhA, GlpX(2), SucD, ArcC, PfkA, TpiA, and DhaL), synthesis [CarB, GlnE, WecC, PdxS(2), PunA, and GlmS], stress response (DnaK, Cdr, A0A1G1SP02, Hpf, LuxS, and Fur), transport (ArsA, A0A1G1SJA6, YxdL, and ArtM) and one hypothetical protein.
The regulation of proteins involved in the response to arsenic treatment is different depending on the studied strain as shown in Figure 3. For instance, in Exiguobacterium sp. SH31 the robust up regulation occurs on ArsA and Cdr proteins in both As conditions. The detection of ArsA protein in response to arsenic is described here for the first time for the Exiguobacterium genus. On the other hand, the up regulation of Cdr protein would demonstrate the activation of the antioxidant mechanism, necessary for counteracting the oxidative stress caused by arsenic (Weiss et al., 2009; Ciprandi et al., 2012; Slyemi and Bonnefoy, 2012; Kruger et al., 2013).
Figure 3. Expression heat map of the identified proteins. Table shows protein expression levels in response to arsenic conditions for the three studied strains. Number indicates fold change for As(III) and As(V) regarding control condition. Each column represents the mean data of three replicate for each As condition. The graduated scale color codes were from blue (low level of intensity) to red (high level of intensity), asterisks represent statistical significance. ∗p ≤ 0.05; ∗∗p ≤ 0.01; and ∗∗∗p ≤ 0.001.
The response to As in this strain is also manifested on several proteins related to metabolism (Figure 3). For instance, the GlpX protein is a fructose-1,6-bisphosphatase and was up-regulated by both species of As. This enzyme would generate Pi to save energy, in response to phosphate transport regulation (to avoid As influx) (Movahedzadeh et al., 2004; Elias et al., 2012), and generate reducing power in the form of NADPH throught the pentose phosphate pathway (Nelson and Cox, 2008). Additionally, the glutamine–fructose-6-phosphate aminotransferase (GlmS) was up-regulated by both As species, this protein catalyzes the first step in the hexoamines pathway using fructose-6P to produce glucosamine-6P, which is a precursor for cell wall synthesis. Another protein that was significantly up-regulated in response to As(III) is WecC (UDP-N-acetyl-D-mannosamine dehydrogenase). WecC participates in the synthesis of polysaccharides that could be involved in the biofilm production, a known defense strategy. The extracellular matrix has also been reported as a strategy for sequestering of toxic compounds, as they get trapped in the structure (Weiss et al., 2009).
The pyruvate dehydrogenase E1 component (PdhA) was up-regulated only in response to As(III), this was previously described in Staphylococcus exposed to As(V) (Srivastava et al., 2012). The ATP-dependent 6-phosphofructokinase (PfkA) that catalyzes the fructose-1,6-bisphosphate formation is down-regulated only by As(V), corresponding to the cell stress state and the need for reductant generation and energy salvage. This is consistent with the slight increase in the triosephosphate isomerase (TpiA) in As(III), that generates glyceraldehyde 3-phosphate, indicating activation of late stage of glycolysis, with reductants gaining (Trujillo et al., 2014). The dihydroxyacetone kinase subunit L (DhaL) and the glycine dehydrogenase subunit 2 (GcvPB) were found to be significantly down-regulated in both arsenic conditions. The carbamate kinase (ArcC) was down-regulated in both arsenic condition but it is only significant in As(V) condition. The glutamine synthetase (GlnE) is a negative regulator of glutamine synthesis concerning to cell nitrogen levels and it was up-regulated in both As conditions (Leigh and Dodsworth, 2007), suggesting an increase in energy generation. As a whole these changes in expression level of different metabolic pathways demonstrate that energy generation is one of the bacterial strategies to overcome As related stress (Nyström, 2004).
Pyridoxal phosphate has been previously associated with the response to several stress conditions mainly acid, osmotic and as an important factor against oxidative stress (Han et al., 2005; Schnell et al., 2015; Xie et al., 2017). Here we found, that a subunit of the pyridoxal 5′-phosphate synthase (PdxS) was significantly down-regulated in response to As(V) and up-regulated in presence of As(III). This compound is a co-factor in the cysteine synthesis which is a key component of thiols (antioxidants) (Nelson and Cox, 2008; Schnell et al., 2015). Also, purine nucleoside phosphorylase (PunA) was down-regulated in As(III) and As(V) condition, this enzyme participates in the formation of nucleic acid precursors and has also been demonstrated that during stress they can become sources of energy, carbon and nitrogen (Kilstrup et al., 2005).
Among the proteins identified as related to stress response, the S-ribosylhomocysteine lyase (LuxS) was found to be over expressed in both As conditions, LuxS function is associated with quorum sensing and biofilm formation. The transcriptional repressor (Fur), ribosome hibernation promoting factor (Hpf) and a metallophosphoesterase (A0A1G1SP02) are down-regulated in both As conditions as previously reported (Pandey et al., 2012; Belfiore et al., 2013; Sacheti et al., 2014). DnaK chaperon was only up-regulated in this strain on As(III) condition. In previous works, DnaK protein was detected as part of arsenic response as well as other stress situations, participating in misfolded and aggregated proteins recovery (Zhang et al., 2007; Daware et al., 2012; Belfiore et al., 2013).
In regards to transport, the YxdL protein, associated to the bacitracin resistance was increased in response to As(III). Transport through the membrane is one of the most common mechanisms to avoid toxicity used by bacteria (Nies and Silver, 1995). The proteins of cellular transport were strongly down regulated in K. pneumoniae in response to As(III), to avoid toxic influx (Daware et al., 2012). On the contrary, the transporters ArtM and A0A1G1SJA6 were down-regulated, this could be a way of preventing toxic influx as aforementioned.
Another strain studied was Exiguobacterium sp. SH1S21, that showed contrasting results compared to SH31, namely, the absence of protein spots corresponding to SucD, ArcC, CarB, WecC, PunA, LuxS, Fur, A0A1G1SP02 and to GlpX and PdxS protein spots (only on one of the two protein spots) on both As conditions, evidencing clearly a different pattern. On the other hand, the strong induction of GcvPB, Hpf, and ArsA in both As conditions was observed. The identified proteins were either absent or up-regulated but no significantly down-regulated in SH1S21. ArsA demonstrates an active response against arsenic as aforementioned. The expression of the enzymes that participate on transcription and translation such as Hpf has been proposed as cellular stress indicators, because protein biosynthesis is increased in response to adverse conditions (Sacheti et al., 2014). Furthermore, the glycine cleavage system (GcvPB), in addition to generate helpful molecules from glycine could also generate important reductants against As associated oxidative stress (Okamura-Ikeda et al., 1993). Moreover, PfkA and PdxS were up-regulated in As(III) while YdxL and DhaL were only over expressed in As(V). DhaL catalyzes the formation of dihydroxyacetone phosphate and pyruvate that would be feeding into the carbohydrates metabolism for energy generation (Gutknecht et al., 2001). It is worth mentioning that the regulation of PfkA and YdxL proteins is contrary to that observed in SH31 strain, suggesting the relevance of a different defense mechanism in this strain.
Finally, comparing Exiguobacterium sp. SH0S7 with the SH31 strain, the most relevant findings are: the detection of a second PdxS protein exclusively on this strain, being repressed in As(III) but induced in As(V); the presence of an hypothetical protein, although has no significant change is exclusive for this strain, which is the one with the highest As tolerance among the three. The PdxS protein identified only in SH0S7 is regulated opposite to the PdxS protein identified in SH31 according to As conditions. This observation suggests a modification of the PdxS protein that is dependent on the arsenic condition and the strain, however, this remains to be demonstrated. Moreover, another exclusive finding for this strain is the induction of the A0A1G1SJA6 transporter in both conditions, which is poorly characterized and could be a determining factor on the great tolerance of this strain, this protein is a good candidate for further investigation. The induction of ArsA, Cdr, LuxS, Hpf and the repression of Fur supports our previous statements about an active response against arsenic and its associated stress.
Additionally, other up-regulated proteins, in both As conditions and involved in metabolism cell were GlpX, DhaL, ArcC and SucD. The carbamate kinase (ArcC), is associated with several pathways, such as the metabolism of purines, proline, arginine, and glutamate (important part of glutathione), carbamoyl phosphate can be used as a source of phosphate in glycolysis and the energy metabolism, it has also been reported as part of osmotic stress response in several bacteria (Casiano-Colón and Marquis, 1988; Ceylan et al., 2012). On the other hand, Carbamoil-fosfato sintasa I (CarB) is absent in this strain regarding SH31, and it produces carbomyl phosphate with a greater energy cost which would be inconvenient for the cell under As stress (Nyunoya and Lusty, 1983). Also, Succinate–CoA ligase (SucD) participates in the TCA cycle, in a step that produces energy in form of ATP/GTP and CoA-SH as reducing agent (Joyce et al., 1999). Proteins related to energy metabolism were the most enriched from the ones that were differentially expressed. Comprising proteins that participate in pathways like pentose phosphate, glycolysis, amino acid metabolism that would be in accordance to the necessary requirements for the bacteria to prosper under As-generated stress.
Finally, in order to validate the protein regulation observed we have studied the regulation level of protein transcripts. For that, we carried out qRT-PCR quantification of the transcripts for ten of the identified proteins (Figure 4A). In the comparison of As(III) and As(V) conditions to control condition, we observed a statistically significant regulation of 8, 6, and 10 transcripts studied for the SH31, SH1S21, and SH0S7 strains, respectively. The protein and transcript expression levels were compared, and a correlation index was calculated. The mRNA levels are overall well correlated at the protein level, the TpiA transcript/protein which has the most notorious negative correlation (Figure 4B).
Figure 4. Transcriptomic expression of genes that code for the identified proteins. (A) Gene and protein fold change expression: Bars represents relative fold change gene expression related to control condition of selected genes, measured under the same As conditions as in the proteomic experiments (colored by condition). Plotted data is an average of three independent experiments with three technical replicates each. Asterisks represent statistical significance of transcriptional expression experiments both As compared to control condition. The protein mean fold changes (three independent experiments) obtained from proteomic analysis are represented by the black circles. The data for both As conditions are represented relative to the control values. (B) Correlation index between transcriptional and protein fold change expression values. Positive correlations are displayed in blue and negative correlations in red color. Color intensity and circle size are proportional to the correlation coefficients; asterisks represent statistical significance. ∗p ≤ 0.05; ∗∗p ≤ 0.01; and ∗∗∗p ≤ 0.001.
The proteins identified in the supernatant of Exiguobacterium bacteria subject to arsenic conditions might reflect altered biological processes and pathways. To carry out functional analysis, the 25 differentially expressed proteins were subjected to gene ontology and protein-protein interaction analysis. From these protein sets, a total of five biological processes were enriched in Gene Ontology analyzed by Ontologizer (Bauer et al., 2008) and PANTHER (Mi and Thomas, 2009). The most obvious enriched categories (p-value and ratio observed genes/total genes described in the process) were fructose 6-phosphate metabolic process (GO:0006002) and aldehyde biosynthetic process (GO:0046184). The proteins assigned to these two categories were PfkA (both), GlmS and TpiA, PdxS, respectively. The fructose 1,6-bisphosphate metabolic process (GO:0030388) was also identified as co-occurring terms of fructose 6-phosphate metabolic process (GO:0006002) with two proteins assigned: GlpX, PfkA. The glycolysis (P00024) was the only pathway enriched in PANTHER. For the protein-protein interaction analysis, we built a wider network by looking at connected proteins (first-degree neighbors) with our set of 25 identified proteins using the merge network imported from Cytoscape v3.6.0 (Shannon et al., 2003). Only one highly connected “hub” protein was identified: catabolite control protein A (CcpA) connecting six identified proteins (Supplementary Figure S2).
The cdr gene encodes for a coenzyme A disulfide reductase (dehydrogenase), which has been associated with oxidative stress response. Interestingly, exploring the SH31 strain genome we found that the cdr gene is located within the genomic context of the ars operon. The same contexts were observed in the SH1S21 and SH0S7 strains genomes (GenBank accessions: SH1S21: GCA_004337175.1 and SH0S7: GCA_004337195.1). This shows a possible association of its function with the As(V) detoxification mechanism. This relationship has never been described before. In order to verify if this statement is valid for the genus, we carried out a comparative analysis of the ars operon (and arsenic related genes arsC and acr3) of these three strains in addition to 33 other Exiguobacterium genomes available in GenBank (Supplementary Table S2). This result led us to conclude that independent of the operon composition (arsRDAB or arsRB) and the differential tolerance level to As, the gene cdr is present on every case downstream from the gene arsB (Figure 5). It is interesting to observe that the arsC reductase seems to be more associated to the strains of group 2 and the acr3 accessory pump would be more related to the altiplanic strains or those with a higher level of As resistance, as reported before (Castro-Severyn et al., 2017). Strikingly, the genetic sequence of the acr3 accessory pump is not present in SH1S21 strain, which could be corresponding to the low As resistance level presented by this strain compared to the other two.
Figure 5. Genetic organization of relevant arsenic resistance genes. Genes of interest in Exiguobacterium strains: arsR arsenical resistance operon repressor; arsD arsenical resistance operon trans-acting repressor; arsA arsenical pump-driving ATPase; Fe–S putative iron sulfur protein; arsB arsenical pump membrane protein; cdr coenzyme-A disulfide reductase; arsC arsenate reductase; acr3 arsenite efflux pump. Heat scale shows identity percentage of all strains sequence compared, using as reference the Exiguobacterium sp. SH31 genes. Grouped according to Vishnivetskaya et al. (2009).
To validate the LuxS protein regulation observed in 2D-GE, we carried out the quantification of the LuxS at protein level through western blot as well as it was previously validated at transcript level. An up regulation of LuxS was observed for As(III) condition compared to the control conditions (1.47 and 1.38, for SH31 and SH0S7, respectively). This regulation is weaker, in As(V) condition (1.21 and 1.27 for SH31 and SH0S7, respectively). These results confirm the proteomic data of SH31 and SH0S7 strains with an upregulation in both As conditions (Supplementary Figure S3). In SH1S21 strain, LuxS expression level did not vary in any arsenic condition compared to the control [0.76 and 0.91, As(III) and As(V), respectively], being non-correlative neither with proteomic observation nor with the transcriptional gene expression. These results support our findings and could imply relevance of LuxS protein in the capacity of the studied strains to tolerate arsenic.
The organisms living in the different environments found in high-altitude Andean lakes have to tolerate/resist diverse extreme conditions as well as the co-occurrence of these (Albarracín et al., 2015, 2016). Among the most relevant conditions for this area are desiccation, temperature variation, osmotic pressure, solar radiation and mineral composition which is very variable, including, in most cases, toxic components such as arsenic (Risacher and Fritz, 2009; Uribe et al., 2015; Hernández et al., 2016). Specifically, the three sampled spots in the Salar de Huasco showed variability in regards to physicochemical parameters (Figure 1A). Salinity is one of the most influencing factors that pressure and shape microbial communities’ composition (Canfora et al., 2014; Kimbrel et al., 2018). In Salar de Huasco the great microbial diversity and its variation within the area have been previously described (Dorador et al., 2008, 2010). In this relatively small area, salinity variation goes from freshwater to hypersaline water, which would contribute to diversity. This is in accord to the salinity values that we found, from 8.1% in H1 to 77.2% in H4 collection spots (Figure 1A).
Arsenic presence specifically in northern Chile is well documented, due to mining exploitation (mineral richness) and the great health problems associated (Ferreccio and Sancha, 2006; Demergasso et al., 2007; Roh et al., 2018; Tapia et al., 2018). In spite of this, only limited As measurements have been reported for Salar de Huasco (De Gregori et al., 2003; Herrera et al., 2009). Our results show an important variation of As concentration in the analyzed sediments, which increases proportionally from north to south, corresponding to the water flux model described by Hernández et al. (2016). Underground sources and superficial flow feed these water bodies, and aquifers that provide the underground water are refilled by seasonal rain percolation. Furthermore, mineral composition levels are similar between the streams and their underground sources. However, evaporation and natural flux cause a gradual accumulation in sediments by stratification and precipitation. This phenomenon explains the As increase on the southern site (H4), where the flow or shallow current is gradually weaker in comparison to the northern ones (Acosta and Custodio, 2008; Hernández et al., 2016).
Enrichment of bacteria capable of metabolizing arsenic compounds has been reported in Salar de Ascotan and Salar de Atacama, located also in northern Chile, where conditions are similar to those found in Salar de Huasco. This is related to the high salinity and As concentrations present in these environments, confirming the fact that a metabolism based on bio-energetically favorable electron acceptors such as As(V) becomes more important when the salinity is near the saturation level (Lara et al., 2012). Also, it has been reported the presence and enrichment of bacteria capable to precipitate As (as realgar and orpiment minerals) in brine and boron deposits (Demergasso et al., 2007).
The As(V) and As(III) tolerance levels shown for our three strains contrast with those previously reported for this genus: strains WK6 (Anderson and Cook, 2004), KM05 (Sunita et al., 2012), S17 (Belfiore et al., 2013), PS NCIM 5463 (Sacheti et al., 2014), AS-9 (Pandey and Bhatt, 2015), antarcticum B7 (da Costa et al., 2018), and profundum PT2 (Andreasen et al., 2018). We highlight the ability of SH0S7 strain, isolated from H0 site, to tolerate 20 mM of As(III), one of the highest concentrations reported so far.
The capacity to grow and tolerate the presence of high concentrations of As in all three strains of Exiguobacterium was evaluated using half of the determined MIC value for both As(V) and As(III) (Figure 1B). All strains grew on the presence of both As species and we calculated the specific growth rates (μ) (Blanch and Clark, 1997) and the generation time (GT) (Álvarez-Ordóñez et al., 2010) for each strain. We observed that the As(III) causes a greater effect as a delay growth in the three studied strains affecting significantly μ (diminished) and GT (increased) parameters (Supplementary Table S3). This was expected because it was previously reported the negative effect of As(III) on bacterial growth due to its higher toxicity (Petänen et al., 2003; Poirel et al., 2013). In regard to As(V), these parameters were less affected and even a slightly better growth rate was observed in SH1S21 and SH0S7 strains regarding control condition. This phenomenon is related to the fact that the bacteria can use the energy generated by As(V) reduction (Ordoñez et al., 2015).
Qualitative and quantitative differences in proteomic profiles were observed not only between control and As conditions for each strain, but also among the strains. Indeed, a global coherence between the strains proteomic patterns was not observed, considering the presence/absence of the identified spots and their expression levels (Figure 3 and Table 1). It is interesting to note that the levels of regulation reported in our study are much greater, compared to previously reported results, comprising a wide range of fold change values from 0.26 to 12.8 (Ciprandi et al., 2012; Daware et al., 2012; Belfiore et al., 2013).
The protein levels of a cell reflect the state of physiological response to the conditions in which it is found on a determined point in time, against any alteration (Cleiss-Arnold et al., 2010). Consequently, it is relevant to study the changes in protein patterns as effect of external pressures and understanding the state of the cell as a system. All differences observed between the three strains could be the result of their adaptations to their environment specific conditions and the identified proteins could have a relevant role in the arsenic tolerance level.
Each studied strain presents a slightly different protein expression pattern in response to As. In the Exiguobacterium sp. SH31 strain we found that the ArsA and Cdr proteins are expressed in both As conditions, this would suggest an active response to address arsenic toxicity (Slyemi and Bonnefoy, 2012), as this protein is part of ars operon and its expression is correlated to the activation of the whole system (Andres and Bertin, 2016). Additionally, Cdr has an important antioxidant role in organisms that do not have classic detoxification systems (Cárdenas et al., 2012; Mateos et al., 2017). Moreover, it has been found that CoASH is the major low-molecular-weight thiol in Borrelia burgdorferi being able to reduce H2O2 and then be regenerated by Cdr, supporting a role associated with ROS defense (Boylan et al., 2006). In regard to metabolism, key proteins such as GlpX and GlmS were up-regulated by both species of As, suggesting the requirement for Pi generation and avoid As influx; also, production of NADPH as a reductant molecule as well as cell wall synthesis. Additionally, polysaccharide synthesis (WecC) is affected by the presence of As(III), that could be related to biofilm production.
Also, PdhA was up-regulated only in response to As(III), suggesting part of the response to As is to generate reductants that could be counteracting As-associated oxidative stress (Nelson and Cox, 2008). In line with this results, the expression of PdxS is up-regulated in presence of As(III). This compound is a co-factor in the cysteine synthesis which is a key component of thiols (antioxidants) (Nelson and Cox, 2008; Schnell et al., 2015). Also, an increase in cell nitrogen levels associated with the upregulation of the GlnE is an indicator of an increase in energy generation, parts of these pathways can feed TCA cycle as it has been demonstrated in Ferroplasma in response to As(III) (Baker-Austin et al., 2007). The metabolic response to As has not been completely clarified given the fact that there are not enough data to support robust arguments and the investigations available have been done with very diverse organism from particular niches (Andres and Bertin, 2016). All these changes in expression of proteins related to metabolic pathways demonstrate that energy generation is one of the bacterial strategies to overcome As-related stress (Nyström, 2004).
As it was previously reported for H. arsenicoxydans and Exiguobacterium sp. S17 (Cleiss-Arnold et al., 2010; Belfiore et al., 2013) we found that PunA expression is repressed in As(III) and As(V) conditions. Also, LuxS, a general stress protein, associated with the control of controlling quorum sensing and biofilm formation (Sewald et al., 2007; Hardie and Heurlier, 2008; Remonsellez et al., 2018) is over expressed in both As condition. The resistance to As is increased by the activation of the global stress response, as well as the protection against metals/metalloid associated oxidative stress (Daware et al., 2012). The induction of proteins related to the iron uptake under As exposure has been previously reported (Zhang et al., 2016). Fe is an important cofactor that participated in several central metabolism, biosynthetic pathways and gene regulation (Hassan and Troxell, 2013). This phenomenon would correspond to the Fur protein down regulation in our study. In SH31, transport-related proteins, like YxdL was increased in response to As(III). Transport through the membrane is one of the most common mechanisms to avoid toxicity used by bacteria (Nies and Silver, 1995). The proteins of cellular transport were strongly repressed in K. pneumoniae in response to As(III), to avoid toxic influx (Daware et al., 2012). These changes in transport processes modify the pathways of anabolism and catabolism to compensate for the lack of biomolecules that in regular conditions are taken from extracellular media (Andres and Bertin, 2016). It has been widely reported that molecular transport is essential for bacteria survival during As-associated stress, for both intake and extrusion. However, detecting transporters in proteomics is challenging because they are probably insoluble and could be constitutively expressed, as reported by Belfiore et al. (2013) for ArsB and ACR3. Our results show that the genes for both transporters are in SH31 genome (Figure 5; Castro-Severyn et al., 2017) and here we demonstrated that the expression level of ArsA is increased at transcript and protein levels in response to As (Figure 4).
Exiguobacterium sp. SH1S21 shows a different expression pattern in some proteins, the activation of As response is evidence by the upregulation of ArsA, also, stress response proteins such as Hpf and GcvPB are also found to be upregulated. Additionally, we showed that the sequence of the acr3 accessory pump is absent in SH1S21 strain and also Cdr, LuxS, Fur and ArcC proteins were not detected or not regulated in SH1S21 compared to SH31 and SH0S27 strains independently of As conditions. These results suggest a role of these proteins in the mechanisms involved in the low As resistance level presented by this strain.
Furthermore, Exiguobacterium sp. SH0S7, that has the highest As tolerance from the three strains, has a second PdxS protein detected exclusively on this strain, interestingly we found an induction of the A0A1G1SJA6 transporter in both conditions, this could be relevant to the ability to tolerate high concentration of the metalloid, and needs further investigation. Also, the induction of ArsA, Cdr, LuxS, Hpf and the repression of Fur is consistent with observations made in the other strains. Another important protein induced is the Carbamate kinase (ArcC) associated to key pathways. In this strain is also evidenced the importance of metabolic modulation under this stress, as during glycolysis, it has been found that As(V) is able to interfere with this metabolic process by the substituting PO4–2 inside the cell (Goyer and Clarkson, 2001; Andres and Bertin, 2016). The maintenance of the energy supply or the ability to adapt to other carbon sources is essential to ensure survival of the cell. In the same context, the protein CcpA which ensures optimal energy usage under diverse conditions (Tomoyasu et al., 2010) was identified as hub protein connecting six proteins, suggesting a rapid adaptation to energy utilization and prioritization of available carbon sources in response to arsenic. It has been previously found that oxidative stresses caused by arsenic resulted in altered levels of enzymes related to carbohydrate metabolism (Shahid et al., 2014).
Overall, one of the most prominent results obtained from the proteomic analysis was the detection of the arsenic pump-driving ATPase ArsA. Being up-regulated in both As conditions for the three strains, with a great magnitude against As(III) (Figure 3). In this case, transcriptional expression of the gene that codes for this protein showed a positive correlation. ArsA protein participates in the active expulsion of the As(III) from the cell and it is part of the ars operon, which has been proposed as the main mechanism used by the bacteria belonging to the Exiguobacterium genus to tolerate the As-associated stress. Interestingly, to date, this protein has never been described in a proteomic study (Belfiore et al., 2013; Sacheti et al., 2014). Cdr, and LuxS proteins, which are related to stress (Boylan et al., 2006; Sewald et al., 2007; Gohara and Yap, 2018), are up-regulated in the presence of both As species, suggesting a relevant role in the As tolerance. ArsA and Cdr proteins are the most up-regulated proteins in response to both As conditions, although the latter does not show significant changes in the SH1S21 strain. In an opposite way PunA, A0A1G1SP02 and Fur were the only proteins mainly down-regulated under both arsenic conditions for SH31 strain. Furthermore, those three proteins were not detected in SH1S21 strain, except for Fur which was detected and down-regulated in SH0S7 strains for both As conditions. It is interesting to note that the Cdr, LuxS and Fur proteins, all associated with stress response change their expression patterns mostly in SH31 and SH0S27 strains.
In regards to correlation between transcript and protein levels, a few cases are inconsistent and some hypothesis can be advanced. For example, Hpf protein in the SH31 strain could be post-transcriptionally regulated. The TpiA protein expression varies in a manner opposite to the level of expression of the transcript whatever the studied strain. As has been reported, correlation levels are highly variable and it has been suggested that this may be dependent on different biological factors, the efficiency of transduction, the half-life of the molecules and the technology used to generate the data (de Sousa Abreu et al., 2009; Maier et al., 2011).
Bacteria from the Exiguobacterium genus are adapted to use multiple strategies that enable them to prosper under stress conditions that they face in their particular niches, specifically, the high toxicity generated by As. Mainly, those are based on the expulsion of the toxic compound, the maintenance of the redox state to avoid the oxidative stress and the repair of the unavoidable damages. They manage to do this using a great arsenal of proteins related to the protein synthesis, detoxification, generation of energy, transport and global stress. It is likely that the resistance/tolerance or response that a microorganism presents during stress conditions is originated by evolutionary adaptation to their ecologic niche. As it is observed in these three strains, they modify or supplement their basal defense mechanisms particularly against As to ensure their survival, as consequence they had different response patterns and tolerance levels. Only one protein, ArsA was regulated in the same way in the three strains independently of arsenic conditions suggesting that each strain sets up different resistance mechanisms/pathway to arsenic. This phenomenon can be further studied and explored to clarify the complex response net and molecular response to As-associated stress. Finally, we believe that the future challenges that should be embark are the generation of data bases and integration of computational/correlation methods for omics data from diverse sources with robust statistical analyses. Thus, we would be able to obtain the most of this large amount of data generated and ensure the interpretations and conclusion drawn from this are reliable, necessary for the next steps such as synthetic biology that depends on the availability of a large volume of high-quality data.
All the proteomics data for this study has been deposited in the ProteomeXchange Consortium via the PRIDE partner repository with the dataset identifier PXD014701.
JC-S, CS, FM, and LM conceived and designed the study. JC-S, FM, CS, and FR performed the field work. JC-S and LM processed the samples and performed the 2D gels experiments. MS and MD performed the mass spectrometry. JC-S, NS, YS, VG, CP-E, EC-N, and LM analyzed the data. CS, FR, EC-N, FM, and LM contributed reagents, materials, and analysis tools. JC-S, CP-E, FR, EC-N, LM, and CS wrote the manuscript. All authors read and approved the final manuscript.
This research was sponsored by the CONICYT (Comisión Nacional para la Investigación Científica y Tecnológica de Chile) grants and the Centre National de la Recherche Scientifique (CNRS-France). CS was funded by the CONICYT FONDECYT 1160315, ECOS-CONICYT 170023, and Universidad Andrés Bello Núcleo DI-3-17/N UNAB. FR was funded by the CONICYT FONDECYT 11100414. EC-N was funded by the CONICYT FONDECYT Inicio 11160905. JC-S, CP-E, YS, CC, and AB were funded by the CONICYT National Doctoral Fellowship. The funders had no role in study design, data collection and analysis, decision to publish, or preparation of the manuscript.
The authors declare that the research was conducted in the absence of any commercial or financial relationships that could be construed as a potential conflict of interest.
We would like to thank the sequencing service of Pontificia Universidad Católica de Chile that works through CONICYT – FONDEQUIP EQM150077. EC-N would like to thank Universidad Andrés Bello’s high-performance computing cluster, Dylan, for providing data storage, support, and computing power for genomic analyses. We would also like to thank the Pôle Protéome de Montpellier, specifically to the Functional Proteomic and Clinic Proteomic Platforms for the invaluable collaboration in the development of this research.
The Supplementary Material for this article can be found online at: https://www.frontiersin.org/articles/10.3389/fmicb.2019.02161/full#supplementary-material
Acosta, O., and Custodio, E. (2008). Impactos ambientales de las extracciones de agua subterránea en el salar de Huasco (norte de Chile). Bol. Geol. Min. 119, 33–50.
Albarracín, V. H., Gärtner, W., and Farias, M. E. (2016). Forged under the sun: life and art of extremophiles from Andean lakes. Photochem. Photobiol. 92, 14–28. doi: 10.1111/php.12555
Albarracín, V. H., Kurth, D., Ordoñez, O. F., Belfiore, C., Luccini, E., Salum, G. M., et al. (2015). High-Up: a remote reservoir of microbial extremophiles in Central Andean wetlands. Front. Microbiol. 6:1404. doi: 10.3389/fmicb.2015.01404
Altschul, S., Gish, W., Miller, W., Myers, E., and Lipman, D. (1990). Basic local alignment search tool. J. Mol. Biol. 215, 403–410. doi: 10.1016/S0022-2836(05)80360-2
Álvarez-Ordóñez, A., Fernández, A., Bernardo, A., and López, M. (2010). Acid tolerance in Salmonella typhimurium induced by culturing in the presence of organic acids at different growth temperatures. J. Food Microbiol. 27, 44–49. doi: 10.1016/j.fm.2009.07.015
Anderson, C., and Cook, G. (2004). Isolation and characterization of arsenate-reducing bacteria from arsenic-contaminated sites in New Zealand. Curr. Microbiol. 48, 341–347. doi: 10.1007/s00284-003-4205-3
Andreasen, R., Li, Y., Rehman, Y., Ahmed, M., Meyer, R. L., and Sabri, A. N. (2018). Prospective role of indigenous Exiguobacterium profundum PT 2 in arsenic biotransformation and biosorption by planktonic cultures and biofilms. J. Appl. Microbiol. 124, 431–443. doi: 10.1111/jam.13636
Andres, J., and Bertin, P. (2016). The microbial genomics of arsenic. FEMS Microbiol. Rev. 40, 299–322. doi: 10.1093/femsre/fuv050
Baker-Austin, C., Dopson, M., Wexler, M., Sawers, R. G., Stemmler, A., Rosen, B. P., et al. (2007). Extreme arsenic resistance by the acidophilic archaeon ‘Ferroplasma acidarmanus’ Fer1. Extremophiles 11, 425–434. doi: 10.1007/s00792-006-0052-z
Bauer, S., Grossmann, S., Vingron, M., and Robinson, P. N. (2008). Ontologizer 2.0—a multifunctional tool for GO term enrichment analysis and data exploration. Bioinformatics 24, 1650–1651. doi: 10.1093/bioinformatics/btn250
Belfiore, C., Ordonez, O. F., and Farías, M. E. (2013). Proteomic approach of adaptive response to arsenic stress in Exiguobacterium sp. S17, an extremophile strain isolated from a high-altitude Andean Lake stromatolite. Extremophiles 17, 421–431. doi: 10.1007/s00792-013-0523-y
Bhattacharjee, H., and Rosen, B. (2007). “Arsenic metabolism in prokaryotic and eukaryotic microbes,” in Molecular Microbiology of Heavy Metals, eds D. H. Nies, and S. Silver, (Heidelberg: Springer Press), 371–406. doi: 10.1007/7171_2006_086
Bissen, M., and Frimmel, F. (2003). Arsenic—a review. Part II: oxidation of arsenic and its removal in water treatment. Acta Hydrochim. Hydrobiol. 31, 97–107. doi: 10.1002/aheh.200300485
Boylan, J. A., Hummel, C. S., Benoit, S., Garcia-Lara, J., Treglown-Downey, J., Crane, E. J. III, et al. (2006). Borrelia burgdorferi bb0728 encodes a coenzyme A disulphide reductase whose function suggests a role in intracellular redox and the oxidative stress response. Mol. Microbiol. 59, 475–486. doi: 10.1111/j.1365-2958.2005.04963.x
Cai, L., Liu, G., Rensing, C., and Wang, G. (2009). Genes involved in arsenic transformation and resistance associated with different levels of arsenic-contaminated soils. BMC Microbiol. 9:4. doi: 10.1186/1471-2180-9-4
Canfora, L., Bacci, G., Pinzari, F., Papa, G. L., Dazzi, C., and Benedetti, A. (2014). Salinity and bacterial diversity: to what extent does the concentration of salt affect the bacterial community in a saline soil? PLoS One 9:e106662. doi: 10.1371/journal.pone.0106662
Cánovas, D., Cases, I., and De Lorenzo, V. (2003). Heavy metal tolerance and metal homeostasis in Pseudomonas putida as revealed by complete genome analysis. Environ. Microbiol. 5, 1242–1256. doi: 10.1111/j.1462-2920.2003.00463.x
Cárdenas, J. P., Moya, F., Covarrubias, P., Shmaryahu, A., Levicán, G., Holmes, D. S., et al. (2012). Comparative genomics of the oxidative stress response in bioleaching microorganisms. Hydrometallurgy 127, 162–167. doi: 10.1016/j.hydromet.2012.07.014
Casiano-Colón, A. I. D. A., and Marquis, R. E. (1988). Role of the arginine deiminase system in protecting oral bacteria and an enzymatic basis for acid tolerance. Appl. Environ. Microbiol. 54, 1318–1324.
Castro-Severyn, J., Remonsellez, F., Valenzuela, S. L., Salinas, C., Fortt, J., Aguilar, P., et al. (2017). Comparative genomics analysis of a new Exiguobacterium strain from Salar de Huasco reveals a repertoire of stress-related genes and arsenic resistance. Front. Microbiol. 8:456. doi: 10.3389/fmicb.2017.00456
Ceylan, S., Yilan, G., Akbulut, B. S., Poli, A., and Kazan, D. (2012). Interplay of adaptive capabilities of Halomonas sp. AAD12 under salt stress. J. Biosci. Bioeng. 114, 45–52. doi: 10.1016/j.jbiosc.2012.02.030
Ciprandi, A., Baraúna, R. A., Santos, A. V., Gonçalves, E. C., Carepo, M. S. P., Schneider, M. P. C., et al. (2012). Proteomic response to arsenic stress in Chromobacterium violaceum. J. Integ. OMICS 2, 69–73.
Cleiss-Arnold, J., Koechler, S., Proux, C., Fardeau, M. L., Dillies, M. A., Coppee, J. Y., et al. (2010). Temporal transcriptomic response during arsenic stress in Herminiimonas arsenicoxydans. BMC Genomics 11:709. doi: 10.1186/1471-2164-11-709
Collins, M., Lund, B., Farrow, J., and Schleifer, K. (1983). Chemotaxonomic study of an alkalophilic bacterium, Exiguobacterium aurantiacum gen. nov., sp. nov. J. Gen. Microbiol. 129, 2037–2042. doi: 10.1099/00221287-129-7-2037
da Costa, W. L. O., de Aragão Araújo, C. L., Dias, L. M., de Sousa Pereira, L. C., Alves, J. T. C., Araújo, F. A., et al. (2018). Functional annotation of hypothetical proteins from the Exiguobacterium antarcticum strain B7 reveals proteins involved in adaptation to extreme environments, including high arsenic resistance. PLoS One 13:e0198965. doi: 10.1371/journal.pone.0198965
Daware, V., Kesavan, S., Patil, R., Natu, A., Kumar, A., Kulkarni, M., et al. (2012). Effects of arsenite stress on growth and proteome of Klebsiella pneumoniae. J. Biotechnol. 158, 8–16. doi: 10.1016/j.jbiotec.2011.12.013
De Gregori, I., Fuentes, E., Rojas, M., Pinochet, H., and Potin-Gautier, M. (2003). Monitoring of copper, arsenic and antimony levels in agricultural soils impacted and non-impacted by mining activities, from three regions in Chile. J. Environ. Monit. 5, 287–295. doi: 10.1039/b211469k
de Sousa Abreu, R., Penalva, L. O., Marcotte, E. M., and Vogel, C. (2009). Global signatures of protein and mRNA expression levels. Mol. Biosyst. 5, 1512–1526. doi: 10.1039/b908315d
Demergasso, C. S., Guillermo, C. D., Lorena, E. G., Mur, J. J. P., and Pedrós-Alió, C. (2007). Microbial precipitation of arsenic sulfides in Andean salt flats. Geomicrobiol. J. 24, 111–123. doi: 10.1080/01490450701266605
Deutsch, E. W., Csordas, A., Sun, Z., Jarnuczak, A., Perez-Riverol, Y., Ternent, T., et al. (2016). The ProteomeXchange consortium in 2017: supporting the cultural change in proteomics public data deposition. Nucleic Acids Res. 45, D1100–D1106. doi: 10.1093/nar/gkw936
Dorador, C., Vila, I., Imhoff, J. F., and Witzel, K. P. (2008). Cyanobacterial diversity in Salar de Huasco, a high altitude saline wetland in northern Chile: an example of geographical dispersion? FEMS Microbiol. Ecol. 64, 419–432. doi: 10.1111/j.1574-6941.2008.00483.x
Dorador, C., Vila, I., Remonsellez, F., Imhoff, J. F., and Witzel, K. P. (2010). Unique clusters of Archaea in Salar de Huasco, an athalassohaline evaporitic basin of the Chilean Altiplano. FEMS Microbiol. Ecol. 73, 291–302. doi: 10.1111/j.1574-6941.2010.00891.x
Elias, M., Wellner, A., Goldin-Azulay, K., Chabriere, E., Vorholt, J. A., Erb, T. J., et al. (2012). The molecular basis of phosphate discrimination in arsenate-rich environments. Nature 491, 134–137. doi: 10.1038/nature11517
Ferreccio, C., and Sancha, A. (2006). Arsenic exposure and its impact on health in Chile. J. Health Popul. Nutr. 24, 164–175.
Flynn, H. C., Mc Mahon, V., Diaz, G. C., Demergasso, C. S., Corbisier, P., Meharg, A. A., et al. (2002). Assessment of bioavailable arsenic and copper in soils and sediments from the Antofagasta region of northern Chile. Sci. Total Environ. 286, 51–59. doi: 10.1016/s0048-9697(01)00962-7
Follmann, H., and Brownson, C. (2009). Darwin’s warm little pond revisited: from molecules to the origin of life. Naturwissenschaftem 96, 1265–1292. doi: 10.1007/s00114-009-0602-1
Frazer, B. (2012). Cancer cluster in Chile linked to arsenic contamination. World Rep. 379:603. doi: 10.1016/s0140-6736(12)60253-0
Gentleman, R. C., Carey, V. J., Bates, D. M., Bolstad, B., Dettling, M., Dudoit, S., et al. (2004). Bioconductor: open software development for computational biology and bioinformatics. Genome Biol. 5:80.
Gihring, T., Druschel, G., McCleskey, R., Hamers, R., and Banfield, J. (2001). Rapid arsenite oxidation by Thermus aquaticus and Thermus thermophilus: field and laboratory investigations. Environ. Sci. Tech. 35, 3857–3862.
Gohara, D. W., and Yap, M. N. F. (2018). Survival of the drowsiest: the hibernating 100S ribosome in bacterial stress management. Curr. Gen. 64, 753–760. doi: 10.1007/s00294-017-0796-2
Goyer, R. A., and Clarkson, T. W. (2001). “Toxic effects of metals,” in Casarett and Doull’s Toxicology: the Basic Science of Poisons, eds D. Curtis, and M. O. Amdur, (New York, NY: McGraw-Hill), 811–867.
Gutknecht, R., Beutler, R., Garcia-Alles, L. F., Baumann, U., and Erni, B. (2001). The dihydroxyacetone kinase of Escherichia coli utilizes a phosphoprotein instead of ATP as phosphoryl donor. EMBO J. 20, 2480–2486. doi: 10.1093/emboj/20.10.2480
Han, B., Runnells, T., Zimbron, J., and Wickramasinghe, R. (2002). Arsenic removal from drinking water by flocculation and microfiltration. Desalination 145, 293–298. doi: 10.1016/s0011-9164(02)00425-3
Han, Y., Zhou, D., Pang, X., Zhang, L., Song, Y., Tong, Z., et al. (2005). Comparative transcriptome analysis of Yersinia pestis in response to hyperosmotic and high-salinity stress. Res. J. Microbiol. 156, 403–415. doi: 10.1016/j.resmic.2004.10.004
Hardie, K. R., and Heurlier, K. (2008). Establishing bacterial communities by ‘word of mouth’: LuxS and autoinducer 2 in biofilm development. Nat. Rev. Microbiol. 6, 635–643. doi: 10.1038/nrmicro1916
Hassan, H. M., and Troxell, B. (2013). Transcriptional regulation by Ferric Uptake Regulator (Fur) in pathogenic bacteria. Front. Cell Infect. Microbiol. 3:59. doi: 10.3389/fcimb.2013.00059
Hernández, K. L., Yannicelli, B., Olsen, L. M., Dorador, C., Menschel, E. J., Molina, V., et al. (2016). Microbial activity response to solar radiation across contrasting environmental conditions in Salar de Huasco, Northern Chilean Altiplano. Front. Microbiol. 7:1857. doi: 10.3389/fmicb.2016.01857
Herrera, V., Amaro, A., and Carrasco, C. (2014). Speciation of Arsenic in a Saline Aquatic Ecosystem in Northern Chile. One Century of Arsenicosis in Latin America (1914-2014). Boca Raton, FL: CRC Press.
Herrera, V., De Gregori, I., and Pinochet, H. (2009). Assesment of trace elements and mobility of arsenic and manganese in lagoon sediments of the Huasco and Coposa salt flats, Chilean altiplano. J. Chil. Chem. Soc. 54, 454–459.
Hrimpeng, K., Prapagdee, B., Banjerdkij, P., Vattanaviboon, P., Dubbs, J., and Mongkolsuk, S. (2006). Challenging Xanthomonas campestris with low levels of arsenic mediates cross-protection against oxidant killing. FEMS Microbiol. Lett. 262, 121–127. doi: 10.1111/j.1574-6968.2006.00383.x
Jomova, K., Jenisova, Z., Feszterova, M., Baros, S., Liska, J., Hudecova, D., et al. (2011). Arsenic: toxicity, oxidative stress and human disease. J. Appl. Toxicol. 31, 95–107. doi: 10.1002/jat.1649
Joyce, M. A., Fraser, M. E., Brownie, E. R., James, M. N., Bridger, W. A., and Wolodko, W. T. (1999). Probing the nucleotide-binding site of Escherichia coli succinyl-CoA synthetase. Biochemistry 38, 7273–7283. doi: 10.1021/bi990527s
Kilstrup, M., Hammer, K., Ruhdal Jensen, P., and Martinussen, J. (2005). Nucleotide metabolism and its control in lactic acid bacteria. FEMS Microbiol. Rev. 29, 555–590. doi: 10.1016/j.femsre.2005.04.006
Kimbrel, J. A., Ballor, N., Wu, Y. W., David, M. M., Hazen, T. C., Simmons, B. A., et al. (2018). Microbial community structure and functional potential along a hypersaline gradient. Front. Microbiol. 9:1492. doi: 10.3389/fmicb.2018.01492
Kruger, M. C., Bertin, P. N., Heipieper, H. J., and Arsène-Ploetze, F. (2013). Bacterial metabolism of environmental arsenic—mechanisms and biotechnological applications. Appl. Microbiol. Biotechnol. 97, 3827–3841. doi: 10.1007/s00253-013-4838-5
Lara, J., González, L. E., Ferrero, M., Díaz, G. C., Pedrós-Alió, C., and Demergasso, C. (2012). Enrichment of arsenic transforming and resistant heterotrophic bacteria from sediments of two salt lakes in Northern Chile. Extremophiles 16, 523–538. doi: 10.1007/s00792-012-0452-1
Leigh, J. A., and Dodsworth, J. A. (2007). Nitrogen regulation in bacteria and archaea. Annu. Rev. Microbiol. 61, 349–377. doi: 10.1146/annurev.micro.61.080706.093409
Liu, S., Athar, M., Lippai, I., Waldren, C., and Hei, T. (2001). Induction of oxyradicals by arsenic: implication for mechanism of genotoxicity. Proc. Natl. Acad. Sci. U.S.A. 98, 1643–1648. doi: 10.1073/pnas.031482998
Maier, T., Schmidt, A., Güell, M., Kühner, S., Gavin, A. C., Aebersold, R., et al. (2011). Quantification of mRNA and protein and integration with protein turnover in a bacterium. Mol. Syst. Biol. 7:511. doi: 10.1038/msb.2011.38
Malasarn, D., Keeffe, J., and Newman, D. (2008). Characterization of the arsenate respiratory reductase from Shewanella sp. strain ANA-3. J. Bacteriol. 190, 135–142. doi: 10.1128/jb.01110-07
Mandal, B. K., and Suzuki, K. T. (2002). Arsenic round the world: a review. Talanta 58, 201–235. doi: 10.1016/s0039-9140(02)00268-0
Mateos, L. M., Villadangos, A. F., Alfonso, G., Mourenza, A., Marcos-Pascual, L., Letek, M., et al. (2017). The arsenic detoxification system in corynebacteria: basis and application for bioremediation and redox control. Adv. Appl. Microbiol. 99, 103–137. doi: 10.1016/bs.aambs.2017.01.001
Mi, H., and Thomas, P. (2009). “PANTHER pathway: an ontology-based pathway database coupled with data analysis tools,” in Protein Networks and Pathway Analysis, eds Y. Nikolsky, and J. Bryant, (Totowa, NJ: Humana Press), 123–140. doi: 10.1007/978-1-60761-175-2_7
Movahedzadeh, F., Rison, S. C. G., Wheeler, P. R., Kendall, S. L., Larson, T. J., and Stoker, N. G. (2004). The Mycobacterium tuberculosis Rv1099c gene encodes a GlpX-like class II fructose 1, 6-bisphosphatase. Microbiology 150, 3499–3505. doi: 10.1099/mic.0.27204-0
Nelson, D. L., and Cox, M. M. (2008). Lehninger: Principles of Biochemistry, eds D. L. Nelson, and M. M. Cox, (New York, NY: WH Freeman and Company), 1328.
Nies, D. H., and Silver, S. (1995). Ion efflux systems involved in bacterial metal resistances. J. Ind. Microbiol. 14, 186–199. doi: 10.1007/bf01569902
Nyström, T. (2004). MicroReview: growth versus maintenance: a trade-off dictated by RNA polymerase availability and sigma factor competition? Mol. Microbiol. 54, 855–862. doi: 10.1111/j.1365-2958.2004.04342.x
Nyunoya, H., and Lusty, C. J. (1983). The carB gene of Escherichia coli: a duplicated gene coding for the large subunit of carbamoyl-phosphate synthetase. Proc. Natl. Acad. Sci. U.S.A. 80, 4629–4633. doi: 10.1073/pnas.80.15.4629
Ohtsuka, T., Yamaguchi, N., Makino, T., Sakurai, K., Kimura, K., Kudo, K., et al. (2013). Arsenic dissolution from Japanese paddy soil by a dissimilatory arsenate-reducing bacterium Geobacter sp. OR-1. Environ. Sci. Technol. 47, 6263–6271. doi: 10.1021/es400231x
Okamura-Ikeda, K., Ohmura, Y., Fujiwara, K., and Motokawa, Y. (1993). Cloning and nucleotide sequence of the gcv operon encoding the Escherichia coli glycine-cleavage system. Eur. J. Biochem. 216, 539–548. doi: 10.1111/j.1432-1033.1993.tb18172.x
Ordoñez, O. F., Flores, M., Dib, J., Paz, A., and Farias, M. E. (2009). Extremophile culture collection from andean lakes: extreme pristine environments that host a wide diversity of microorganisms with tolerance to UV radiation. Microb. Ecol. 58, 461–473. doi: 10.1007/s00248-009-9527-7
Ordoñez, O. F., Lanzarotti, E., Kurth, D., Gorriti, M. F., Revale, S., Cortez, N., et al. (2013). Draft genome sequence of the polyextremophilic Exiguobacterium sp. strain S17, isolated from hyperarsenic lakes in the Argentinian Puna. Genome Announc. 1:e0480-13. doi: 10.1128/genomeA.00480-13
Ordoñez, O. F., Lanzarotti, E. O., Kurth, D. G., Cortez, N., Farias, M. E., and Turjanski, A. G. (2015). Genome comparison of two Exiguobacterium strains from high altitude andean lakes with different arsenic resistance: identification and 3D modeling of the Acr3 efflux pump. Front. Environ. Sci. 3:50. doi: 10.3389/fenvs.2015.00050
Pacyna, J., and Pacyna, E. (2001). An assessment of global and regional emissions of trace metals to the atmosphere from anthropogenic sources worldwide. Environ. Rev. 9, 269–298. doi: 10.1139/a01-012
Pandey, N., and Bhatt, R. (2015). Arsenic resistance and accumulation by two bacteria isolated from a natural arsenic contaminated site. J. Basic Microbiol. 55, 1275–1286. doi: 10.1002/jobm.201400723
Pandey, S., Rai, R., and Rai, L. C. (2012). Proteomics combines morphological, physiological and biochemical attributes to unravel the survival strategy of Anabaena sp. PCC7120 under arsenic stress. J. Proteom. 75, 921–937. doi: 10.1016/j.jprot.2011.10.011
Perez-Riverol, Y., Csordas, A., Bai, J., Bernal-Llinares, M., Hewapathirana, S., Kundu, D. J., et al. (2018). The PRIDE database and related tools and resources in 2019: improving support for quantification data. Nucleic Acids Res. 47, 442–450. doi: 10.1093/nar/gky1106
Petänen, T., Lyytikäinen, M., Lappalainen, J., Romantschuk, M., and Kukkonen, J. V. K. (2003). Assessing sediment toxicity and arsenite concentration with bacterial and traditional methods. Environ. Pollut. 122, 407–415. doi: 10.1016/s0269-7491(02)00307-x
Pfaffl, M. W. (2001). A new mathematical model for relative quantification in real-time RT–PCR. Nucleic Acids Res. 29:45. doi: 10.1093/nar/29.9.e45
Philips, S., and Taylor, M. (1976). Oxidation of arsenite to arsenate by Alcaligenes faecalis. Appl. Environ. Microbiol. 32, 392–399.
Poirel, J., Joulian, C., Leyval, C., and Billard, P. (2013). Arsenite-induced changes in abundance and expression of arsenite transporter and arsenite oxidase genes of a soil microbial community. Res. J. Microbiol. 164, 457–465. doi: 10.1016/j.resmic.2013.01.012
Qin, J., Rosen, B., Zhang, Y., Wang, G., Franke, S., and Rensing, C. (2006). Arsenic detoxification and evolution of trimethylarsine gas by a microbial arsenite S-adenosylmethionine methyltransferase. Proc. Natl. Acad. Sci. U.S.A. 103, 2075–2080. doi: 10.1073/pnas.0506836103
Remonsellez, F., Castro-Severyn, J., Aguilar Espinosa, P. M., Fortt, J., Salinas, C., Barahona, S., et al. (2018). Characterization and salt response in recurrent halotolerant Exiguobacterium sp. SH31 isolated from sediments of Salar de Huasco, Chilean Altiplano. Front. Microbiol. 9:2228. doi: 10.3389/fmicb.2018.02228
Risacher, F., and Fritz, B. (2009). Origin of salts and brine evolution of Bolivian and Chilean salars. Aquat. Geochem. 15, 123–157. doi: 10.1007/s10498-008-9056-x
Ritchie, M. E., Phipson, B., Wu, D., Hu, Y., Law, C. W., Shi, W., et al. (2015). limma powers differential expression analyses for RNA-sequencing and microarray studies. Nucleic Acids Res. 43:e47. doi: 10.1093/nar/gkv007
Roh, T., Steinmaus, C., Marshall, G., Ferreccio, C., Liaw, J., and Smith, A. H. (2018). Age at exposure to arsenic in water and mortality 30–40 years after exposure cessation. Am. J. Epidemiol. 187, 2297–2305. doi: 10.1093/aje/kwy159
Sacheti, P., Patil, R., Dube, A., Bhonsle, H., Thombre, D., Marathe, S., et al. (2014). Proteomics of arsenic stress in the gram-positive organism Exiguobacterium sp. PS NCIM 5463. Appl. Microbiol. Biotechnol. 98, 6761–6773. doi: 10.1007/s00253-014-5873-6
Schneider, C. A., Rasband, W. S., and Eliceiri, K. W. (2012). NIH Image to ImageJ: 25 years of image analysis. Nat. Methods 9, 671–675. doi: 10.1038/nmeth.2089
Schnell, R., Sriram, D., and Schneider, G. (2015). Pyridoxal-phosphate dependent mycobacterial cysteine synthases: structure, mechanism and potential as drug targets. Biochim. Biophys. Acta 1854, 1175–1183. doi: 10.1016/j.bbapap.2014.11.010
Sewald, X., Saum, S. H., Palm, P., Pfeiffer, F., Oesterhelt, D., and Müller, V. (2007). Autoinducer-2-producing protein LuxS, a novel salt-and chloride-induced protein in the moderately halophilic bacterium Halobacillus halophilus. Appl. Environ. Microbiol. 73, 371–379. doi: 10.1128/aem.01625-06
Shahid, F., Rizwan, S., Khan, M. W., Khan, S. A., Naqshbandi, A., and Yusufi, A. N. K. (2014). Studies on the effect of sodium arsenate on the enzymes of carbohydrate metabolism, brush border membrane, and oxidative stress in the rat kidney. Environ. Toxicol. Pharmacol. 37, 592–599. doi: 10.1016/j.etap.2014.01.012
Shannon, P., Markiel, A., Ozier, O., Baliga, N. S., Wang, J. T., Ramage, D., et al. (2003). Cytoscape: a software environment for integrated models of biomolecular interaction networks. Genome Res. 13, 2498–2504. doi: 10.1101/gr.1239303
Slyemi, D., and Bonnefoy, V. (2012). How prokaryotes deal with arsenic. Environ. Microbiol. Rep. 4, 571–586. doi: 10.1111/j.1758-2229.2011.00300.x
Smith, A., Goycolea, M., Haque, R., and Biggs, M. (1998). Marked increase in bladder and lung cancer mortality in a region of Northern Chile due to arsenic in drinking water. Am. J. Epidemiol. 147, 660–669. doi: 10.1093/oxfordjournals.aje.a009507
Srivastava, S., Verma, P. C., Singh, A., Mishra, M., Singh, N., Sharma, N., et al. (2012). Isolation and characterization of Staphylococcus sp. strain NBRIEAG-8 from arsenic contaminated site of West Bengal. Appl. Microbiol. Biotechnol. 95, 1275–1291. doi: 10.1007/s00253-012-3976-5
Sunita, M. S. L., Prashant, S., Chari, P. B., Rao, S. N., Balaravi, P., and Kishor, P. K. (2012). Molecular identification of arsenic-resistant estuarine bacteria and characterization of their ars genotype. Ecotoxicology 21, 202–212. doi: 10.1007/s10646-011-0779-x
Takebe, F., Hara, I., Matsuyama, H., and Yumoto, I. (2007). Effect of H2O2 under low- and high-aeration-level conditions on growth and catalase activity in Exiguobacterium oxidotolerans T-2-2T. J. Biosci. Bioeng. 104, 464–469. doi: 10.1263/jbb.104.464
Tapia, J., Davenport, J., Townley, B., Dorador, C., Schneider, B., Tolorza, V., et al. (2018). Sources, enrichment, and redistribution of As, Cd, Cu, Li, Mo, and Sb in the Northern Atacama Region, Chile: implications for arid watersheds affected by mining. J. Geochem. Explor. 185, 33–51. doi: 10.1016/j.gexplo.2017.10.021
Tomoyasu, T., Tabata, A., Hiroshima, R., Imaki, H., Masuda, S., Whiley, R. A., et al. (2010). Role of catabolite control protein A in the regulation of intermedilysin production by Streptococcus intermedius. Infect. Immun. 78, 4012–4021. doi: 10.1128/IAI.00113-10
Trujillo, C., Blumenthal, A., Marrero, J., Rhee, K. Y., Schnappinger, D., and Ehrt, S. (2014). Triosephosphate isomerase is dispensable in vitro yet essential for Mycobacterium tuberculosis to establish infection. mBio 5, e00085–14. doi: 10.1128/mBio.00085-14
Uribe, J., Muñoz, J. F., Gironás, J., Oyarzún, R., Aguirre, E., and Aravena, R. (2015). Assessing groundwater recharge in an Andean closed basin using isotopic characterization and a rainfall-runoff model: Salar del Huasco basin, Chile. Hydrogeol. J. 32, 1535–1551. doi: 10.1007/s10040-015-1300-z
Vishnivetskaya, T. A., and Kathariou, S. (2005). Putative transposases conserved in Exiguobacterium isolates from ancient Siberian permafrost and from contemporary surface habitats. Appl. Environ. Microbiol. 71, 6954–6962. doi: 10.1128/AEM.71.11.6954-6962.2005
Vishnivetskaya, T. A., Kathariou, S., and Tiedje, J. M. (2009). The Exiguobacterium genus: biodiversity and biogeography. Extremophiles 13, 541–555. doi: 10.1007/s00792-009-0243-5
Wei, T., and Simko, V. (2017). R package “corrplot”: Visualization of a correlation matrix. Version 0.84. Available at: https://github.com/taiyun/corrplot
Weiss, S., Carapito, C., Cleiss, J., Koechler, S., Turlin, E., Coppee, J.-Y., et al. (2009). Enhanced structural and functional genome elucidation of the arsenite-oxidizing strain Herminiimonas arsenicoxydans by proteomics data. Biochimie 91, 192–203. doi: 10.1016/j.biochi.2008.07.013
Xiao, K., Li, L., Ma, L., Zhang, S., Bao, P., Zhang, T., et al. (2016). Metagenomic analysis revealed highly diverse microbial arsenic metabolism genes in paddy soils with low-arsenic contents. Environ. Pollut. 211, 1–8. doi: 10.1016/j.envpol.2015.12.023
Xie, F., Li, G., Wang, Y., Zhang, Y., Zhou, L., Wang, C., et al. (2017). Pyridoxal phosphate synthases PdxS/PdxT are required for Actinobacillus pleuropneumoniae viability, stress tolerance and virulence. PLoS One 12:e0176374. doi: 10.1371/journal.pone.0176374
Zhang, J., Cao, T., Tang, Z., Shen, Q., Rosen, B. P., and Zhao, F.-J. (2015). Arsenic methylation and volatilization by arsenite S-adenosylmethionine methyltransferase in Pseudomonas alcaligenes NBRC14159. Appl. Environ. Microbiol. 81, 2852–2860. doi: 10.1128/AEM.03804-14
Zhang, Y., Chen, S., Hao, X., Su, J.-Q., Xue, X., Yan, Y., et al. (2016). Transcriptomic analysis reveals adaptive responses of an Enterobacteriaceae strain LSJC7 to arsenic exposure. Front. Microbiol. 7:636. doi: 10.3389/fmicb.2016.00636
Keywords: Exiguobacterium, arsenic, tolerance, proteomic, polyextremophile
Citation: Castro-Severyn J, Pardo-Esté C, Sulbaran Y, Cabezas C, Gariazzo V, Briones A, Morales N, Séveno M, Decourcelle M, Salvetat N, Remonsellez F, Castro-Nallar E, Molina F, Molina L and Saavedra CP (2019) Arsenic Response of Three Altiplanic Exiguobacterium Strains With Different Tolerance Levels Against the Metalloid Species: A Proteomics Study. Front. Microbiol. 10:2161. doi: 10.3389/fmicb.2019.02161
Received: 07 June 2019; Accepted: 03 September 2019;
Published: 26 September 2019.
Edited by:
Jesse G. Dillon, California State University, Long Beach, United StatesReviewed by:
Michal Letek, University of Roehampton, United KingdomCopyright © 2019 Castro-Severyn, Pardo-Esté, Sulbaran, Cabezas, Gariazzo, Briones, Morales, Séveno, Decourcelle, Salvetat, Remonsellez, Castro-Nallar, Molina, Molina and Saavedra. This is an open-access article distributed under the terms of the Creative Commons Attribution License (CC BY). The use, distribution or reproduction in other forums is permitted, provided the original author(s) and the copyright owner(s) are credited and that the original publication in this journal is cited, in accordance with accepted academic practice. No use, distribution or reproduction is permitted which does not comply with these terms.
*Correspondence: Claudia P. Saavedra, Y3NhYXZlZHJhQHVuYWIuY2w=
Disclaimer: All claims expressed in this article are solely those of the authors and do not necessarily represent those of their affiliated organizations, or those of the publisher, the editors and the reviewers. Any product that may be evaluated in this article or claim that may be made by its manufacturer is not guaranteed or endorsed by the publisher.
Research integrity at Frontiers
Learn more about the work of our research integrity team to safeguard the quality of each article we publish.