- 1School of Microbiology, University College Cork, Cork, Ireland
- 2Environmental Research Institute, University College Cork, Cork, Ireland
The emergence of antibiotic resistant microorganisms has led to an increased need for the discovery and development of novel antimicrobial compounds. Frequent rediscovery of the same natural products (NPs) continues to decrease the likelihood of the discovery of new compounds from soil bacteria. Thus, efforts have shifted toward investigating microorganisms and their secondary metabolite biosynthesis potential, from diverse niche environments, such as those isolated from marine sponges. Here we investigated at the genomic level two Streptomyces spp. strains, namely SM17 and SM18, isolated from the marine sponge Haliclona simulans, with previously reported antimicrobial activity against clinically relevant pathogens; using single molecule real-time (SMRT) sequencing. We performed a series of comparative genomic analyses on SM17 and SM18 with their closest terrestrial relatives, namely S. albus J1074 and S. pratensis ATCC 33331 respectively; in an effort to provide further insights into potential environmental niche adaptations (ENAs) of marine sponge-associated Streptomyces, and on how these adaptations might be linked to their secondary metabolite biosynthesis potential. Prediction of secondary metabolite biosynthetic gene clusters (smBGCs) indicated that, even though the marine isolates are closely related to their terrestrial counterparts at a genomic level; they potentially produce different compounds. SM17 and SM18 displayed a better ability to grow in high salinity medium when compared to their terrestrial counterparts, and further analysis of their genomes indicated that they possess a pool of 29 potential ENA genes that are absent in S. albus J1074 and S. pratensis ATCC 33331. This ENA gene pool included functional categories of genes that are likely to be related to niche adaptations and which could be grouped based on potential biological functions such as osmotic stress, defense; transcriptional regulation; symbiotic interactions; antimicrobial compound production and resistance; ABC transporters; together with horizontal gene transfer and defense-related features.
Introduction
With the emergence and rapid spread of antibiotic resistant microorganisms, displaying resistance to many currently available antibiotics, a concerted effort continues to be needed to discover novel antimicrobial agents (Thabit et al., 2015; Rolain et al., 2016). Members of the Streptomyces genus are also known to produce a broad range of other natural products (NPs) which possess immunosuppressant, anti-fungal, anti-cancer, anti-parasitic and anti-thrombotic activities (Hwang et al., 2014; Ser et al., 2017). However, the frequent re-discovery of previously characterized bioactive compounds from terrestrial Streptomyces, has somewhat limited the interest of researchers in terrestrial ecosystems as potential reservoirs for novel biomolecules (Yagüe et al., 2012; Dalisay et al., 2013; Paulus et al., 2017). Instead, interest has begun to focus on the isolation of Streptomyces from other environmental niches; with Streptomyces involved in symbiotic relationships or associated with plants, insects, fungi, lichens, sea-cucumbers, seaweeds and marine sponges also attracting increased attention as potential reservoirs for these types of bioactive molecules (Motohashi et al., 2010; Seipke et al., 2012; van der Meij et al., 2017). The ability of these Streptomyces to colonize such a wide variety of hosts is due in part to their ability to produce useful NPs, such as antimicrobials which help their hosts defend themselves against predators or pathogenic bacteria and fungi (Adnani et al., 2017; van der Meij et al., 2017).
Marine ecosystems are attracting particular attention, where extreme and rapidly changing environmental conditions such as differences in pressure, salinity, pH, light intensity, temperature and oligotrophic conditions are believed to be linked to secondary metabolites production (Abdelmohsen et al., 2014; van der Meij et al., 2017). In this respect, marine ecosystems have been a particularly fruitful source of Streptomyces strains which have the potential to produce new bioactive NPs (Hassan et al., 2017; Jin et al., 2018; Xu et al., 2018), with marine Streptomyces being isolated from seashores, coastal waters, bottom sediments, fishes, molluscs, sponges, seaweeds and mangroves (Manivasagan et al., 2014; Ser et al., 2017).
Marine sponges (phylum Porifera) in particular are known to be a rich source of bioactive compounds, many of which are produced by the bacteria which reside within the sponge host (Abdelmohsen et al., 2014; Fuerst, 2014). Many of these bioactives have antimicrobial activities, making these sponge-associated microbial and fungal communities a potentially valuable source of novel antimicrobials (Baker et al., 2009; Flemer et al., 2012; Hoppers et al., 2015; Indraningrat et al., 2016; Jackson et al., 2018). While sponge bacteria-derived antimicrobial compounds have to date been identified from 35 different genera, the most predominant producing genera include Streptomyces, Pseudovibrio and Bacillus strains (Indraningrat et al., 2016). Of these, Streptomyces are the predominant genus, producing around 30% of the compounds identified to date (Indraningrat et al., 2016). Good examples of bioactive compounds produced from Streptomyces associated with marine sponges include: mayamycin, produced by Streptomyces sp. HB202 isolated from Halichondria panicea (Schneemann et al., 2010); the naphthacene glycoside SF2446A2, produced by Streptomyces sp. RV15 isolated from Dysidea tupha (Reimer et al., 2015); and Petrocidin A, produced by Streptomyces sp. SBT348 isolated from Petrosia ficiformis (Cheng et al., 2017).
As previously mentioned, in addition to marine sponges, many Streptomyces strains have also evolved symbiotic relationships with plants, fungi, and insects, amongst others; and there is increasing evidence that the host may control which metabolic pathways are activated within their symbionts, such as in the tunicate Lissoclinum patella and the squid Euprymna scolopes (Kwan et al., 2014; Gromek et al., 2016). In Streptomyces spp., it is clear that not only do they benefit from the resources of the hosts they interact with, but that these interactions control the expression of secondary metabolite biosynthetic gene clusters (smBGCs); thereby promoting the high degree of chemical diversity observed in the secondary metabolites being produced by these organisms (van der Meij et al., 2017). An example is the recent report that exposure of the endosymbiont Streptomyces ACT-52A to Aplysilla rosea promoted production of bioactive compounds with antibacterial activity (Mehbub et al., 2016). The factors involved in controlling the expression of these smBGCs are likely to be quite diverse, given the large degree of variability in the habitats and potential hosts, and how they are presumably influencing the secondary metabolite biosynthetic potential of Streptomyces symbionts (Adnani et al., 2017). Thus, it is clear that an increased knowledge of the genetics underpinning the interactions and signaling between the sponge host and the symbiont is required, through identification of smBGCs in the genomes of these sponge associated Streptomyces strains, coupled with identification of potential environmental “triggers” from the sponge, from other sponge endosymbionts, and/or from the surrounding marine environment that may regulate transcription of these smBGCs (Mehbub et al., 2016; Adnani et al., 2017; van der Meij et al., 2017).
To this end, we recently sequenced the genomes of 13 Streptomyces spp. isolated from both shallow water and deep-sea sponges, that displayed antimicrobial activities against a number of clinically relevant bacterial and yeast species (Kennedy et al., 2009; Jackson et al., 2018). Using the antiSMASH (antibiotics and Secondary Metabolite Analysis Shell) software (Blin et al., 2017), the strains were found to host abundant smBGCs which potentially encode polyketides, non-ribosomal peptide synthases (NRPS), siderophores, lantipeptides, and bacteriocins (Jackson et al., 2018). Thus, these strains appear to be a promising source of novel bioactive secondary metabolites, as the abundance and diversity of smBGCs displayed high degrees of novelty. In addition, the strains were enriched for genes potentially involved in the biosynthesis and transport of compatible solutes and for heat-shock proteins, genes which are typically associated with marine adaptations (Penn and Jensen, 2012; Tian et al., 2016).
Around sixty marine adaptation genes (MAGs) have previously been proposed for the obligate marine actinomycete genus Salinispora, with the function of these genes being associated with electron transport, sodium and ABC transporters, together with channels and pores (Penn and Jensen, 2012). Even though sponge-associated Streptomyces are marine bacteria, the environmental niche occupied by these organisms differs quite markedly from Salinispora, thus the genetic adaptions may not necessarily be similar. This was confirmed by the Zotchev group, when the draft genome of two sponge associated Streptomyces strains where analyzed for MAGs, revealing the presence of only seven of the Salinispora MAG gene pool (Ian et al., 2014). They suggested that specific marine sponge genetic adaptations may exist, given that different genes were identified in these sponge-associated Streptomyces which were absent in their soil counterparts (Ian et al., 2014). However, drawing conclusions for these genetic adaptations is quite difficult due to the limited number of sponge-associated Streptomyces genomes that are currently available. To this end, we sequenced the genomes of Streptomyces strains SM17 and SM18, two of the aforementioned 13 sponge-derived Streptomyces spp. that had displayed antimicrobial activity, using the PacBio RSII Single Molecule, Real-Time (SMRT) sequencing platform. This allowed us to study not only the smBGCs that these bacteria possess, but also other genetic characteristics that may be involved in their life cycle; such as for example adaptation to the marine environment and symbiosis. By employing comparative genomics, we compared the genomes of these strains with their most closely related terrestrial type-strain relatives, with complete genomes available in the GenBank database (namely S. albus J1074 for SM17 and S. pratensis ATCC 33331 for SM18), in an attempt to identify genes potentially associated with ENA, together with genes encoding potentially novel smBGCs.
Materials and Methods
Bacterial Strains, Maintenance and Differential Growth Assessment
The SM17 and SM18 strains were isolated from the marine sponge Haliclona simulans (Kilkieran Bay, Galway, Ireland), as previously described (Kennedy et al., 2009). The S. albus J1074 strain was provided by Dr. Andriy Luzhetskyy (Helmholtz Institute for Pharmaceutical Research Saarland, Germany), while S. flavogriseus/S. pratensis ATCC 33331 was obtained from the American Type Culture Collection (ATCC Inc., United States). SM17, SM18, S. albus J1074 and S. flavogriseus/S. pratensis ATCC 33331 spores were propagated on mannitol-soya (MS) agar medium at 28°C for 8–10 days and stored in 20% glycerol at −80°C. Strains were cultivated on ISP2 and ISP2 plus artificial sea water (ASW) medium when indicated, for differential growth analysis. The ASW was obtained by adding 3% Instant Ocean® Sea Salt (Instant Ocean Inc., United States) to the medium. It is important to note that the ATCC 33331 strain, due to a more recent taxonomy classification (Rong et al., 2013), is described with two different names: in GenBank as S. pratensis ATCC 33331 (new classification), and in the ATCC® culture collection as S. flavogriseus ATCC 33331 (old classification). From now on, the ATCC 33331 isolate will be referred to as S. pratensis ATCC 33331.
Genome Sequencing, Assembly and Annotation
Biomass from the SM17 and SM18 strains was obtained after cultivation on TSB medium for 3 days at 28°C and 220 rpm. Genomic DNA from SM17 was isolated using the DNeasy Blood & Cell Culture DNA Midi Kit (Qiagen Inc.); and by using the phenol-chloroform-isoamyl alcohol extraction method for SM18 (Wilson, 2001). Genome sequencing was performed by Macrogen (Seoul, South Korea), using the PacBio RSII sequencing platform.
The PacBio raw reads were processed and quality filtered using the BamTools toolkit v2.4.1 (subread length >1000, subread quality >0.75) (Barnett et al., 2011). The genome assemblies were performed using the Canu v1.7 software (Koren et al., 2017), followed by assembly polishing using Quiver v2.1.0 (Pacific Biosciences Inc). The assembly coverage check was performed using the BBMap program v37.901. Genome assembly statistics were calculated using the QUAST v4.6.3 program (Gurevich et al., 2013). Genome annotation was performed using the Prokka v1.12 program for this study’s analyses (Seemann, 2014), and with the NCBI Prokaryotic Genome Annotation Pipeline for data submission on the GenBank database (Tatusova et al., 2016; Benson et al., 2018). Prediction of smBGCs was performed using the antiSMASH 4 software (Blin et al., 2017). Similarity clustering of smBGCs families was performed using the Biosynthetic Genes Similarity Clustering and Prospecting Engine (BiG-SCAPE, version 2018100) (Navarro-Muñoz et al., 2018) and Cytoscape (v3.7.1) (Shannon et al., 2003), with annotations based on the Minimum Information about a Biosynthetic Gene cluster (MIBiG) repository (v1.4) (Medema et al., 2015). Genome maps were generated using the Artemis v17.0.1 and the DNAPlotter v17.0.1 programs (Rutherford et al., 2000; Carver et al., 2009). Proteins of interest were manually annotated using the NCBI BLAST tool; the GenBank database; and the Conserved Domain Database (CDD) (Johnson et al., 2008; Marchler-Bauer et al., 2015; Benson et al., 2018).
Comparative Genomics
The closest reference strains for the sponge-derived isolates SM17 and SM18 were determined by employing a phylogenetic analysis performed in two steps: (1) based on the 16S rRNA sequence of the SM17 and SM18 isolates, we picked the top 30 most similar Streptomyces species to each of the isolates (for a total of 60 genomes from the database), with complete genome available in GenBank (Benson et al., 2018), using the NCBI BLAST tool (Johnson et al., 2008) (2) we then performed a phylogenetic analysis employing concatenated sequences (Gadagkar et al., 2005) of the 16S rRNA and the housekeeping genes atpD (ATP synthase subunit beta), gyrB (DNA gyrase subunit B), recA (recombinase RecA), rpoB (DNA-directed RNA polymerase subunit beta), and trpB (tryptophan synthase beta chain), of the SM17 and SM18 strains, plus the previously determined top 60 most similar Streptomyces species. Alignment of the concatenated sequences was performed using the MAFFT program (Katoh and Standley, 2013), and phylogeny was determined using the MrBayes program (Ronquist et al., 2012), applying the General Time Reversible (GTR) model of nucleotide substitution with gamma-distributed rates across sites with a proportion of invariable sites (Waddell and Steel, 1997), and an average standard deviation of split frequencies cut off of 0.01. The final condensed tree, with a posterior probability cut off of 95%, was generated using MEGA X (Kumar et al., 2018) and Inkscape2. To further support genomic similarities between the SM17 and SM18 strain and their closest type-strain terrestrial relative determined with the phylogeny analysis, alignments of the individual housekeeping genes were performed and sequence similarity was determined, using the NCBI BLAST tool (Johnson et al., 2008); and whole genome nucleotide alignments were performed using the MUMmer 3.0 program (Kurtz et al., 2004). Plasmids sequences were determined by similarity searches in the GenBank database (Benson et al., 2018). Orthologous gene analysis was performed using the Roary v3.12.0 program, with an identity cut-off set to 50% (Page et al., 2015). The Roary outputs were processed using the R software environment in the RStudio IDE (Racine, 2012; RStudio Team, 2015; R Core Team, 2018), with data frame handling using the plyr package (Wickham, 2011); and Venn diagrams generated using the venn package (Dusa, 2018).
Accession Numbers
The complete genome sequences of SM17, SM18, and the SM17 plasmid sequences pSM17A, pSM17B, pSM17C, have been deposited in GenBank under the accession numbers CP029338, CP029342, CP029339, CP029340, and CP029341, respectively. The closest reference genomes used in this study for comparative purposes were S. albus J1074 (accession no. CP004370.1) and S. pratensis ATCC 33331 (accession no. CP002475.1).
Results and Discussion
Genome Sequencing and Assembly
The genomes of the marine sponge-derived Streptomyces spp. isolates SM17 and SM18 were sequenced using the PacBio RSII sequencing platform, which generated a total of 140,538 and 87,756 subreads respectively, after adapter removal and quality/length filtering (Table 1A). The PacBio sequencing provided long read lengths, averaging 9,702 and 8,923 bp for SM17 and SM18, respectively. Combining the large number of reads and their long length, an approximate sequencing coverage of 194× and 101× was obtained for SM17 and SM18, respectively.
The genome assemblies for both isolates were of a very high quality, resulting in single contig assemblies of the chromosomes, without gaps or ambiguous bases (Ns), with a total genome size comprising of 7,179,914 bp (including plasmids sequences, with 6,975,788 bp for the chromosome alone) for SM17; and 7,703,166 bp (without plasmids) for SM18 (Table 1A). High quality genome assemblies are highly advantageous for determining the core genome; identifying genome sequence and structure variants; analyzing gene acquisition and duplication; together with exploring the potential presence of smBGCs at a genetic level, which is particularly relevant for studies on the Streptomyces genus (Bentley et al., 2002; Schmid et al., 2018). Although a few marine Streptomyces spp. isolates have recently had their genomes sequenced, the majority of these consist of considerably fragmented sequences due to the complexity of the genome assemblies; which to a large extent hinders an in-depth analysis of these organisms at a genomic level, particularly with respect to analyzing the presence of smBGCs (Gomez-Escribano et al., 2015; Jackson et al., 2018). To our knowledge, this is one of the first studies to report the complete genome sequence of marine sponge-derived Streptomyces spp. isolates.
The sequencing approach employed allowed the identification of plasmids in the SM17 isolate – pSM17A, pSM17B, and pSM17C (Table 1B). A series of factors led to their classification as plasmids, instead of simply fragments of the chromosome. Firstly, the contigs were much smaller than the super contig determined to be the chromosome: 153,923 bp, 28,056 bp, and 22,147 bp, respectively, when compared to 6,975,788 bp for the chromosome. In addition, their GC content varied from that of the chromosome, which is characteristic of exogenous and plasmid DNA (Nishida, 2012). The approximate sequencing coverage of the sequences was also varied, which is an indicator of differences in the copy number of the plasmid molecules, with pSM17B having a considerably larger coverage of 548×, as opposed to 170× for pSM17A and 95× for pSM17C (Rasko et al., 2007). Finally, they were determined to share high sequence identity to other plasmids from Streptomyces spp. deposited in the GenBank database, as shown in Table 1B (Guo et al., 2011; Wang et al., 2012; Liu et al., 2016).
Potential Terminal Inverted Repeats (TIRs) with an estimated size of approximately 13.4 kb and 14.6 kb were identified in both the SM17 and SM18 chromosomes respectively, using a reciprocal BLASTN approach at the ends of the chromosome sequences (Gomez-Escribano et al., 2015). The Streptomyces genus is known to possess linear chromosomes with TIRs, with lengths varying among species; ranging from 14 bp in Streptomyces hygroscopicus 5008 to over 1 Mbp in S. coelicolor (Weaver et al., 2004; Wu et al., 2012). Although TIRs are commonly encountered in Streptomyces spp., their function has not yet been definitively proven, with suggested roles been proposed including chromosome stability, replication and recombination; and genome plasticity (Volff et al., 1997; Goshi et al., 2002; Choulet et al., 2006a,b; Lin et al., 2009). The main genomic features of SM17 and the three plasmids, and SM18 (number of base pairs, coding sequences (CDSs), GC% content, and the TIRs regions) are presented in the genome maps in Figure 1.
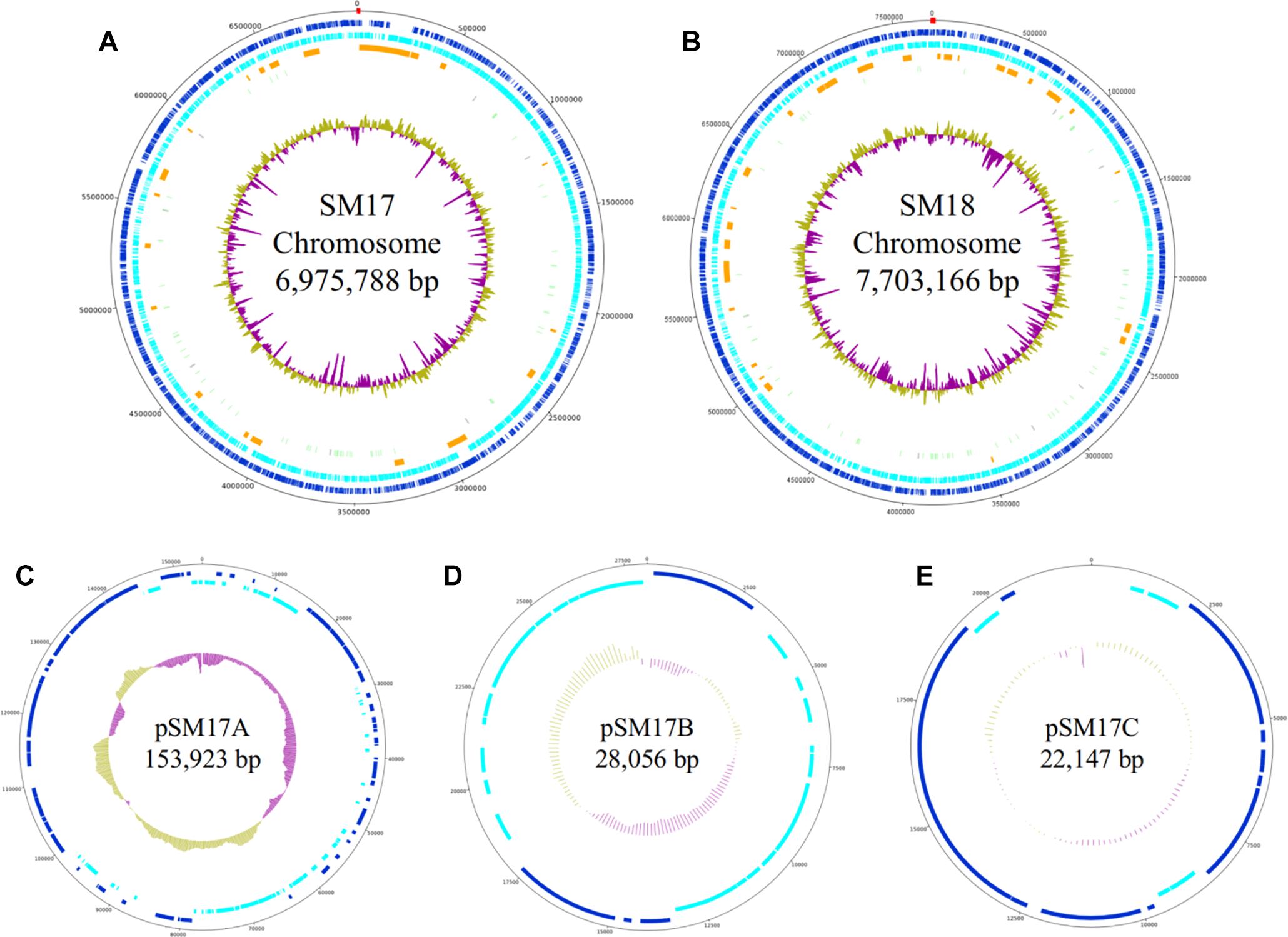
Figure 1. Genome maps of the SM17 and the SM18 chromosomes (A,B), and the SM17 plasmids pSM17A (C), pSM17B (D), pSM17C (E), generated using the Artemis and DNAplotter programs. All the molecules were in silico-determined to be linear, although they are represented in a circular fashion, and the sizes are not representative of the scale. The following are represented from the outer to the inner circles: the nucleotide position; coding sequences (CDSs) in the forward strand (in blue); CDSs in the reverse strand (in cyan); regions of putative secondary metabolite biosynthetic gene clusters (smBGCs, in orange); tRNA and rRNA genes (in gray and green, respectively); GC% plot on default settings (above average in olive and below average in purple). In (A,B), detailed in red are the regions determined to be the terminal inverted repeats (TIRs).
Determining the Closest Terrestrial Type-Strain Relative for the Marine Sponge-Derived Isolates
In order to analyze possible niche adaptations in the marine sponge-derived SM17 and SM18 isolates, phylogenetic and whole-genome alignment analyses were performed to identify the closest terrestrial type-strain relative, with the complete genome sequence available in GenBank, of each isolate; with a view to performing subsequent phenotypic, morphological and genomic comparisons once these relatives had been determined.
Phylogenetic analysis was performed using the 16S rRNA and other housekeeping aforementioned genes, which allowed us to determine that S. albus J1074 and S. pratensis ATCC 33331 were the closest type-strain relative to the SM17 and SM18 strains, respectively (Figure 2). Notably, SM17 and J1074 – a derivative of the soil isolate Streptomyces albus G (Chater and Wilde, 1976, 1980) – are included in the same sub-clade, while SM18 and ATCC 33331 are not, indicating that the latter pair are more distantly related than the former. Nevertheless, further analyses were performed with the ATCC 33331 strain, as it was the type-strain included in the SM18 clade that was readily available in culture collections. Also, it is important to note that the ATCC 33331 strain is the only soil-derived isolate present in the SM18 clade (NCBI BioSample: SAMN00191232), while SirexAA-E was isolated from an insect/microbe symbiotic community (Bianchetti et al., 2013); PAMC26508 was isolated in association with the Antarctic lichen Cladonia borealis (Shin et al., 2013); and S501 was isolated from the sediment from a seaside wetland (NCBI BioSample: SAMN10144670). Thus, for these aforementioned reasons (being a type-strain with its complete genome available on GenBank, isolated from soil, and available from culture collections), the ATCC 33331 strain was determined to be the most suitable isolate identified in the SM18 clade for the purposes of this study.
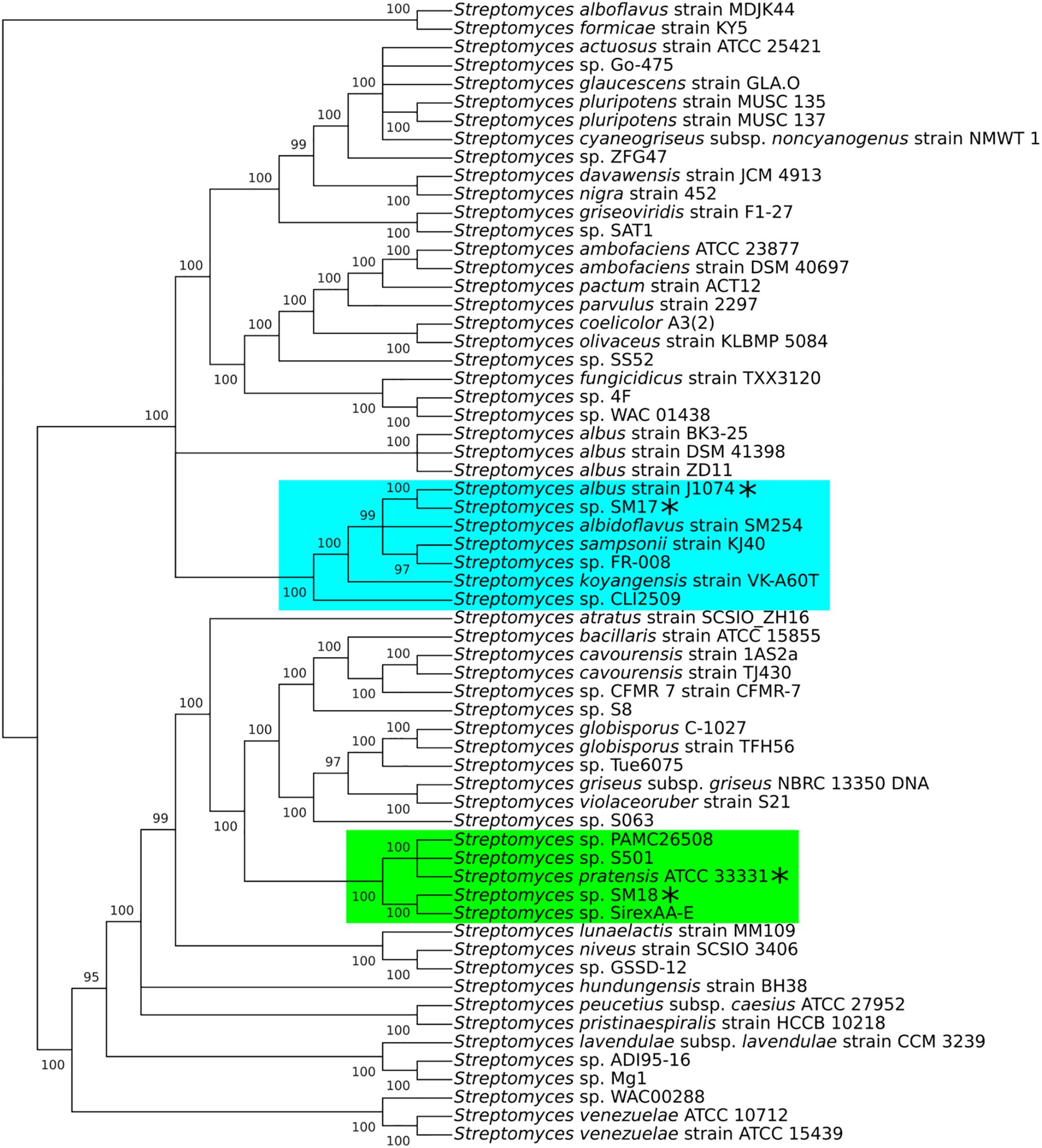
Figure 2. Phylogenetic tree of the concatenated nucleotide sequence of the 16S rRNA gene, plus the housekeeping genes atpD, gyrB, recA, rpoB, and tyrB. Including in this analysis are the SM17 and SM18 isolates, plus 60 Streptomyces isolates with complete genomes available in the GenBank database. Generated using MrBayes and MEGA X, with a posterior probability cut off of 95%.
To further support the similarities between our marine strains, SM17 and SM18, and their closest terrestrial counterparts, J1074 and ATCC 33331, alignments of the individual 16S rRNA and the other housekeeping genes were performed with NCBI BLASTN and BLASTX (Table 2). The high identity values determined by the analysis allowed further comparisons to be determined between the related pairs, and also between all four Streptomyces strains. Notably, the identities for the SM17- S. albus J1074 pair are higher (>99% for all the genes analyzed) than those for the SM18 – S. pratensis ATCC 33331 pair; (91% to 99% identity depending on the gene using BLASTN, and >95% using BLASTX). This further indicates that SM17 and S. albus J1074 are very closely related organisms – possibly even belonging to the same species, while the SM18 and S. pratensis ATCC 33331 are more distantly related.
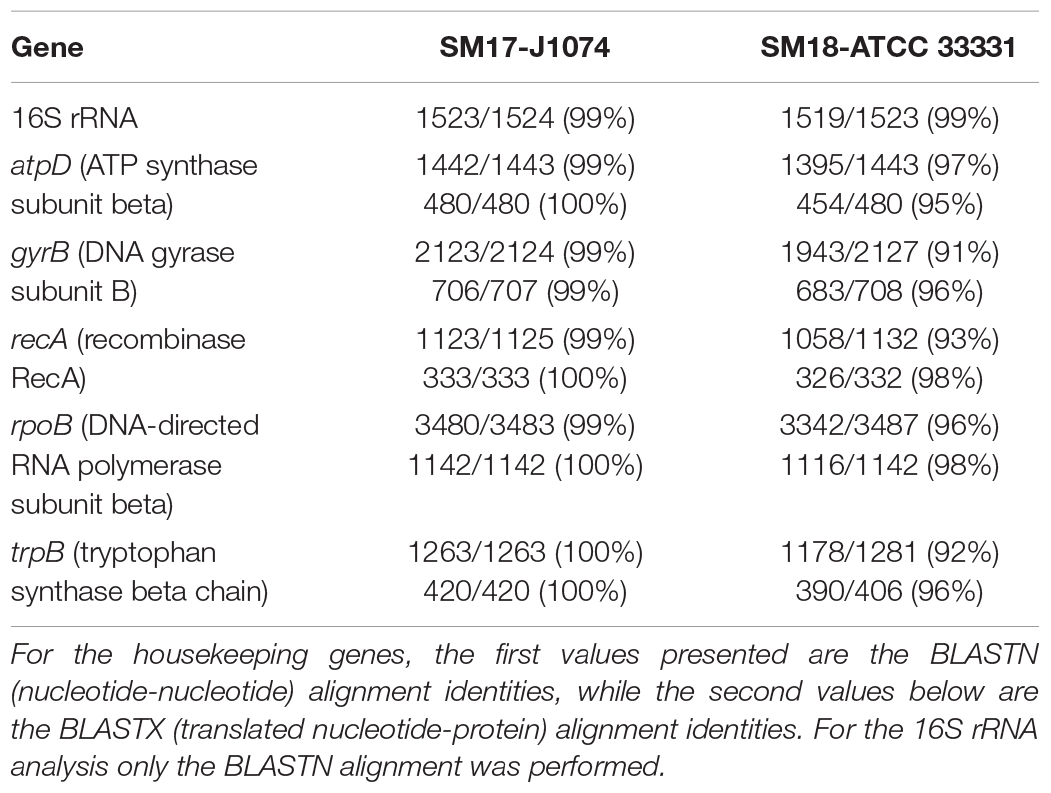
Table 2. 16S rRNA and housekeeping gene alignment comparisons using the NCBI BLAST tool, between the pairs SM17 and S. albus J1074 (second column: SM17-J1074); and SM18 and S. pratensis ATCC 33331 (third column: SM18-ATCC 33331).
Following the 16S rRNA and housekeeping genes analyses, S. albus J1074 and S. pratensis ATCC 33331 were selected for subsequent similarity analysis using a whole-genome alignment approach with the MUMmer program (Supplementary Figure S1). Large sections of the genomes are quite well conserved between the marine sponge-derived isolates and their closest relative organism, particularly when comparing SM17 with S. albus J1074 (Supplementary Figure S1A). This result further confirms previous analyses, and further supports S. albus J1074 and S. pratensis ATCC 33331 as suitable terrestrial relatives, for comparative purposes.
Interestingly, previous studies also reported Streptomyces spp. marine sponge-derived isolates that were determined to be closely related to S. albus J1074 (Ian et al., 2014; Iniyan et al., 2016; Almeida et al., 2018). Some of these strains, namely PVA 94-07; GBA 94-10; and Streptomyces albus ICN33; were isolated from completely different sample types and geographic locations than those of the current study. While SM17, which based on the aforementioned comparative analysis appears to be closely related to S. albus J1074, was isolated from the sponge Haliclona simulans from Kilkieran Bay (Galway, Ireland), at a depth of 15 m; the strains PVA 94-07 and GBA 94-10 were isolated from the sponges Phakellia ventilabrum and Geodia barretti, respectively; from the Tautra ridge (Trondheim fjord, Norway), at a depth of 121 m (Ian et al., 2014), while Streptomyces albus ICN33 was isolated from the sponge Acanthella elongata, from the Colachel coast (Kanyakumari District, Tamil Nadu), at an unspecified depth (Iniyan et al., 2016). This raises the possibility that “albus-like” Streptomyces strains may be ubiquitously associated with marine sponges.
Phenotype, Morphology, and Differential Growth Assessment
Members of the Streptomyces genus are known to be capable of colonizing a wide variety of different ecosystems, including soil, rhizosphere, lake and marine sediments, and have also been reported to be associated with insects, lichen, and sponges (Goodfellow and Fiedler, 2010; Bianchetti et al., 2013; Rashad et al., 2015; Liu et al., 2017; Ay et al., 2018; Jackson et al., 2018). Thus, it is reasonable to assume that these organisms possess a genetic plasticity and capability that facilitates their adaptation to such varied environmental niches (Hoff et al., 2018). Interestingly, previous studies have reported that Streptomyces spp. isolated from marine environments often possess the capacity of growing independently of the presence of sea salts in the growth medium (Goodfellow and Fiedler, 2010; Ian et al., 2014). In fact, many marine isolates often display very active metabolic profiles under such conditions (Goodfellow and Fiedler, 2010). To assess whether the SM17 and SM18 isolates had phenotypical and/or morphological differences with respect to their ability to grow under different conditions, they were cultured in ISP2 medium with and without the presence of ASW and compared with their terrestrial relatives (Figure 3), in a similar fashion to work previously conducted by the Zotchev group (Ian et al., 2014).
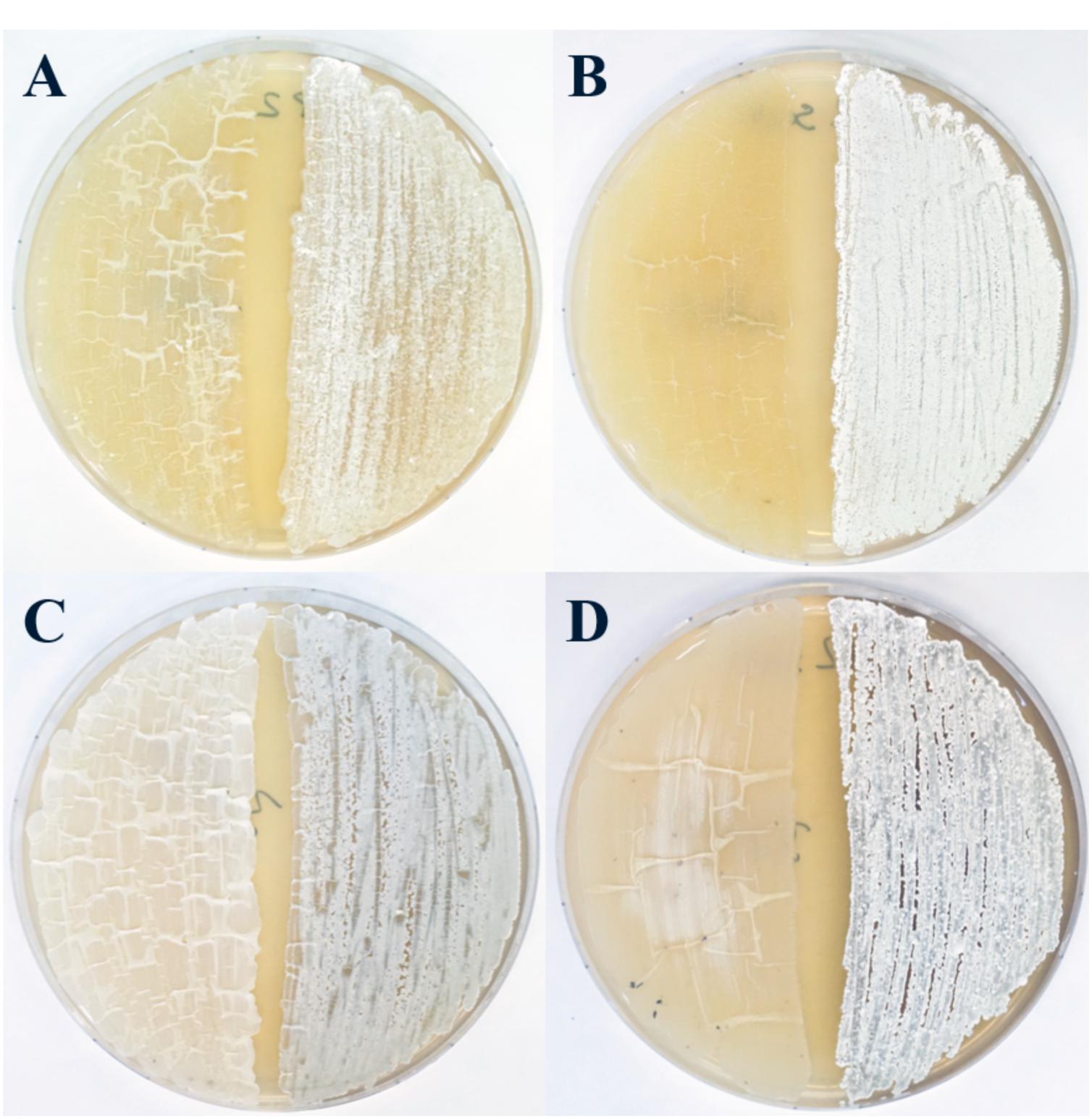
Figure 3. Differential growth assessment of marine and terrestrial Streptomyces strains. From left to right, (A) S. albus J1074 and SM17 on ISP2 agar medium; (B) S. albus J1074 and SM17 on ISP2 + ASW agar medium; (C) S. pratensis ATCC 33331 and SM18 on ISP2 agar medium; (D) S. pratensis ATCC 33331 and SM18 on ISP2 + ASW agar medium, following 3 days growth.
All the pair-wise comparisons showed clear morphological differences between the marine sponge-derived isolates and their respective terrestrial counterparts (Figure 3). All the isolates grew effectively in the ISP2 medium without ASW (Figures 3A,C), even though there were slight differences regarding growth and sporulation; with the SM17 isolate being able to grow and sporulate more rapidly in comparison to S. albus J1074 (Figure 3A). There was no clear difference in the growth of SM18 and S. pratensis ATCC 33331 on the ISP2 growth medium without ASW, although they clearly displayed very different morphological features (Figure 3C). On the other hand, when grown on the ISP2 medium with ASW, S. albus J1074 was clearly less capable of growing in the presence of sea salts, while SM17 thrived (Figure 3B). This result is particularly interesting, since, as previously shown (Figure 2, Table 2, and Supplementary Figure S1), these two organisms are genetically very similar. In contrast, there were less marked differences in the ability of both SM18 and S. pratensis ATCC 33331 to grow in the presence of sea salts (Figure 3D). While SM18 appeared to grow better, nevertheless S. pratensis ATCC 33331 was still able to grow in the ISP2 medium containing sea salts albeit more slowly than SM18; and indeed, more slowly than when S. pratensis ATCC 33331 was cultured in the absence of ASW (Figure 3C). From these observations, it became clear that a more thorough analysis of the SM17 and SM18 genomes might provide some interesting insights regarding potential genome-wide adaptations that may have occurred in these marine isolates, which may have resulted in them being able to grow more efficiently in the ISP2 medium supplemented with ASW; relative to their terrestrial counterparts.
Prediction of Secondary Metabolite Biosynthetic Gene Clusters (smBGCs)
Members of the Actinomycetales order are historically known to produce a broad range of bioactive compounds of biotechnological and clinical interest, and among them, the Streptomyces genus excels, with over 10,000 bioactive compounds produced by members of the genus being discovered to date (Hwang et al., 2014; Ziemert et al., 2016; Kamjam et al., 2017; Lee et al., 2018). The marine sponge-derived SM17 and SM18 strains have previously been reported to possess antimicrobial activity against gram-negative and gram-positive bacteria – including the methicillin-resistant S. aureus (MRSA), and yeasts (Kennedy et al., 2009; Jackson et al., 2018). To provide insights at a genomic level regarding which compounds might be responsible for the previously observed antimicrobial activity, we employed the antiSMASH program in an attempt to predict the presence of putative smBGCs, based on homology to known smBGCs deposited in the databases (Blin et al., 2017). Several gene clusters were predicted to be present in both SM17 and SM18 (Supplementary Tables S1, S2), with a total of 20 potential smBGCs in SM17, and 26 in SM18; with a variety of cluster types being assigned, including: type I polyketide synthases (T1pks), type II polyketide synthases (T2pks), type III polyketide synthases (T3pks), non-ribosomal peptide synthetases (NRPS), lantipeptides, bacteriocins, and terpenes. These types of clusters are known to produce a variety of compounds with antimicrobial activity, including: erythromycin (T1pks); tetracenomycin (T2pks); germicidin (T3pks); daptomycin (NRPS); nisin (lantipeptide/lantibiotic/bacteriocin); and pentalenolactone (terpene) (Shen, 2003; Tetzlaff et al., 2006; Robbel and Marahiel, 2010; Shi et al., 2011; Yamada et al., 2015; Čihák et al., 2017).
The antiSMASH predictions were also further analyzed using the BiG-SCAPE program (Navarro-Muñoz et al., 2018), which allowed us to cluster the predicted smBGCs into gene cluster families (GCFs) based on their sequences and Pfam protein families similarities (El-Gebali et al., 2019), and also to compare them to known smBGCs available from the latest version of the MIBiG repository (version 1.4) (Medema et al., 2015), which can also assist in improving the annotations of the predicted smBGCs. Based on their similarity to known smBGCs, some of the bioactive compounds predicted to be encoded by these smBCGs may be compatible with the previously determined antimicrobial capabilities of the SM17 and SM18 isolates (Figure 4 and Supplementary Tables S1, S2). For example, SM17 appears to possess a candicidin, an antimycin, and a polycyclic tetramate macrolactam cluster (SGR PTMs) (Figure 4 and Supplementary Table S1), with similarity to the candicidin, antimycin and tetramate macrolactam sequences from Streptomyces sp. FR-008, Streptomyces sp. S4 and Streptomyces griseus in the database, respectively, and which are known to have anti-fungal properties (Campelo and Gil, 2002; Chen et al., 2003; Seipke et al., 2011; Luo et al., 2013). SM17 also contains clusters that may potentially encode for the production of surugamides (Figure 4) and the glycopeptide antibiotic mannopeptimycin (Supplementary Table S1), with the former possessing gene similarity with the surugamide A/D sequence from Streptomyces albus in the database (Ninomiya et al., 2016), and the latter sharing similarity to the mannopeptimycin sequence from Streptomyces hygroscopicus in the database, with the main biosynthetic genes being present in the predicted smBGC (Singh et al., 2003; Magarvey et al., 2006). SM18 appears to possess a cluster encoding the anti-bacterial compound bafilomycin (Figure 4 and Supplementary Table S2), with similarity to the bafilomycin sequence from Streptomyces lohii (Bowman et al., 1988; Zhang et al., 2013; Nara et al., 2017); as well other clusters with similarity to known smBGCs that encode anti-fungal and anti-bacterial compounds such as SGR PTMs, curamycin, and caboxamycin (Figure 4), from Streptomyces griseus (Luo et al., 2013), Streptomyces curacoi (Galmarini and Deulofeu, 1961), and Streptomyces sp. NTK 937 (Hohmann et al., 2009; Losada et al., 2017), respectively.
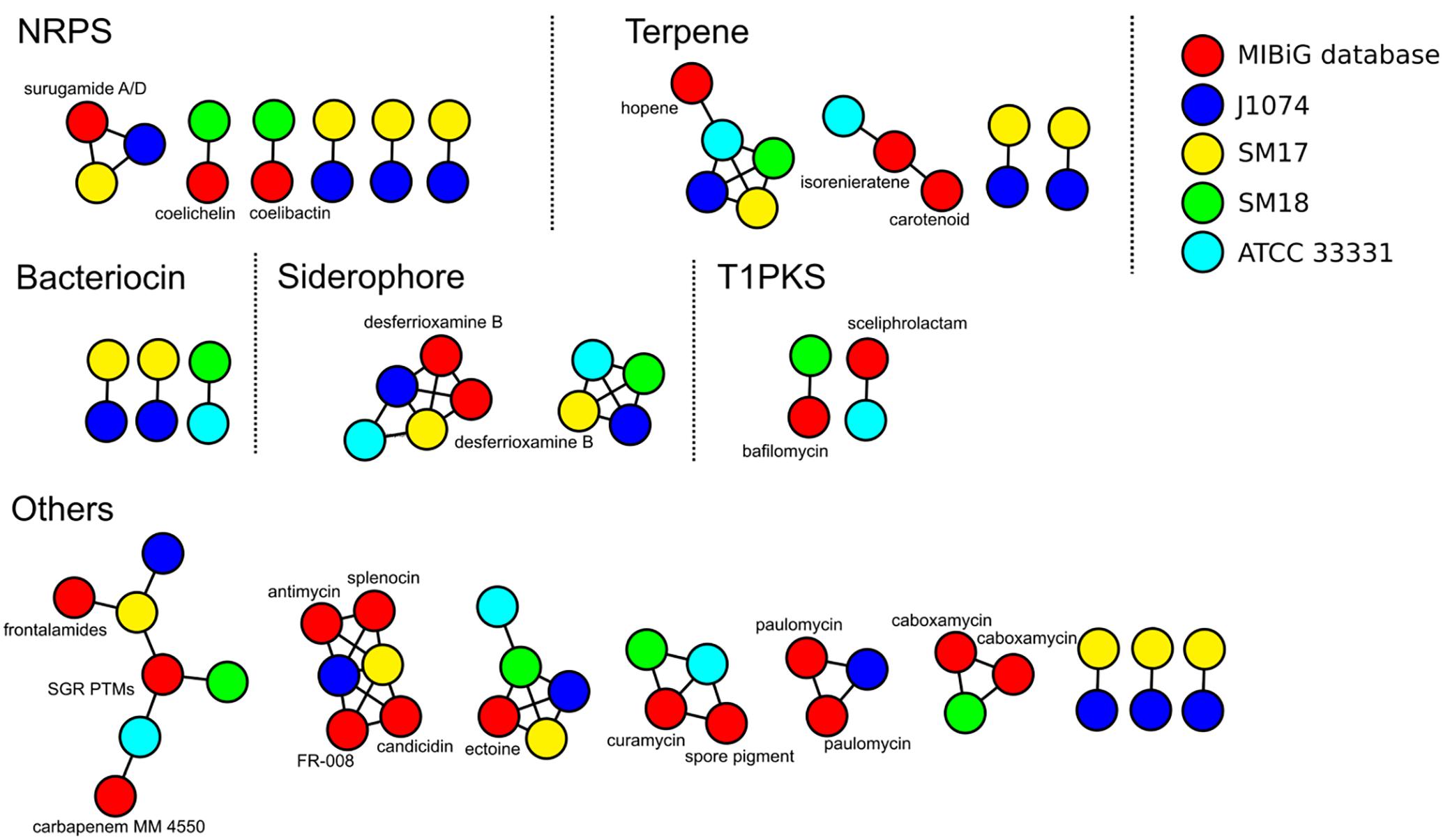
Figure 4. Gene clusters families (GCFs) analysis using antiSMASH (version 4), BiG-SCAPE (version 20181005), MIBiG database (version 1.4), and CytoScape. Each node represents a smBGC predicted in the respective organism (labeled in different colors), and the interactions represent cluster similarity. Annotations of the MIBiG database smBGCs are labeled accordingly. Singletons, i.e., smBGCs without similarities with the smBGCs in the MIBiG database, or without similarities with the smBGCs predicted in the other genomes analyzed in this study, are not included in this figure.
We also performed the antiSMASH and BiG-SCAPE analysis on S. albus J1074 and S. pratensis ATCC 33331 genomes, in an effort to determine to what extent the marine sponge-derived isolates SM17 and SM18 may potentially produce similar and/or unique compounds when compared to their terrestrial counterparts (Figure 4 and Supplementary Tables S3, S4). Based on the BiG-SCAPE similarity clustering, a Venn diagram was generated, representing the presence/absence of GCFs in the SM17, SM18, S. albus J1074, and S. pratensis ATCC 33331 genomes (Figure 5). In keeping with the phylogeny results which indicated that SM17 and S. albus J1074 were very closely related organisms, the smBGCs predictions and similarity clustering results were also strikingly similar (Figures 4, 5). Among a total of 42 predicted smBGCs in both genomes (22 in S. albus J1074 and 20 in SM17), 10 seem to be unique (6 in S. albus J1074, and 4 in SM17) (Figure 5). In contrast, there was a much larger number of predicted unique smBGCs between SM18 and S. pratensis ATCC 33331, where amongst a total of 53 predicted clusters (27 in S. pratensis ATCC 33331 and 26 in SM18), only 6 appear to be present in both genomes; with the majority being potentially unique (20 in S. pratensis ATCC 33331 and 20 in SM18) (Figure 5). Also, a total of 4 smBGCs were shared among all of the strains analyzed (Figure 5), and these were determined to be: hopene; SGR PTMs family of smBGCs, ectoine, and a predicted siderophore smBGC without significant similarity to sequences in the MIBiG database (Figures 4, 5).
Notably, smBGCs encoding the production of desferri- oxamines, which are hydroxamate siderophores, while present in S. albus J1074, S. pratensis ATCC 33331 and SM17 (Figures 4, 5), are absent in the SM18 genome (Supplementary Table S2 and Figure 4). Siderophores are specialized metabolites that function to scavenge Fe3 + , and hence are crucial for sessile organisms to assimilate iron (Hider and Kong, 2010). Genes involved in desferrioxamines production, in particular, are widely conserved in marine microorganisms, and are believed to be present in all Streptomyces species (Tierrafría et al., 2011; Cruz-Morales et al., 2017). Thus, this may be the first report of a Streptomyces isolate that does not possess a smBGC that encodes for the production of desferrioxamines. The SM18 isolate does, however, possess smBGCs encoding other siderophores, such as coelichelin, and mirubactin (Supplementary Table S2 and Figure 4), which may circumvent for the lack of production of desferrioxamines with respect to iron acquisition in the strain.
During processing of the data for this study, a newer version of the antiSMASH webserver (version 5) was released (Blin et al., 2019). Using this new version of antiSMASH did not result in any major differences being detected in the data being analyzed, it did however result in the identification of a smBGC encoding mycemycin in the SM18 genome. Mycemycin is a relatively newly identified compound, from marine and soil Streptomyces isolates, belonging to the dibenzoxazepinone (DBP) family, which possesses HIV-1 reverse transcriptase inhibitory activity (Liu et al., 2015; Song et al., 2018). Production of the DBP family of compounds appear to date to be rare in the microbial world, and these compounds possess a broad range of interesting activities, including anti-HIV and anti-tumor activities (Zhang et al., 2018). Thus, pursuing the identification of new members of this family of compounds may be worthwhile, and it is interesting to report the potential presence of a smBGC encoding the production of mycemycin in another Streptomyces isolate.
Nevertheless, it is clear that further analysis would need to be undertaken to confirm that these compounds are in fact being produced by the SM17 and SM18 isolates, as some of these smBGCs are likely to be cryptic and the compounds may not be produced under certain culture conditions (Rutledge and Challis, 2015; Rigali et al., 2018). Given that SM17 and S. albus J1074 are genetically very similar, it is perhaps reasonable to expect that regulation of secondary metabolite production may to some extent be similar in both strains. Therefore, it may be possible to use what is currently known about the better studied S. albus J1074 isolate to gain a better understanding regarding the expression of certain smBGCs and the metabolic pathways involved in SM17 (Hoz et al., 2017; Kallifidas et al., 2018; Nguyen et al., 2018).
Comparative Genomics
A series of comparative genomics analyses was then performed in order to further characterize the marine sponge-derived isolates SM17 and SM18 at the genome level, and in particular to compare them to their respective closest terrestrial relative.
Analysis of Orthologous Genes
The Roary program was used to determine the pan-genome; the core genome; the accessory genome; and the strain-specific genome (the genes that are uniquely present in only one of the isolates), in the marine sponge-derived isolates Streptomyces sp. strain SM17 and Streptomyces sp. strain SM18, and their respective closest terrestrial relatives S. albus J1074 and S. pratensis ATCCC 33331 (Figure 6) (Page et al., 2015). The pan-genome was determined to consist of 11,305 genes; while the core genome consisted of 3,303 genes (∼29% of the pan-genome); and the accessory genome consisted of 8,002 genes (∼71% of the pan-genome). For the strain-specific genomes, SM17 had 485 unique genes; SM18 had 1,860 unique genes; S. albus J1074 had 258 unique genes; and S. pratensis ATCC 33331 had 1,874 unique genes. This is a combined total of 4,477 unique genes (∼39% of the pan-genome, and ∼56% of the accessory genome). Notably, in keeping with what we had previously observed with the phylogeny and whole-genome alignment analyses, the SM17 and J1074 strains shared a very large number of orthologous genes (a total of 5,515 shared genes, or ∼89% and ∼94% of the SM17 and the J1074 total number of CDSs, respectively), further indicating that they are very closely related organisms. In contrast, SM18 and S. pratensis ATCC 33331 shared a much lower proportion of their genes: 4,469 genes (or ∼67% and ∼66% for the SM18 and ATCC 33331 total number of CDSs, respectively). A total of 64 orthologous genes were found to be commonly present in the marine sponge-derived isolates SM17 and SM18, while absent in their terrestrial counterparts J1074 and ATCC 33331 (Figure 6). Given that they are absent in both terrestrial relatives, we undertook further analyses of these genes to assess their potential function(s) in an effort to provide insights into potential ENAs in both these sponge-derived isolates.
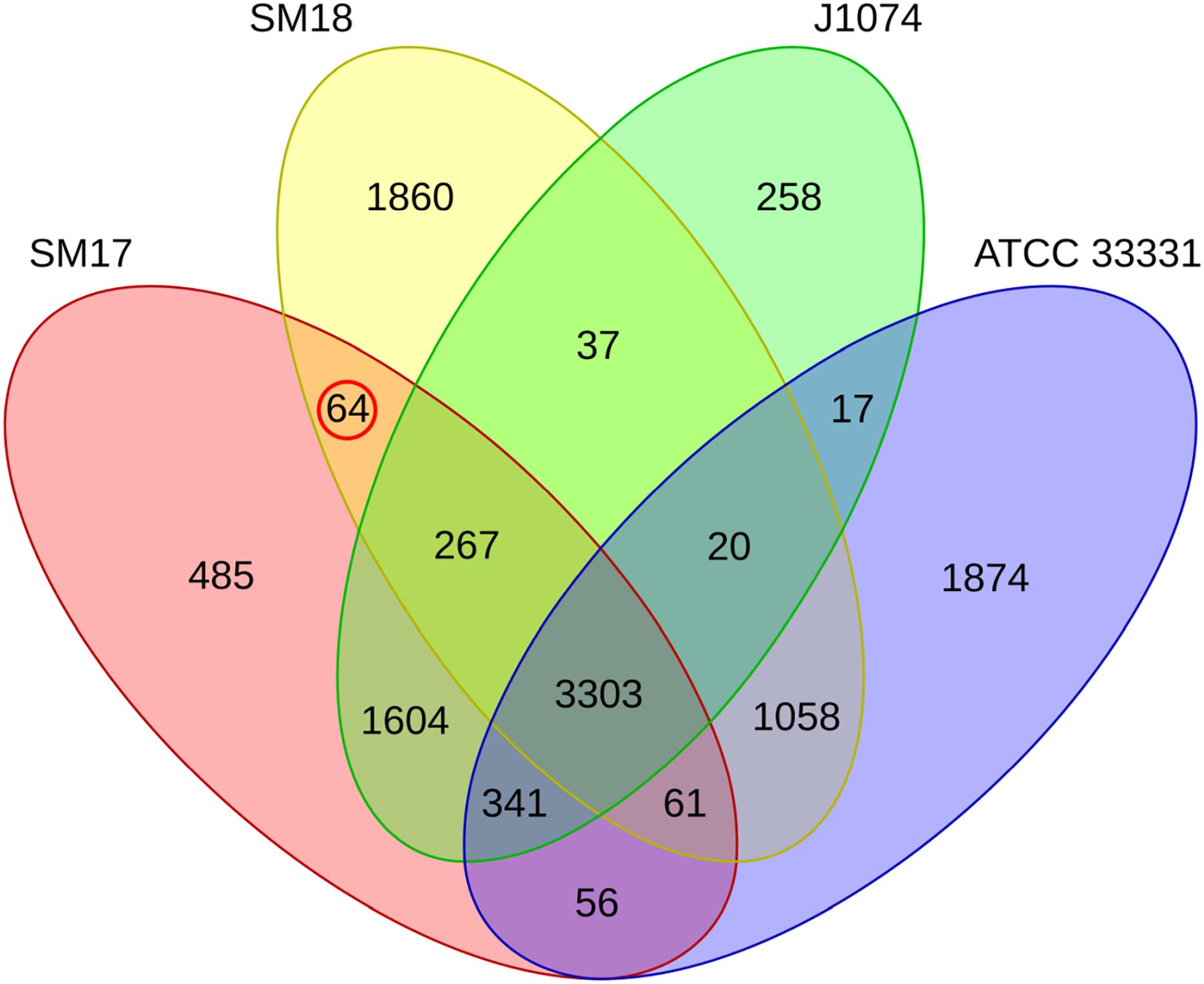
Figure 6. Venn diagram representing the presence/absence of orthologous genes in the SM17, SM18, S. albus J1074, and the S. pratensis ATCC 33331 genomes. Orthologous genes that are present commonly in the marine sponge-derived isolates SM17 and SM18, while absent in their terrestrial counterparts J1074 and ATCC 33331, are circled in red.
Orthology Analysis of smBGC-Associated Genes
The genes previously determined to be associated with smBGCs, using the antiSMASH program, were subsequently analyzed using the Roary program, to identify smBGCs-associated genes which were shared or unique between the four genomes (Supplementary Figure S2) (Page et al., 2015; Blin et al., 2017). With respect to potential smBGCs-associated genes, very few genes appeared to be conserved in all the organisms (a total of 58 genes, corresponding to 2.8% of the total smBGCs-associated gene pool, or 0.017% of the core genome) (Supplementary Figure S2). The largest number of unique smBGCs-associated genes is present in the SM18 isolate (623 genes), followed by S. pratensis ATCC 33331 (485 genes) which may be indicative of a greater potential to produce diverse secondary metabolites in these isolates. In contrast, SM17 and S. albus J1074 appear to possess a lower quantity of unique smBGCs-associated genes; with 132 and 150 unique genes, respectively (Supplementary Figure S2).
Interestingly, when comparing the Venn diagrams from Figure 6 and Supplementary Figure S2, it appears that a large portion of the unique genes present in the isolates are potentially related to the production of secondary metabolites. For SM17, ∼27% (132 out of 485) of the unique genes are potentially smBGCs-associated genes, while for SM18 this percentage is ∼33% (623 out of 1860); ∼58% (150 out of 258) for S. albus J1074; and ∼26% (485 out of 1874) for S. pratensis ATCC 33331. Taken together, these results indicate that, even for closely related Streptomyces spp. isolates (particularly when considering the pair SM17 and S. albus J1074), there is still potential to discover different secondary metabolites from these strains, with potentially unique characteristics. Previous reports have also indicated that the use of closely related Streptomyces strains to identify new smBGCs is useful for the identification of novel specialized biosynthetic pathways (Antony-Babu et al., 2017; Vicente et al., 2018).
Groups of Orthologous Genes Commonly Present in the Marine Sponge-Derived Isolates
Given that the SM17 and SM18 were isolated from a marine sponge and have been shown to be more adapted to higher salinity medium (Figure 3), it is likely that the identification of genes that are commonly present in SM17 and SM18 but not in their terrestrial relatives J1074 and ATCC 33331 may help in the identification of potential ENAs that these strains might possess, at a genetic level. The previous analysis of orthologous genes allowed us to determine which groups of orthologous genes are present commonly in the marine sponge-derived isolates SM17 and SM18, while absent in their terrestrial counterparts J1074 and ATCC 33331, as highlighted in Figure 6. This was performed by taking into account sequence homology and gene synteny (e.g., splitting paralogous genes with the Roary program); hence different copies of a gene can belong to different orthologous group due to potentially different evolutionary events such as gene duplication or lateral gene transfer occurring in the Streptomyces genomes (Zhou et al., 2012; Page et al., 2015). Thus, from here on the orthologous genes will be referred to simply as “genes.” In doing this we identified a potential ENA gene pool which consisted of 64 genes (Table 3). These were then manually annotated using the NCBI GenBank, CDD, UniProt, and the InterPro databases (Johnson et al., 2008; Marchler-Bauer et al., 2011; UniProt Consortium, 2015; Benson et al., 2018; Mitchell et al., 2018), and hypothetical proteins were removed, resulting in a final total of 57 genes (Supplementary Table S5). The ENA gene pool included functional categories of genes that are likely to be related to niche adaptations in the marine sponge-derived isolates, and included a total of 29 genes that could be grouped based on potential biological functions such as osmotic stress, defense; transcriptional regulation; symbiotic interactions; antimicrobial compounds production and resistance; ABC transporters; together with horizontal gene transfer and defense-related features (Table 3).
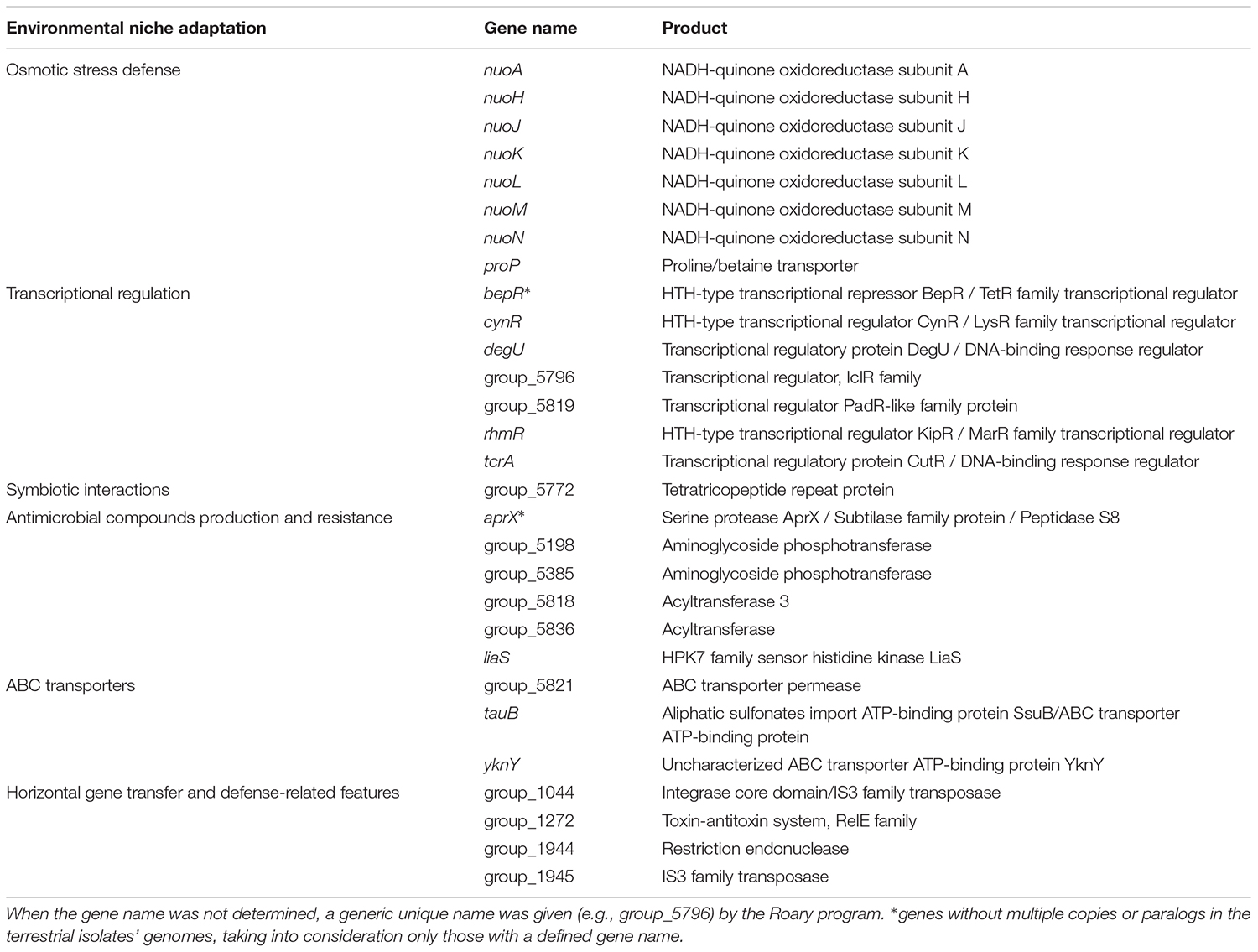
Table 3. Groups of orthologous genes and their respective annotations (excluding hypothetical proteins), which are present commonly in the sponge-derived isolates SM17 and SM18, while absent in their terrestrial counterparts J1074 and ATCC 33331.
Resistance to osmotic stress
For bacteria to survive in marine environments where salinity levels of approximately 3.5% exist, they must be able to simultaneously overcome stresses due to both high osmotic pressure and high Na + concentrations (Yaakop et al., 2016); together with other stresses including pressure, temperature and oligotrophic conditions (Xie et al., 2018). Bacteria typically respond to variations in external osmotic pressure by accumulating or releasing solutes, thereby attenuating water fluxes and maintaining cellular homeostasis (Wood, 2015). The marine sponge-derived isolates SM17 and SM18 appeared to grow and differentiate more rapidly when grown on media containing artificial seawater, when compared to their closely related terrestrial counterparts (Figure 3), thus indicating a potential increased fitness to higher salinity environments, as also previously described in other marine Streptomyces isolates (Ian et al., 2014). Previous studies with marine Actinomycetes, specifically with the genera Salinispora, Streptomyces, and Kocuria, have proposed that the NADH-quinone oxidoreductases nuoAHJKLMN genes, which encode a proton pump, could be classified as potential MAGs (Penn and Jensen, 2012; Ian et al., 2014; Sun et al., 2018). This proton pump is believed to create a proton-motive force which generates ATP, helping to maintain a proton gradient in seawater (Penn and Jensen, 2012; Ian et al., 2014; Sun et al., 2018). We identified the nuoAHJKLMN genes in the ENA gene pool in both SM17 and SM18 (Table 3). Further analysis indicated that both isolates possessed one extra copy of these genes when compared to their terrestrial counterparts, and that these genes were organized in an operon-like structure, similar to that previously reported in Salinispora arenicola CNS-205 and in Kocuria flava S43 (Sun et al., 2018). Furthermore, the same gene synteny for the partial nuo-operon was present in Streptomyces sp. SM17, Streptomyces sp. SM18, Salinispora arenicola CNS-205, Salinispora tropica CNB-440, and Kocuria flava S43 (Figure 7); with nuoA, followed by a hypothetical protein, and then followed by nuoH, nuoJ, nuoK, nuoL, nuoM, and nuoN. It is important, however, to note that differences in sequence identity and reading frames are present (Supplementary Table S6 and Figure 7), which may indicate that different evolutionary events may have occurred in the aforementioned genomes. The presence of this partial nuo-operon in the sponge derived SM17 and SM18 isolates and in the other marine actinomycetes (Salinispora arenicola CNS-205, Salinispora tropica CNB-440, and Kocuria flava S43), which are absent in their terrestrial counterparts J1074 and ATCC 33331, may explain, at least in part, the increased tolerance to salinity we observed in SM17 and SM18 relative to J1074 and ATCC 33331; which although still able to grow in the presence of ASW, grew much more slowly (Figure 3). Another important mechanism which bacteria employ as a defense mechanism against osmotic stress is both the synthesis and the uptake of compatible solutes, such as proline, glycine, betaine and ectoine, in order to maintain membrane turgor pressure (Krämer, 2010; Lim and Lee, 2015). Extra copies of the proP gene, which encodes a potential proline/betaine transporter (ProP), were found in both the SM17 and SM18 strains. It has been shown in E. coli that the ProP transporter acts both as an osmoregulator and as an osmosensor; and is capable of transporting proline, glycine betaine, proline betaine, carnitine, ectoine and other compounds (MacMillan et al., 1999; Roessler and Muller, 2001; Burg and Ferraris, 2008). Therefore, the proP genes may also be related to the increased capacity of the SM17 and SM18 strains to tolerate hyperosmotic environments, as evidenced by their growth on the ASW medium (Figure 3).
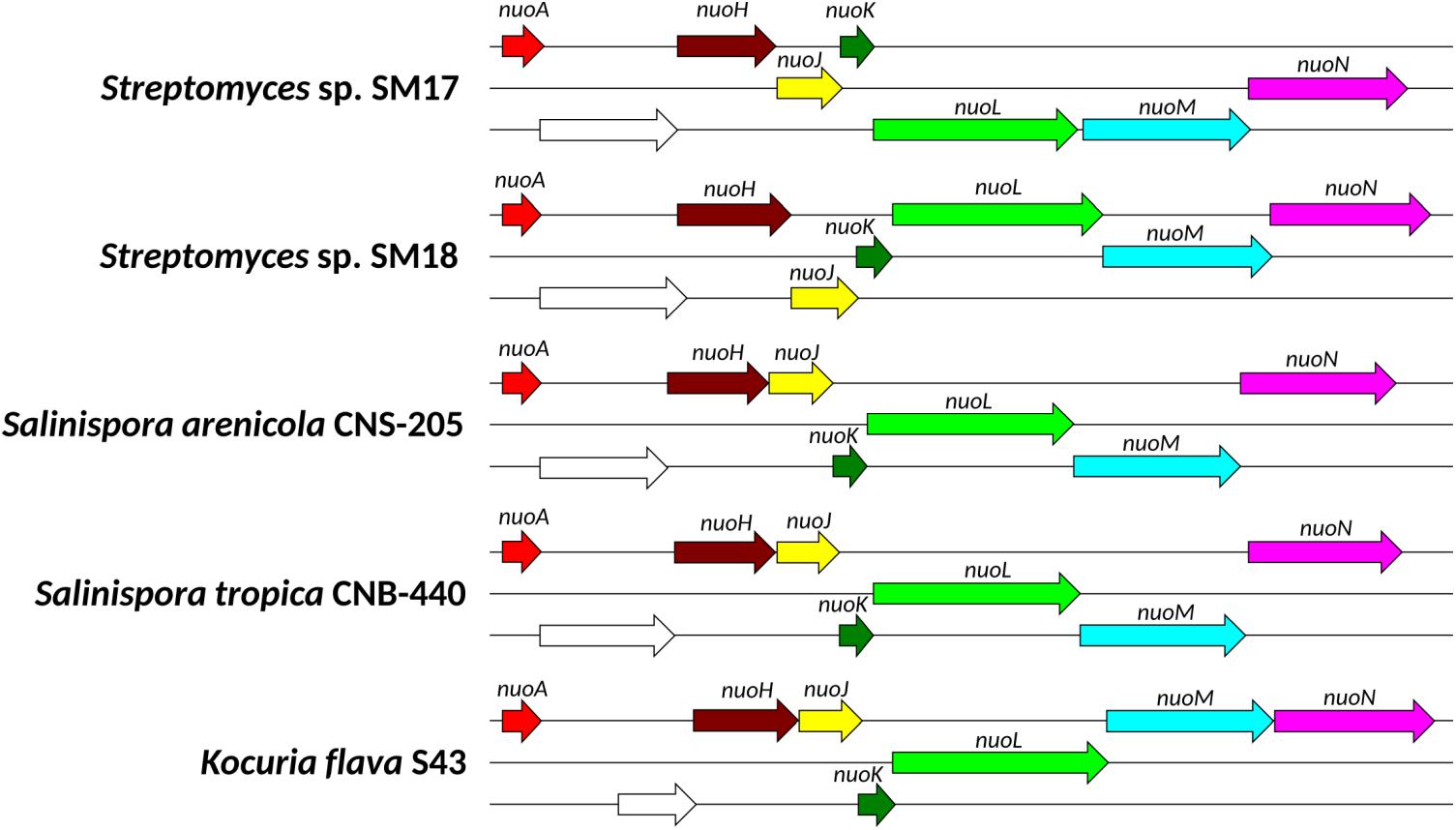
Figure 7. Graphical representation of the gene synteny of the partial nuo-operon present in the genomes of the marine isolates Streptomyces sp. SM17, Streptomyces sp. SM18, Salinispora arenicola CNS-205, Salinispora tropica CNB-440, and Kocuria flava S43, while absent in the terrestrial isolates Streptomyces albus J1074 and Streptomyces pratensis ATCC 33331. Each of the three lines represent a reading frame and the arrows represent a gene, with their respective gene names. Genes with the same color are homologs, while the ones in white are hypothetical proteins with no homologs in the UniProt or PDB databases.
Antimicrobial compounds production and resistance
For many years the main ecological function of antibiotics production in bacteria in natural environments was believed to be inhibition of the growth of other microorganisms, thereby conferring a selective advantage on the producing strain with respect to colonization of particular environmental niches (Linares et al., 2006). In this respect, antibiotic production may be employed as a defense mechanism for the Streptomyces spp. isolates SM17 and SM18 – and other members of the symbiotic community – against other competitor microorganisms in the marine sponge host; as has been previously reported to be the case with other antibiotic producing microorganisms, such as Streptomyces spp. which have been isolated from different hosts including plants and insects (Bondarev et al., 2013; van der Meij et al., 2017; Ceapã et al., 2018; Engl et al., 2018). Furthermore, antibiotics may also play an important role in the overall defense of the sponge host itself by protecting it against pathogens, in a biological interaction defined as defensive symbiosis (Clay, 2014), which has been reported in a number of systems, including beewolf wasps and antibiotic-producing Streptomyces bacteria (Engl et al., 2018). Nevertheless, more recently it has been proposed that in natural environments antibiotics may also act as small molecules with signaling functions, functioning in a similar fashion to quorum sensing molecules; acting for example to alter the expression of genes; to induce biofilm formation; or to modulate colony morphology – all of which may be important in coordinated communication within symbiotic communities (Romero et al., 2011). Thus, antibiotics may have a number of roles in a niche environment such as in marine sponges, which may include both defense-related and signaling roles (Linares et al., 2006; Romero et al., 2011). The presence of a wide variety of predicted smBGCs in both SM17 and SM18 – many of which are potentially involved in the production of antimicrobial compounds (Supplementary Tables S1, S2), coupled with the previously reported antimicrobial activities in these strains (Kennedy et al., 2009; Jackson et al., 2018); supports a possible role for these two Streptomyces spp. isolates in defensive symbiosis in Haliclona simulans, from which they were isolated. In this respect two acyltransferase genes potentially involved in the biosynthesis of type II PKS antibiotics, or type I PKSs that require discrete acyltransferase enzymes, were present in the ENA gene pool (Table 3) (Cheng et al., 2003; Zhang et al., 2017). In addition, a subtilase-like serine protease gene (aprX) was also identified in the ENA gene pool (Table 3), which belongs to a family of proteins that are known to play a number of different biological roles, including involvement in the biosynthesis of antimicrobial peptides, with some possessing algicidal properties, which could potentially be relevant from a sponge defense perspective (Lee et al., 2000; Barra et al., 2017; Montalbán-López et al., 2018). Protease producing marine bacteria are known to be important in the degradation of organic nitrogen which is essential for nitrogen recycling in marine sediments (Zhang et al., 2015). Marine bacterial proteases are also known to play a role in sponge host nitrogen metabolism, which may also explain the presence of this specific protease in the marine isolates SM17 and SM18, and its absence in their terrestrial counterparts (Li et al., 2016; Kiran et al., 2018). Further work on these specific proteases might also be relevant from an industrial perspective, given the interest in proteases of marine origin which are typically cold adapted, salt tolerant, with broad optimal pH values (Li et al., 2016) and which are particularly suited for a number of biotechnological applications, including laundry detergents, food processing, the leather and textile industries, and in waste water treatment applications (Li et al., 2013; Salwan and Sharma, 2018).
A LiaS-encoding gene was also present in the ENA pool, which has previously been reported to be part of the two-component LiaS/LiaR regulatory system, a stress-sensing module that is conserved in Firmicutes bacteria and which is involved in the response to a subset of cell wall-active antibiotics such as bacitracin and vancomycin in Bacillus subtilis; while also being involved in response to cationic antimicrobial peptides and secretion stress (Mascher et al., 2004). In Listeria monocytogenes, the LiaS/R system also plays an important role in resistance to the food preservative nisin (Collins et al., 2012). The presence of antibiotic resistance-related genes in our two sponge-derived isolates may be significant from two perspectives. Firstly, they may function as part of a self-resistance mechanism in these strains, allowing them to be protected from the antimicrobial compounds that they themselves are producing; and/or secondly, as a resistance mechanism to protect themselves from the antimicrobial compounds produced by other microorganisms within the sponge symbiotic community (Wright, 2005, 2012).
ABC transporters
ATP-binding cassette (ABC) transporters are ATP-dependent protein complexes that are widespread in all forms of life and which are vital in mediating the transport of both organic and inorganic molecules across cell membranes (ter Beek et al., 2014; Wilkens, 2015). In bacteria, they confer resistance to antibiotics and to other toxic compounds through efflux/transport mechanisms (Greene et al., 2018); and are also involved in nutrient acquisition and in helping to maintain osmotic balance in the cell (Wood, 2007; Fan et al., 2013; Teichmann et al., 2018). The ENA gene pool includes an yknY-like ABC transporter (Table 3), which has been reported to be involved in the efflux of the sporulation-delaying protein (SDP) in Bacillus spp., although it is still poorly characterized in other genera, such as in Streptomyces (González-Pastor et al., 2003; Xu et al., 2016; Greene et al., 2018). The SDP protein is a killing factor exported by cells that have started the sporulation process, therefore inducing the lysis of sister cells, making more nutrients available, and ultimately delaying the sporulation process and maintaining regular cell growth (González-Pastor et al., 2003). Thus, it is reasonable to assume that the bacterial members of the sponge symbiotic community may employ similar mechanisms and resistance genes targeting these potentially harmful proteins, which may be the case in both SM17 and SM18. A tauB/ssuB-like ABC transporter was also present in the ENA gene pool, which may be responsible in allowing more versatile nutrient acquisition and cycling – specifically for nitrate and sulfonate – for the marine Streptomyces isolates SM17 and SM18, as it has been previously suggested to be the case for marine sponge symbiotic communities, through metagenome binning analysis (Karimi et al., 2018).
Transcriptional regulation
Being able to efficiently respond to changes in their environment is crucial in helping bacteria adapt to and survive within these environments (Feklístov et al., 2014; Daniel-Ivad et al., 2018); and, as previously mentioned, it is particularly important for the sponge-derived bacteria to be able to react appropriately to osmotic and other environmental stresses such as the presence of antibiotics and other potentially harmful compounds; the lack of nutrients; or allowing cell-to-cell communication through quorum sensing.
Transcriptional regulators play a crucial role in allowing bacteria to respond appropriately to numerous environmental stimuli and are believed to be intrinsically linked to lifestyle and environmental adaptation in bacteria (Stock et al., 1990; Feklístov et al., 2014; Daniel-Ivad et al., 2018). Since the SM17 and SM18 isolates inhabit the same niche environment and are subsequently exposed to similar conditions, it is likely that they employ similar adaptive mechanisms in response to those conditions. The ENA gene pool does include a range of transcriptional regulators (Table 3), further indicating that the marine sponge-derived isolates SM17 and SM18 share signal transduction mechanisms that are absent in their terrestrial counterparts, which may account for important niche adaptations that have been acquired. Notably, the TetR, LysR, DegU, IclR, PadR, and CutR families of transcriptional regulators are present in the ENA gene pool. These are commonly associated with mechanisms that could also be potential adaptations employed by the sponge-derived isolates SM17 and SM18, such as for example: antibiotics production (TetR, DegU); antibiotics resistance (TetR, LysR); multidrug resistance (IclR, PadR), quorum sensing (TetR, LysR, IclR); sporulation (IclR); detoxification (PadR); salt stress response (DegU); and copper stress response (CutR) (Huillet et al., 2006; Molina-Henares et al., 2006; Maddocks and Oyston, 2008; Fibriansah et al., 2012; Rademacher and Masepohl, 2012; Cuthbertson and Nodwell, 2013; Rodríguez et al., 2013; Tian et al., 2014; Hoffmann and Bremer, 2016).
For example, a gene encoding a LysR family transcriptional regulator, that is present uniquely in the SM18 genome, is located upstream of a gene which appears to encode a Beta-lactamase enzyme family protein, which are enzymes that provide mechanisms of resistance to β-lactam antibiotics (Majiduddin et al., 2002; Naas et al., 2017). In addition, a gene encoding a IclR family transcriptional regulator that is present in both the SM17 and SM18 genomes, is located upstream of a proP gene, which potentially encodes a proline/betaine transporter and, which previously mentioned, could be related to osmotic regulation in these organisms (MacMillan et al., 1999; Roessler and Muller, 2001; Burg and Ferraris, 2008). While a gene encoding a transcriptional regulator PadR-like family protein which is present in both the SM17 and SM18 genomes; is located upstream of a gene coding an ABC transporter, which as previously mentioned, could be involved with nutrient acquisition, resistance to toxic molecules, or in maintaining osmotic balance in these isolates (Wood, 2007; Fan et al., 2013; Greene et al., 2018; Teichmann et al., 2018).
Genomic evolution through horizontal gene transfer
Horizontal gene transfer (HGT) is an important mechanism in bacterial genome evolution, and commonly involves the acquisition of mobile genetic elements (MGEs) (Bellanger et al., 2014). Previous studies have reported that the genomes of symbiotic bacteria – including sponge symbionts – possess a higher number of MGEs than those of free-living microorganisms (Thomas et al., 2010; Fan et al., 2012, 2013). It has been proposed that MGEs play a crucial role in co-evolution with the host and convergent evolution of marine sponge symbiotic communities in a number of ways, such as enabling the members of the symbiotic community to share important traits for niche adaptation (Fan et al., 2012), such as for example genes related to stress tolerance, antibiotics resistance, and nutrient acquisition. In addition, the MGEs can function in the deactivation or removal of non-essential genes, such as those that are only required by free-living bacteria, or those related to functions that are already being performed by other members of the symbiotic community (Fan et al., 2012). Two genes encoding transposases were found in the ENA gene pool (Table 3), indicating that they may be involved in HGT events and co-evolution between the marine sponge isolates SM17 and SM18. Also, the three plasmids that were identified in SM17 (Table 1B and Figure 1), which are absent in its terrestrial counterpart S. albus J1074, provide additional evidence of potential genomic evolution through transferable elements occurring within the marine sponge microbiota.
The high filter feeding rates of sponges mean that they are likely to be exposed to phage attack from the plankton, and that bacterial sponge symbionts may be subjected to phage-mediated transduction which can lead to cell lysis (Thomas et al., 2010). Therefore, it might be expected that sponge bacterial symbiotic communities would require defense mechanisms to protect themselves from foreign DNA, such as restriction modification (R-M) systems and toxin-antitoxin (T-A) systems (Fan et al., 2012; Horn et al., 2016; Slaby et al., 2017). R-M systems are also linked to MGEs in that they can be transferred via the MGEs, or they can act as MGEs in transposon-like structures (Furuta and Kobayashi, 2013). In the ENA gene pool, we identified one restriction endonuclease that could be part of a transferrable R-M system and one T-A system gene from the RelE family in SM17 and SM18 (Table 3). This further highlights the possibility that HGT events may be occurring between the sponge-derived isolates and the possibility of shared niche adaptations between them, and also the requirement for defense mechanisms against foreign DNA in the symbiotic bacteria. Importantly, T-A systems have also been proposed to provide mechanisms to cope with stress – such as nutrient stress – by either programmed cell death or by inducing bacteriostasis, which may be another important role played by the T-A systems in symbiotic communities in oligotrophic environments (Van Melderen, 2010; de Goeij et al., 2013).
Eukaryotic-like proteins and potential host interaction
Metagenomic and genomic studies have reported that bacterial symbionts contain a large number of genes encoding for eukaryotic-like proteins (ELPs) (Reynolds and Thomas, 2016). ELPs contain repeat domains that are commonly found in eukaryotic proteins, such as tetratricopeptide repeats (TPRs), and are believed to play an important role in symbiotic relationships, by mediating protein-protein interactions for a range of cellular proteins (Thomas et al., 2010; Li et al., 2015; Reynolds and Thomas, 2016). These ELPs may have a broader function in mediating bacterial-sponge interactions and may modulate the host’s behavior (Li et al., 2015; Reynolds and Thomas, 2016). The ENA gene pool contained a tetratricopeptide repeat-containing protein, which is a class of ELP that has been proposed to function as a means for symbiotic bacteria to avoid digestion, or as a mechanism for the sponge to distinguish between food and symbionts (Thomas et al., 2010). The fact that the relatively phylogenetically distant SM17 and SM18 isolates possess orthologs of the same TPR, while their closest terrestrial relatives do not; suggests that this protein may indeed play a role in the symbiotic interactions between these bacteria and their sponge host Haliclona simulans.
ENA Gene Pool Genes Commonly Present in Other Environmental Streptomyces Isolates
In a similar fashion to the aforementioned analysis of orthologous genes, an additional analysis was performed, including the genomes from the other isolates previously determined to belong to the SM17 and SM18 phylogenetic clades (Figure 2). The aim was to assess whether genes present in the SM17 and SM18’s ENA gene pool are also present in other closely related relatives derived from other diverse environments, given the possibility that they may possess adaptations to their particular environmental niches that overlap with those identified in our marine sponge-associated SM17 and SM18 strains.
In the previously identified SM17 clade (Figure 2), in addition to its closely related terrestrial type-strain J1074, the clade also included the environmental isolates Streptomyces albidoflavus SM254, which was isolated from copper-rich subsurface fluids within an iron mine (Badalamenti et al., 2016); Streptomyces sampsonii KJ40, which was isolated from rhizosphere soil in a poplar plantation (Li et al., 2018); Streptomyces koyangensis VK-A60T, which was isolated from rhizosphere soil in a radish plantation (Lee et al., 2005); and Streptomyces sp. CLI2509, which is a fungus-derived isolate (Wyche et al., 2017). It is important to note that the SM17 clade also included the Streptomyces sp. FR-008 strain, however, this strain was not included in the analysis since it does not appear to be an environmental isolate, and it is a product of protoplast breeding of strains with little information in the literature regarding their isolation source (NCBI BioSample: SAMN03120580). The SM18 clade (Figure 2), in addition to its closely related terrestrial type-strain ATCC 33331, also included the environmental isolates Streptomyces sp. PAMC26508, which is an endosymbiotic bacterium isolated from the Antarctic lichen Cladonia borealis (Shin et al., 2013); Streptomyces sp. S501, isolated in sediment from a seaside wetland (NCBI BioSample: SAMN10144670); and Streptomyces sp. SirexAA-E, isolated from an insect/microbe symbiotic community (Bianchetti et al., 2013).
Interestingly, the majority of the genes present in the ENA gene pool were also present in the genomes of the other isolates. This is perhaps not surprising given the potential similarity in environmental stresses that these isolates may encounter, as the marine sponge-associated SM17 and SM18 strains; since they were all isolated from either (1) symbiotic communities, (2) high osmotic pressure environments and/or 3) aquatic environments. For example, the aforementioned nuo operon genes (Figure 7); potentially involved in adaptation to osmotic stress, are also present in the KJ40, the VK-A60T, and SM254 strains from the SM17 clade (Figure 2). It is well documented that osmoadaptation is an important trait possessed by rhizosphere-derived bacteria, since water uptake and exclusion of solutes such as Na+ and Cl– by plants roots are likely to induce changes in osmolarity (Miller and Wood, 1996; Qurashi and Sabri, 2011), and for that reason salt-tolerant bacterium are commonly isolated from plant rhizospheres (Yuwono, 2005; Qurashi and Sabri, 2011). Thus, it is reasonable to assume that the presence of the nuo operon genes in the KJ40 and in the VK-A60T strains, both rhizosphere-derived isolates, may also be related to an increased resistance to osmotic stress, as it also seems to be the case to our marine sponge-derived isolates. Likewise, it is also possible that the SM254 strain, isolated from copper-rich subsurface fluids in an iron mine, will be exposed to osmotic stress and hence require appropriate adaptations to these conditions. Hence, it is plausible that the genes encoded in the nuo operon are not an adaptive response that is exclusively employed by some marine bacteria, as previously suggested (Penn and Jensen, 2012; Ian et al., 2014; Sun et al., 2018), but rather a more general mechanism of osmoadaptation that may be employed by bacteria in other environments as well.
Similarly, proP gene homologs were also present in all of the other genomes analyzed, with exception to the fungus-derived CLI2905 strain from the SM17 clade (Figure 2). Thus, given as has been previously discussed, that ProP acts both as an osmoregulator and as an osmosensor, together with transporting compatible solutes in E. coli; it may also be related to osmoadaptation in these isolates. These observations further highlight the potential adaptations which have been proposed in the ENA gene pool, that may be present in these other closely related relatives derived from other diverse environments, which may overlap with those identified in our marine sponge-associated SM17 and SM18 strains.
Conclusion
The Streptomyces genus is exceptionally important when it comes to the identification and production of bioactive molecules, but those derived from the marine environment are currently particularly not well characterized. This study provides novel insights into possible ENAs employed by Streptomyces spp. isolated from marine sponges, and how these are potentially linked to diverse secondary metabolite biosynthesis. By providing high quality genomic information for the SM17 and SM18 strains isolated from Haliclona simulans, which have been previously shown to have antimicrobial activity against important pathogens, we were able to perform several comparative analyses with their terrestrial counterparts S. albus J1074 and S. pratensis ATCC 33331. The genomic analyses identified a diversity of putative smBGCs, which could potentially explain the previously determined antimicrobial activities reported for these marine isolates, such as smBGCs potentially encoding the production of candicidin, antimycin, SGR PTMs, surugamides, and mannopeptimycin, in SM17; and smBGCs potentially encoding the production of bafilomycin, SGR PTMs, curamycin, and caboxamycin, in SM18. Several smBGCs appear to be unique in the marine isolates in comparison to their terrestrial counterparts, which is particularly true in the case of the Streptomyces sp. SM18 isolate, when compared to S. pratensis ATCC 33331. Interestingly, while SM18 contains smBGCs encoding the production of siderophores such as coelichelin and mirubactin, it lacks the smBGC encoding the production of desferrioxamines; which is to our knowledge the first report of a Streptomyces isolate lacking this capacity. Comparative genomics analysis allowed us to identify genes that could be involved in mechanisms that may be relevant for their adaptation to their particular environmental niche, including resistance to osmotic stress; transcriptional regulation; symbiotic interactions; antimicrobial compounds production and resistance; ABC transporters; and HGT and other potential defense-related features. Expanding on the genetic knowledge of these organisms and their underlying mechanisms of adaptability is important, in not only allowing us to gain a better understanding of marine bacteria and their evolution, but also in helping with the discovery of potential new bioactive small molecules and in how to potentially manipulate and optimize their production.
Data Availability
The complete genome sequences of SM17, SM18 and the plasmid sequences pSM17A, pSM17B, and pSM17C have been deposited in GenBank under the accession numbers CP029338, CP029342, CP029339, CP029340, and CP029341 respectively. The closest reference genomes used in this study for comparative purposes were S. albus J1074 (accession no. CP004370.1) and S. pratensis ATCC 33331 (accession no. CP002475.1).
Author Contributions
EA, AC, SJ, and AD conceived and designed the experiments and analyzed the data. EA and AC performed the experiments. EA and AD wrote the manuscript.
Funding
EA acknowledges the funding support of the Brazilian National Council for Scientific and Technological Development (CNPq). The project was supported by a research grant from the Marine Institute through the NMBLI project (Grant-Aid Agreement PBA/MB/16/01) and by the Marine Biotechnology ERA/NET, NEPTUNA project (Contract No. PBA/MB/15/02) and by Science Foundation Ireland (SSPC-2, 12/RC/2275).
Conflict of Interest Statement
The authors declare that the research was conducted in the absence of any commercial or financial relationships that could be construed as a potential conflict of interest.
Supplementary Material
The Supplementary Material for this article can be found online at: https://www.frontiersin.org/articles/10.3389/fmicb.2019.01713/full#supplementary-material
Footnotes
References
Abdelmohsen, U. R., Bayer, K., and Hentschel, U. (2014). Diversity, abundance and natural products of marine sponge-associated actinomycetes. Nat. Prod. Rep. 31, 381–399. doi: 10.1039/C3NP70111E
Adnani, N., Rajski, S. R., and Bugni, T. S. (2017). Symbiosis-inspired approaches to antibiotic discovery. Nat. Prod. Rep. 34, 784–814. doi: 10.1039/C7NP00009J
Almeida, E. L., Margassery, L. M., Kennedy, J., and Dobson, A. D. W. (2018). Draft genome sequence of the antimycin-producing bacterium Streptomyces sp. Strain SM8, isolated from the marine sponge Haliclona simulans. Genome Announc. 6:e01535-17. doi: 10.1128/genomeA.01535-17
Antony-Babu, S., Stien, D., Eparvier, V., Parrot, D., Tomasi, S., and Suzuki, M. T. (2017). Multiple Streptomyces species with distinct secondary metabolomes have identical 16S rRNA gene sequences. Sci. Rep. 7:11089. doi: 10.1038/s41598-017-11363-1
Ay, H., Nouioui, I., del Carmen Montero-Calasanz, M., Klenk, H.-P., Isik, K., Cetin, D., et al. (2018). Streptomyces sediminis sp. nov. isolated from crater lake sediment. Antonie Van Leeuwenhoek 111, 493–500. doi: 10.1007/s10482-017-0970-z
Badalamenti, J. P., Erickson, J. D., and Salomon, C. E. (2016). Complete genome sequence of Streptomyces albus SM254, a potent antagonist of bat white-nose syndrome pathogen Pseudogymnoascus destructans. Genome Announc. 4:e00290-16. doi: 10.1128/genomeA.00290-16
Baker, P. W., Kennedy, J., Dobson, A. D. W., and Marchesi, J. R. (2009). Phylogenetic diversity and antimicrobial activities of fungi associated with Haliclona simulans isolated from Irish coastal waters. Mar. Biotechnol. 11, 540–547. doi: 10.1007/s10126-008-9169-7
Barnett, D. W., Garrison, E. K., Quinlan, A. R., Stromberg, M. P., and Marth, G. T. (2011). BamTools: a C++ API and toolkit for analyzing and managing BAM files. Bioinformatics 27, 1691–1692. doi: 10.1093/bioinformatics/btr174
Barra, L., Barac, P., König, G. M., Crüsemann, M., and Dickschat, J. S. (2017). Volatiles from the fungal microbiome of the marine sponge Callyspongia cf. flammea. Org. Biomol. Chem. 15, 7411–7421. doi: 10.1039/C7OB01837A
Bellanger, X., Payot, S., Leblond-Bourget, N., and Guédon, G. (2014). Conjugative and mobilizable genomic islands in bacteria: evolution and diversity. FEMS Microbiol. Rev. 38, 720–760. doi: 10.1111/1574-6976.12058
Benson, D. A., Cavanaugh, M., Clark, K., Karsch-Mizrachi, I., Ostell, J., Pruitt, K. D., et al. (2018). GenBank. Nucleic Acids Res. 46, D41–D47. doi: 10.1093/nar/gkx1094
Bentley, S. D., Chater, K. F., Cerdeño-Tárraga, A.-M., Challis, G. L., Thomson, N. R., James, K. D., et al. (2002). Complete genome sequence of the model actinomycete Streptomyces coelicolor A3(2). Nature 417, 141–147. doi: 10.1038/417141a
Bianchetti, C. M., Harmann, C. H., Takasuka, T. E., Hura, G. L., Dyer, K., and Fox, B. G. (2013). Fusion of dioxygenase and lignin-binding domains in a novel secreted enzyme from cellulolytic Streptomyces sp. SirexAA-E. J. Biol. Chem. 288, 18574–18587. doi: 10.1074/jbc.M113.475848
Blin, K., Shaw, S., Steinke, K., Villebro, R., Ziemert, N., Lee, S. Y., et al. (2019). antiSMASH 5.0: updates to the secondary metabolite genome mining pipeline. Nucleic Acids Res. 47, W81–W87. doi: 10.1093/nar/gkz310
Blin, K., Wolf, T., Chevrette, M. G., Lu, X., Schwalen, C. J., Kautsar, S. A., et al. (2017). antiSMASH 4.0—improvements in chemistry prediction and gene cluster boundary identification. Nucleic Acids Res. 45, W36–W41. doi: 10.1093/nar/gkx319
Bondarev, V., Richter, M., Romano, S., Piel, J., Schwedt, A., and Schulz-Vogt, H. N. (2013). The genus Pseudovibrio contains metabolically versatile bacteria adapted for symbiosis. Environ. Microbiol. 15, 2095–2113. doi: 10.1111/1462-2920.12123
Bowman, E. J., Siebers, A., and Altendorf, K. (1988). Bafilomycins: a class of inhibitors of membrane ATPases from microorganisms, animal cells, and plant cells. Proc. Natl. Acad. Sci. U.S.A. 85, 7972–7976. doi: 10.1073/pnas.85.21.7972
Burg, M. B., and Ferraris, J. D. (2008). Intracellular organic osmolytes: function and regulation. J. Biol. Chem. 283, 7309–7313. doi: 10.1074/jbc.R700042200
Campelo, A. B., and Gil, J. A. (2002). The candicidin gene cluster from Streptomyces griseus IMRU 3570. Microbiology 148, 51–59. doi: 10.1099/00221287-148-1-51
Carver, T., Thomson, N., Bleasby, A., Berriman, M., and Parkhill, J. (2009). DNAPlotter: circular and linear interactive genome visualization. Bioinformatics 25, 119–120. doi: 10.1093/bioinformatics/btn578
Ceapã, C. D., Vázquez-Hernández, M., Rodríguez-Luna, S. D., Cruz Vázquez, A. P., Jiménez Suárez, V., Rodríguez-Sanoja, R., et al. (2018). Genome mining of Streptomyces scabrisporus NF3 reveals symbiotic features including genes related to plant interactions. PLoS One 13:e0192618. doi: 10.1371/journal.pone.0192618
Chater, K. F., and Wilde, L. C. (1976). Restriction of a bacteriophage of Streptomyces albus G involving endonuclease SalI. J. Bacteriol. 128, 644–650.
Chater, K. F., and Wilde, L. C. (1980). Streptomyces albus G mutants defective in the SalGI restriction-modification system. Microbiology 116, 323–334. doi: 10.1099/00221287-116-2-323
Chen, S., Huang, X., Zhou, X., Bai, L., He, J., Jeong, K. J., et al. (2003). Organizational and mutational analysis of a complete FR-008/candicidin gene cluster encoding a structurally related polyene complex. Chem. Biol. 10, 1065–1076. doi: 10.1016/J.CHEMBIOL.2003.10.007
Cheng, C., Othman, E., Stopper, H., Edrada-Ebel, R., Hentschel, U., Abdelmohsen, U., et al. (2017). Isolation of petrocidin a, a new cytotoxic cyclic dipeptide from the marine sponge-derived bacterium Streptomyces sp. SBT348. Mar. Drugs 15, 383. doi: 10.3390/md15120383
Cheng, Y.-Q., Tang, G.-L., and Shen, B. (2003). Type I polyketide synthase requiring a discrete acyltransferase for polyketide biosynthesis. Proc. Natl. Acad. Sci. U.S.A. 100, 3149–3154. doi: 10.1073/pnas.0537286100
Choulet, F., Aigle, B., Gallois, A., Mangenot, S., Gerbaud, C., Truong, C., et al. (2006a). Evolution of the terminal regions of the Streptomyces linear chromosome. Mol. Biol. Evol. 23, 2361–2369. doi: 10.1093/molbev/msl108
Choulet, F., Gallois, A., Aigle, B., Mangenot, S., Gerbaud, C., Truong, C., et al. (2006b). Intraspecific variability of the terminal inverted repeats of the linear chromosome of Streptomyces ambofaciens. J. Bacteriol. 188, 6599–6610. doi: 10.1128/JB.00734-06
Čihák, M., Kameník, Z., Šmídová, K., Bergman, N., Benada, O., Kofroňová, O., et al. (2017). Secondary metabolites produced during the germination of Streptomyces coelicolor. Front. Microbiol. 8:2495. doi: 10.3389/fmicb.2017.02495
Clay, K. (2014). Defensive symbiosis: a microbial perspective. Funct. Ecol. 28, 293–298. doi: 10.1111/1365-2435.12258
Collins, B., Guinane, C. M., Cotter, P. D., Hill, C., and Ross, R. P. (2012). Assessing the contributions of the LiaS histidine kinase to the innate resistance of Listeria monocytogenes to nisin, cephalosporins, and disinfectants. Appl. Environ. Microbiol. 78, 2923–2929. doi: 10.1128/AEM.07402-11
Cruz-Morales, P., Ramos-Aboites, H. E., Licona-Cassani, C., Selem-Mójica, N., Mejía-Ponce, P. M., Souza-Saldívar, V., et al. (2017). Actinobacteria phylogenomics, selective isolation from an iron oligotrophic environment and siderophore functional characterization, unveil new desferrioxamine traits. FEMS Microbiol. Ecol. 93:fix086. doi: 10.1093/femsec/fix086
Cuthbertson, L., and Nodwell, J. R. (2013). The TetR family of regulators. Microbiol. Mol. Biol. Rev. 77, 440–475. doi: 10.1128/MMBR.00018-13
Dalisay, D. S., Williams, D. E., Wang, X. L., Centko, R., Chen, J., and Andersen, R. J. (2013). Marine sediment-derived Streptomyces bacteria from British Columbia, Canada are a promising microbiota resource for the discovery of antimicrobial natural products. PLoS One 8:e77078. doi: 10.1371/journal.pone.0077078
Daniel-Ivad, M., Pimentel-Elardo, S., and Nodwell, J. R. (2018). Control of specialized metabolism by signaling and transcriptional regulation: opportunities for new platforms for drug discovery? Annu. Rev. Microbiol. 72, 25–48. doi: 10.1146/annurev-micro-022618-042458
de Goeij, J. M., van Oevelen, D., Vermeij, M. J. A., Osinga, R., Middelburg, J. J., de Goeij, A. F. P. M., et al. (2013). Surviving in a marine desert: the sponge loop retains resources within coral reefs. Science 342, 108–110. doi: 10.1126/science.1241981
Dusa, A. (2018). venn : Draw Venn Diagrams. Available at: https://cran.r-project.org/package=venn (accessed January 15, 2019).
El-Gebali, S., Mistry, J., Bateman, A., Eddy, S. R., Luciani, A., Potter, S. C., et al. (2019). The Pfam protein families database in 2019. Nucleic Acids Res. 47, D427–D432. doi: 10.1093/nar/gky995
Engl, T., Kroiss, J., Kai, M., Nechitaylo, T. Y., Svatoš, A., and Kaltenpoth, M. (2018). Evolutionary stability of antibiotic protection in a defensive symbiosis. Proc. Natl. Acad. Sci. U.S.A. 115, E2020–E2029. doi: 10.1073/pnas.1719797115
Fan, L., Liu, M., Simister, R., Webster, N. S., and Thomas, T. (2013). Marine microbial symbiosis heats up: the phylogenetic and functional response of a sponge holobiont to thermal stress. ISME J. 7, 991–1002. doi: 10.1038/ismej.2012.165
Fan, L., Reynolds, D., Liu, M., Stark, M., Kjelleberg, S., Webster, N. S., et al. (2012). Functional equivalence and evolutionary convergence in complex communities of microbial sponge symbionts. Proc. Natl. Acad. Sci. U.S.A. 109, E1878–E1887. doi: 10.1073/pnas.1203287109
Feklístov, A., Sharon, B. D., Darst, S. A., and Gross, C. A. (2014). Bacterial sigma factors: a historical, structural, and genomic perspective. Annu. Rev. Microbiol. 68, 357–376. doi: 10.1146/annurev-micro-092412-155737
Fibriansah, G., Kovács, ÁT., Pool, T. J., Boonstra, M., Kuipers, O. P., and Thunnissen, A.-M. (2012). Crystal structures of two transcriptional regulators from Bacillus cereus Define the conserved structural features of a PadR subfamily. PLoS One 7:e48015. doi: 10.1371/journal.pone.0048015
Flemer, B., Kennedy, J., Margassery, L. M., Morrissey, J. P., O’Gara, F., and Dobson, A. D. W. (2012). Diversity and antimicrobial activities of microbes from two Irish marine sponges, Suberites carnosus and Leucosolenia sp. J. Appl. Microbiol. 112, 289–301. doi: 10.1111/j.1365-2672.2011.05211.x
Fuerst, J. A. (2014). Diversity and biotechnological potential of microorganisms associated with marine sponges. Appl. Microbiol. Biotechnol. 98, 7331–7347. doi: 10.1007/s00253-014-5861-x
Furuta, Y., and Kobayashi, I. (2013). “Restriction-modification systems as mobile epigenetic elements,” in Madame Curie Bioscience Database, eds A. P. Roberts and P. Mullany (Austin, TX: Landes Bioscience).
Gadagkar, S. R., Rosenberg, M. S., and Kumar, S. (2005). Inferring species phylogenies from multiple genes: concatenated sequence tree versus consensus gene tree. J. Exp. Zool. Part B Mol. Dev. Evol. 304B, 64–74. doi: 10.1002/jez.b.21026
Galmarini, O. L., and Deulofeu, V. (1961). Curamycin—I: isolation and characterization of some hydrolysis products. Tetrahedron 15, 76–86. doi: 10.1016/0040-4020(61)80010-0
Gomez-Escribano, J. P., Castro, J. F., Razmilic, V., Chandra, G., Andrews, B., Asenjo, J. A., et al. (2015). The Streptomyces leeuwenhoekii genome: de novo sequencing and assembly in single contigs of the chromosome, circular plasmid pSLE1 and linear plasmid pSLE2. BMC Genomics 16:485. doi: 10.1186/s12864-015-1652-8
González-Pastor, J. E., Hobbs, E. C., and Losick, R. (2003). Cannibalism by sporulating bacteria. Science 301, 510–513. doi: 10.1126/science.1086462
Goodfellow, M., and Fiedler, H. P. (2010). A guide to successful bioprospecting: informed by actinobacterial systematics. Antonie van Leeuwenhoek 98, 119–142. doi: 10.1007/s10482-010-9460-2
Goshi, K., Uchida, T., Lezhava, A., Yamasaki, M., Hiratsu, K., Shinkawa, H., et al. (2002). Cloning and analysis of the telomere and terminal inverted repeat of the linear chromosome of Streptomyces griseus. J. Bacteriol. 184, 3411–3415. doi: 10.1128/JB.184.12.3411-3415.2002
Greene, N. P., Kaplan, E., Crow, A., and Koronakis, V. (2018). Antibiotic resistance mediated by the MacB ABC transporter family: a structural and functional perspective. Front. Microbiol. 9:950. doi: 10.3389/fmicb.2018.00950
Gromek, S. M., Suria, A. M., Fullmer, M. S., Garcia, J. L., Gogarten, J. P., Nyholm, S. V., et al. (2016). Leisingera sp. JC1, a bacterial isolate from hawaiian bobtail squid eggs, produces indigoidine and differentially inhibits vibrios. Front. Microbiol. 7:1342. doi: 10.3389/fmicb.2016.01342
Guo, P., Cheng, Q., Xie, P., Fan, Y., Jiang, W., and Qin, Z. (2011). Characterization of the multiple CRISPR loci on Streptomyces linear plasmid pSHK1. Acta Biochim. Biophys. Sin. 43, 630–639. doi: 10.1093/abbs/gmr052
Gurevich, A., Saveliev, V., Vyahhi, N., and Tesler, G. (2013). QUAST: quality assessment tool for genome assemblies. Bioinformatics 29, 1072–1075. doi: 10.1093/bioinformatics/btt086
Hassan, S. S., Anjum, K., Abbas, S. Q., Akhter, N., Shagufta, B. I., Shah, S. A. A., et al. (2017). Emerging biopharmaceuticals from marine actinobacteria. Environ. Toxicol. Pharmacol. 49, 34–47. doi: 10.1016/j.etap.2016.11.015
Hider, R. C., and Kong, X. (2010). Chemistry and biology of siderophores. Nat. Prod. Rep. 27, 637–657. doi: 10.1039/b906679a
Hoff, G., Bertrand, C., Piotrowski, E., Thibessard, A., and Leblond, P. (2018). Genome plasticity is governed by double strand break DNA repair in Streptomyces. Sci. Rep. 8:5272. doi: 10.1038/s41598-018-23622-w
Hoffmann, T., and Bremer, E. (2016). “Management of osmotic stress by Bacillus Subtilis: genetics and physiology,” in Stress and Environmental Regulation of Gene Expression and Adaptation in Bacteria, ed. F. de Bruijn (Hoboken, NJ: John Wiley & Sons, Inc.), 657–676. doi: 10.1002/9781119004813.ch63
Hohmann, C., Schneider, K., Bruntner, C., Irran, E., Nicholson, G., Bull, A. T., et al. (2009). Caboxamycin, a new antibiotic of the benzoxazole family produced by the deep-sea strain Streptomyces sp. NTK 937. J. Antibiot. 62, 99–104. doi: 10.1038/ja.2008.24
Hoppers, A., Stoudenmire, J., Wu, S., and Lopanik, N. B. (2015). Antibiotic activity and microbial community of the temperate sponge, Haliclona sp. J. Appl. Microbiol. 118, 419–430. doi: 10.1111/jam.12709
Horn, H., Slaby, B. M., Jahn, M. T., Bayer, K., Moitinho-Silva, L., Förster, F., et al. (2016). An enrichment of CRISPR and other defense-related features in marine sponge-associated microbial metagenomes. Front. Microbiol. 7:1751. doi: 10.3389/fmicb.2016.01751
Hoz, J. F., Méndez, C., Salas, J. A., and Olano, C. (2017). Novel bioactive paulomycin derivatives produced by Streptomyces albus J1074. Molecules 22:E1758. doi: 10.3390/molecules22101758
Huillet, E., Velge, P., Vallaeys, T., and Pardon, P. (2006). LadR, a new PadR-related transcriptional regulator from Listeria monocytogenes, negatively regulates the expression of the multidrug effux pump MdrL. FEMS Microbiol. Lett. 254, 87–94. doi: 10.1111/j.1574-6968.2005.00014.x
Hwang, K.-S., Kim, H. U., Charusanti, P., Palsson, B. Ø, and Lee, S. Y. (2014). Systems biology and biotechnology of Streptomyces species for the production of secondary metabolites. Biotechnol. Adv. 32, 255–268. doi: 10.1016/j.biotechadv.2013.10.008
Ian, E., Malko, D. B., Sekurova, O. N., Bredholt, H., Rückert, C., Borisova, M. E., et al. (2014). Genomics of sponge-associated Streptomyces spp. Closely Related to Streptomyces albus J1074: insights into marine adaptation and secondary metabolite biosynthesis potential. PLoS One 9:e96719. doi: 10.1371/journal.pone.0096719
Indraningrat, A., Smidt, H., and Sipkema, D. (2016). Bioprospecting sponge-associated microbes for antimicrobial compounds. Mar. Drugs 14:E87. doi: 10.3390/md14050087
Iniyan, A. M., Mary, T. R. J., Joseph, F.-J. R. S., Kannan, R. R., and Vincent, S. G. P. (2016). Cell wall distracting anti-Methicillin-resistant Staphylococcus aureus compound PVI331 from a marine sponge associated Streptomyces. J. Appl. Biomed. 14, 273–283. doi: 10.1016/j.jab.2016.04.003
Jackson, S., Crossman, L., Almeida, E., Margassery, L., Kennedy, J., and Dobson, A. (2018). Diverse and abundant secondary metabolism biosynthetic gene clusters in the genomes of marine sponge derived Streptomyces spp. Isolates Mar. Drugs 16:E67. doi: 10.3390/md16020067
Jin, J., Yang, X., Liu, T., Xiao, H., Wang, G., Zhou, M., et al. (2018). Fluostatins M–Q featuring a 6-5-6-6 ring skeleton and high oxidized A-Rings from marine Streptomyces sp. PKU-MA00045. Mar. Drugs 16:E87. doi: 10.3390/md16030087
Johnson, M., Zaretskaya, I., Raytselis, Y., Merezhuk, Y., McGinnis, S., and Madden, T. L. (2008). NCBI BLAST: a better web interface. Nucleic Acids Res. 36, W5–W9. doi: 10.1093/nar/gkn201
Kallifidas, D., Jiang, G., Ding, Y., and Luesch, H. (2018). Rational engineering of Streptomyces albus J1074 for the overexpression of secondary metabolite gene clusters. Microb. Cell Fact. 17:25. doi: 10.1186/s12934-018-0874-2
Kamjam, M., Sivalingam, P., Deng, Z., and Hong, K. (2017). Deep sea actinomycetes and their secondary metabolites. Front. Microbiol. 8:760. doi: 10.3389/fmicb.2017.00760
Karimi, E., Slaby, B. M., Soares, A. R., Blom, J., Hentschel, U., and Costa, R. (2018). Metagenomic binning reveals versatile nutrient cycling and distinct adaptive features in alphaproteobacterial symbionts of marine sponges. FEMS Microbiol. Ecol. 94:fiy074. doi: 10.1093/femsec/fiy074
Katoh, K., and Standley, D. M. (2013). MAFFT multiple sequence alignment software version 7: improvements in performance and usability. Mol. Biol. Evol. 30, 772–780. doi: 10.1093/molbev/mst010
Kennedy, J., Baker, P., Piper, C., Cotter, P. D., Walsh, M., Mooij, M. J., et al. (2009). Isolation and analysis of bacteria with antimicrobial activities from the marine sponge Haliclona simulans collected from irish waters. Mar. Biotechnol. 11, 384–396. doi: 10.1007/s10126-008-9154-1
Kiran, G. S., Sekar, S., Ramasamy, P., Thinesh, T., Hassan, S., Lipton, A. N., et al. (2018). Marine sponge microbial association: towards disclosing unique symbiotic interactions. Mar. Environ. Res. 140, 169–179. doi: 10.1016/j.marenvres.2018.04.017
Koren, S., Walenz, B. P., Berlin, K., Miller, J. R., Bergman, N. H., and Phillippy, A. M. (2017). Canu: scalable and accurate long-read assembly via adaptive k-mer weighting and repeat separation. Genome Res. 27, 722–736. doi: 10.1101/gr.215087.116
Krämer, R. (2010). Bacterial stimulus perception and signal transduction: response to osmotic stress. Chem. Rec. 10, 217–229. doi: 10.1002/tcr.201000005
Kumar, S., Stecher, G., Li, M., Knyaz, C., and Tamura, K. (2018). MEGA X: molecular evolutionary genetics analysis across computing platforms. Mol. Biol. Evol. 35, 1547–1549. doi: 10.1093/molbev/msy096
Kurtz, S., Phillippy, A., Delcher, A. L., Smoot, M., Shumway, M., Antonescu, C., et al. (2004). Versatile and open software for comparing large genomes. Genome Biol. 5:R12. doi: 10.1186/gb-2004-5-2-r12
Kwan, J. C., Tianero, M. D. B., Donia, M. S., Wyche, T. P., Bugni, T. S., and Schmidt, E. W. (2014). Host control of symbiont natural product chemistry in cryptic populations of the tunicate Lissoclinum patella. PLoS One 9:e95850. doi: 10.1371/journal.pone.0095850
Lee, J. Y., Lee, J. Y., Jung, H. W., and Hwang, B. K. (2005). Streptomyces koyangensis sp. nov., a novel actinomycete that produces 4-phenyl-3-butenoic acid. Int. J. Syst. Evol. Microbiol. 55, 257–262. doi: 10.1099/ijs.0.63168-0
Lee, L.-H., Chan, K.-G., Stach, J., Wellington, E. M. H., and Goh, B.-H. (2018). Editorial: the search for biological active agent(s) from actinobacteria. Front. Microbiol. 9:824. doi: 10.3389/fmicb.2018.00824
Lee, S.-O., Kato, J., Takiguchi, N., Kuroda, A., Ikeda, T., Mitsutani, A., et al. (2000). Involvement of an extracellular protease in algicidal activity of the marine bacterium Pseudoalteromonas sp. Strain A28. Appl. Environ. Microbiol. 66, 4334–4339. doi: 10.1128/AEM.66.10.4334-4339.2000
Li, H.-J., Tang, B.-L., Shao, X., Liu, B.-X., Zheng, X.-Y., Han, X.-X., et al. (2016). Characterization of a new S8 serine protease from marine sedimentary Photobacterium sp. A5–7 and the function of its protease-associated domain. Front. Microbiol. 7:2016. doi: 10.3389/fmicb.2016.02016
Li, Q., Yi, L., Marek, P., and Iverson, B. L. (2013). Commercial proteases: present and future. FEBS Lett. 587, 1155–1163. doi: 10.1016/j.febslet.2012.12.019
Li, S., Zhang, B., Zhu, H., and Zhu, T. (2018). Cloning and expression of the chitinase encoded by ChiKJ406136 from Streptomyces Sampsonii (Millard & Burr) Waksman KJ40 and its antifungal effect. Forests 9:699. doi: 10.3390/f9110699
Li, Z.-Y., Wang, Y.-Z., He, L.-M., and Zheng, H.-J. (2015). Metabolic profiles of prokaryotic and eukaryotic communities in deep-sea sponge Neamphius huxleyi indicated by metagenomics. Sci. Rep. 4:3895. doi: 10.1038/srep03895
Lim, B., and Lee, K. (2015). Stability of the osmoregulated promoter-derived proP mRNA is posttranscriptionally regulated by RNase III in Escherichia coli. J. Bacteriol. 197, 1297–1305. doi: 10.1128/JB.02460-14
Lin, Y., Hahn, M.-Y., Roe, J.-H., Huang, T.-W., Tsai, H.-H., Lin, Y.-F., et al. (2009). Streptomyces telomeres contain a promoter. J. Bacteriol. 191, 773–781. doi: 10.1128/JB.01299-08
Linares, J. F., Gustafsson, I., Baquero, F., and Martinez, J. L. (2006). Antibiotics as intermicrobial signaling agents instead of weapons. Proc. Natl. Acad. Sci. U.S.A. 103, 19484–19489. doi: 10.1073/pnas.0608949103
Liu, C., Jiang, Y., Lei, H., Chen, X., Ma, Q., Han, L., et al. (2017). Four new nanaomycins produced by Streptomyces hebeiensis derived from lichen. Chem. Biodivers. 14:e1700057. doi: 10.1002/cbdv.201700057
Liu, N., Song, F., Shang, F., and Huang, Y. (2015). Mycemycins A–E, new dibenzoxazepinones isolated from two different Streptomycetes. Mar. Drugs 13, 6247–6258. doi: 10.3390/md13106247
Liu, Q., Xiao, L., Zhou, Y., Deng, K., Tan, G., Han, Y., et al. (2016). Development of Streptomyces sp. FR-008 as an emerging chassis. Synth. Syst. Biotechnol. 1, 207–214. doi: 10.1016/J.SYNBIO.2016.07.002
Losada, A. A., Cano-Prieto, C., García-Salcedo, R., Braña, A. F., Méndez, C., Salas, J. A., et al. (2017). Caboxamycin biosynthesis pathway and identification of novel benzoxazoles produced by cross-talk in Streptomyces sp. NTK 937. Microb. Biotechnol. 10, 873–885. doi: 10.1111/1751-7915.12716
Luo, Y., Huang, H., Liang, J., Wang, M., Lu, L., Shao, Z., et al. (2013). Activation and characterization of a cryptic polycyclic tetramate macrolactam biosynthetic gene cluster. Nat. Commun. 4:2894. doi: 10.1038/ncomms3894
MacMillan, S. V., Alexander, D. A., Culham, D. E., Kunte, H. J., Marshall, E. V., Rochon, D., et al. (1999). The ion coupling and organic substrate specificities of osmoregulatory transporter ProP in Escherichia coli. Biochim. Biophys. Acta Biomembr. 1420, 30–44. doi: 10.1016/S0005-2736(99)00085-1
Maddocks, S. E., and Oyston, P. C. F. (2008). Structure and function of the LysR-type transcriptional regulator (LTTR) family proteins. Microbiology 154, 3609–3623. doi: 10.1099/mic.0.2008/022772-0
Magarvey, N. A., Haltli, B., He, M., Greenstein, M., and Hucul, J. A. (2006). Biosynthetic pathway for mannopeptimycins, lipoglycopeptide antibiotics active against drug-resistant gram-positive pathogens. Antimicrob. Agents Chemother. 50, 2167–2177. doi: 10.1128/aac.01545-05
Majiduddin, F. K., Materon, I. C., and Palzkill, T. G. (2002). Molecular analysis of beta-lactamase structure and function. Int. J. Med. Microbiol. 292, 127–137. doi: 10.1078/1438-4221-00198
Manivasagan, P., Kang, K.-H., Sivakumar, K., Li-Chan, E. C. Y., Oh, H.-M., and Kim, S.-K. (2014). Marine actinobacteria: an important source of bioactive natural products. Environ. Toxicol. Pharmacol. 38, 172–188. doi: 10.1016/j.etap.2014.05.014
Marchler-Bauer, A., Derbyshire, M. K., Gonzales, N. R., Lu, S., Chitsaz, F., Geer, L. Y., et al. (2015). CDD: NCBI’s conserved domain database. Nucleic Acids Res. 43, D222–D226. doi: 10.1093/nar/gku1221
Marchler-Bauer, A., Lu, S., Anderson, J. B., Chitsaz, F., Derbyshire, M. K., DeWeese-Scott, C., et al. (2011). CDD: a conserved domain database for the functional annotation of proteins. Nucleic Acids Res. 39, D225–D229. doi: 10.1093/nar/gkq1189
Mascher, T., Zimmer, S. L., Smith, T.-A., and Helmann, J. D. (2004). Antibiotic-inducible promoter regulated by the cell envelope stress-sensing two-component system LiaRS of Bacillus subtilis. Antimicrob. Agents Chemother. 48, 2888–2896. doi: 10.1128/AAC.48.8.2888-2896.2004
Medema, M. H., Kottmann, R., Yilmaz, P., Cummings, M., Biggins, J. B., Blin, K., et al. (2015). The minimum information about a biosynthetic gene cluster (MIBiG) specification. Nat. Chem. Biol. 11, 625–631. doi: 10.1038/nchembio.1890
Mehbub, M. F., Tanner, J. E., Barnett, S. J., Franco, C. M. M., and Zhang, W. (2016). The role of sponge-bacteria interactions: the sponge Aplysilla rosea challenged by its associated bacterium Streptomyces ACT-52A in a controlled aquarium system. Appl. Microbiol. Biotechnol. 100, 10609–10626. doi: 10.1007/s00253-016-7878-9
Miller, K. J., and Wood, J. M. (1996). Osmoadaptation by rhizosphere bacteria. Annu. Rev. Microbiol. 50, 101–136. doi: 10.1146/annurev.micro.50.1.101
Mitchell, A. L., Attwood, T. K., Babbitt, P. C., Blum, M., Bork, P., Bridge, A., et al. (2018). InterPro in 2019: improving coverage, classification and access to protein sequence annotations. Nucleic Acids Res. 47, D351–D360. doi: 10.1093/nar/gky1100
Molina-Henares, A. J., Krell, T., Eugenia Guazzaroni, M., Segura, A., and Ramos, J. L. (2006). Members of the IclR family of bacterial transcriptional regulators function as activators and/or repressors. FEMS Microbiol. Rev. 30, 157–186. doi: 10.1111/j.1574-6976.2005.00008.x
Montalbán-López, M., Deng, J., van Heel, A. J., and Kuipers, O. P. (2018). Specificity and application of the lantibiotic protease NisP. Front. Microbiol. 9:160. doi: 10.3389/fmicb.2018.00160
Motohashi, K., Takagi, M., Yamamura, H., Hayakawa, M., and Shin-ya, K. (2010). A new angucycline and a new butenolide isolated from lichen-derived Streptomyces spp. J. Antibiot. 63, 545–548. doi: 10.1038/ja.2010.94
Naas, T., Oueslati, S., Bonnin, R. A., Dabos, M. L., Zavala, A., Dortet, L., et al. (2017). Beta-lactamase database (BLDB) – structure and function. J. Enzyme Inhib. Med. Chem. 32, 917–919. doi: 10.1080/14756366.2017.1344235
Nara, A., Hashimoto, T., Komatsu, M., Nishiyama, M., Kuzuyama, T., and Ikeda, H. (2017). Characterization of bafilomycin biosynthesis in Kitasatospora setae KM-6054 and comparative analysis of gene clusters in Actinomycetales microorganisms. J. Antibiot. 70, 616–624. doi: 10.1038/ja.2017.33
Navarro-Muñoz, J. C., Selem-Mojica, N., Mullowney, M. W., Kautsar, S., Tryon, J. H., Parkinson, E. I., et al. (2018). A computational framework for systematic exploration of biosynthetic diversity from large-scale genomic data. BioRxiv 445270. doi: 10.1101/445270
Nguyen, T. B., Kitani, S., Shimma, S., and Nihira, T. (2018). Butenolides from Streptomyces albus J1074 act as external signals to stimulate avermectin production in Streptomyces avermitilis. Appl. Environ. Microbiol. 84:e02791-17. doi: 10.1128/AEM.02791-17
Ninomiya, A., Katsuyama, Y., Kuranaga, T., Miyazaki, M., Nogi, Y., Okada, S., et al. (2016). Biosynthetic gene cluster for surugamide A encompasses an unrelated decapeptide, surugamide F. ChemBioChem 17, 1709–1712. doi: 10.1002/cbic.201600350
Nishida, H. (2012). Evolution of genome base composition and genome size in bacteria. Front. Microbiol. 3:420. doi: 10.3389/fmicb.2012.00420
Page, A. J., Cummins, C. A., Hunt, M., Wong, V. K., Reuter, S., Holden, M. T. G., et al. (2015). Roary: rapid large-scale prokaryote pan genome analysis. Bioinformatics 31, 3691–3693. doi: 10.1093/bioinformatics/btv421
Paulus, C., Rebets, Y., Tokovenko, B., Nadmid, S., Terekhova, L. P., Myronovskyi, M., et al. (2017). New natural products identified by combined genomics-metabolomics profiling of marine Streptomyces sp. MP131-18. Sci. Rep. 7:42382. doi: 10.1038/srep42382
Penn, K., and Jensen, P. R. (2012). Comparative genomics reveals evidence of marine adaptation in Salinispora species. BMC Genomics 13:86. doi: 10.1186/1471-2164-13-86
Qurashi, A. W., and Sabri, A. N. (2011). Osmoadaptation and plant growth promotion by salt tolerant bacteria under salt stress. African J. Microbiol. Res. 5, 3546–3554. doi: 10.5897/AJMR11.736
R Core Team (2018). R: A Language and Environment for Statistical Computing. Vienna: R Foundation for Statistical Computing.
Racine, J. S. (2012). RSTUDIO: a platform-independent IDE for R and sweave. J. Appl. Econ. 27, 167–172. doi: 10.1002/jae.1278
Rademacher, C., and Masepohl, B. (2012). Copper-responsive gene regulation in bacteria. Microbiology 158, 2451–2464. doi: 10.1099/mic.0.058487-0
Rashad, F. M., Fathy, H. M., El-Zayat, A. S., and Elghonaimy, A. M. (2015). Isolation and characterization of multifunctional Streptomyces species with antimicrobial, nematicidal and phytohormone activities from marine environments in Egypt. Microbiol. Res. 175, 34–47. doi: 10.1016/J.MICRES.2015.03.002
Rasko, D. A., Rosovitz, M. J., Økstad, O. A., Fouts, D. E., Jiang, L., Cer, R. Z., et al. (2007). Complete sequence analysis of novel plasmids from emetic and periodontal Bacillus cereus isolates reveals a common evolutionary history among the B. cereus-group plasmids, including Bacillus anthracis pXO1. J. Bacteriol. 189, 52–64. doi: 10.1128/JB.01313-06
Reimer, A., Blohm, A., Quack, T., Grevelding, C. G., Kozjak-Pavlovic, V., Rudel, T., et al. (2015). Inhibitory activities of the marine streptomycete-derived compound SF2446A2 against Chlamydia trachomatis and Schistosoma mansoni. J. Antibiot. 68, 674–679. doi: 10.1038/ja.2015.54
Reynolds, D., and Thomas, T. (2016). Evolution and function of eukaryotic-like proteins from sponge symbionts. Mol. Ecol. 25, 5242–5253. doi: 10.1111/mec.13812
Rigali, S., Anderssen, S., Naômé, A., and van Wezel, G. P. (2018). Cracking the regulatory code of biosynthetic gene clusters as a strategy for natural product discovery. Biochem. Pharmacol. 153, 24–34. doi: 10.1016/j.bcp.2018.01.007
Robbel, L., and Marahiel, M. A. (2010). Daptomycin, a bacterial lipopeptide synthesized by a nonribosomal machinery. J. Biol. Chem. 285, 27501–27508. doi: 10.1074/jbc.R110.128181
Rodríguez, H., Rico, S., Díaz, M., and Santamaría, R. I. (2013). Two-component systems in Streptomyces: key regulators of antibiotic complex pathways. Microb. Cell Fact. 12:127. doi: 10.1186/1475-2859-12-127
Roessler, M., and Muller, V. (2001). Osmoadaptation in bacteria and archaea: common principles and differences. Environ. Microbiol. 3, 743–754. doi: 10.1046/j.1462-2920.2001.00252.x
Rolain, J.-M., Abat, C., Jimeno, M.-T., Fournier, P.-E., and Raoult, D. (2016). Do we need new antibiotics? Clin. Microbiol. Infect. 22, 408–415. doi: 10.1016/j.cmi.2016.03.012
Romero, D., Traxler, M. F., Lòpez, D., and Kolter, R. (2011). Antibiotics as signal molecules. Chem. Rev. 111, 5492–5505. doi: 10.1021/cr2000509
Rong, X., Doroghazi, J. R., Cheng, K., Zhang, L., Buckley, D. H., and Huang, Y. (2013). Classification of Streptomyces phylogroup pratensis (Doroghazi and Buckley, 2010) based on genetic and phenotypic evidence, and proposal of Streptomyces pratensis sp. nov. Syst. Appl. Microbiol. 36, 401–407. doi: 10.1016/j.syapm.2013.03.010
Ronquist, F., Teslenko, M., van der Mark, P., Ayres, D. L., Darling, A., Höhna, S., et al. (2012). MrBayes 3.2: efficient bayesian phylogenetic inference and model choice across a large model space. Syst. Biol. 61, 539–542. doi: 10.1093/sysbio/sys029
RStudio Team (2015). RStudio: Integrated Development Environment for R. Available at: http://www.rstudio.com/ (accessed January 15, 2019).
Rutherford, K., Parkhill, J., Crook, J., Horsnell, T., Rice, P., Rajandream, M.-A., et al. (2000). Artemis: sequence visualization and annotation. Bioinformatics 16, 944–945. doi: 10.1093/bioinformatics/16.10.944
Rutledge, P. J., and Challis, G. L. (2015). Discovery of microbial natural products by activation of silent biosynthetic gene clusters. Nat. Rev. Microbiol. 13, 509–523. doi: 10.1038/nrmicro3496
Salwan, R., and Sharma, V. (2018). “The role of actinobacteria in the production of industrial enzymes,” in New and Future Developments in Microbial Biotechnology and Bioengineering, eds B. P. Singh, V. K. Gupta, and A. K. Passari (Cambridge, MA: Elsevier), 165–177. doi: 10.1016/B978-0-444-63994-3.00011-4
Schmid, M., Muri, J., Melidis, D., Varadarajan, A. R., Somerville, V., Wicki, A., et al. (2018). Comparative genomics of completely sequenced Lactobacillus helveticus genomes provides insights into strain-specific genes and resolves metagenomics data down to the strain level. Front. Microbiol. 9:63. doi: 10.3389/fmicb.2018.00063
Schneemann, I., Kajahn, I., Ohlendorf, B., Zinecker, H., Erhard, A., Nagel, K., et al. (2010). Mayamycin, a cytotoxic polyketide from a Streptomyces strain isolated from the marine sponge Halichondria panicea. J. Nat. Prod. 73, 1309–1312. doi: 10.1021/np100135b
Seemann, T. (2014). Prokka: rapid prokaryotic genome annotation. Bioinformatics 30, 2068–2069. doi: 10.1093/bioinformatics/btu153
Seipke, R. F., Barke, J., Brearley, C., Hill, L., Yu, D. W., Goss, R. J. M., et al. (2011). A single Streptomyces symbiont makes multiple antifungals to support the fungus farming ant Acromyrmex octospinosus. PLoS One 6:e22028. doi: 10.1371/journal.pone.0022028
Seipke, R. F., Kaltenpoth, M., and Hutchings, M. I. (2012). Streptomyces as symbionts: an emerging and widespread theme? FEMS Microbiol. Rev. 36, 862–876. doi: 10.1111/j.1574-6976.2011.00313.x
Ser, H.-L., Tan, L. T.-H., Law, J. W.-F., Chan, K.-G., Duangjai, A., Saokaew, S., et al. (2017). Focused review: cytotoxic and antioxidant potentials of mangrove-derived Streptomyces. Front. Microbiol. 8:2065. doi: 10.3389/fmicb.2017.02065
Shannon, P., Markiel, A., Ozier, O., Baliga, N. S., Wang, J. T., Ramage, D., et al. (2003). Cytoscape: a software environment for integrated models of biomolecular interaction networks. Genome Res. 13, 2498–2504. doi: 10.1101/gr.1239303
Shen, B. (2003). Polyketide biosynthesis beyond the type I, II and III polyketide synthase paradigms. Curr. Opin. Chem. Biol. 7, 285–295. doi: 10.1016/S1367-5931(03)00020-6
Shi, Y., Yang, X., Garg, N., and van der Donk, W. A. (2011). Production of lantipeptides in Escherichia coli. J. Am. Chem. Soc. 133, 2338–2341. doi: 10.1021/ja109044r
Shin, S. C., Ahn, D. H., Kim, S. J., Lee, H., Oh, T.-J., Lee, J. E., et al. (2013). Advantages of single-molecule real-time sequencing in high-GC content genomes. PLoS One 8:e68824. doi: 10.1371/journal.pone.0068824
Singh, M. P., Petersen, P. J., Weiss, W. J., Janso, J. E., Luckman, S. W., Lenoy, E. B., et al. (2003). Mannopeptimycins, new cyclic glycopeptide antibiotics produced by Streptomyces hygroscopicus LL-AC98: antibacterial and mechanistic activities. Antimicrob. Agents Chemother. 47, 62–69. doi: 10.1128/AAC.47.1.62-69.2003
Slaby, B. M., Hackl, T., Horn, H., Bayer, K., and Hentschel, U. (2017). Metagenomic binning of a marine sponge microbiome reveals unity in defense but metabolic specialization. ISME J. 11, 2465–2478. doi: 10.1038/ismej.2017.101
Song, F., Liu, N., Liu, M., Chen, Y., and Huang, Y. (2018). Identification and characterization of mycemycin biosynthetic gene clusters in Streptomyces olivaceus FXJ8.012 and Streptomyces sp. FXJ1.235. Mar. Drugs 16, 98. doi: 10.3390/md16030098
Stock, J. B., Stock, A. M., and Mottonen, J. M. (1990). Signal transduction in bacteria. Nature 344, 395–400. doi: 10.1038/344395a0
Sun, W., Liu, C., Zhang, F., Zhao, M., and Li, Z. (2018). Comparative genomics provides insights into the marine adaptation in sponge-derived Kocuria flava S43. Front. Microbiol. 9:1257. doi: 10.3389/fmicb.2018.01257
Tatusova, T., DiCuccio, M., Badretdin, A., Chetvernin, V., Nawrocki, E. P., Zaslavsky, L., et al. (2016). NCBI prokaryotic genome annotation pipeline. Nucleic Acids Res. 44, 6614–6624. doi: 10.1093/nar/gkw569
Teichmann, L., Kümmel, H., Warmbold, B., and Bremer, E. (2018). OpuF, a new Bacillus compatible solute ABC transporter with a substrate-binding protein fused to the transmembrane domain. Appl. Environ. Microbiol. 84:e01728-18. doi: 10.1128/AEM.01728-18
ter Beek, J., Guskov, A., and Slotboom, D. J. (2014). Structural diversity of ABC transporters. J. Gen. Physiol. 143, 419–435. doi: 10.1085/jgp.201411164
Tetzlaff, C. N., You, Z., Cane, D. E., Takamatsu, S., Omura, S., and Ikeda, H. (2006). A gene cluster for biosynthesis of the sesquiterpenoid antibiotic pentalenolactone in Streptomyces avermitilis. Biochemistry 45, 6179–6186. doi: 10.1021/bi060419n
Thabit, A. K., Crandon, J. L., and Nicolau, D. P. (2015). Antimicrobial resistance: impact on clinical and economic outcomes and the need for new antimicrobials. Expert Opin. Pharmacother. 16, 159–177. doi: 10.1517/14656566.2015.993381
Thomas, T., Rusch, D., DeMaere, M. Z., Yung, P. Y., Lewis, M., Halpern, A., et al. (2010). Functional genomic signatures of sponge bacteria reveal unique and shared features of symbiosis. ISME J. 4, 1557–1567. doi: 10.1038/ismej.2010.74
Tian, R.-M., Wang, Y., Bougouffa, S., Gao, Z.-M., Cai, L., Zhang, W.-P., et al. (2014). Effect of copper treatment on the composition and function of the bacterial community in the sponge Haliclona cymaeformis. mBio 5:e01980. doi: 10.1128/mBio.01980-14
Tian, X., Zhang, Z., Yang, T., Chen, M., Li, J., Chen, F., et al. (2016). Comparative genomics analysis of Streptomyces species reveals their adaptation to the marine environment and their diversity at the genomic level. Front. Microbiol. 7:998. doi: 10.3389/fmicb.2016.00998
Tierrafría, V. H., Ramos-Aboites, H. E., Gosset, G., and Barona-Gómez, F. (2011). Disruption of the siderophore-binding desE receptor gene in Streptomyces coelicolor A3(2) results in impaired growth in spite of multiple iron-siderophore transport systems. Microb. Biotechnol. 4, 275–285. doi: 10.1111/j.1751-7915.2010.00240.x
UniProt Consortium (2015). UniProt: a hub for protein information. Nucleic Acids Res. 43, D204–D212. doi: 10.1093/nar/gku989
van der Meij, A., Worsley, S. F., Hutchings, M. I., and van Wezel, G. P. (2017). Chemical ecology of antibiotic production by actinomycetes. FEMS Microbiol. Rev. 41, 392–416. doi: 10.1093/femsre/fux005
Van Melderen, L. (2010). Toxin–antitoxin systems: why so many, what for? Curr. Opin. Microbiol. 13, 781–785. doi: 10.1016/j.mib.2010.10.006
Vicente, C., Thibessard, A., Lorenzi, J.-N., Benhadj, M., Hôtel, L., Gacemi-Kirane, D., et al. (2018). Comparative genomics among closely related Streptomyces strains revealed specialized metabolite biosynthetic gene cluster diversity. Antibiotics 7:E86. doi: 10.3390/antibiotics7040086
Volff, J. N., Viell, P., and Altenbuchner, J. (1997). Artificial circularization of the chromosome with concomitant deletion of its terminal inverted repeats enhances genetic instability and genome rearrangement in Streptomyces lividans. Mol. Gen. Genet. 253, 753–760. doi: 10.1007/s004380050380
Waddell, P. J., and Steel, M. (1997). General time-reversible distances with unequal rates across sites: mixing Γ and inverse gaussian distributions with invariant sites. Mol. Phylogenet. Evol. 8, 398–414. doi: 10.1006/mpev.1997.0452
Wang, T., Chen, Z., Cheng, Q., Zhou, M., Tian, X., Xie, P., et al. (2012). Characterization of replication and conjugation of plasmid pWTY27 from a widely distributed Streptomyces species. BMC Microbiol. 12:253. doi: 10.1186/1471-2180-12-253
Weaver, D., Karoonuthaisiri, N., Tsai, H.-H., Huang, C.-H., Ho, M.-L., Gai, S., et al. (2004). Genome plasticity in Streptomyces: identification of 1 Mb TIRs in the S. coelicolor A3(2) chromosome. Mol. Microbiol. 51, 1535–1550. doi: 10.1111/j.1365-2958.2003.03920.x
Wickham, H. (2011). The split-apply-combine strategy for data analysis. J. Stat. Softw. 40, 1–29. doi: 10.18637/jss.v040.i01
Wilkens, S. (2015). Structure and mechanism of ABC transporters. F1000Prime Rep. 7:14. doi: 10.12703/P7-14
Wilson, K. (2001). Preparation of genomic DNA from bacteria. Curr. Protoc. Mol. Biol. 56, 2.4.1–2.4.5. doi: 10.1002/0471142727.mb0204s56
Wood, J. M. (2007). Bacterial osmosensing transporters. Methods Enzymol. 428, 77–107. doi: 10.1016/S0076-6879(07)28005-X
Wood, J. M. (2015). Bacterial responses to osmotic challenges. J. Gen. Physiol. 145, 381–388. doi: 10.1085/jgp.201411296
Wright, G. D. (2005). Bacterial resistance to antibiotics: enzymatic degradation and modification. Adv. Drug Deliv. Rev. 57, 1451–1470. doi: 10.1016/j.addr.2005.04.002
Wright, G. D. (2012). “The origins of antibiotic resistance,” in Antibiotic Resistance. Handbook of Experimental Pharmacology, Vol. 211, ed. A. Coates (Berlin: Springer).
Wu, H., Qu, S., Lu, C., Zheng, H., Zhou, X., Bai, L., et al. (2012). Genomic and transcriptomic insights into the thermo-regulated biosynthesis of validamycin in Streptomyces hygroscopicus 5008. BMC Genomics 13:337. doi: 10.1186/1471-2164-13-337
Wyche, T. P., Ruzzini, A. C., Schwab, L., Currie, C. R., and Clardy, J. (2017). Tryptorubin A: a polycyclic peptide from a fungus-derived Streptomycete. J. Am. Chem. Soc. 139, 12899–12902. doi: 10.1021/jacs.7b06176
Xie, C.-L., Xia, J.-M., Wang, J.-S., Lin, D.-H., and Yang, X.-W. (2018). Metabolomic investigations on Nesterenkonia flava revealed significant differences between marine and terrestrial actinomycetes. Mar. Drugs 16:E356. doi: 10.3390/md16100356
Xu, X.-N., Chen, L.-Y., Chen, C., Tang, Y.-J., Bai, F.-W., Su, C., et al. (2018). Genome mining of the marine actinomycete Streptomyces sp. DUT11 and discovery of tunicamycins as anti-complement agents. Front. Microbiol. 9:1318. doi: 10.3389/fmicb.2018.01318
Xu, Y., Guo, J., Wang, L., Jiang, R., Jin, X., Liu, J., et al. (2016). The crystal structure of the YknZ extracellular domain of ABC transporter YknWXYZ from Bacillus amyloliquefaciens. PLoS One 11:e0155846. doi: 10.1371/journal.pone.0155846
Yaakop, A. S., Chan, K.-G., Ee, R., Lim, Y. L., Lee, S.-K., Manan, F. A., et al. (2016). Characterization of the mechanism of prolonged adaptation to osmotic stress of Jeotgalibacillus malaysiensis via genome and transcriptome sequencing analyses. Sci. Rep. 6:33660. doi: 10.1038/srep33660
Yagüe, P., Lopez-Garcia, M. T., Rioseras, B., Sanchez, J., and Manteca, A. (2012). New insights on the development of Streptomyces and their relationships with secondary metabolite production. Curr. Trends Microbiol. 8, 65–73.
Yamada, Y., Kuzuyama, T., Komatsu, M., Shin-ya, K., Omura, S., Cane, D. E., et al. (2015). Terpene synthases are widely distributed in bacteria. Proc. Natl. Acad. Sci. U.S.A. 112, 857–862. doi: 10.1073/pnas.1422108112
Yuwono, T. (2005). Metabolism of betaine as a carbon source by an osmotolerant bacterium isolated from the weed rhizosphere. World J. Microbiol. Biotechnol. 21, 69–73. doi: 10.1007/s11274-004-1935-8
Zhang, C., Yang, Z., Qin, X., Ma, J., Sun, C., Huang, H., et al. (2018). Genome mining for mycemycin: discovery and elucidation of related methylation and chlorination biosynthetic chemistries. Org. Lett. 20, 7633–7636. doi: 10.1021/acs.orglett.8b03373
Zhang, W., Fortman, J. L., Carlson, J. C., Yan, J., Liu, Y., Bai, F., et al. (2013). Characterization of the bafilomycin biosynthetic gene cluster from Streptomyces lohii. ChemBioChem 14, 301–306. doi: 10.1002/cbic.201200743
Zhang, X.-Y., Han, X.-X., Chen, X.-L., Dang, H.-Y., Xie, B.-B., Qin, Q.-L., et al. (2015). Diversity of cultivable protease-producing bacteria in sediments of Jiaozhou Bay, China. Front. Microbiol. 6:1021. doi: 10.3389/fmicb.2015.01021
Zhang, Z., Pan, H.-X., and Tang, G.-L. (2017). New insights into bacterial type II polyketide biosynthesis. F1000Res. 6:172. doi: 10.12688/f1000research.10466.1
Zhou, Z., Gu, J., Li, Y.-Q., and Wang, Y. (2012). Genome plasticity and systems evolution in Streptomyces. BMC Bioinform. 13:S8. doi: 10.1186/1471-2105-13-S10-S8
Keywords: comparative genomics, Streptomyces, environmental adaptation, marine sponge bacteria, secondary metabolite biosynthetic gene clusters, single molecule real-time sequencing
Citation: Almeida EL, Carrillo Rincón AF, Jackson SA and Dobson ADW (2019) Comparative Genomics of Marine Sponge-Derived Streptomyces spp. Isolates SM17 and SM18 With Their Closest Terrestrial Relatives Provides Novel Insights Into Environmental Niche Adaptations and Secondary Metabolite Biosynthesis Potential. Front. Microbiol. 10:1713. doi: 10.3389/fmicb.2019.01713
Received: 24 April 2019; Accepted: 11 July 2019;
Published: 26 July 2019.
Edited by:
Levente Bodrossy, CSIRO Oceans and Atmosphere, AustraliaReviewed by:
Usama Ramadan Abdelmohsen, Minia University, EgyptPaul Jensen, Scripps Institution of Oceanography, United States
Pablo Cruz-Morales, Lawrence Berkeley National Laboratory, United States
Copyright © 2019 Almeida, Carrillo Rincón, Jackson and Dobson. This is an open-access article distributed under the terms of the Creative Commons Attribution License (CC BY). The use, distribution or reproduction in other forums is permitted, provided the original author(s) and the copyright owner(s) are credited and that the original publication in this journal is cited, in accordance with accepted academic practice. No use, distribution or reproduction is permitted which does not comply with these terms.
*Correspondence: Alan D. W. Dobson, YS5kb2Jzb25AdWNjLmll