- 1School of Agriculture and Biology, Shanghai Jiao Tong University, Shanghai, China
- 2State Key Laboratory of Microbial Metabolism, Shanghai Jiao Tong University, Shanghai, China
In an effort to balance the demands of plant growth promoting and biological control agents in a single product, the technology on the co-cultivation of two microbes, Trichoderma asperellum GDFS1009 and Bacillus amyloliquefaciens 1841 has been developed and demonstrated its effectiveness in synergistic interactions and its impact on the plant growth and biocontrol potential. In this study, optimization of T. asperellum and B. amyloliquefaciens growth in a single medium was carried out using response surface methodology (RSM). The optimal medium for enhanced growth was estimated as 2% yeast extract, 2% molasses and 2% corn gluten meal. T. asperellum evolved the complicated molecular mechanisms in the co-culture by the induction of BLR-1/BLR-2, VELVET, and NADPH oxidases genes. In performance with these genes, conserved signaling pathways, such as heterotrimeric G proteins and mitogen-activated protein kinases (MAPKs) had also involved in this molecular orchestration. The co-cultivation induced the expression of T. asperellum genes related to secondary metabolism, mycoparasitism, antioxidants and plant growth. On the other hand, the competition during co-cultivation induced the production of new compounds that are not detected in axenic cultures. In addition, the co-culture significantly enhanced the plant growth and protection against Fusarium graminearum. The present study demonstrated the potential of co-cultivation technology could be a used to grow the T. asperellum GDFS1009 and B. amyloliquefaciens 1841 synergistically to improve the production of mycoparasitism related enzymes, secondary metabolites, and plant growth promoting compounds to significantly enhance the plant growth and protection against plant pathogens.
Introduction
The genus Trichoderma is recognized for its biocontrol function against the fungal phytopathogens (Contreras-Cornejo et al., 2016) such as Rhizoctonia solani, Botrytis cinerea, Sclerotium sclerotiorum, Pythium spp., and Fusarium spp. Trichoderma mycoparasitism involves the detection, attachment and coiling in the region of the host hyphae, and release the antibiotic metabolites and cell-wall degrading enzymes (Zeilinger et al., 2016). The Trichoderma species produce several compounds, such as isonitrile, diketopiperazines, sesquiterpenes, polyketides, alkylpyrones, and peptaibols (Patil et al., 2016). However, Trichoderma asperellum, a less studied fungus, is also a successful biological control agent against a wide range of plant pathogens (Marcello et al., 2010; Wu et al., 2017). T. asperellum has been identified to antagonize the pathogen Fusarium oxysporum, Corynespora cassiicola, and Curvularia aeria. Further, its efficacy has also been demonstrated (Baiyee et al., 2019; Veenstra et al., 2019). B. amyloliquefaciens is a root-colonizing biocontrol bacterium and is employed to battle several phyto-pathogens. It has been shown advantages to plants through the soil and hydroponic applications (Chowdhury et al., 2015). It inhibits the growth of pathogens, and delay the infection, though reasonable exclusion and competition with the pathogen (Huang et al., 2014; Tan et al., 2016). Bacillus amyloliquefaciens have also been found to antagonize B. cinerea and Fusarium solani (Freitas et al., 2019). Since the T. asperellum and B. amyloliquefaciens have the various benefits to the plant growth and development, in the present investigation, we aimed to develop the consortium of T. asperellum and B. amyloliquefaciens through the co-cultivation technology.
Despite, the biocontrol and plant growth promoting mechanism of B. amyloliquefaciens and T. asperellum are different; the co-cultivation technology could enable both organisms to improve the biocontrol and plant growth. Microbial co-cultivation has been effectively used to induce the expression of several cryptic pathways. Co-cultivation increase the production of new secondary metabolites through the activation of signaling molecules (Wakefield et al., 2017). It also leads to the synthesis of enzymes to increase the metabolite production (Abdelmohsen et al., 2015). Wu et al. (2018) found that the antibiosis of B. amyloliquefaciens ACCC11060 and T. asperellum GDFS1009 was increased under co-cultivation conditions. Hence, in the present study, the optimal medium for B. amyloliquefaciens 1841 and T. asperellum GDFS1009 cultivation was developed using response surface methodology. Further, the differential expression of sporulations, enzymes and secondary metabolites related genes were analyzed and tested its efficacy on the plant growth and biocontrol activity.
Materials and Methods
Strains
The biocontrol fungus T. asperellum GDFS1009 was obtained from the China General Microbiological Culture Collection Center (CGMCC NO. 9512) Beijing, China. The biocontrol bacterium B. amyloliquefaciens 1841 was obtained from the Laboratory of Microbial Fermentation, Sichuan University, China. Fusarium graminearum (wheat head blight and root rot pathogen) F. graminearum (maize stack pathogen), B. cinerea and F. oxysporum were used as a target pathogen for the examination of mycoparasitism activity.
Optimization of Co-culture Medium
1 ml of T. asperellum GDFS1009 inoculums (106 spores/ml) was pre-cultured at different time intervals (0, 4, and 48 h) in 100 mL of TY (Benoit et al., 2015), NB (Peptone 1%; Beef extract 1%; Sodium chloride 0.5%) and YMC broth (Yeast extract 2%; Molasses 1% and Corn Gluten meal 1%), subsequently 100 μl of the B. amyloliquefaciens 1841 inoculums (1.0 OD at 600 nm) was inoculated into the preculture medium and incubated at 180 rpm at 28°C for 2 days. 1 ml of T. asperellum GDFS1009 and 100 μL of B. amyloliquefaciens 1841 were singly inoculated into 100 ml of TY, NB and YMC broth, incubated in shaker for 4 days. After incubation, the broth cultures were serially diluted and plated in the PDA containing streptomycin and chloramphenicol to estimate the growth of fungus. Whereas, the bacterial growth was estimated using the nutrient agar containing nystatin and cycloheximide.
Experimental Design and Data Analysis
The ingredients of YMC medium on the growth of T. asperellum GDFS1009 and B. amyloliquefaciens 1841 were enhanced by central composite design (CCD) at five distinct levels. The impact of the yeast extract (X1), molasses (X2) and corn gluten meal (X3) on the growth of T. asperellum GDFS1009 and B. amyloliquefaciens 1841 (Y) were accessed. The growth of T. asperellum GDFS1009 and B. amyloliquefaciens 1841 were taken as the average of the duplicate. The experimental designs with the observed and predicted values for growth of T. asperellum GDFS1009 and B. amyloliquefaciens 1841 were displayed in Table 1. The second order polynomial coefficients were determined and examined using the Minitab package version 18. The general type of the second-degree polynomial equation was resolved as depicted by Karuppiah et al. (2013). Statistical investigation was carried out using Minitab package version 18. The anticipated values for the growth of T. asperellum GDFS1009 and B. amyloliquefaciens 1841 were confirmed by a trial using the optimum values of the factors obtained from the CCD response optimization.
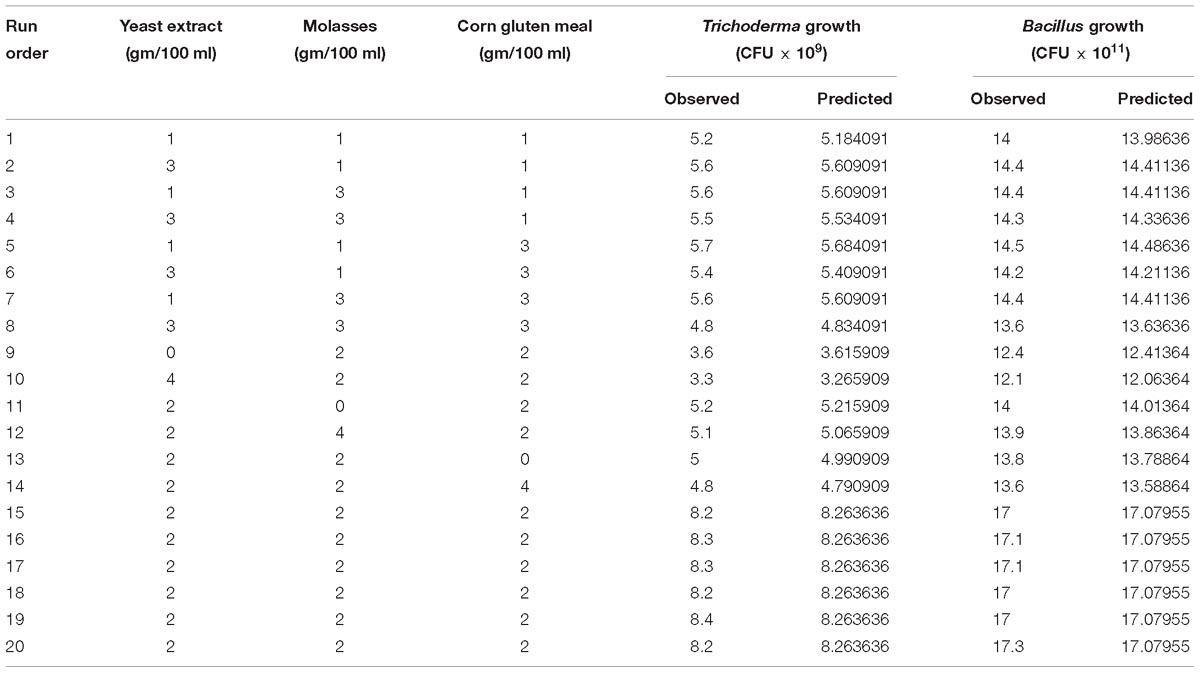
Table 1. Central composite design and results along with the observed and predicted values of the T. asperellum and B. amyloliquefaciens growth in the co-culture conditions.
Gene Expression Study
The expression of genes related to the sporulation and secondary metabolites is induced by a several environmental stimuli (Netzker et al., 2015). Hence, the expression of the genes related to sporulation (VEL 1, TMK, GPR 1, BLR 1, BLR 2, and ENV1) (Mukherjee et al., 2003; Casas-Flores et al., 2004; Brunner et al., 2008; Bazafkan et al., 2017), mycoparasitism related enzymes (NAG 1, NAG 2, PAP A, PAP B, ECH, AF, ACC, BGN13, BGN 16, and EG1) (Chet et al., 2004; Viterbo et al., 2010; Yang, 2017) secondary metabolism (genes encoding three NRPSs, two PKSs, O-methyl transferase B, and cytochrome P450) (Mukherjee and Kenerley, 2010) and antioxidants (NOX and CAT) (Montero-Barrientos et al., 2011) of Trichoderma. In addition, macrolactin and difficidin genes (Goodson et al., 2017) of B. amyloliquefaciens were studied in the axenic and co-culture of the T. asperellum and B. amyloliquefaciens grown in the YMC medium at 4th day.
For RNA isolation, the cultures were snap-frozen in liquid nitrogen, ground, and extracted using TRIeasy total RNA extraction reagent (YEASEN) according to the manufacturer’s protocol. For RT-PCR, cDNA was synthesized from 1 μg of RNA using Prime Script RT reagent kit with DNA eraser (Takara). Real-time PCR was performed using Super Real premix plus SYBR green kit (Tiangen Biotech) in the Roche light cycler 96. The PCR conditions were as follows: 95°C for 5 min, followed by 40 cycles of 95°C for 10 s, 60°C for 30 s, and 72°C for 30 s. Each PCR reaction was carried out in triplicate. Subsequently, threshold-dependent cycling, melting was executed from 60 to 95°C at 0.2°C/s melt rate with a smooth curve. Primer specificity was confirmed through melt curve study. Cq values were normalized to the 18S rRNA and 16S rRNA Cq values for 2−ΔΔCT relative quantification. 18S rRNA and 16S rRNA genes were used as the reference gene for Trichoderma and Bacillus, respectively. The monocultures were treated as the control. The sequences of the primers used for real time PCR are given the Supplementary Table S1.
Enzyme Assay
The axenic and co-culture of T. asperellum and B. amyloliquefaciens were cultured in YMC medium. The cultures were vacuum-filtrated, and the filtrate was used as the crude protein extract. Each enzyme activity was assayed twice with three replicates. Chitinase activity was assessed using a chitinase enzyme Activity Determination Kit (Shanghai Cablebridge Biotechnology Co., Ltd.), according to the manufacturer’s instructions. An enzymatic unit was defined as that releasing 1.0 mmol of p-nitrophenol from the appropriate substrate per min at 37 °C. Activities of β-1,3-glucanase and cellulase were measured using laminarin and carboxy methyl cellulose as the substrates, respectively. β-1,3-glucanase and cellulase activity was assessed using a β-1,3-glucanase and Cellulase enzyme Activity Determination Kit (Shanghai Cablebridge Biotechnology Co., Ltd.), respectively, according to the manufacturers instruction. The protease activity was assayed using casein as a substrate using the Neutral Protease Activity Determination Kit (Shanghai Cablebridge Biotechnology Co., Ltd.) according to the manufacturers instruction.
Analysis Secondary Metabolites Using GC MS Analysis
The axenic and co-culture of T. asperellum GDFS1009 and B. amyloliquefaciens 1841 were grown in shake flask culture on YMC medium (Yeast extract 2%; Molasses 2% and Corn Gluten meal 2%). After fermentation for 7 days at 28°C at 180 rpm/min, fermentation broths were filtered. To extract the secondary metabolites from the axenic and co-culture of T. asperellum GDFS1009 and B. amyloliquefaciens 1841 fermented broth, the filtered liquid culture (4 L) was mixed with equal volume of ethyl acetate (4 L) and shaken vigorously for 5 min in separating flask. After resolving for 1 h, the organic phase was separated and concentrated to dryness by means of rotary evaporator.
Bioactive metabolites present in the ethyl acetate extract of the axenic and co-culture was resolved using gas chromatography– mass spectroscopy systems. The 7890A gas chromatograph and a 5975C mass spectrometer (Agilent Technologies, Milan, Italy) were utilized to perform GC-MS investigation. The HP-5 GC column (30 m × 0.32 mm i.d., 0.50 μm film thickness) were utilized to distinguish the secondary metabolites as described by Srinivasa et al. (2017). The helium gas at the stream rate of 1 ml/minute was utilized to distinguish the secondary metabolites. The recognized metabolites were compared with the mass spectra and the information system libraries of Wiley-2009 and NIST-2007.
Antagonism Assays
Fifty ml of the axenic and co-culture fermented broth were filtered and blended into 100 ml of PDA medium and poured into the petri dishes. The pathogens [F. oxysporum, Fusarium graminearum (wheat head blight and root rot pathogen and maize stack pathogen), and B. cinerea] were inoculated into the petri dishes and incubated at 28°C for 5 days.
The abilities of the axenic and co-culture respond to the inhibition of pathogen growth were studied using the simulated antagonistic assay (Mendoza-Mendoza et al., 2003). Briefly, the axenic and co-cultures were prepared in YMC medium, washed and transferred into 4 days old F. graminearum (wheat head blight and root rot pathogen) culture grown in the Vogel’s minimal medium (VMS) medium (Davis, 2000). After 24 h incubation with shaking, the spores of F. graminearum, T. asperellum GDFS1009 and B. amyloliquefaciens 1841 were counted using the light microscope. The axenic and co-cultures were harvested, frozen, and the RNA was extracted. Induction of the mycoparasitism-related gene prb1/sp1 encoding serine protease, which response to F. graminearum (Pozo et al., 2004) was assessed by quantitative RT-PCR.
Seed Germination Assay
The spore suspension was adjusted to 5 × 109 ml and supplemented with 0.15% (v/v) of seed coating agents (Jilin Bada Pesticide Co. Ltd.) as an adhesive. The 20 g of wheat seeds (Ningmai 13) were disinfected and treated with the 2 ml of each axenic and co-culture spore suspension. Ten seeds from every treatment were set in to the Petri dish containing Whatman filter paper in triplicates. Sterilized distilled water was added to maintain the moisture and the seeds were sprouted in dark at 25°C. The developmental parameters, for example, shoot length, root length, seedling length, seedling wet weight, seedling dry weight and vigor index were recorded after 5 days.
Biocontrol Assays Under Greenhouse Conditions
The wheat seeds treated with axenic and co-culture were germinated and planted in pot soil, and grown at 28°C for 12 h under lights, and at 24°C in the dark for 12 h. One month seedlings were prepared for the assay. The spore suspension of axenic and co-culture grew in YMC medium was collected and diluted to a concentration of 1 × 106 conidia ml−1 with sterile distilled water and evenly applied to the potting soil. After treatment with axenic and co-cultures for 7 days, the conidial suspension of F. graminearum was prepared to a final concentration of 1 × 106 conidia ml−1 and applied to the potting soil. Inoculation with the only pathogen and distilled water was taken as controls. The plant growth promoting and biocontrol parameters such as shoot length, root length, wet weight, dry weight and number of grains and disease index was calculated as described by Wang et al. (2015).
Statistical Analysis
All these experiments were carried out using three replicates and were repeated at least twice, with reproducible results. The figures were plotted using microsoft office excel and origin 6.0 with standard error bars. The medium was optimized statistically using Minitab 18 software. Values were given as mean ± SEM. For multiple comparisons, two-way ANOVA with post hoc LSD, Duncan and Bonferroni were performed using the SPSS 2.0. Student’s t-test was used to analyze the gene expression between the axenic and co-cultures using the SPSS 2.0. P < 0.05 was considered as significant.
Results
Screening of Different Media for the Growth of T. asperellum (TA) and B. amyloliquefaciens (BA) in the Axenic and Co-culture
The growth of T. asperellum (TA) and B. amyloliquefaciens (BA) in the axenic and co-culture conditions in complete and chemically defined culture media were shown in Table 2. The maximum growth of T. asperellum and B. amyloliquefaciens was observed in the 48th hour TA pre-culture inoculated with the BA in YMC medium followed by NB and TY medium. Whereas, the growth of Trichoderma was affected in the co-culture of B. amyloliquefaciens inoculated into the 0th hour TA pre-culture. The growth of T. asperellum and B. amyloliquefaciens axenic cultures were increased in the YMC medium. The results indicated that axenic and co-culture of T. asperellum and B. amyloliquefaciens were enhanced in the YMC medium. Further, the sequential inoculation of T. asperellum and B. amyloliquefaciens was effective than that of co- inoculation.
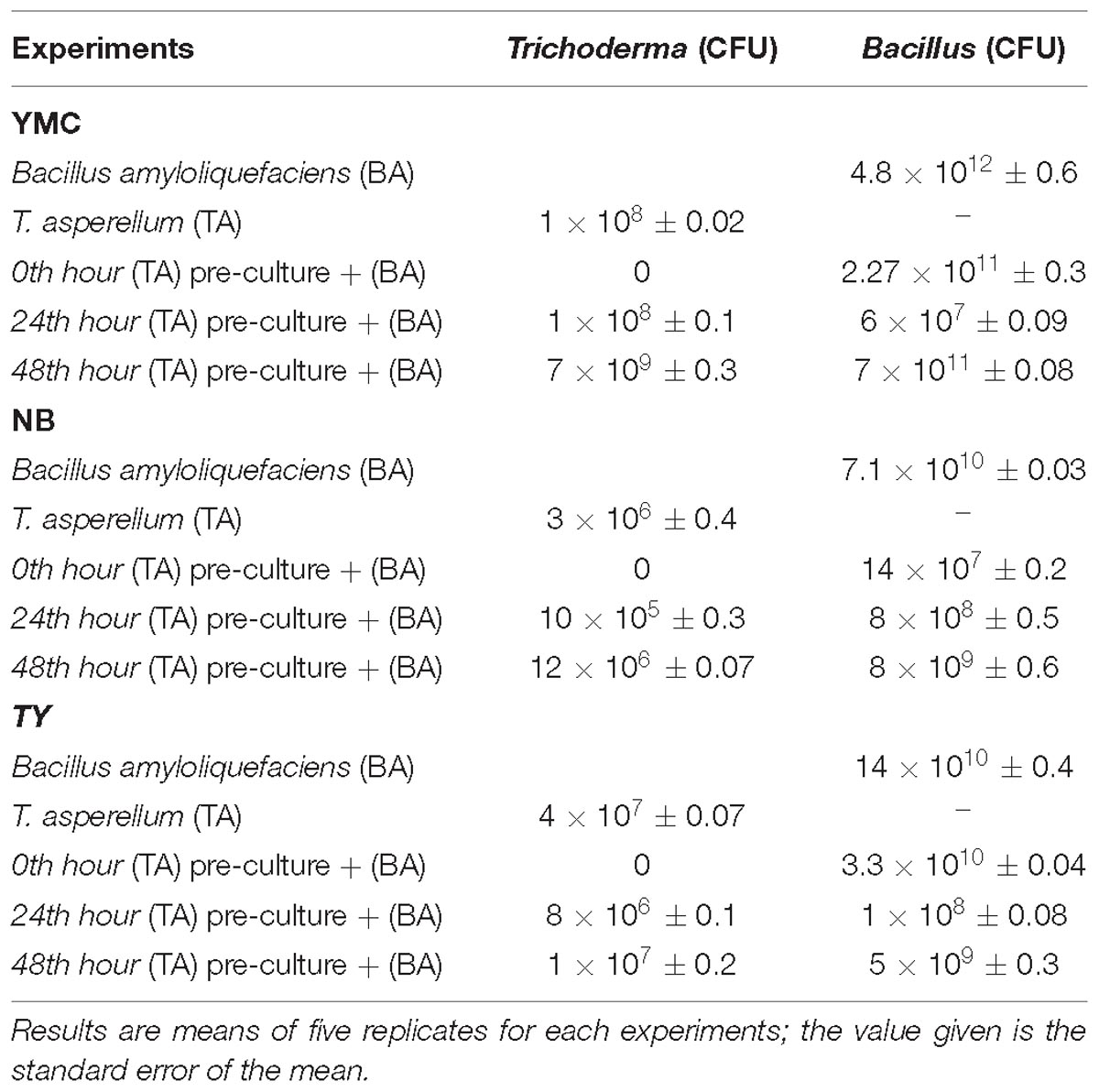
Table 2. Growth rate of T. asperellum and B. amyloliquefaciens in axenic and co-culture on different media.
Optimization of Medium Components Using Central Composite Design
Preliminary experiments showed that YMC medium influenced the growth of T. asperellum and B. amyloliquefaciens in both axenic and co-culture conditions. So, Central Composite Design was used to optimize the medium components of YMC medium. The consequence of yeast extract, molasses and corn gluten meal at five levels and their relations on the growth of T. asperellum and B. amyloliquefaciens was shown in Table 1.
Analysis of variance (ANOVA) for the growth of T. asperellum and B. amyloliquefaciens was depicted in Table 3. The results revealed that R2 was 0.9992 and 0.9985, demonstrating that the experiment was fitted and elucidate 99.92 and 99.85% of the variability on the growth of T. asperellum and B. amyloliquefaciens, respectively, in co-culture conditions. Similarly, F-Test of the regression was also reported to be significant. Whereas, the lack of fit, 0.956 and 0.988 obtained for T. asperellum and B. amyloliquefaciens were not significant. These outcomes demonstrate that the model picked clarified the impacts of yeast extract, molasses and corn gluten meal on the growth of T. asperellum and B. amyloliquefaciens in the co-cultivation. The second-order polynomial equation on the growth of T. asperellum (Y1) and B. amyloliquefaciens (Y2) were as follows.
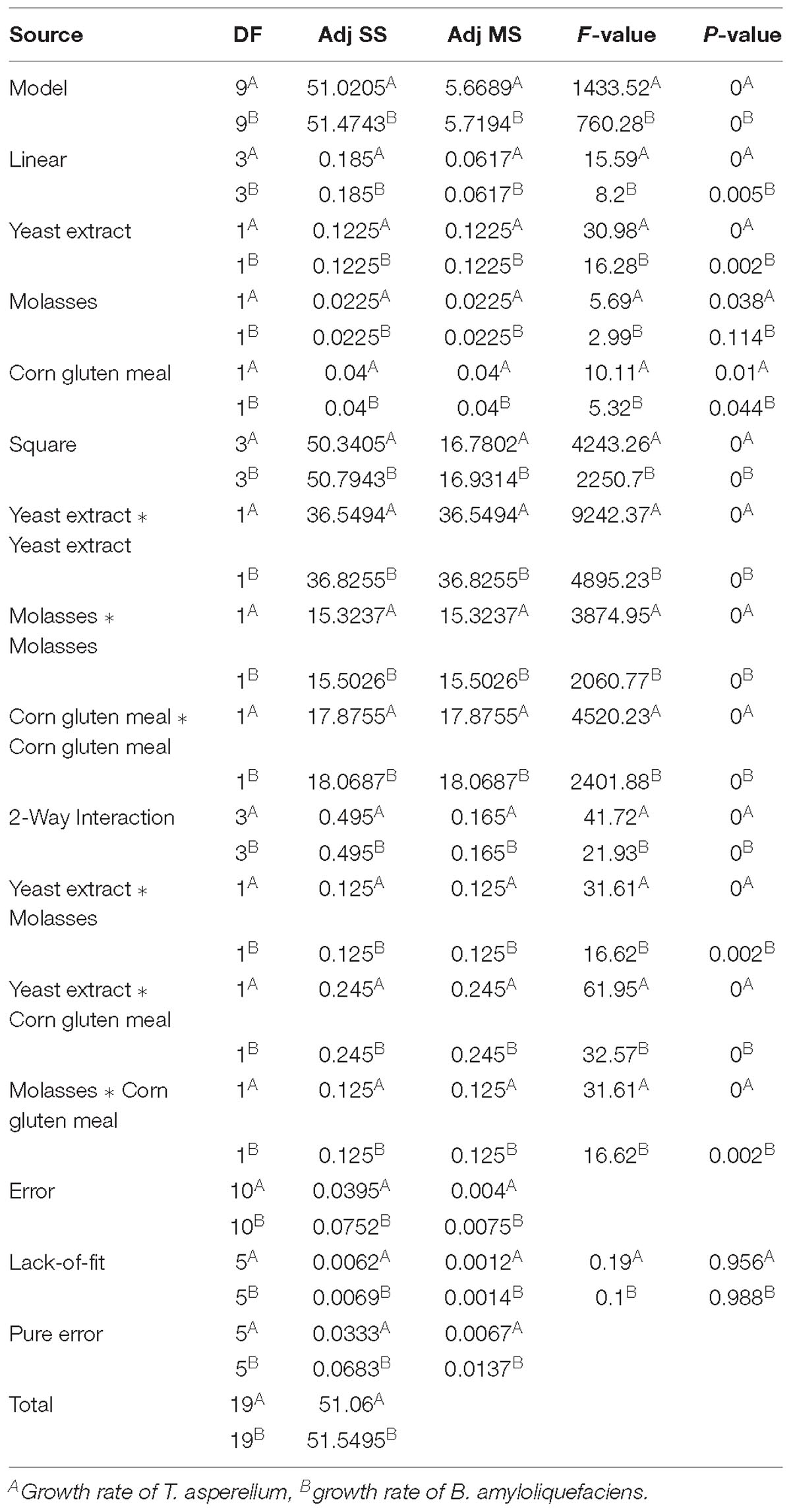
Table 3. Statistical analysis of central composite design showing coefficient values, t- and p-values for each variable on growth of T. asperellum and B. amyloliquefaciens.
Where Y1 and Y2 is the growth of T. asperellum, and B. amyloliquefaciens, respectively; X1, X2, and X3 are the coded values of the test variables yeast extract, molasses, and corn gluten meal, respectively.
The results demonstrated that yeast extract, molasses, and corn gluten meal had positive effect on the growth of T. asperellum, and B. amyloliquefaciens. Among the three factors tested, yeast extract had the most noteworthy effect on the growth of T. asperellum, and B. amyloliquefaciens, as given by the direct coefficient. Followed by, molasses and corn gluten meal showed the positive effect on the growth of T. asperellum, and B. amyloliquefaciens. These variables likewise demonstrated huge negative quadratic effects on the growth of T. asperellum, and B. amyloliquefaciens, demonstrating that growth of T. asperellum, and B. amyloliquefaciens, increased and decreased based on the ideal level of each factors. The association between these parameters was likewise noteworthy, as appeared low P-values (P < 0.05) for intelligent terms. Fitted reaction for the above deterioration was plotted (Figure 1). The charts were plotted for 2 factors while keeping the other on central level. The surface plots confirmed that factors are unimodal in nature. The optimal concentrations of yeast extract, molasses, and corn gluten meal were anticipated in genuine units and they were 2.0 g each with a growth of T. asperellum, and B. amyloliquefaciens. The confirmation experiments showed that colony count of T. asperellum and B. amyloliquefaciens were 8.7 × 109 and 17.3 × 1011, respectively. This was higher than the anticipated results and confirmed the accuracy of the model.
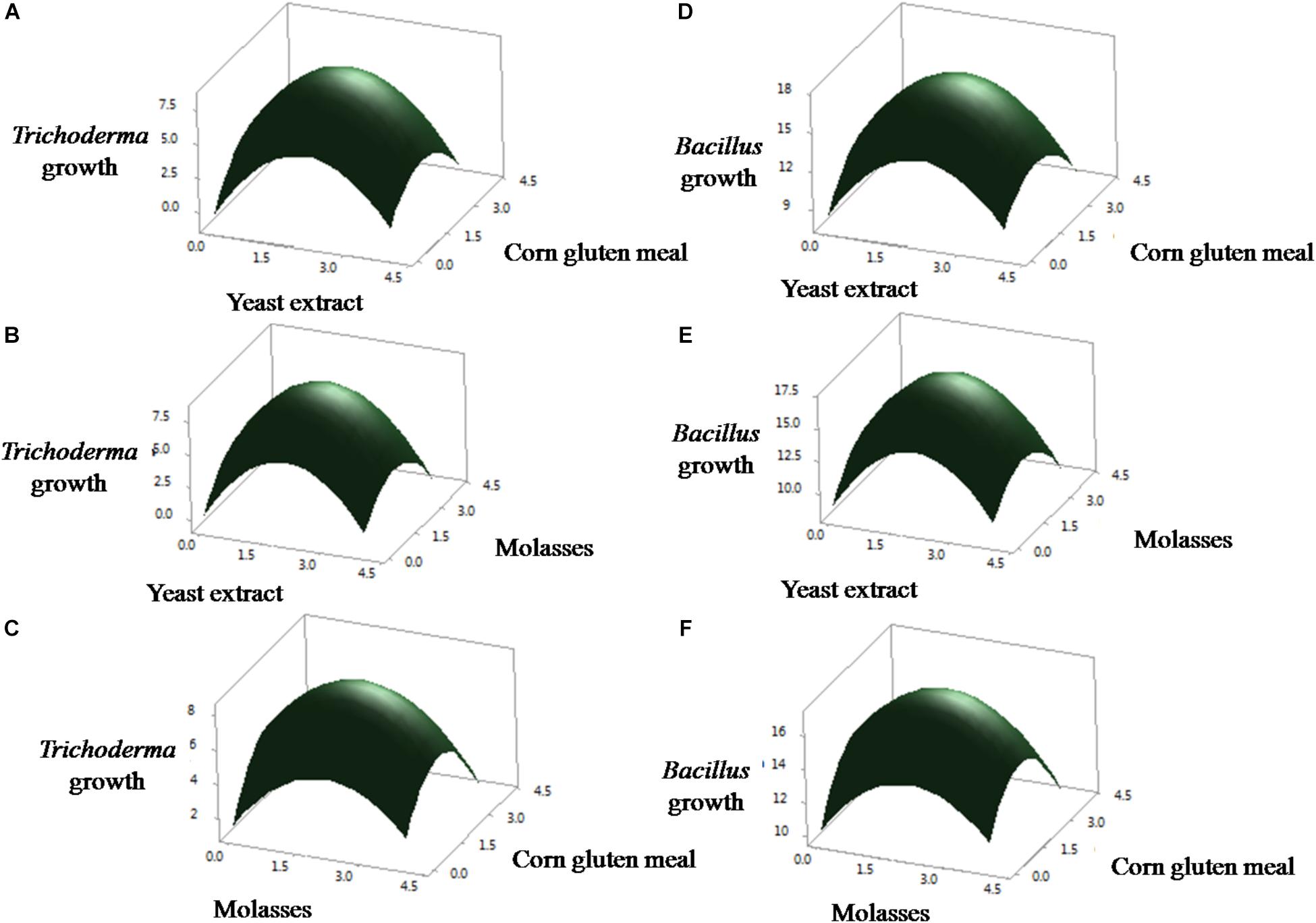
Figure 1. Statistical optimization of the T. asperellum and B. amyloliquefaciens growth in co-culture using RSM. (A–C) Effect of yeast extract, corn gluten meal and molasses on the growth of T. asperellum. (D–F) Effect of yeast extract, corn gluten meal and molasses on the growth of B. amyloliquefaciens.
Differential Gene Expression in the Co-culture of T. asperellum and B. amyloliquefaciens
Distinct molecular expressions were observed for each species under co-cultivation conditions. This was measured by means of qRT-PCR from co-cultivation treatments contrasted to their particular axenic controls. Differentially expressed genes were characterized as fold change among axenic and co-cultivation. The number of differentially expressed genes belonging to each functional category was normalized against the reference genes, 18S rRNA and 16srRNA genes for T. asperellum and B. amyloliquefaciens, respectively.
Differences in gene functions were observed between axenic and co-culture. Co-cultivation resulted in the upregulation of T. asperellum genes involved in the sporulation, secondary metabolism, antagonism and plant growth promoting related enzymes and antioxidants. The sporulation-related genes such as Velvet (Vel 1), Mitogen-Activated Protein Kinase (TMKa), G protein receptor 1 (GPR1), blue-light-regulated genes (BLR1 and BLR2) were upregulated in T. asperellum in response to B. amyloliquefaciens (Figure 2A). Similarly, T. asperellum genes involved in secondary metabolisms such as non-ribosomal peptide synthetase (NP1 and NP2), Putative ferrichrome synthetase (NP3), Cytochrome P450 (Tri 13) 1, O-methyl transferase (OMT) and Polyketide synthetase (PK1 and PK2) were also upregulated (Figure 2B).
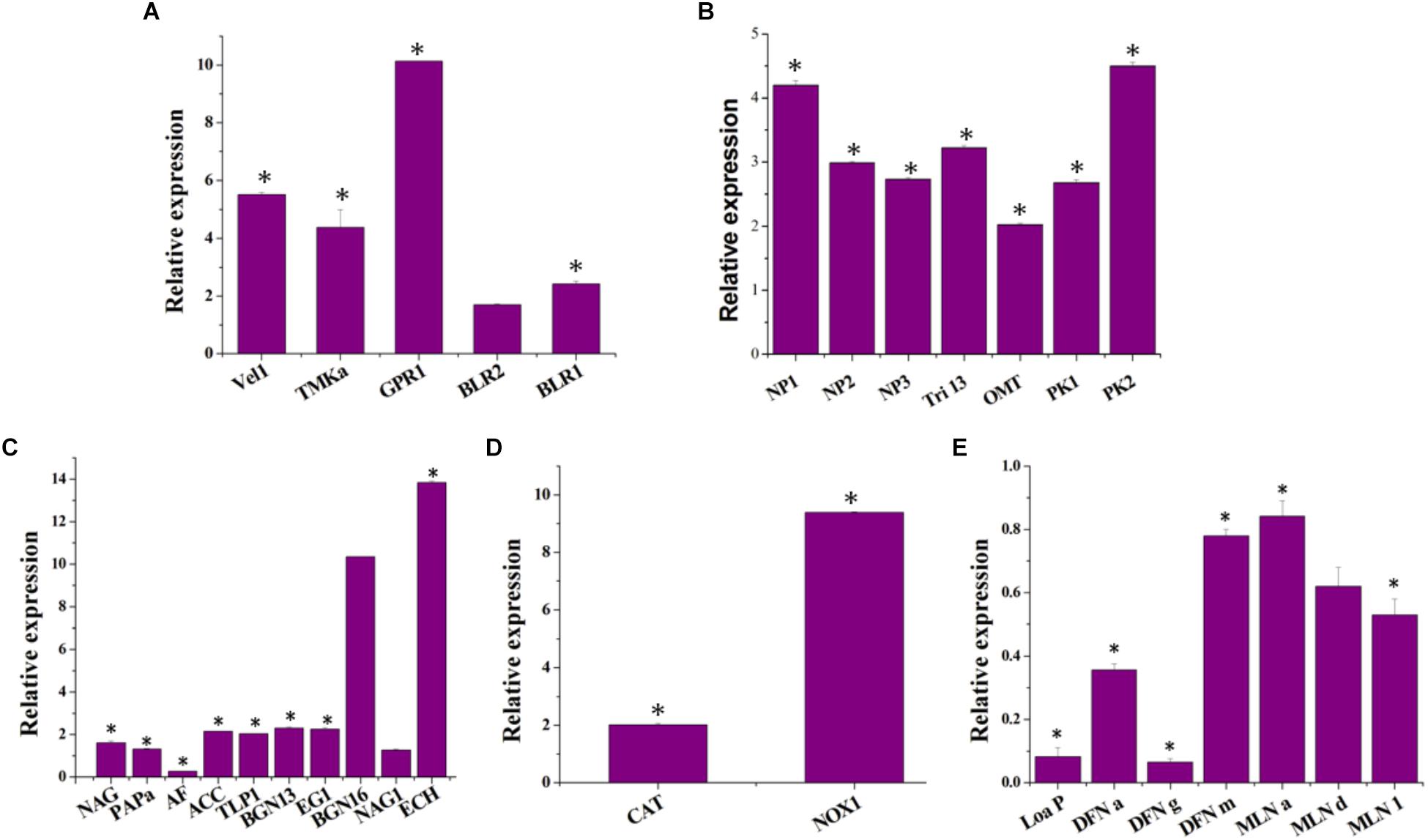
Figure 2. Impact of co-cultivation on the transcript levels of (A) the T. asperellum morphology related genes [Mitogen-Activated Protein Kinase (TMKa), G protein receptor 1 (GPR1), blue-light-regulated genes (BLR1 and BLR2) and ENVOY (ENV 1)], (B) secondary metabolism related genes [non-ribosomal peptide synthetase (NP1 and NP2), Putative ferrichrome synthetase (NP3), Cytochrome P450 (Tri 13) 1, O-methyl transferase (OMT) and Polyketide synthetase (PK1 and PK2)]; (C) mycoparasitism-related genes [chitinase (ech42), β-1,3-glucanase (bgn13.1), β1,6-glucanase (bgn16.1), β-1,4-glucanase (egl), N-acetyl-glucosaminidases (nag1 and nag2), aspartyl protease (Pap A), trypsin-like protease (TLP 1) and α–L–arabinofuranosidases (AF)]; and plant growth promoting enzyme [1–Aminocyclopropane–1–carboxylate (ACC) deaminase (ACC)]. (D) Anti-oxidant genes [NADPH oxidase (NOX), catalase (CAT)] and (E) genes encoding B. amyloliquefaciens macrolactin and difficidin in the co-culture [intrinsic terminators located within the polyketide synthase (PKS) gene cluster encoding for the antibiotic difficidin (dfn) (Loa P), beginning, middle and end of the difficidin operon (dfnA, dfnG, and dfnM); beginning, middle and end of the macrolactin operon (MLN a, MLN d, and MLN i)]. Data resulted from biological triplicate cultures with qPCR technical duplicates. The value in parentheses is the standard error of the mean. ∗represent significant differences between the axenic and co-culture (P < 0.05).
To determine whether co-cultivation is involved in the regulation of genes encoding the pathogen cell wall degrading enzymes, the transcription of six mycoparasitism-related genes Ech42 (chitinase), BGN13.1 (β-1,3-glucanase), BGN16.1 (β1,6-glucanase), Egl (β-1,4-glucanase) and NAG1 and NAG2 (N-acetyl-glucosaminidases) were measured in axenic and co-culture. The expression of Ech42 has upregulated 13.83-fold in co-culture compared to the axenic culture of T. asperellum (Figure 2C). BGN13 and BGN16 gene expression levels were also induced by B. amyloliquefaciens (Figure 2C). In Co-culture NAG1, NAG2, and EG1 transcription were increased 1.26, 1.6, and 2.25 folds compared to the axenic culture. The expression of aspartyl protease (PAP A), trypsin-like protease (TLP 1) and 1–aminocyclopropane–1–carboxylate deaminase (ACC) were up-regulated 1.31-, 2.02-, and 2.14-folds, respectively. As shown in Figure 2D, the highest transcript levels of NADPH oxidases (NOX) and catalase (CAT) were detected in the co-cultivation. B. amyloliquefaciens showed transcriptional responses to competitive co-cultivation (Figure 2E). 2−ΔΔCT of the differentially expressed macrolactin and difficidin genes of B. amyloliquefaciens were less than 1 (Figure 2E), which reveals that these genes were down-regulated in response to competitive co-cultivation.
Induction of Enzyme Production by the Co-cultivation of T. asperellum and B. amyloliquefaciens
Taking into account on the enhanced gene expression of enzymes related to mycoparasitism in co-culture, we compared the chitinase, β 1–3 glucanase, cellulase and protease activities in the axenic and co-culture medium. As shown in Figure 3, higher levels of chitinase activity were detected in the co-culture than in the axenic culture of T. asperellum; these differences were coinciding with the gene expression study. Whereas, chitinase, β 1–3 glucanase and cellulase activities were not detected in the axenic culture of B. amyloliquefaciens.
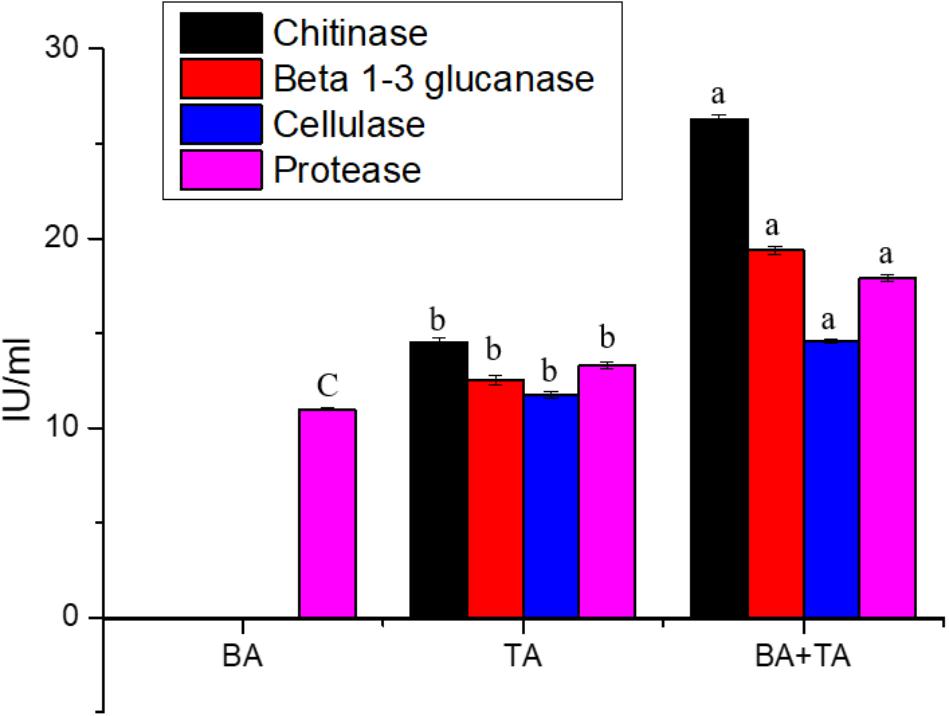
Figure 3. Enzyme activities associated with culture filtrates from the axenic and co-culture of T. asperellum and B. amyloliquefaciens grown on YMC medium. Results are means of five replicates for each treatment; the value in parentheses is the standard error of the mean. Different letters above the bars are significantly different (P < 0.05) based on the ANOVA.
Induction of Secondary Metabolites by the Co-cultivation of T. asperellum and B. amyloliquefaciens
We hypothesized that axenic and co-culture differs from one another in their production of secondary metabolites (Figure 4A). After fermentation in YMC medium, the secondary metabolites were extracted using ethyl acetate and analyzed via gas chromatography-mass spectrometry. The compounds with various classes such as Heterocyclic compounds, Aromatic hydrocarbon, Pyrone derivative, Polyketide, Amine, Alkenes, Alkane, Ethers, Alcohols, Fatty acids, Terpenes, Halogens, Fatty esters, Polymers, Amino acids, Nitrogen-containing compounds, Amino fatty acids, Sulfur-containing compounds, Peptide, Cyclic peptide, Nitrosamines, sulfenamides, Aldehydes, Ketones, and Organophosphorus were obtained (Supplementary Table S2). There was notable variability in the number of metabolites between axenic and co-culture. We observed 68 metabolites in the co-culture, which was higher than the axenic culture of T. asperellum (42) and B. amyloliquefaciens (32) (Figures 4B,C).
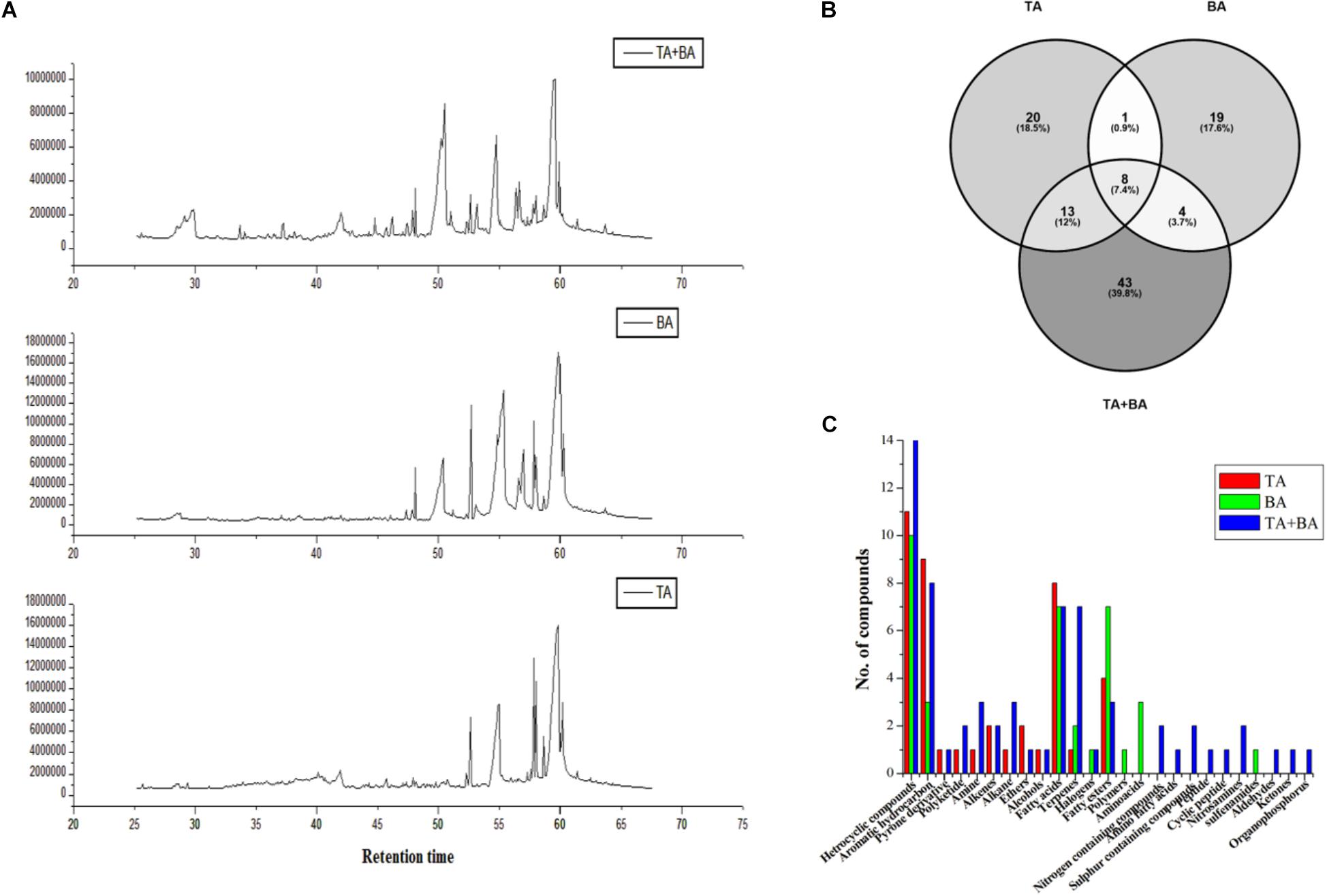
Figure 4. Metabolites differences between the axenic and co-culture of T. asperellum and B. amyloliquefaciens. (A) Differences in the GC MS spectrum (B) total number of metabolites detected in the axenic and co-culture (C) metabolites class differences in the axenic and co-culture.
Induction of Antagonistic Activity by the Co-cultivation of T. asperellum and B. amyloliquefaciens
To determine whether the product of axenic and co-culture is involved in the antagonistic capacity against phytopathogenic fungi, PDA or PDA supplemented axenic and co-culture filtrate plates were inoculated in the center with mycelial plugs of F. graminearum (wheat head blight and root rot pathogen and maize stack pathogen), F. oxysporum and B. cinerea. The radial growth of F. graminearum was highly inhibited by both co-culture filtrates followed by the axenic culture filtrate of T. asperellum, and B. amyloliquefaciens, compared to its respective control. Similarly, the radial growth of F. oxysporum and B. cinerea was highly inhibited by the co-culture filtrates (Figure 5 and Supplementary Figure S1).
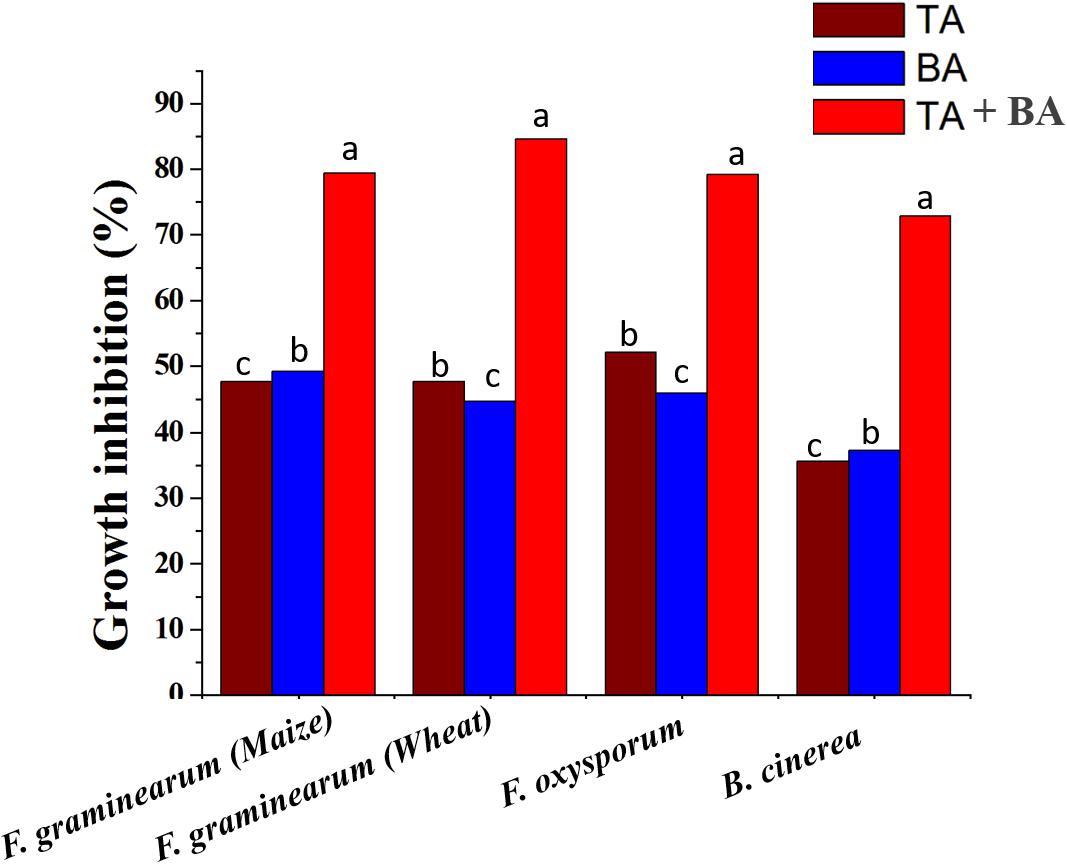
Figure 5. The inhibitory spectrum of axenic and co-culture of T. asperellum and B. amyloliquefaciens fermentation liquor against plant pathogenic fungi. Results are means of five replicates for each treatment; the value in parentheses is the standard error of the mean. Different letters above the bars are significantly different (P < 0.05) based on the ANOVA.
In the stimulated antagonism assay, the spore count of F. graminearum (wheat head blight and root rot pathogen) was reduced to 7∗103 and 4.5∗102 in the TA and TA + BA, respectively (Table 4). The inhibition of the F. graminearum was increased in the co-culture conditions (Figures 6A–D). The growth inhibition of F. graminearum by the axenic and co-culture of T. asperellum and B. amyloliquefaciens were correlated with the expression of mycoparasitism related gene tvsp1/prb1 (coding a serine protease). The relative gene expression of tvsp1/prb1 gene was highly induced in the co-culture of TA + BA compared to the TA (Figure 6E).
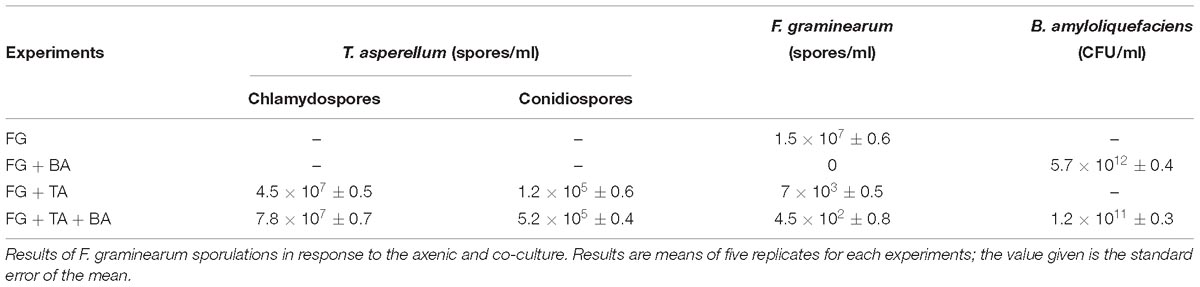
Table 4. Antagonist interactions between the axenic and co-culture in stimulated antagonism assay against F. graminearum (wheat head blight and root rot pathogen).
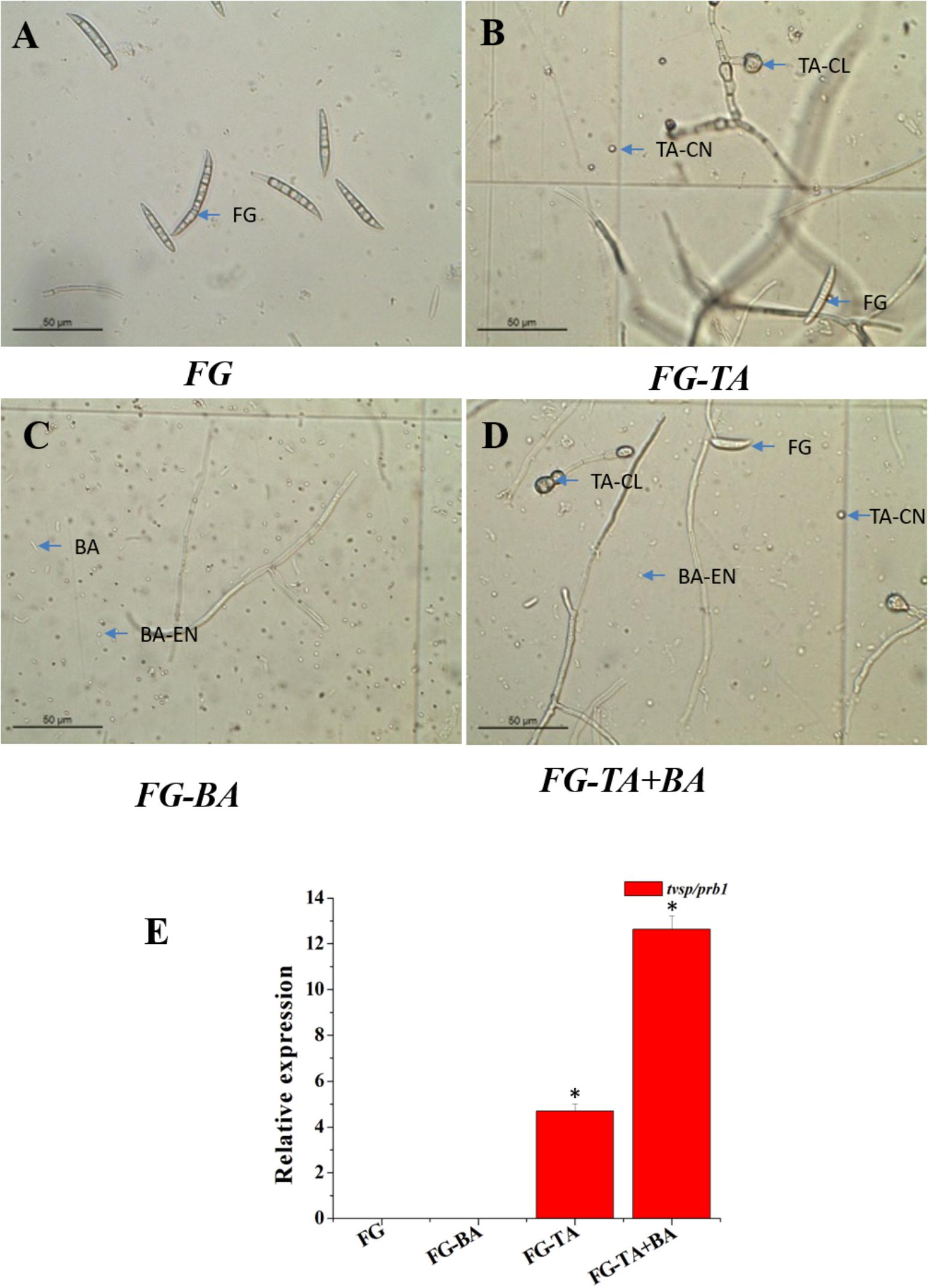
Figure 6. Antagonist interactions between the axenic and co-culture in stimulated antagonism assay against F. graminearum (wheat head blight and root rot pathogen). (A) Spores of F. graminearum (FG). (B) Sporulation of F. graminearum (FG) in response to the axenic culture of T. asperellum (TA). (C) Sporulation of F. graminearum (FG) in response to the axenic culture of B. amyloliquefaciens (BA). (D) Sporulation of F. graminearum (FG) in response to the Co- culture of T. asperellum (TA) and B. amyloliquefaciens (BA). (E) Induction of serine protease (tvsp1/prb1) expression in a stimulated antagonism assay (RT-PCR). F. graminearum spores (FG), T. asperellum conidiospores (TA-CN), T. asperellum chlamydospores (TA-CL), B. amyloliquefaciens (BA), B. amyloliquefaciens endospores (BA-EN). ∗represent significant differences between the axenic and co-culture (P < 0.05).
Induction of the Wheat Seed Germination by the Co-cultivation of T. asperellum and B. amyloliquefaciens
In the current study, it was observed that the seeds treated with the axenic and co-culture of T. asperellum and B. amyloliquefaciens were essentially higher than control (untreated seeds) (Table 5). Treatment TA + BA was found to be significantly superior and effective with 3.76 and 6.48 cm of the shoot and root length of wheat variety Ningmai 13 compared to the control, which was followed by TA (3.34 and 5.56 cm) and BA (2.08 and 4.22 cm). The positive impact of seed treatment was also documented for seedling length, wet weight, dry weight and vigor index. TA + BA treatment excelled overall significant superior performance by contributing seedling length (10.24 cm), wet and dry weight (2.66 and 0.65 mg) and vigor index (984).
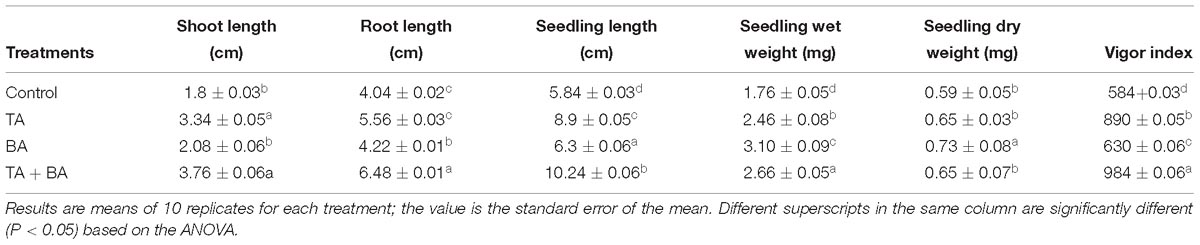
Table 5. Effect of axenic and co-culture of T. asperellum and B. amyloliquefaciens on wheat seed germination.
Induction of the Wheat Growth and Bio-Control Activity by the Co-cultivation of T. asperellum and B. amyloliquefaciens
The axenic and co-culture of T. asperellum and B. amyloliquefaciens were significantly increased the emergence of wheat seedlings compared with the control, which is also consistent with the increased emergence with the presence of F. graminearum (Figure 7A). Compared to the axenic culture of T. asperellum (TA) and B. amyloliquefaciens (BA), the co-culture of TA + BA resulted in the highest plant growth and emergence of wheat grains both in the presence and absence of F. graminearum (Figure 7B). similarly, the wet and dry weight of the plants treated with TA + BA was also significantly increased compared to the axenic culture (Figures 7B,C). 7 days after pathogen infection, all treatments were observed to ensure biocontrol efficiency compared to the control. The wheat plants treated with co-culture of T. asperellum and B. amyloliquefaciens showed healthy with fewer symptoms in root compared with the control treatment, which was only inoculated with the pathogen (Figures 7D–F). Followed by, the axenic culture of T. asperellum and B. amyloliquefaciens effectively controlled root rot, and the disease index (Figure 7D).
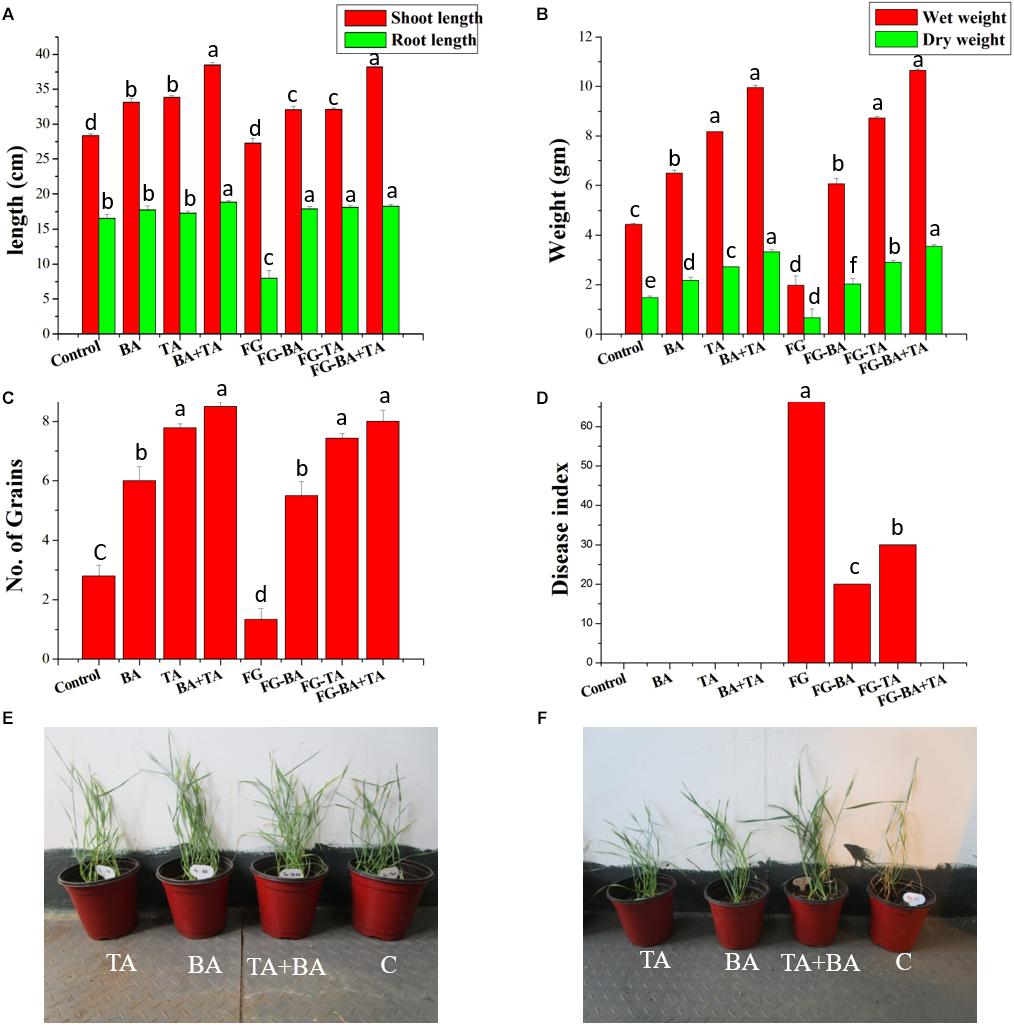
Figure 7. Effect of axenic and co-culture of T. asperellum and B. amyloliquefaciens on the plant growth and biological control of F. graminearum in green house conditions. (A) Shoot and root length of different treatments (B) wet and dry weight of wheat plants (C) number of grains per plant (D) disease index of F. graminearum in control and treated (E) Wheat plants grown in pots treated with TA (T. asperellum); BA (B. amyloliquefaciens) TA + BA (T. asperellum and B. amyloliquefaciens) C (control). (F) Wheat plants grown in pots containing F. graminearum treated with TA; BA; TA + BA; C (control). Results are means of five replicates for each treatment; the value in parentheses is the standard error of the mean. Different letters above the bars are significantly different (P < 0.05) based on the ANOVA.
Discussion
Co-cultivation methods have long been used to study the interactions between microbial populations. Recently, Co-cultivation has become a particular interest to study the changes in metabolic systems. Further, microbial co-cultivation is an approach motivated by inter-microbial competition and communication for the stimulation of novel metabolites by the activation of silent genes (Netzker et al., 2015). The usage of microbial consortium in the agriculture system may improve efficacy, stability and uniformity of the microbes in the plant disease control and plant growth promotion under diverse soil and environmental conditions (Stockwell et al., 2011). Similarly, co-culture system also enhances the plant growth and defense potential. The application of microbial co-culture stimulates the antioxidant enzyme activities and the phenylpropanoid pathway increases the total phenolics, proline, and pathogenesis-related (PR) proteins in the plants. Sarma et al. (2015) reviewed the consortium of biological control agents (BCAs) to control plant diseases in comparison with the use of individual agents. The beneficial effect of T. asperellum and B. amyloliquefaciens includes protection against pathogens, growth promotion, induction of disease resistance, and production of bioactive compounds with antibiotic and antitumor properties (Brunner et al., 2005; Xie et al., 2018). The previous study on the co-cultivation of B. amyloliquefaciens and T. asperellum inhibited the growth of B. cinerea (Wu et al., 2018). Hence in the present study, stimulation of diverse cellular processes such as sporulation, secondary metabolism, enzyme productions in the co-culture of T. asperellum GDFS1009 and B. amyloliquefaciens 1841 were studied to evaluate its effect on biocontrol, and plant growth.
Selection and optimization of the culture medium are expected to improve the growth of both B. amyloliquefaciens and T. asperellum for future biocontrol agent industry production. Initially, basic approaches like the screening of different media were used to enhance the growth of both cultures. Taking into consideration of three different mediums (NB, TY, and YMC), YMC enhanced the growth of both B. amyloliquefaciens and T. asperellum. The results inferred that yeast extract, molasses, and corn gluten meal enhance the growth of both T. asperellum GDFS1009 and B. amyloliquefaciens 1841. Yeast extract contains less complex mixes, for example, amino acids and peptides with the rich wellsprings of nutrients particularly those having a place with B-complex. Molasses contains huge measures of sugars, nutrients, and minerals, while corn gluten meal contains about 65% of rough proteins. It uncovers that the YMC medium containing all supplements, for example, carbohydrates, proteins, vitamins, and minerals. Thus, yeast extract, molasses, and corn gluten meal were chosen and further upgraded by utilizing Central Composite Design. The CCD results uncovered a high comparability between the anticipated and test results, which mirrored the exactness and appropriateness of RSM to upgrade the growth of T. asperellum GDFS1009 and B. amyloliquefaciens 1841 in submerged culture. The growth of the B. amyloliquefaciens and T. asperellum increased with the increment in each parameter up to the certain level, after which it was declined. This could be because of the loss of supplement in culture medium. The surface diagrams inferred the ideal estimations of yeast extract, molasses and corn gluten meal for the greatest growth of T. asperellum (8.2636 × 109), and B. amyloliquefaciens (17.0795 × 1011) was 2 g each. So as to approve the sufficiency of the model, confirmation tests were directed under anticipated ideal maturation conditions. The outcomes have demonstrated that the model is acceptable.
Conidiation of the Trichoderma is influenced by light, mechanical injury and environmental conditions such as nutrients and pH. The photoreceptor complex BLR-1/BLR-2, ENVOY, VELVET, and NADPH oxidases have been involved in the conidiation process. In performance with these genes, conserved signaling pathways, such as heterotrimeric G proteins, MAPKs and cAMP-dependent protein kinase A (cAMP-PKA) are also involved in the conidiation process (Carreras-Villasenor et al., 2012). Hence, in the present investigation, the expression of blue-light-regulated genes (BLR1 and BLR2), ENVOY (ENV 1), velvet (Vel1) Mitogen-Activated Protein Kinase (TMKa) and G protein receptor 1 (GPR1) in the axenic and co-culture of the T. asperellum in YMC medium was studied. All these genes were upregulated in the co-culture. These gene expressions were correlated with the increase in sporulation of co-culture. Hence, the technology on the co-cultivation of the T. asperellum and B. amyloliquefaciens could be used to increase the Trichoderma spore concentration. These findings correlated well with the model predicted by the Carreras-Villasenor et al. (2012). In the present study, the nutrient-rich medium and the co-cultivation strategy influenced the expression of blue-light-regulated genes (BLR1 and BLR2), ENVOY (ENV 1), velvet (Vel1), Mitogen-Activated Protein Kinase (TMKa) and G protein receptor 1 (GPR1). But the mechanism behind the activation of these genes is unknown.
The assessment of signaling pathways of Trichoderma revealed that heterotrimeric G-proteins and mitogen-activated protein (MAP) kinases influence biocontrol-relevant mode of actions such as the synthesis of hydrolytic enzymes and antibiotics (Zeilinger et al., 2016). MAPK signaling was also found to be implicated in stimulation of plant systemic resistance in Trichoderma virens (Mukherjee et al., 2003) and stress response in Trichoderma harzianum (Delgado-Jarana et al., 2006). The role of cAMP pathway during Trichoderma biocontrol exposed stimulation of mycoparasitism-associated coiling and chitinase and secondary metabolites (Omann and Zeilinger, 2010). The present study found that expression of Mitogen-Activated Protein Kinase (TMKa) and G protein receptor 1 (GPR1) in co-culture was induced by the B. amyloliquefaciens. The over expression of Mitogen-Activated Protein Kinase (TMKa) and G protein receptor 1 (GPR1) might be responsible to increase the antagonism and biocontrol activity of the T. asperellum and B. amyloliquefaciens co-culture.
The expression of genes concerned in the production of secondary metabolites is directed by a hierarchical network of regulators that respond to multiple environmental signals (Netzker et al., 2015). To address this question, we examined changes in secondary metabolite gene expression of the T. asperellum in respond to the B. amyloliquefaciens. We found that secondary metabolite gene such as non-ribosomal peptide synthetase (NP1 and NP2), Putative ferrichrome synthetase (NP3), Cytochrome P450 (Tri 13) 1, OMT and Polyketide synthetase (PK1 and PK2) were expressed at higher levels in co-culture. Chettri et al. (2012) and Lind et al. (2015) explained that the expression of velvet protein complex alters expression of secondary metabolite gene clusters and developmental processes in many fungi. Similarly, in the present investigation both velvet and secondary metabolite related genes were up-regulated in the co-culture. Further, the expression of velvet genes showed the relationship with sporulation and the regulation of NRPS gene expression. It supports the assumption that fungal secondary metabolism is synchronized by a network of transcriptional regulators with response to different environmental stimuli.
The present study affords an involvement in understanding the role of a T. asperellum protein related to ROS production in the interaction between B. amyloliquefaciens. It has been established that NOX genes are stimulated by the existence of pathogens in mammals and plants (Wang et al., 2016). Montero-Barrientos et al. (2011) detected the motifs for binding the regulators of oxygen metabolism, sexual development, and PR proteins in the nox1 promoter region and suggest that NOX proteins as an important enzyme for sexual development. It was clear that the polysaccharides present in the cell wall of fungal pathogens and plant materials would induce the expression of NOX gene. In the present study, we observed that the NOX gene was also induced by the bacteria. ROS liberation by NADPH oxidases is a common signaling system among all organisms. The sequence of ROS scavenging systems such as ascorbate peroxidases, glutathione, superoxide dismutases, and catalases, associated with NADPH oxidases to maintain the ROS homeostasis in cells (Montero-Barrientos et al., 2011). Similarly, in the present study, we observed the sequential up-regulation of catalase gene in response to the expression of NOX gene in the co-culture. The transcriptomic response of T. harzianum T34 NOX1 over expression with Pythium ultimum upregulated the genes encoding proteases, including aspartic, subtilisin serine, and trypsin-like proteases (Montero-Barrientos et al., 2011). Similarly, we observed that induction of genes encoding aspartyl protease, trypsin-like protease, chitinase, β-1,3-glucanase, β1,6-glucanase, β-1,4-glucanase and N-acetyl-glucosaminidases in co-culture.
The optimistic effect of co-cultivation of fungi on the liberation of enzymes has been studied previously (Lima et al., 2016). However, no reports have illustrated the effects of co-cultivating the two most agriculturally important bacteria and fungus (T. asperellum and B. amyloliquefaciens) on the enzyme production. In this paper, we have addressed this question. Several co-cultivation technologies resulted in enhanced activity for numerous enzymes, but often not for all enzymes. This implies that the co-cultivation does not enhance the protein secretion but rather it enhances the specific enzymes, which was concluded by the estimation of total extracellular protein in co-culture medium. This was also dependent on the carbon source supplemented in the medium. Hence, we used a nutrient-rich media (YMC). The result revealed that co-cultivation can result in specific up-regulation of genes encoding the enzymes such as N-acetyl-glucosaminidases (NAG1 and NAG 2), β-1,3-glucanase (BGN 13), β-1,4-glucanase (EG1), β1,6-glucanase (BGN 16), NAG 1, chitinase (ECH 42) and aspartyl protease (Pap A) and enzyme production such as chitinase, β 1–3 glucanase, cellulase and protease compared to the axenic incubations. Similarly, Copete-Pertuz et al. (2019) identified a triple combination inoculated with Trichoderma viride and Aspergillus terreus into a 7th day preculture of Leptosphaerulina sp. improved ligninolytic enzymes production when compared to the Leptosphaerulina sp. monoculture.
Hydrolytic enzymes and antibiotics of Trichoderma are the most essential components to kill other fungi (Hermosa et al., 2013). In the present study, the highest antagonistic activity and the biocontrol efficiency of the co-culture observed might be due to the overexpression of hydrolytic enzymes and secondary metabolites in the co-culture conditions. In addition to hydrolytic enzymes and antibiotics, proteases like Prb1/Sp1 in co-culture and axenic culture of T. asperellum was induced during the stimulated antagonism assay and proved definitive roles in biocontrol.
Bacillus strains are generally studied for their valuable role in plant growth and biological control of plant disease and pest (Mota et al., 2017). In the present study, the antagonism, biocontrol and plant growth were induced in the axenic culture of B. amyloliquefaciens compared to the control. B. amyloliquefaciens produce polyketides such as macrolactin, difficidin, and oxidifficidin (Goodson et al., 2017). These compounds have been used as biocontrol agents. Hence, we accessed the gene expression of Loa P [intrinsic terminators located within the polyketide synthase (PKS) gene cluster encoding for the antibiotic difficidin (dfn)], dfnA, dfnG, dfnM (beginning, middle and end of the difficidin operon) MLN a, MLN d, MLN i (beginning, middle and end of the macrolactin operon). The results inferred that The B. amyloliquefaciens secondary metabolite genes were not expressed in the co-culture conditions. The reason behind the down-regulation of these genes was unknown.
This study further revealed the highest potential of Bacillus and Trichoderma co-cultivations on the plant growth promotion. The enzyme 1-aminocyclopropane-1-carboxylate (ACC) deaminase (ACCD, EC 4.1.99.4) is widespread among rhizobacteria (Glick, 2014) and reduce ACC, the precursor of ethylene, to α-ketobutyrate and ammonia. Thereby, it induces the growth of plant and root under biotic and abiotic stress (Glick, 2014). For the first time, Zhang et al. (2016) analyzed the ACC deaminase gene of the biocontrol fungus T. asperellum ACCC30536. In this present investigation, the expression of ACC deaminase was up-regulated in co-cultivation. This was supported by the seed germination assay and plant growth.
Comprehensive interactions take place between filamentous fungi and other living materials mostly with the help of volatile metabolites. Secondary metabolites of fungi could play a role in plant defense. This proposes that the volatile metabolite contributes to the antifungal activity (Leylaie and Zafari, 2018). Hence, in the present investigation, the changes in the secondary metabolites in axenic and co-culture were detected using the GC-MS analysis. The most abundant metabolite originally characterized by Collins and Halim (1972) was identified to be 6-pentyl-alpha-pyronein (6-PP). It was found in both axenic and co-culture of T. asperellum. It was identified as one of the key metabolites of T. asperellum. This compound is oxygen-containing heterocyclic compound which produces coconut odor, a typical characteristic of the Trichoderma (Fadel et al., 2015). This compound induces the cellular function, antibiosis, plant growth promotion and defense response (Fadel et al., 2015). α-cuprenene is a basic structure required for antimicrobial sesquiterpene quinone (Melillo et al., 2013) was found only in the co-culture medium. This might be responsible for the production of more terpenes in the co-culture medium.
Iturins are a group of antifungal, cyclic lipopeptides, produced by Bacillus (Meena and Kanwar, 2015). The iturin group of compound includes iturin A–E, bacillomycin D, F, and L, and mycosubtilin. These groups of compound contain seven α-amino acids (A1–A7) and one distinctive β-amino fatty acid (βAA). In the present investigation the alpha amino acids and amino fatty acids were detected in the B. amyloliquefaciens axenic culture and co-culture indicated the production of iturin like compounds. Kai et al. (2008) observed that dual culture system of bacteria with fungi and Arabidopsis thaliana emits volatiles and act as antibiotics. Similarly, in the present investigation, the co-culture system produced the new volatile compounds such as alkanes, alkenes, alcohols, esters, ketones, sulfur-containing compounds and terpenoids.
The heterocyclic compound, 1,2-Benzisothiazol-3(2H)-one was detected only in the co-culture. 1,2-Benzisothiazol-3(2H)-one play a major role in several pharmaceutical applications with broad spectrum bioactivity (Mahmoud et al., 2011; Lai et al., 2013). Amongst, appropriately substituted 1,2-benzisothiazol-3(2H)-ones have been appeared to show potent antifungal and antivirus activity (Dou et al., 2011; Tiew et al., 2012). The production of 1,2-Benzisothiazol-3(2H)-one in co-culture medium could be also involved in the improvement of antifungal and biocontrol activity of T. asperellum in respond to the B. amyloliquefaciens co-culture.
Proline plays a vital role in plant growth and development. It is essential for several cell wall proteins that play significant roles in plant growth such as cell wall signal transduction cascades, plant development and stress tolerance (Kavi Kishor et al., 2015). In the present investigation, the L-Proline, N-pivaloyl- ethyl ester was found in only co-culture system and this might be responsible for the highest seed germination, biocontrol and plant growth (Table 5 and Figure 7). Similarly, Wu et al. (2018) observed that the proline metabolism was induced in the co-culture of B. amyloliquefaciens ACCC11060 and T. asperellum using co-inoculation method.
The global biopesticide and biofertilizer market is constantly rising due to guidelines of agricultural legislations and regulations (Woo et al., 2014). The current research trend toward the development of biopesticide and biofertilizer focused toward the formulation of microbial consortia by mixing two separately grown microbial culture. Therefore, For the first time, in the present study the two different plant growth promoting and biological control agents were synergistically co-cultivated and optimized using the economic medium to reduce the production cost of two beneficial microbes at same time. Despite the different nature of two agriculturally important microbes, the co-cultivation of B. amyloliquefaciens 1841 and T. asperellum GDFS1009, stimulated different signaling pathways and enhanced the production of antagonistic and mycoparasitism related enzymes, secondary metabolites, and plant growth promoting compounds. Further, the greenhouse study confirmed that the biocontrol and plant growth was more effective in the plants treated with co-culture. The enhanced production of the biologically active compounds and plant growth recommended that this co-cultivation technology could be used as the next generation biopesticide and biofertilizer. Further, the scale-up of this co-cultivation technology in the fermentor and field study will facilitate the use in agriculture sector.
Author Contributions
VK, and JC conceived and designed the experiments and wrote the Manuscript. VK performed the experiments. VK, JS, TL, MV, and JC analyzed the data.
Funding
This work was supported by the National Key R&D Programs-Key International Intergovernmental Scientific and Technological Innovation Cooperation Projects (2017YFE0104900), National Key Research and Development Program of China (2017YFD0200403), National Natural Science Foundation of China (Nos. 31872015 and 31672072), and The Key Project of Science and Technology of Shanghai (No. 18391902400); CARS-02.
Conflict of Interest Statement
The authors declare that the research was conducted in the absence of any commercial or financial relationships that could be construed as a potential conflict of interest.
Supplementary Material
The Supplementary Material for this article can be found online at: https://www.frontiersin.org/articles/10.3389/fmicb.2019.01068/full#supplementary-material
References
Abdelmohsen, U. R., Grkovic, T., Balasubramanian, S., Kamel, M. S., Quinn, R. J., and Hentschel, U. (2015). Elicitation of secondary metabolism in actinomycetes. Biotechnol. Adv. 33, 798–811. doi: 10.1016/j.biotechadv.2015.06.003
Baiyee, B., Pornsuriya, C., Ito, S.-I., and Sunpapao, A. (2019). Trichoderma spirale T76-1 displays biocontrol activity against leaf spot on lettuce (Lactuca sativa L.) caused by Corynespora cassiicola or Curvularia aeria. Biol. Control 129, 195–200.
Bazafkan, H., Dattenböck, C., Stappler, E., Beier, S., and Schmoll, M. (2017). Interrelationships of VEL1 and ENV1 in light response and development in Trichoderma reesei. PLoS One 12:e0175946. doi: 10.1371/journal.pone.0175946
Benoit, I., Van Den Esker, M. H., Patyshakuliyeva, A., Mattern, D. J., Blei, F., Zhou, M., et al. (2015). Bacillus subtilis attachment to Aspergillus niger hyphae results in mutually altered metabolism. Environ. Microbiol. 17, 2099–2113. doi: 10.1111/1462-2920.12564
Brunner, K., Omann, M., Pucher, M. E., Delic, M., Lehner, S. M., Domnanich, P., et al. (2008). Trichoderma G protein-coupled receptors: functional characterisation of a cAMP receptor-like protein from Trichoderma atroviride. Curr. Genet. 54, 283–299. doi: 10.1007/s00294-008-0217-7
Brunner, K., Zeilinger, S., Ciliento, R., Woo, S. L., Lorito, M., Kubicek, C. P., et al. (2005). Improvement of the fungal biocontrol agent trichoderma atroviride to enhance both antagonism and induction of plant systemic disease resistance. Appl. Environ. Microbiol. 71, 3959–3965.
Carreras-Villasenor, N., Sanchez-Arreguin, J. A., and Herrera-Estrella, A. H. (2012). Trichoderma: sensing the environment for survival and dispersal. Microbiology 158, 3–16. doi: 10.1099/mic.0.052688-0
Casas-Flores, S., Rios-Momberg, M., Bibbins, M., Ponce-Noyola, P., and Herrera-Estrella, A. (2004). BLR-1 and BLR-2, key regulatory elements of photoconidiation and mycelial growth in trichoderma atroviride. Microbiology 150, 3561–3569.
Chet, I., Harel, M., and Viterbo, A. (2004). Isolation of two aspartyl proteases from Trichoderma asperellum expressed during colonization of cucumber roots ⋆. FEMS Microbiol. Lett. 238, 151–158.
Chettri, P., Calvo, A. M., Cary, J. W., Dhingra, S., Guo, Y., Mcdougala, R. L., et al. (2012). The veA gene of the pine needle pathogen Dothistroma septosporum regulates sporulation and secondary metabolism. Fungal Genet. Biol. 49, 141–151. doi: 10.1016/j.fgb.2011.11.009
Chowdhury, S. P., Hartmann, A., Gao, X., and Borriss, R. (2015). Biocontrol mechanism by root-associated Bacillus amyloliquefaciens FZB42 - a review. Front. Microbiol. 6:780. doi: 10.3389/fmicb.2015.00780
Collins, R. P., and Halim, A. F. (1972). Characterization of the major aroma constituent of the fungus Trichoderma viride. J. Agric. Food Chem. 20,437–438.
Contreras-Cornejo, H. A., Macias-Rodriguez, L., Del-Val, E., and Larsen, J. (2016). Ecological functions of Trichoderma spp. and their secondary metabolites in the rhizosphere: interactions with plants. FEMS Microbiol. Ecol. 92:fiw036. doi: 10.1093/femsec/fiw036
Copete-Pertuz, L. S., Alandete-Novoa, F., Placido, J., Correa-Londono, G. A., and Mora-Martinez, A. L. (2019). Enhancement of ligninolytic enzymes production and decolourising activity in Leptosphaerulina sp. by co-cultivation with Trichoderma viride and Aspergillus terreus. Sci. Total Environ. 646, 1536–1545. doi: 10.1016/j.scitotenv.2018.07.387
Davis, R. H. (2000). Neurospora: Contributions of a Model Organism. Oxford: Oxford University Press.
Delgado-Jarana, J., Sousa, S., Gonzalez, F., Rey, M., and Llobell, A. (2006). ThHog1 controls the hyperosmotic stress response in Trichoderma harzianum. Microbiology 152, 1687–1700.
Dou, D., Alex, D., Du, B., Tiew, K.-C., Aravapalli, S., Mandadapu, S. R., et al. (2011). Antifungal activity of a series of 1,2-benzisothiazol-3(2H)-one derivatives. Bioorg. Med. Chem. 19, 5782–5787. doi: 10.1016/j.bmc.2011.08.029
Fadel, H. H. M., Mahmoud, M. G., Asker, M. M. S., and Lotfy, S. N. (2015). Characterization and evaluation of coconut aroma produced by Trichoderma viride EMCC-107 in solid state fermentation on sugarcane bagasse. Electr. J. Biotechnol. 18, 5–9.
Freitas, M. A., Medeiros, F. H. V., Melo, I. S., Pereira, P. F., Peñaflor, M. F. G. V., Bento, J. M. S., et al. (2019). Stem inoculation with bacterial strains Bacillus amyloliquefaciens (GB03) and Microbacterium imperiale (MAIIF2a) mitigates fusarium root rot in cassava. Phytoparasitica 47, 135–142.
Glick, B. R. (2014). Bacteria with ACC deaminase can promote plant growth and help to feed the world. Microb. Res. 169, 30–39. doi: 10.1016/j.micres.2013.09.009
Goodson, J. R., Klupt, S., Zhang, C., Straight, P., and Winkler, W. C. (2017). LoaP is a broadly conserved antiterminator protein that regulates antibiotic gene clusters in Bacillus amyloliquefaciens. Nat. Microbiol. 2:17003. doi: 10.1038/nmicrobiol.2017.3
Hermosa, R., Rubio, M. B., Cardoza, R. E., Nicolas, C., Monte, E., and Gutierrez, S. (2013). The contribution of trichoderma to balancing the costs of plant growth and defense. Int. Microbiol. 16, 69–80.
Huang, J., Wei, Z., Tan, S., Mei, X., Shen, Q., and Xu, Y. (2014). Suppression of bacterial wilt of tomato by bioorganic fertilizer made from the antibacterial compound producing strain bacillus amyloliquefaciens HR62. J. Agric. Food Chem. 62, 10708–10716. doi: 10.1021/jf503136a
Kai, M., Vespermann, A., and Piechulla, B. (2008). The growth of fungi and Arabidopsis thaliana is influenced by bacterial volatiles. Plant Signal. Behav. 3, 482–484.
Karuppiah, V., Aarthi, C., Sivakumar, K., and Kannan, L. (2013). Statistical optimization and anticancer activity of a red pigment isolated from Streptomyces sp. PM4. Asian Pac. J. Trop. Biomed. 3, 650–656. doi: 10.1016/S2221-1691(13)60131-8
Kavi Kishor, P. B., Hima Kumari, P., Sunita, M. S. L., and Sreenivasulu, N. (2015). Role of proline in cell wall synthesis and plant development and its implications in plant ontogeny. Front. Plant Sci. 6:544. doi: 10.3389/fpls.2015.00544
Lai, H., Dou, D., Aravapalli, S., Teramoto, T., Lushington, G. H., Mwania, T. M., et al. (2013). Design, synthesis and characterization of novel 1,2-benzisothiazol-3(2H)-one and 1,3,4-oxadiazole hybrid derivatives: potent inhibitors of dengue and west nile virus NS2B/NS3 proteases. Bioorg. Med. Chem. 21, 102–113. doi: 10.1016/j.bmc.2012.10.058
Leylaie, S., and Zafari, D. (2018). Antiproliferative and antimicrobial activities of secondary metabolites and phylogenetic study of endophytic trichoderma species from vinca plants. Front. Microbiol. 9:1484. doi: 10.3389/fmicb.2018.01484
Lima, M. S., Damasio, A. R., Crnkovic, P. M., Pinto, M. R., Da Silva, A. M., Da Silva, J. C., et al. (2016). Co-cultivation of Aspergillus nidulans recombinant strains produces an enzymatic cocktail as alternative to alkaline sugarcane bagasse pretreatment. Front. Microbiol. 7:583. doi: 10.3389/fmicb.2016.00583
Lind, A. L., Wisecaver, J. H., Smith, T. D., Feng, X., Calvo, A. M., and Rokas, A. (2015). Examining the evolution of the regulatory circuit controlling secondary metabolism and development in the fungal genus aspergillus. PLoS Genet. 11:e1005096. doi: 10.1371/journal.pgen.1005096
Mahmoud, A. H., Mohamed Abouzid, K. A., El Ella, D. A. E. R. A., and Hamid Ismail, M. A. (2011). Optimization of benzoisothiazole dioxide inhibitory activity of the NS5B polymerase of HCV genotype 4 using ligand-steered homological modeling, reaction-driven scaffold-hopping and enovo workflow. Bioinformation 7, 328–333.
Marcello, C. M., Steindorff, A. S., Da Silva, S. P., Silva Rdo, N., Mendes Bataus, L. A., and Ulhoa, C. J. (2010). Expression analysis of the exo-beta-1,3-glucanase from the mycoparasitic fungus Trichoderma asperellum. Microbiol. Res. 165, 75–81. doi: 10.1016/j.micres.2008.08.002
Meena, K. R., and Kanwar, S. S. (2015). Lipopeptides as the antifungal and antibacterial agents: applications in food safety and therapeutics. BioMed Res. Int. 2015, 1–9. doi: 10.1155/2015/473050
Melillo, E., Setroikromo, R., Quax, W. J., and Kayser, O. (2013). Production of α-cuprenene in xanthophyllomyces dendrorhous: a step closer to a potent terpene biofactory. Microb. Cell Fact. 12:13. doi: 10.1186/1475-2859-12-13
Mendoza-Mendoza, A., Pozo, M. J., Grzegorski, D., Martinez, P., Garcia, J. M., Olmedo-Monfil, V., et al. (2003). Enhanced biocontrol activity of trichoderma through inactivation of a mitogen-activated protein kinase. Proc. Natl. Acad. Sci. U.S.A. 100, 15965–15970.
Montero-Barrientos, M., Hermosa, R., Cardoza, R. E., Gutiérrez, S., and Monte, E. (2011). Functional analysis of the Trichoderma harzianum nox1 gene, encoding an NADPH oxidase, relates production of reactive oxygen species to specific biocontrol activity against Pythium ultimum. Appl. Environ. Microbiol. 77, 3009–3016. doi: 10.1128/AEM.02486-10
Mota, M. S., Gomes, C. B., Souza Junior, I. T., and Moura, A. B. (2017). Bacterial selection for biological control of plant disease: criterion determination and validation. Braz. J. Microbiol. 48, 62–70. doi: 10.1016/j.bjm.2016.09.003
Mukherjee, P. K., and Kenerley, C. M. (2010). Regulation of morphogenesis and biocontrol properties in Trichoderma virens by a VELVET Protein, Vel1. Appl. Environ. Microbiol. 76, 2345–2352. doi: 10.1128/AEM.02391-09
Mukherjee, P. K., Latha, J., Hadar, R., and Horwitz, B. A. (2003). TmkA, a mitogen-activated protein kinase of trichoderma virens, is involved in biocontrol properties and repression of conidiation in the dark. Eukaryotic Cell 2, 446–455.
Netzker, T., Fischer, J., Weber, J., Mattern, D. J., Konig, C. C., Valiante, V., et al. (2015). Microbial communication leading to the activation of silent fungal secondary metabolite gene clusters. Front. Microbiol. 6:299. doi: 10.3389/fmicb.2015.00299
Omann, M., and Zeilinger, S. (2010). How a mycoparasite employs g-protein signaling: using the example of trichoderma. J. Signal. Transduct. 2010:123126. doi: 10.1155/2010/123126
Patil, A. S., Patil, S. R., and Paikrao, H. M. (2016). “Trichoderma Secondary Metabolites: Their Biochemistry and Possible Role in Disease Management,” in Microbial-Mediated Induced Systemic Resistance in Plants, eds D. K. Choudhary and A. Varma (Singapore: Springer Singapore), 69–102.
Pozo, M. J., Baek, J. M., Garcia, J. M., and Kenerley, C. M. (2004). Functional analysis of tvsp1, a serine protease-encoding gene in the biocontrol agent trichoderma virens. Fungal Genet. Biol. 41, 336–348.
Sarma, B. K., Yadav, S. K., Singh, S., and Singh, H. B. (2015). Microbial consortium-mediated plant defense against phytopathogens: readdressing for enhancing efficacy. Soil Biol. Biochem. 87, 25–33.
Srinivasa, N., Sriram, S., Singh, C., and Shivashankar, K. S. (2017). Secondary metabolites approach to study the bio-efficacy of Trichoderma asperellum isolates in india. Int. J. Curr. Microbiol. App. Sci. 6, 1105–1123.
Stockwell, V. O., Johnson, K. B., Sugar, D., and Loper, J. E. (2011). Mechanistically compatible mixtures of bacterial antagonists improve biological control of fire blight of pear. Phytopathology 101, 113–123. doi: 10.1094/PHYTO-03-10-0098
Tan, S., Gu, Y., Yang, C., Dong, Y., Mei, X., Shen, Q., et al. (2016). Bacillus amyloliquefaciens T-5 may prevent Ralstonia solanacearum infection through competitive exclusion. Biol. Fertil. Soils 52, 341–351.
Tiew, K.-C., Dou, D., Teramoto, T., Lai, H., Alliston, K. R., Lushington, G. H., et al. (2012). Inhibition of dengue virus and west nile virus proteases by click chemistry-derived benz[d]isothiazol-3(2H)-one derivatives. Bioorg. Med. Chem. 20, 1213–1221. doi: 10.1016/j.bmc.2011.12.047
Veenstra, A., Rafudeen, M. S., and Murray, S. L. (2019). Trichoderma asperellum isolated from african maize seed directly inhibits Fusarium verticillioides growth in vitro. Eur. J. Plant Pathol. 153, 279–283.
Viterbo, A., Landau, U., Kim, S., Chernin, L., and Chet, I. (2010). Characterization of ACC deaminase from the biocontrol and plant growth-promoting agent Trichoderma asperellum T203. FEMS Microbiol. Lett. 305, 42–48. doi: 10.1111/j.1574-6968.2010.01910.x
Wakefield, J., Hassan, H. M., Jaspars, M., Ebel, R., and Rateb, M. E. (2017). Dual induction of new microbial secondary metabolites by fungal bacterial co-cultivation. Front. Microbiol. 8:1284. doi: 10.3389/fmicb.2017.01284
Wang, M., Ma, J., Fan, L., Fu, K., Yu, C., Gao, J., et al. (2015). Biological control of southern corn leaf blight byTrichoderma atrovirideSG3403. Biocontrol Sci. Technol. 25, 1133–1146.
Wang, Y.-J., Wei, X.-Y., Jing, X.-Q., Chang, Y.-L., Hu, C.-H., Wang, X., et al. (2016). The fundamental role of NOX family proteins in plant immunity and their regulation. Int. J. Mol. Sci. 17:805. doi: 10.3390/ijms17060805
Woo, S. L., Ruocco, M., Vinale, F., Nigro, M., Marra, R., Lombardi, N., et al. (2014). Trichoderma-based products and their widespread use in agriculture. Open Mycol. J. 8, 71–126.
Wu, Q., Ni, M., Dou, K., Tang, J., Ren, J., Yu, C., et al. (2018). Co-culture of Bacillus amyloliquefaciens ACCC11060 and Trichoderma asperellum GDFS1009 enhanced pathogen-inhibition and amino acid yield. Microb. Cell Fact. 17:155. doi: 10.1186/s12934-018-1004-x
Wu, Q., Zhang, L., Xia, H., Yu, C., Dou, K., Li, Y., et al. (2017). Omics for understanding synergistic action of validamycin a and Trichoderma asperellum GDFS1009 against maize sheath blight pathogen. Sci. Rep. 7:40140. doi: 10.1038/srep40140
Xie, S., Jiang, H., Ding, T., Xu, Q., Chai, W., and Cheng, B. (2018). Bacillus amyloliquefaciens FZB42 represses plant miR846 to induce systemic resistance via a jasmonic acid-dependent signalling pathway. Mol. Plant Pathol. 19, 1612–1623. doi: 10.1111/mpp.12634
Yang, P. (2017). The gene task1 is involved in morphological development, mycoparasitism and antibiosis of Trichoderma asperellum. Biocontrol Sci. Technol. 27, 620–635.
Zeilinger, S., Gruber, S., Bansal, R., and Mukherjee, P. K. (2016). Secondary metabolism in trichoderma – chemistry meets genomics. Fungal Biol. Rev. 30, 74–90.
Keywords: co-cultivation, T. asperellum, B. amyloliquefaciens, plant growth, biocontrol
Citation: Karuppiah V, Sun J, Li T, Vallikkannu M and Chen J (2019) Co-cultivation of Trichoderma asperellum GDFS1009 and Bacillus amyloliquefaciens 1841 Causes Differential Gene Expression and Improvement in the Wheat Growth and Biocontrol Activity. Front. Microbiol. 10:1068. doi: 10.3389/fmicb.2019.01068
Received: 10 December 2018; Accepted: 29 April 2019;
Published: 16 May 2019.
Edited by:
Raffaella Balestrini, Institute for Sustainable Plant Protection, Italian National Research Council (IPSP-CNR), ItalyReviewed by:
Walter Chitarra, CREA Council for Agricultural Research and Economics, ItalyYun Chen, Zhejiang University, China
Copyright © 2019 Karuppiah, Sun, Li, Vallikkannu and Chen. This is an open-access article distributed under the terms of the Creative Commons Attribution License (CC BY). The use, distribution or reproduction in other forums is permitted, provided the original author(s) and the copyright owner(s) are credited and that the original publication in this journal is cited, in accordance with accepted academic practice. No use, distribution or reproduction is permitted which does not comply with these terms.
*Correspondence: Jie Chen, amllY2hlbjU5QHNqdHUuZWR1LmNu