- 1Department of Chemical and Process Engineering, University of Canterbury, Christchurch, New Zealand
- 2Scion, Te Papa Tipu Innovation Park, Rotorua, New Zealand
- 3Geomicrobiology Research Group, Department of Geothermal Sciences, GNS Science, Taupō, New Zealand
- 4School of Biological Sciences, Monash University, Clayton, VIC, Australia
- 5School of Biological Sciences, University of Canterbury, Christchurch, New Zealand
Metabolic flexibility in aerobic methane oxidizing bacteria (methanotrophs) enhances cell growth and survival in instances where resources are variable or limiting. Examples include the production of intracellular compounds (such as glycogen or polyhydroxyalkanoates) in response to unbalanced growth conditions and the use of some energy substrates, besides methane, when available. Indeed, recent studies show that verrucomicrobial methanotrophs can grow mixotrophically through oxidation of hydrogen and methane gases via respiratory membrane-bound group 1d [NiFe] hydrogenases and methane monooxygenases, respectively. Hydrogen metabolism is particularly important for adaptation to methane and oxygen limitation, suggesting this metabolic flexibility may confer growth and survival advantages. In this work, we provide evidence that, in adopting a mixotrophic growth strategy, the thermoacidophilic methanotroph, Methylacidiphilum sp. RTK17.1 changes its growth rate, biomass yields and the production of intracellular glycogen reservoirs. Under nitrogen-fixing conditions, removal of hydrogen from the feed-gas resulted in a 14% reduction in observed growth rates and a 144% increase in cellular glycogen content. Concomitant with increases in glycogen content, the total protein content of biomass decreased following the removal of hydrogen. Transcriptome analysis of Methylacidiphilum sp. RTK17.1 revealed a 3.5-fold upregulation of the Group 1d [NiFe] hydrogenase in response to oxygen limitation and a 4-fold upregulation of nitrogenase encoding genes (nifHDKENX) in response to nitrogen limitation. Genes associated with glycogen synthesis and degradation were expressed constitutively and did not display evidence of transcriptional regulation. Collectively these data further challenge the belief that hydrogen metabolism in methanotrophic bacteria is primarily associated with energy conservation during nitrogen fixation and suggests its utilization provides a competitive growth advantage within hypoxic habitats.
Introduction
Aerobic methane oxidizing bacteria (methanotrophs) serve as the primary biological sink for the potent greenhouse gas methane (CH4) (Kirschke et al., 2013). Methanotrophs grow by oxidizing CH4 to methanol with a particulate or soluble methane monooxygenase enzyme (pMMO/sMMO) and subsequently yield reducing equivalents (e.g., NADH) for cellular respiration and biosynthesis through the oxidation of methanol to carbon dioxide (CO2). The gammaproteobacterial (Type I) and alphaproteobacterial (Type II) methanotrophs generate biomass by assimilating the intermediates formaldehyde or formate via the ribulose monophosphate (RuMp) or serine pathways (Hanson and Hanson, 1996) respectively, whereas the verrucomicrobial methanotrophs oxidize methanol directly to formate (Keltjens et al., 2014) and generate biomass by fixing inorganic carbon (CO2) via the Calvin–Benson–Bassham cycle (Khadem et al., 2012b). Despite the apparent restriction of most methanotrophs to grow on one carbon compounds (C1), they thrive at the interface of various oxic/anoxic habitats (e.g., peat bogs, forest soils, wetlands, rice paddies and geothermal environments) (Dunfield et al., 2007; Singh et al., 2010; Knief, 2015), where the availability of oxidant (O2), energy and carbon resources for growth is likely to fluctuate. Given the methane monooxygenase reaction (CH4 + O2 + [NAD(P)H + H+]/QH2 CH3OH + NAD(P)+/Q + H2O) and the aerobic respiratory chain require a continual source of reductant and oxidant, methanotrophic bacteria must regulate their carbon, energy and resource allocation to fulfill metabolic demands for cellular growth and persistence (Hanson and Hanson, 1996).
Many bacterial species, including methanotrophs, accumulate biopolymers (e.g., glycogen, polyhydroxyalkanoates), phospholipids, and intracellular osmolytes (e.g., ectoine, sucrose) (Strong et al., 2016) in response to unbalanced growth conditions. This allows resources to be strategically conserved for assistance in times of starvation. The biosynthesis of glycogen, a highly branched polysaccharide consisting of α-1,4 bonded glucose residues with additional α-1,6 branched sidechains, is a common metabolic strategy for carbon storage that is shared among evolutionarily distant species (Wilson et al., 2010). Glycogen production has been widely described within Type I methanotroph species (Linton and Cripps, 1978; Eshinimaev et al., 2002) and the production of this compound has recently been reported in the verrucomicrobial methanotroph, Methylacidiphilum fumarolicum SolV (Khadem et al., 2012a). The physiological role of glycogen production in methanotrophs is not precisely understood, although it is believed to serve a role in environmental survival during periods of starvation and has been implicated to symbiotic performance, colonization and virulence (Bonafonte et al., 2000; McMeechan et al., 2005; Bourassa and Camilli, 2009; Wilson et al., 2010). Although the accumulation of intracellular glycogen may occur optimally during exponential growth (Gibbons and Kapsimalis, 1963; Eidels and Preiss, 1970), its synthesis is typically associated with entry into stationary phase when growth is limited due to the limitation of some critical nutrient (i.e., nitrogen, phosphate) or in the presence of excess carbon (Wilson et al., 2010). In bacteria, the biosynthesis of glycogen occurs by utilizing ADP-glucose as the glycosyl donor for polymer extension (Preiss, 1984). The precise mechanisms governing glycogen biosynthesis in bacteria, however, remain obscure. It is likely energy availability and redox status play a primary role in regulating glycogen biosynthesis, as ATP acts as substrate for the ADP-glucose producing reaction catalyzed by glucose-1-phosphate adenylyltransferase (Preiss, 1984).
To remain competitive within dynamic environments (Knief et al., 2003; Tavormina et al., 2010), some methanotrophs supplement CH4 usage with other energy-yielding strategies (Dedysh and Dunfield, 2010). Several recent studies have revealed a few strains, notably Methylocella silvestris, utilize a suite of carbon and energy substrates, including simple organic acids, alcohols and short-chain alkane gases (Dedysh et al., 2005; Crombie and Murrell, 2014). Aerobic H2 metabolism has also been shown in a range of methanotrophs (Chen and Yoch, 1987; Shah et al., 1995; Hanczar et al., 2002) and a wide range of hydrogenases have been shown to be distributed in methanotroph genomes (Greening et al., 2016). While H2 oxidation was originally implicated in energy conservation in response to N2 fixation (Takeda, 1988), more recent findings indicate that H2 serves a multifaceted role in the growth and survival of these bacteria. Of the verrucomicrobial methanotrophs, the activity of respiratory-linked group 1d hydrogenases can provide sufficient energy to sustain chemolithoautotrophic growth on H2 alone (Mohammadi et al., 2016; Carere et al., 2017). Further, mixotrophic growth (H2 and CH4) in the thermoacidophile Methylacidiphilum sp. RTK17.1 has been observed under O2-limiting conditions and is proposed to provide a competitive advantage over obligate methanotrophy at oxic/anoxic soil boundaries within geothermal environments (Carere et al., 2017). This suggests that the additional energetic input of H2 may counter the effect of otherwise unbalanced growth conditions.
The influence of H2 metabolism on the production of intracellular energy reservoirs, commonly associated with unbalanced growth, within methanotrophic bacteria has yet to be elucidated. In this work, we investigate the effect of H2 metabolism on glycogen production within the methanotroph, Methylacidiphilum sp. RTK17.1. Chemostat cultivation was performed during O2-replete and O2-limited cultivation, in the presence of NH4+ or N2, with or without H2 in the headspace, to determine the influence of H2 metabolism on observed growth rates, biomass production characteristics and transcriptional regulation. We show that cellular growth rates, molar growth yields, and the allocation of resources between protein and glycogen production vary depending on the supply of H2, O2, and nitrogen (as NH4+ or N2). Transcriptome data provided a basis of findings, showing significant differential regulation of operons encoding the group 1d [NiFe]-hydrogenase, methane monooxygenases, and nitrogenase between the conditions. In turn, these findings enhance understanding of the physiological strategies that methanotrophs use to grow and survive in different environments.
Materials and Methods
Chemostat Cultivation of Methylacidiphilum sp. RTK17.1
Chemostat cultivation was performed to investigate the influence of H2 metabolism on the growth and production of intracellular glycogen reservoirs in Methylacidiphilum sp. RTK17.1 with respect to unbalanced growth conditions (O2 and nitrogen limitation). As previously described, a 1 l bioreactor (BioFlo 110; New Brunswick Scientific, Edison, NJ, United States) equipped with an InPro 6810 Polarographic Oxygen Sensor (Mettler-Toledo, Columbus, OH, United States) was used for all cultivations (Carere et al., 2017). Cultures were continuously incubated at pH 2.5, 50°C with stirring (800 rpm). The reactor was constantly maintained at a volume of 0.5 l with V4 mineral medium (Carere et al., 2017), prepared with or without NH4Cl (0.4 g l–1) addition (as necessary), and supplied at a constant flow rate of 10 ml h–1 (D = 0.02 h–1). Custom gas mixtures were supplied to the chemostat at a rate of 10 ml min–1 and contained approximately (v/v) 3% CH4 and 26% CO2 for all experiments; O2 at (v/v) 14.1% and 3.5%, respectively for O2-replete and -limiting conditions, and H2 at 0.4% (v/v). The balance of all gas mixtures was made up with N2.
Cell densities were monitored at 600 nm using a Ultrospec 10 cell density meter (Amersham Bioscience, United Kingdom) with one unit of OD600 equivalent to 0.43 g l–1 cell dry weight for Methylacidiphilum sp. RTK17.1. Influent and effluent gas concentrations were monitored using a 490 micro GC equipped with a thermal conductivity detector (Agilent Technologies, United States). After achieving a steady-state condition as determined by OD600, gas concentrations were monitored over several days and used as a basis to calculate growth and specific gas consumption rates. Biomass samples of Methylacidiphilum sp. RTK17.1 were harvested during steady-state operation for subsequent transcriptome sequencing, biomass cell dry weight determinations, intracellular glycogen, total protein and amino acid levels measurements.
Transcriptome Sequencing
Cell culture samples for transcriptome sequencing were harvested (10 ml) from steady-state chemostat experiments, pelleted by centrifugation at 5,000 x g (15 min, 4°C), suspended in 1 ml RNAlater Stabilization solution (Thermo Fisher Scientific) and then stored at −80°C until required for further analysis, as per the manufacturer’s recommended protocols. The extraction and sequencing of RNA was performed by Macrogen Inc. (Seoul, Korea). Briefly, isolation of mRNA was performed using the RNeasy Mini kit (Qiagen) according to the manufacturer’s protocol. Following total RNA extraction, ribosomal RNAs were removed using the Ribo-Zero rRNA removal kit (bacteria) and the quality of the remaining RNA was assessed using an Agilent 2100 Bioanalyzer (Agilent). Library construction was performed using the TruSeq Stranded Total RNA Sample Prep (microbe) Kit (Illumina) and sequencing was performed using an Illumina HiSeq2500 platform. From this, an average of 7,988,451 raw untrimmed reads were obtained for each of the five conditions sampled. These reads were then analyzed using the Artificial Intelligence RNA-Seq pipeline (Sequentia Biotech, Barcelona, Spain), as described elsewhere (Vara et al., 2019), which were reduced to an average of 7,287,318 following quality filtering and trimming. Retained paired-end reads (100 bp) were then mapped to the genome of Methylacidiphilum infernorum strain V4 (GCA_000019665.1) (Hou et al., 2008) using the ‘different genotype’ setting. An average of 80.01% reads were mapped to genes within the reference genome for the five experimental conditions (condition 1: O2 limiting, N2, no H2 addition; condition 2: O2 limiting, N2, H2 addition; condition 3: O2 limiting, NH4+, H2 addition; condition 4: O2 replete, NH4+, H2 addition; condition 5: O2 replete, NH4+, no H2 addition). Following this, differential gene expression profiles and accompanying statistical analysis was performed to investigate regulation using the edgeR (Robinson et al., 2010) tool available within the Artificial Intelligence RNA-Seq pipeline. Synonymous conditions were grouped as replicates for differential gene expression analysis during oxygen limiting (conditions 1, 2, and 3) and oxygen excess (conditions 4 and 5) growth. Likewise, conditions were grouped as replicates for differential gene expression analysis under nitrogen fixing (N2; conditions 1 and 2) and nitrogen excess (NH4+; conditions 3, 4, and 5) growth conditions, respectively. Where provided, expression values are given as FPKM (Fragments per Kilobase Million; Supplementary Table S1) (Mortazavi et al., 2008). Raw and processed transcriptome sequence files (accession numbers GSM3872525-GSM3872529) were subsequently deposited into the Gene Expression Omnibus (GEO1) for archival storage.
Characterization of Biomass
Effluent biomass, produced during experimental steady-state chemostat operation, was collected and stored at 4°C over a period of 7 days for all biomass characterization studies. Following collection of approximately 2 l culture, cells were pelleted (5,000 × g, 20 min, 4°C) and stored at −20°C until required. Characterization of Methylacidiphilum sp. RTK17.1 biomass (crude protein, ash content, amino acid composition) was performed at the Massey University Nutrition Laboratory (accredited to ISO 17025; New Zealand) according to the official methods of analysis of the Association of Official Analytical Communities (AOAC, 2005) international. Briefly, total crude protein and ash content (% w/w) were determined via the Dumas method (AOAC method 968.06) (Ebeling, 1968) and furnace methods, respectively (AOAC method 942.05) (Thiex et al., 2012). Amino acid profile determination of acid-stable residues was performed via reverse-phase high performance liquid chromatography (HPLC) separation using AccQ derivatization of biomass (60–140 mg) samples following oxidization with performic acid and hydrolysis with hydrochloric acid as described in AOAC method 994.12 (AOAC, 2005).
The concentration of glycogen within crude cell extracts of Methylacidiphilum sp. RTK17.1 was determined using a Dionex ICS 3000 HPLC equipped with a Biorad Aminex HPX-87H column and a Shodex RI-101 refractive index detector. Triplicate cell pellets, dried to constant weight (5–15 mg), were suspended in 1M NaOH (0.9 ml) and disrupted by boiling lysis for 1 h within sealed screw top Micro Tubes (Thermo Fisher Scientific). The efficacy (>99%) of cell lysis was confirmed microscopically. Crude extracts were then incubated with amyloglucosidase (35 U/ml crude) from Aspergillus niger (Sigma-Aldrich), following acidification with acetate buffer (0.1 ml) to pH 4.8, for 8 h at 45°C to convert intracellular glycogen reservoirs into glucose. Following this, the resulting glucose was quantified by HPLC and normalized against a standard curve of known glycogen content (Sigma) that had been contemporaneously treated with amyloglucosidase. To account for non-glycogen derived glucose in cell samples, extracts that did not undergo amyloglucosidase treatment were similarly analyzed by HPLC in parallel. Total intracellular glycogen content was then expressed as a function of cell dry weight (% w/w). Observed differences in growth and biomass characteristics were analyzed for statistical significance using the Tukey’s honest significance test tool available in Prism v7.0a (Graphpad Software, Inc.).
Results
Hydrogen Availability Differentially Affects Growth and Biomass Allocation Depending on Oxygen and Nitrogen Availability
The growth characteristics and biomass production of Methylacidiphilum sp. RTK17.1 was compared in chemostats in four different conditions that differed in O2, nitrogen (NH4+/N2), and H2 supply. Results from these experiments are provided in Table 1.
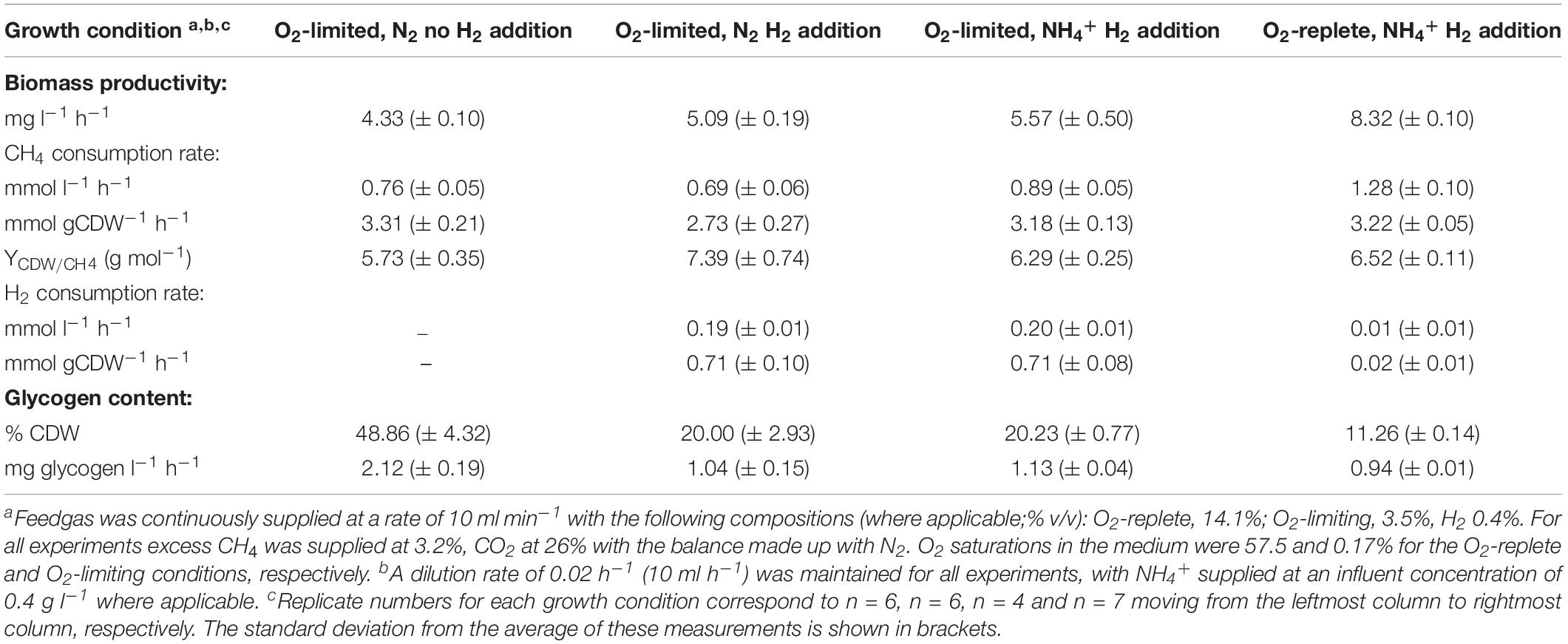
Table 1. Growth and productivity characteristics of Methylacidiphilum sp. RTK17.1 during chemostat cultivation.
Under nitrogen-replete conditions (i.e., NH4+ present in the medium), Methylacidiphilum sp. RTK17.1 displayed many of the same growth characteristics in response to O2 availability as found previously (Carere et al., 2017) and results were consistent with similar studies on M. fumarolicum SolV (Khadem et al., 2012a). The observed rate of steady-state biomass production (mg l–1h–1) of Methylacidiphilum sp. RTK17.1) decreased by 33.1% following the transition from O2-replete to O2-limiting cultivation (p-value: <0.0001). This decrease in growth rate was accompanied by a 30.4% reduction in the volumetric rate (mmol l–1 h–1) of CH4 consumption and a 19-fold increase in the observed rate of H2 oxidation (p-value: <0.0001). With respect to specific gas consumption rates normalized against biomass production (mmol gCDW–1 h–1), observed rates of CH4 consumption did not change significantly (p-value: 0.988), whereas H2 consumption increased 36-fold (p-value: <0.0001). Intracellular glycogen content was observed to increase dramatically from 11.26 (± 0.14)% during O2-replete growth to 20.23 (± 0.77)% (w/w; p-value: < 0.0001) during O2-limiting cultivation.
We observed significant changes in these parameters when Methylacidiphilum sp. RTK17.1 was cultivated under O2-limitation depending on H2 and nitrogen supply. In the presence of H2, observed rates of Methylacidiphilum sp. RTK17.1 biomass production decreased by 8.61% (p-value: 0.019) during N2-fixation compared to growth on NH4+ replete media. In comparison, removal of H2 from the headspace resulted in an 18.67% reduction in the observed rate of biomass production compared to cells cultured in NH4+-replete media (from 5.57 to 4.33 mg l–1 h–1; Table 1; p-value: < 0.0001). These results suggest the activity of respiratory-linked aerobic H2 oxidation may, at least partially, serve to offset the energetic demand imposed by N2 fixation. In support of this, onset of nitrogen limitation in cultures actively respiring H2 did not significantly increase the production of intracellular glycogen (p-value: 0.999). Following the removal of H2 from the reactor feedgas, however, internal glycogen content significantly increased from 20.00 (± 2.93)% to 48.86 (± 4.32)% (w/w; p-value: < 0.0001). This corresponds to a 2.03 fold increase in the volumetric production rate of glycogen (Table 1). In the absence of CH4, at 4°C, cellular glycogen reservoirs were depleted within 100 days (Supplementary Figure S1).
The total protein and amino acid content of Methylacidiphilum sp. RTK17.1 biomass produced during steady-state growth was also determined (Figure 1). A maximum total protein content of 53.9 (± 2.69)% (w/w) was achieved during growth under O2-replete conditions with NH4+ as a readily available source of nitrogen. Compared to O2-replete growth, a minor but insignificant decrease in total protein content of biomass coincided with reduced O2 availability (51.2 ± 2.56%), and in the transition from NH4+ to N2 as a source of nitrogen (51.9 ± 2.56%). Consistent with the observation that glycogen production increased in the absence of H2 (under O2-limiting, N2-fixing growth conditions), the least total protein content of biomass (42.5 ± 8.94%) was observed in conditions where energy availability is likely to constrain cell growth (i.e., O2-limiting, N2-fixing, no H2; p-value: 0.018; Figure 1A). Observed changes in the concentration of specific amino acid residues under all of the growth conditions were generally consistent with the changes associated with total protein determinations (Figure 1C). For each of the experimental conditions, glutamic acid, leucine, aspartic acid, lysine and alanine were the amino acids in greatest abundance whereas methionine, histidine and cysteine were the least abundant residues.
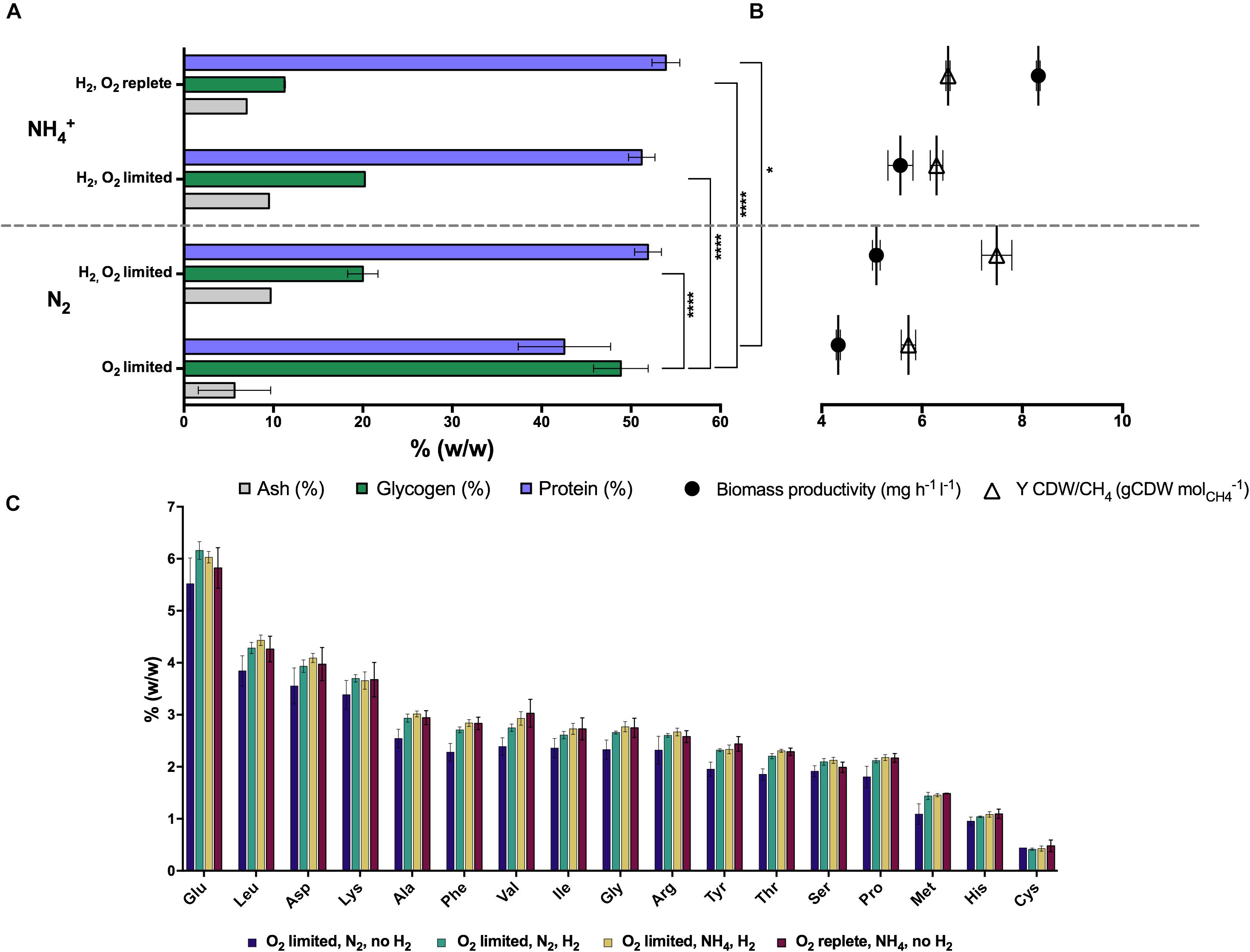
Figure 1. The production of intracellular glycogen in chemostat grown cultures of Methylacidiphilum sp. RTK17.1 is influenced by O2 availability, and H2 and nitrogen metabolism. (A) Total protein, ash and glycogen content of cells, (B) observed growth rates and biomass yields (CDW: cell dry weight), and (C) amino acid content profiles are shown relative to total biomass. For all chemostat growth conditions, Methylacidiphilum sp. RTK17.1 excess CH4 was continuously supplied (3% v/v, 10 ml min– 1). Displayed values represent the average of minimum triplicate samples, with error bars illustrating the standard deviation. Significant differences in cellular glycogen and protein content are shown next to squared brackets (****p-value < 0.0001, *p-value < 0.05).
Transcriptome Analysis Reveals Changes in Hydrogenase, Nitrogenase, and Methane Monooxygenase Expression Between the Conditions
Transcriptome analysis was performed on chemostat grown cultures of Methylacidiphilum sp. RTK17.1 to determine whether genes associated with energy metabolism (CH4 or H2), glycogen synthesis, N2 or CO2 fixation were regulated in response to O2 or nitrogen availability (Figure 2). For these experiments, to resolve the possible influence of H2 on transcriptional responses, a fifth chemostat condition (condition 5: O2 replete, NH4+, no H2 addition) was added to the four growth conditions described in Table 1. In response to O2 limitation, 36 genes were identified as significantly upregulated (p-value: <0.001; False discovery rate (FDR): < 0.05) and 36 genes were significantly downregulated (p-value: < 0.001; FDR: < 0.05). Subunits of the particulate methane monooxygenase operon (pmoCAB1), corresponding to M. infernorum V4 loci Minf_1509–1511, displayed the greatest degree of transcriptional upregulation (average: 9.8 Log2FC, p-value: < 0.0001) in response to O2 limitation. In contrast, the closely related and immediately proximal pmoCAB2 operon (homologous to M. infernorum V4 loci Minf_1506–1508) that was highly expressed during O2 replete growth, was strongly downregulated during O2-limited growth (average: −4.0 Log2FC, p-value: < 0.0001). Interestingly, transcripts for a third putative and relatively divergent pmoCAB3 operon were not detected under the experimental conditions tested.
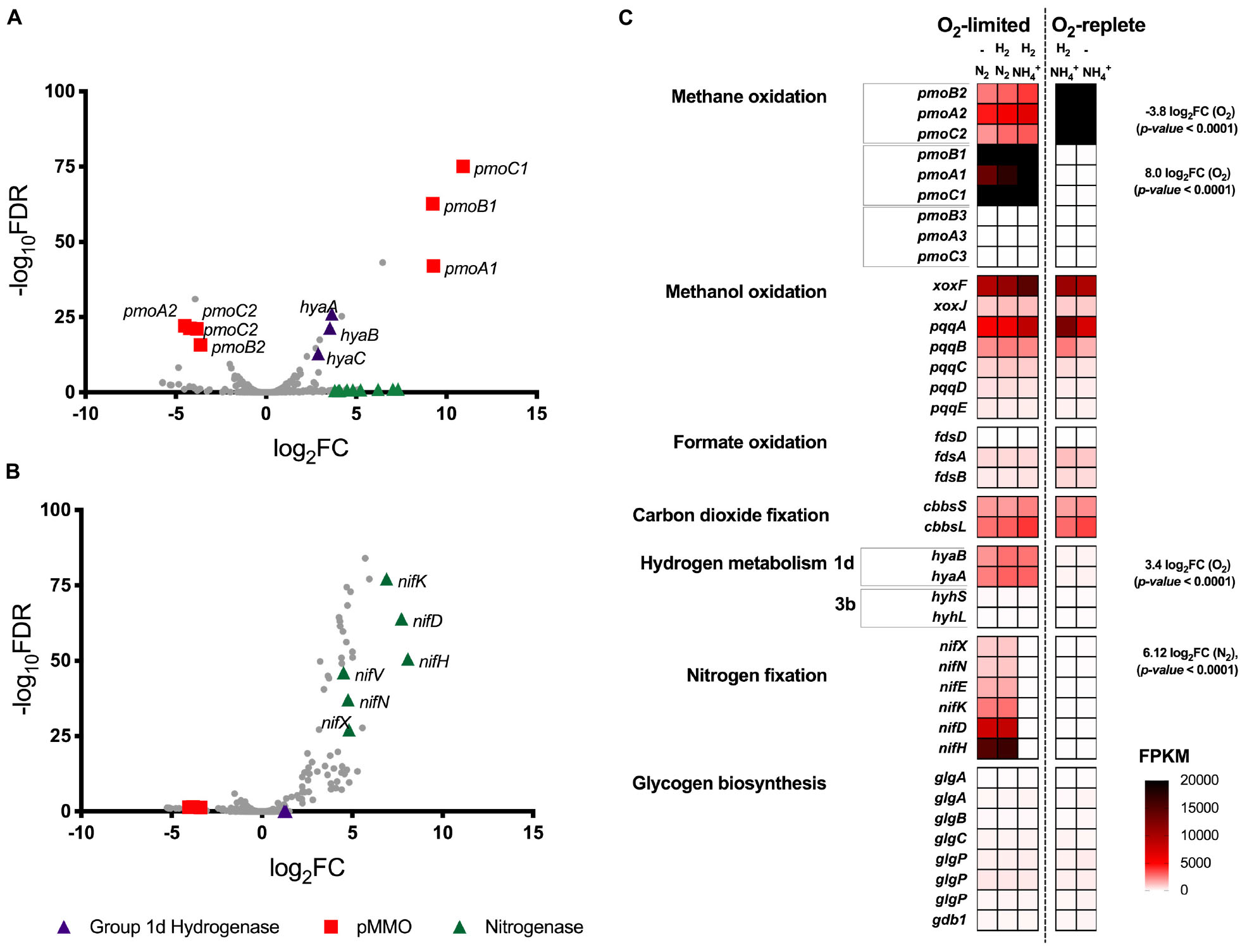
Figure 2. Differential gene expression profiles of chemostat-grown cultures of Methylacidiphilum sp. RTK17.1 grown under O2-replete and O2-limiting conditions in the presence of ammonium (NH4+) or under N2-fixing conditions. (A) Volcano plot showing differential gene expression changes following the transition from oxygen replete to O2-limited growth. (B) Volcano plot showing differential gene expression changes following the transition from nitrogen-replete (NH4+) to N2-fixing growth conditions. Both volcano plots compare data generated from the same five transcriptomes with fold-change values (log2FC) and false discovery rates (FDR) calculated using O2-replete and nitrogen excess as reference conditions, respectively. Each gene is represented by a gray dot and genes of interest are highlighted as per the legend. (C) Heat map of transcript abundance for key genes encoding the structural subunits of enzymes participating in methane oxidation (pmoBAC; particulate methane monooxygenase), methanol oxidation (xoxFJ; methanol dehydrogenase, pqqABCDE; pyrroloquinoline biosynthesis), formate oxidation (fdsDAB; formate dehydrogenase), carbon-dioxide fixation (cbbsSL; Rubisco), hydrogen metabolism (hyaBA and hyhSL; encoding group 1d and 3b [NiFe]-hydrogenases respectively), nitrogen fixation (nifXNEKDH; nitrogenase), and glycogen biosynthesis (glgAABCPPP1). The fragment counts per kilobase million transcripts (FPKM) are shown for steady-state cultures. O2-replete, O2-limiting, nitrogen-replete (NH4+), N2-fixing and the supplementation of H2 into the feed gas during chemostat operation is indicated.
Expression of the complete complement of genes necessary for the oxidation of CH4 to CO2, carbon assimilation via the Calvin-Benson-Bassham cycle, and ATP production via aerobic respiration were detected in all conditions tested (Figure 2C and Supplementary Table S1). However, with exception of a 1.78-fold (p-value: < 0.0001) downregulation of a moxY-like gene (Minf_1448) that encodes a methanol utilization control sensor protein, no significant differences in expression level in these pathways were observed across conditions. With respect to glycogen metabolism, all genes necessary for glycogen synthesis, storage and degradation were expressed, but did not display evidence of transcriptional regulation (Supplementary Table S1). Consistent with the onset of H2 oxidation in chemostat cultures, genes encoding for the large and small subunits of the Group 1d respiratory [NiFe] hydrogenase, and its associated cytochrome b subunit, were upregulated by 3.5-, 3.6-, and 2.9-fold respectively (to a maximum of 3105 FPKM) in response to O2 limitation (Figures 2A,C). Likewise, genes associated with hydrogenase maturation, nickel incorporation and nickel transport were also significantly upregulated (Supplementary Table S1). In contrast, the cytosolic Group 3b [NiFe] hydrogenase was constitutively transcribed at low levels (FPKM < 125).
We further analyzed RNA-seq data to investigate the influence of nitrogen limitation on transcriptional regulation within Methylacidiphilum sp. RTK17.1. During chemostat cultivation in the absence of a supplied nitrogen source (NH4+), 66 genes were upregulated (p-value: < 0.001; FDR: < 0.02) and 13 genes were downregulated (p-value: < 0.001; FDR: < 0.05) when compared to nitrogen excess growth conditions. Consistent with the onset of N2 fixation, a seven-gene operon encoding nitrogenase structural subunits and cofactor biosynthesis proteins (nifHDKENX, Minf_1870–1876) was upregulated (average 6.4 Log2FC; Figures 2B,C). In addition, numerous other genes involved in nitrogenase transcriptional regulation (nifA, Minf_0464), cofactor biosynthesis (nifB, Minf_0453), stabilization (nifW, Minf_0472), and nitrate (Minf_1096) and NH4+ transport (Minf_1075) were also significantly upregulated (Supplementary Table S1). Hydrogenase expression was not significantly different between the conditions, being high for the Group 1d [NiFe]-hydrogenase and low for the Group 3b [NiFe]-hydrogenase.
Discussion
The accumulation and storage of carbon and energy as polymeric reserves is a common strategy employed by microorganisms during unbalanced growth to fortify them against periods of environmental starvation (Wilson et al., 2010). Nitrogen limitation is often cited as triggering the accumulation of carbon-rich reserve polymers (Wanner and Egli, 1990), but there is a lack of detailed understanding of the underlying mechanisms responsible for their production. As with many heterotrophic species, methanotrophs often produce carbon-rich polymers; however despite the prevalence of glycogen production within the Gammaproteobacteria methanotrophs (Pieja et al., 2011a), research has primarily focused on the physiology of polyhydroxybutyrate storage in the Alphaproteobacteria methanotrophs (Pieja et al., 2011a, b; Sundstrom and Criddle, 2015). The requirement for organic carbon compounds to provide both the respiratory energy and carbon necessary for anabolic processes for methanotrophs makes it difficult to untangle the roles of nitrogen, carbon, and energy availability (e.g., ATP) in the production of intracellular glycogen. However, as verrucomicrobial methanotrophs fix CO2 for carbon and supplement their energy requirements via the oxidation of H2 (Mohammadi et al., 2016; Carere et al., 2017), this affords an opportunity (obfuscated by the Type I and II methanotrophs) to investigate the influence of nitrogen, carbon, and energy availability independently.
Our findings show that H2 oxidation influences the production of intracellular glycogen reservoirs in the thermoacidophilic methanotroph, Methylacidiphilum sp. RTK17.1. During chemostat experiments, the maximum glycogen content of Methylacidiphilum sp. RTK17.1 occurred within cells grown in the absence of H2, under nitrogen- and O2- limiting growth conditions [48.86 (± 4.32)%; Table 1 and Figure 1A]. With respect to other studies reporting on the production of carbon storage polymers in methanotroph species, the glycogen content values observed for Methylacidiphilum sp. RTK17.1 are generally congruent. In the closely related thermoacidophile, Methylacidiphilum fumarolicum SolV, a maximum glycogen content of 36% (w/w) was observed (Khadem et al., 2012a) in nitrogen-limited, batch grown cells and a similar value (33% w/w) has been reported in the halotolerant methanotroph Methylotuvimicrobium alkaliphilum 20Z [formerly Methylomicrobium alkaliphilum 20Z (Khmelenina et al., 1999; Orata et al., 2018)]. A maximum of 42.8 (± 17.5)% (w/w) glycogen has been reported in the industrially promising methanotroph, Methylotuvimicrobium buryatense 5GB1 [formerly Methylomicrobium buryatense 5GB1 (Orata et al., 2018)], during batch-growth on methanol, with up to 13.1 (± 4.0)% (w/w) glycogen reported during O2-limited chemostat growth on methane (Gilman et al., 2015).
The variability in reported glycogen content within methanotrophs (intraspecies) and between experimental trials (interspecies) is almost certainly a consequence of both underlying physiological characteristics and the inherent challenges associated with characterizing dynamic batch growth environments. We therefore sought to perform a series of carbon-excess (CO2 and CH4) steady-state experiments to gain insight into the mechanisms governing glycogen production in Methylacidiphilum sp. RTK17.1. With respect to O2 and nitrogen limitation, rates of growth (inferred from observed biomass productivity rates), and changes to glycogen, and to a lesser extent total protein and amino acid contents, were consistent with a cell’s expected response to unbalanced growth conditions. While previous studies have reported significant changes to the amino acid composition of Staphylococcus aureus cultures in response to variable environmental conditions (Alreshidi et al., 2015, 2016), we observed no change to the relative abundance of specific amino acid residues in Methylacidiphilum sp. RTK17.1 cultures under the conditions tested. Nevertheless, a 144% increase in glycogen content was observed during unbalanced growth following the removal of H2 gas supply. Depriving Methylacidiphilum sp. RTK17.1 cultures of the respiratory energy gains afforded from H2 gas oxidation is demonstrative of how this strain dynamically allocates carbon, nitrogen and energy resources.
The observation that Methylacidiphilum sp. RTK17.1 cells produce glycogen and grow more slowly in response to oxygen limitation is consistent with the occurrence of glycogen within the obligate chemolithoautotroph Hydrogenovibrio marinus when grown on H2 and CO2 under O2-limiting conditions (Nishihara et al., 1993). Similarly, production of polyhydroxybutyrate (PHB) has been reported within heterotrophically grown cultures of Azotobacter beijerinckii in response to oxygen limitation (Senior et al., 1972). We speculate that in the absence of sufficient oxygen, both glycogen and PHB reserves likely serve to not only store carbon and energy, but to maintain intracellular redox state. It is also plausible that the anabolic activities required for cell division (i.e., protein, DNA and RNA synthesis) were constrained by ATP availability under O2-limiting conditions. Given protein synthesis requires approximately 19 times more ATP (mmol ATP (g macromolecule)–1) than for saccharide polymerization (Stouthamer, 1979; Russell and Cook, 1995; Russell, 2007), even considering the ATP requirements of CO2 fixation, glycogen biosynthesis likely represents an energetic ‘cost’ savings for Methylacidiphilum sp. RTK171 compared to the ATP-demands of cell growth. These modest increases to intracellular glycogen content in response to O2 limitation are unlikely to negatively impact biomass yields (YATP; Russell and Cook, 1995) while also benefiting cell survivability during periods of starvation. The additional burden imposed by nitrogen limitation not only created unbalanced growth conditions with respect to carbon and nitrogen, but also increased the cell’s ATP requirement via the nitrogenase reaction (N2 + 8H+ + 16ATP → 2NH3 + H2 + 16ADP). Under these growth conditions, glycogen accounted for nearly half of Methylacidiphilum sp. RTK17.1 cell mass. Supplementing CH4 oxidation with an alternative source of respiratory energy (H2), however, was sufficient to offset the ATP burden imposed by N2 fixation and consequently the production of intracellular glycogen was reduced and growth rates increased.
An alternative explanation for our chemostat observations is that synthesis of glycogen during energy-limiting conditions serves as a strategy for ‘metabolic anticipation’. The combined conditions of low O2, nitrogen, and H2 availability are highly limiting for a cell and further resource deprivation is likely to trigger a transition from growth to persistence. Thus, disproportionately allocating biomass into storage compounds under this condition may serve as a ‘bet-hedging’ strategy to enable longer-term survival when conditions worsen. Indeed, the synthesis and storage of intracellular carbon polymers is commonly associated with an increase in viability during periods of environmental starvation. As with PHB, glycogen catabolism supplies reduced electron carriers (e.g., NADH) into the respiratory chain, thereby enabling the continuation of metabolic processes in the absence of an exogenous energy supply (e.g., CH4 or H2). A reduced lag phase following CH4 starvation has previously been linked to the catabolism of glycogen reservoirs within the methanotroph M. fumarolicum SolV (Khadem et al., 2012a). Likewise, in Methylacidiphilum sp. RTK17.1 cultures, we interpret depletions in cellular glycogen content throughout prolonged incubations at 4°C (in the absence of CH4) as evidence it was being consumed to promote survival (Supplementary Figure S1). Within oxic/anoxic habitats, it seems evident that Methylacidiphilum sp. RTK17.1 distributes carbon, energy and nitrogen resources during methanotrophic or mixotrophic growth to fulfill the metabolic demands imposed for cell persistence and/or proliferation (Hanson and Hanson, 1996). Similar phenomena of metabolic anticipation have been observed in other species, for example mycobacteria, which accumulate storage compounds such as triacylglycerols during the early hypoxic response (Daniel et al., 2004; Eoh et al., 2017).
While hydrogenase, methane monooxygenase and nitrogenase all displayed evidence of significant transcriptional regulation in response to O2 and nitrogen limitation, the genes associated with glycogen metabolism were constitutively expressed. These results are consistent with previous findings within the verrucomicrobial methanotrophs (Khadem et al., 2010; Khadem et al., 2012a; Mohammadi et al., 2016; Carere et al., 2017) and suggests the enzymes associated with glycogen metabolism may be allosterically regulated in response to high carbon (i.e., fructose 1,6-bisphosphate) and/or energy contents (i.e., ATP/AMP), as described in other bacterial species (Wilson et al., 2010). Consistent with other verrucomicrobial methanotrophs (Dunfield et al., 2007; Pol et al., 2007; Op den Camp et al., 2009), Methylacidiphilum sp. RTK17.1 also possesses three phylogenetically distinct pmoCAB operons. Based on observed ratios of non-synonymous versus synonymous substitution rates in pmoA orthologs, it has been proposed that the pMMOs encoded in Methylacidiphilum spp. serve functionally distinct roles (Op den Camp et al., 2009). The observation that Methylacidiphilum sp. RTK17.1 transcriptionally regulates pMMO expression in response to oxygen availability therefore supports this hypothesis and is congruent with reports of differential expression in response to oxygen limitation (Khadem et al., 2012a) and during growth on methanol (Erikstad et al., 2012). Finally, it is noteworthy to include that the transcriptional upregulation of the Group 1d [NiFe] hydrogenase occurred in response to O2 limitation; whereas nitrogenase upregulation was induced by nitrogen availability. The transcriptional decoupling of these two enzymes is further evidence that the physiological role of H2 oxidation in methanotrophs (Mohammadi et al., 2016; Carere et al., 2017) is distinct from recycling H2 produced during the nitrogen fixation reaction (Bont, 1976; Dixon, 1976; Chen and Yoch, 1987). Collectively, these findings indicate while H2 oxidation is sufficient to partially offset the energetic costs associated with N2 fixation, the regulation of this enzyme is transcriptionally uncoupled from nitrogen availability.
Data Availability
The raw and processed transcriptome sequence files (accession numbers GSM3872525–GSM3872529) were deposited into the Gene Expression Omnibus (GEO; https://www.ncbi.nlm.nih.gov/geo/) for archival storage.
Author Contributions
CRC, CG, and MS conceived the study. CRC, MS, CG, CC, DG, and BM contributed to the experimental design. CRC, BM, CC, and HP conducted the bioreactor and wet lab experiments. CRC and HP performed the glycogen analysis. CRC, MS, and CG performed the transcriptome experiments and analysis. CRC, CG, MS, and DG wrote the manuscript with input from BM, HP, and CC.
Funding
This work was supported by separate MBIE Strategic Science Investment Funds granted to GNS Science (CRC, MS, and HP) and Scion (DG, BM, and CC). CRC and CG were further supported by The Royal Society of New Zealand (Marsden Grant GNS1601). CC, DG, BM, and MS were also supported by an MBIE Smart Ideas Grant (C05X1710). CG was further supported by an ARC DECRA Fellowship (DE170100310).
Conflict of Interest Statement
The authors declare that the research was conducted in the absence of any commercial or financial relationships that could be construed as a potential conflict of interest.
Acknowledgments
Ngāti Tahu–Ngāti Whaoa are acknowledged as the iwi having mana whenua (customary rights) over the Rotokawa geothermal field, Methylacidiphilum sp. RTK17.1, and associated microorganisms. We thank Ngāti Tahu–Ngāti Whaoa Runanga Trust and Tauhara North 2 Trust for their support of our research. We also thank Prof. Peter Dunfield for valuable comments during the preparation of this manuscript.
Supplementary Material
The Supplementary Material for this article can be found online at: https://www.frontiersin.org/articles/10.3389/fmicb.2019.01873/full#supplementary-material
Footnotes
References
Alreshidi, M. M., Dunstan, R. H., Gottfries, J., Macdonald, M. M., Crompton, M. J., Ang, C.-S., et al. (2016). Changes in the cytoplasmic composition of amino acids and proteins observed in staphylococcus aureus during growth under variable growth conditions representative of the human wound site. PLoS One 11:e0159662. doi: 10.1371/journal.pone.0159662
Alreshidi, M. M., Dunstan, R. H., Macdonald, M. M., Smith, N. D., Gottfries, J., and Roberts, T. K. (2015). Metabolomic and proteomic responses of Staphylococcus aureus to prolonged cold stress. J. Proteom. 121, 44–55. doi: 10.1016/j.jprot.2015.03.010
AOAC (2005). Official Methods of Analysis of AOAC Int. 18th Ed., Method 994.12. Arlington, VA: AOAC international.
Bonafonte, M. A., Solano, C., Sesma, B., Alvarez, M., Montuenga, L., Garcia-Ros, D., et al. (2000). The relationship between glycogen synthesis, biofilm formation and virulence in Salmonella enteritidis. Fems Microbiol. Lett. 191, 31–36. doi: 10.1016/s0378-1097(00)00366-9
Bont, J. A. (1976). Hydrogenase activity in nitrogen-fixing methane-oxidizing bacteria. Antonie Van Leeuwenhoek 42, 255–259. doi: 10.1007/bf00394122
Bourassa, L., and Camilli, A. (2009). Glycogen contributes to the environmental persistence and transmission of Vibrio cholerae. Mol. Microbiol. 72, 124–138. doi: 10.1111/j.1365-2958.2009.06629.x
Carere, C. R., Hards, K., Houghton, K. M., Power, J. F., Mcdonald, B., Collet, C., et al. (2017). Mixotrophy drives niche expansion of Verrucomicrobial methanotrophs. ISME J. 11, 2599–2610. doi: 10.1038/ismej.2017.112
Chen, Y. P., and Yoch, D. C. (1987). Regulation of two nickel-requiring (inducible and constitutive) hydrogenases and their coupling to nitrogenase in Methylosinus trichosporium OB3b. J. Bacteriol. 169, 4778–4783. doi: 10.1128/jb.169.10.4778-4783.1987
Crombie, A. T., and Murrell, J. C. (2014). Trace-gas metabolic versatility of the facultative methanotroph Methylocella silvestris. Nature 510, 148–151. doi: 10.1038/nature13192
Daniel, J., Deb, C., Dubey, V. S., Sirakova, T. D., Abomoelak, B., Morbidoni, H. R., et al. (2004). Induction of a novel class of diacylglycerol acyltransferases and triacylglycerol accumulation in Mycobacterium tuberculosis as it Goes into a dormancy-like state in culture. J. Bacteriol. 186, 5017–5030. doi: 10.1128/jb.186.15.5017-5030.2004
Dedysh, S. N., and Dunfield, P. F. (2010). “Facultative methane oxidizers,” in Handbook of Hydrocarbon and Lipid Microbiology, ed. K. N. Timmis, (Berlin: Springer-Verlag), 1967–1976. doi: 10.1007/978-3-540-77587-4_144
Dedysh, S. N., Knief, C., and Dunfield, P. F. (2005). Methylocella species are facultatively methanotrophic. J. Bacteriol. 187, 4665–4670. doi: 10.1128/jb.187.13.4665-4670.2005
Dixon, R. O. D. (1976). Hydrogenases and efficiency of nitrogen-fixation in aerobes. Nature 262, 173–173. doi: 10.1038/262173a0
Dunfield, P. F., Yuryev, A., Senin, P., Smirnova, A. V., Stott, M. B., and Hou, S. (2007). Methane oxidation by an extremely acidophilic bacterium of the phylum Verrucomicrobia. Nature 450, 879–882. doi: 10.1038/nature06411
Ebeling, M. E. (1968). The dumas method for nitrogen in feeds. J. Assoc. Off. Anal. Chem. 51, 766–770.
Eidels, L., and Preiss, J. (1970). Carbohydrate metabolism in Rhodopseudomonas capsulata enzyme titers, glucose metabolism, and polyglucose polymer synthesis. Arch. Biochem. Biophys. 140, 75–89. doi: 10.1016/0003-9861(70)90011-1
Eoh, H., Wang, Z., Layre, E., Rath, P., Morris, R., Branch Moody, D., et al. (2017). Metabolic anticipation in Mycobacterium tuberculosis. Nat. Microbiol. 2, 17084–17084. doi: 10.1038/nmicrobiol.2017.84
Erikstad, H.-A., Jensen, S., Keen, T. J., and Birkeland, N.-K. (2012). Differential expression of particulate methane monooxygenase genes in the Verrucomicrobial methanotroph ‘Methylacidiphilum kamchatkense’ Kam1. Extremophiles 16, 405–409. doi: 10.1007/s00792-012-0439-y
Eshinimaev, B. T., Khmelenina, V. N., Sakharovskii, V. G., Suzina, N. E., and Trotsenko, Y. A. (2002). Physiological, biochemical, and cytological characteristics of a haloalkalitolerant methanotroph grown on methanol. Microbiology 71, 512–518.
Gibbons, R. J., and Kapsimalis, B. (1963). Synthesis of intracellular iodophilic polysaccharide by streptococcus-mitis. Arch. Oral Biol. 8, 319–329. doi: 10.1016/0003-9969(63)90024-4
Gilman, A., Laurens, L. M., Puri, A. W., Chu, F., Pienkos, P. T., and Lidstrom, M. E. (2015). Bioreactor performance parameters for an industrially-promising methanotroph Methylomicrobium buryatense 5GB1. Microb. Cell Fact. 14:182. doi: 10.1186/s12934-015-0372-8
Greening, C., Biswas, A., Carere, C. R., Jackson, C. J., Taylor, M. C., and Stott, M. B. (2016). Genome and metagenome surveys of hydrogenase diversity indicate H2 is a widely-utilised energy source for microbial growth and survival. ISME J. 10, 761–777. doi: 10.1038/ismej.2015.153
Hanczar, T., Csaki, R., Bodrossy, L., Murrell, C. J., and Kovacs, K. L. (2002). Detection and localization of two hydrogenases in Methylococcus capsulatus (Bath) and their potential role in methane metabolism. Arch. Microbiol. 177, 167–172. doi: 10.1007/s00203-001-0372-4
Hou, S., Makarova, K. S., Saw, J. H. W., Senin, P., Ly, B. V., Zhou, Z., et al. (2008). Complete genome sequence of the extremely acidophilic methanotroph isolate V4, Methylacidiphilum infernorum, a representative of the bacterial phylum Verrucomicrobia. Biol. Direct 3:26. doi: 10.1186/1745-6150-3-26
Keltjens, J. T., Pol, A., Reimann, J., and Den Camp, H. (2014). PQQ-dependent methanol dehydrogenases: rare-earth elements make a difference. Appl. Microbiol. Biotechnol. 98, 6163–6183. doi: 10.1007/s00253-014-5766-8
Khadem, A. F., Pol, A., Jetten, M. S. M., and Op Den Camp, H. J. M. (2010). Nitrogen fixation by the Verrucomicrobial methanotroph ‘Methylacidiphilum fumariolicum’ SolV. Microbiology 156, 1052–1059. doi: 10.1099/mic.0.036061-0
Khadem, A. F., Van Teeseling, M. C. F., Van Niftrik, L., Jetten, M. S. M., Op Den Camp, H. J. M., and Pol, A. (2012a). Genomic and physiological analysis of carbon storage in the Verrucomicrobial methanotroph “Ca. Methylacidiphilum Fumariolicum” SolV. Front. Microbiol. 3:345. doi: 10.3389/fmicb.2012.00345
Khadem, A. F., Wieczorek, A. S., Pol, A., Vuilleumier, S., Harhangi, H. R., and Dunfield, P. F. (2012b). Draft genome sequence of the volcano-inhabiting thermoacidophilic methanotroph Methylacidiphilum fumariolicum strain SolV. J. Bacteriol. 194, 3729–3730. doi: 10.1128/JB.00501-12
Khmelenina, V. N., Kalyuzhnaya, M. G., Sakharovsky, V. G., Suzina, N. E., Trotsenko, Y. A., and Gottschalk, G. (1999). Osmoadaptation in halophilic and alkaliphilic methanotrophs. Arch. Microbiol. 172, 321–329. doi: 10.1007/s002030050786
Kirschke, S., Bousquet, P., Ciais, P., Saunois, M., Canadell, J. G., and Dlugokencky, E. J. (2013). Three decades of global methane sources and sinks. Nat. Geosci. 6, 813–823. doi: 10.1073/pnas.1814297116
Knief, C. (2015). Diversity and habitat preferences of cultivated and uncultivated aerobic methanotrophic bacteria evaluated based on pmoA as molecular marker. Front. Microbiol. 6:1346. doi: 10.3389/fmicb.2015.01346
Knief, C., Lipski, A., and Dunfield, P. F. (2003). Diversity and activity of methanotrophic bacteria in different upland soils. Appl. Environ. Microbiol. 69, 6703–6714. doi: 10.1128/aem.69.11.6703-6714.2003
Linton, J. D., and Cripps, R. E. (1978). The occurrence and identification of intracellular polyglucose storage granules in Methylococcus NCIB 11083 grown in chemostat culture on methane. Arch. Microbiol. 117, 41–48. doi: 10.1007/bf00689349
McMeechan, A., Lovell, M. A., Cogan, T. A., Marston, K. L., Humphrey, T. J., and Barrow, P. A. (2005). Glycogen production by different Salmonella enterica serotypes: contribution of functional glgC to virulence, intestinal colonization and environmental survival. Microbiol. SGM 151, 3969–3977. doi: 10.1099/mic.0.28292-0
Mohammadi, S., Pol, A., Van Alen, T. A., Jetten, M. S. M., and Op Den Camp, H. J. M. (2016). Methylacidiphilum fumariolicum SolV, a thermoacidophilic ‘Knallgas’ methanotroph with both an oxygen-sensitive and -insensitive hydrogenase. ISME J. 11, 945–958. doi: 10.1038/ismej.2016.171
Mortazavi, A., Williams, B. A., Mccue, K., Schaeffer, L., and Wold, B. (2008). Mapping and quantifying mammalian transcriptomes by RNA-Seq. Nat. Meth. 5, 621–628. doi: 10.1038/nmeth.1226
Nishihara, H., Igarashi, Y., Kodama, T., and Nakajima, T. (1993). Production and properties of glycogen in the marine obligate chemolithoautotroph, Hydrogenovibrio marinus. J. Ferment. Bioeng. 75, 414–416. doi: 10.1016/0922-338x(93)90087-o
Op den Camp, H. J., Islam, T., Stott, M. B., Harhangi, H. R., Hynes, A., and Schouten, S. (2009). Environmental, genomic and taxonomic perspectives on methanotrophic Verrucomicrobia. Environ. Microbiol. Rep. 1, 293–306. doi: 10.1111/j.1758-2229.2009.00022.x
Orata, F. D., Meier-Kolthoff, J. P., Sauvageau, D., and Stein, L. Y. (2018). Phylogenomic Analysis of the Gammaproteobacterial methanotrophs (Order Methylococcales) calls for the reclassification of members at the genus and species levels. Front. Microbiol. 9:3162. doi: 10.3389/fmicb.2018.03162
Pieja, A. J., Rostkowski, K. H., and Criddle, C. S. (2011a). Distribution and Selection of Poly-3-hydroxybutyrate production capacity in methanotrophic Proteobacteria. Microb. Ecol. 62, 564–573. doi: 10.1007/s00248-011-9873-0
Pieja, A. J., Sundstrom, E. R., and Criddle, C. S. (2011b). Poly-3-hydroxybutyrate metabolism in the type II methanotroph Methylocystis parvus OBBP. Appl. Environ. Microb. 77, 6012–6019. doi: 10.1128/aem.00509-11
Pol, A., Heijmans, K., Harhangi, H. R., Tedesco, D., Jetten, M. S. M., and Den Camp, H. (2007). Methanotrophy below pH 1 by a new Verrucomicrobia species. Nature 450, 874–878. doi: 10.1038/nature06222
Preiss, J. (1984). Bacterial glycogen synthesis and its regulation. Annu. Rev. Microbiol. 38, 419–458. doi: 10.1146/annurev.micro.38.1.419
Robinson, M. D., Mccarthy, D. J., and Smyth, G. K. (2010). edgeR: a bioconductor package for differential expression analysis of digital gene expression data. Bioinformatics 26, 139–140. doi: 10.1093/bioinformatics/btp616
Russell, J. B. (2007). The energy spilling reactions of bacteria and other organisms. J. Mol. Microb. Biotech. 13, 1–11. doi: 10.1159/000103591
Russell, J. B., and Cook, G. M. (1995). Energetics of bacterial growth: balance of anabolic and catabolic reactions. Microbiol. Rev. 59, 48–62.
Senior, P. J., Beech, G. A., Ritchie, G. A., and Dawes, E. A. (1972). The role of oxygen limitation in the formation of poly-B-hydroxybutyrate during batch and continuous culture of Azotobacter beijerinckii. Biochem. J. 128, 1193–1201. doi: 10.1042/bj1281193
Shah, N. N., Hanna, M. L., Jackson, K. J., and Taylor, R. T. (1995). Batch cultivation of Methylosinus trichosporium OB3B: IV. Production of hydrogen-driven soluble or particulate methane monooxygenase activity. Biotechnol. Bioeng. 45, 229–238. doi: 10.1002/bit.260450307
Singh, B. K., Bardgett, R. D., Smith, P., and Reay, D. S. (2010). Microorganisms and climate change: terrestrial feedbacks and mitigation options. Nat. Rev. Microbiol. 8, 779–790. doi: 10.1038/nrmicro2439
Stouthamer, A. H. (1979). “The search for correlation between theoretical and experimental growth yields,” in International Review Of Biochemistry, Vol. 21, ed. J. R. Quayle, (Baltimore, MD: University Park Press), 1–48.
Strong, P. J., Kalyuzhnaya, M., Silverman, J., and Clarke, W. P. (2016). A methanotroph-based biorefinery: potential scenarios for generating multiple products from a single fermentation. Bioresour. Technol. 215, 314–323. doi: 10.1016/j.biortech.2016.04.099
Sundstrom, E. R., and Criddle, C. S. (2015). Optimization of methanotrophic growth and production of poly(3-Hydroxybutyrate) in a high-throughput microbioreactor system. Appl. Environ. Microbiol. 81, 4767–4773. doi: 10.1128/AEM.00025-15
Takeda, K. (1988). Characteristics of a nitrogen-fixing methanotroph, Methylocystis T-1. Antonie Van Leeuwenhoek 54, 521–534. doi: 10.1007/bf00588388
Tavormina, P. L., Ussler, W., Joye, S. B., Harrison, B. K., and Orphan, V. J. (2010). Distributions of putative aerobic methanotrophs in diverse pelagic marine environments. ISME J. 4, 700–710. doi: 10.1038/ismej.2009.155
Thiex, N., Novotny, L., and Crawford, A. (2012). Determination of ash in animal feed: AOAC official method 942.05 revisited. J. AOAC Int. 95, 1392–1397. doi: 10.5740/jaoacint.12-129
Vara, C., Paytuví-Gallart, A., Cuartero, Y., Le Dily, F., Garcia, F., Salvà-Castro, J., et al. (2019). Three-dimensional genomic structure and cohesin occupancy correlate with transcriptional activity during spermatogenesis. Cell Rep. 28, 352–367.e9. doi: 10.1016/j.celrep.2019.06.037
Wanner, U., and Egli, T. (1990). Dynamics of microbial growth and cell composition in batch culture. FEMS Microbiol. Lett. 75, 19–43. doi: 10.1016/0378-1097(90)90521-q
Keywords: methanotroph, hydrogenase, glycogen, extremophile, methylacidiphilum
Citation: Carere CR, McDonald B, Peach HA, Greening C, Gapes DJ, Collet C and Stott MB (2019) Hydrogen Oxidation Influences Glycogen Accumulation in a Verrucomicrobial Methanotroph. Front. Microbiol. 10:1873. doi: 10.3389/fmicb.2019.01873
Received: 06 June 2019; Accepted: 29 July 2019;
Published: 16 August 2019.
Edited by:
Roland Hatzenpichler, Montana State University, United StatesReviewed by:
Ludmila Chistoserdova, University of Washington, United StatesGrayson L. Chadwick, California Institute of Technology, United States
Lisa Y. Stein, University of Alberta, Canada
Copyright © 2019 Carere, McDonald, Peach, Greening, Gapes, Collet and Stott. This is an open-access article distributed under the terms of the Creative Commons Attribution License (CC BY). The use, distribution or reproduction in other forums is permitted, provided the original author(s) and the copyright owner(s) are credited and that the original publication in this journal is cited, in accordance with accepted academic practice. No use, distribution or reproduction is permitted which does not comply with these terms.
*Correspondence: Carlo R. Carere, Y2FybG8uY2FyZXJlQGNhbnRlcmJ1cnkuYWMubno=