- 1Darwin Bioprospecting Excellence S.L., Paterna, Spain
- 2ADM Biopolis S.L., Paterna, Spain
- 3Instituto de Agroquímica y Tecnología de Alimentos (IATA-CSIC), Paterna, Spain
- 4Institute for Integrative Systems Biology (I2SysBio), University of Valencia-CSIC, Paterna, Spain
- 5Department of Biochemistry and Molecular Biology, University of Valencia, Burjassot, Spain
Microbial communities that are exposed to sunlight typically share a series of adaptations to deal with the radiation they are exposed to, including efficient DNA repair systems, pigment production and protection against oxidative stress, which makes these environments good candidates for the search of novel antioxidant microorganisms. In this research project, we isolated potential antioxidant pigmented bacteria from a dry and highly-irradiated extreme environment: solar panels. High-throughput in vivo assays using Caenorhabditis elegans as an experimental model demonstrated the high antioxidant and ultraviolet-protection properties of these bacterial isolates that proved to be rich in carotenoids. Our results suggest that solar panels harbor a microbial community that includes strains with potential applications as antioxidants.
Introduction
Antioxidants are molecules that can protect cells against oxidative stress. For example, they can play a protective role against the biological damage derived from an excessive cellular production of reactive oxygen species (ROS). ROS are unstable metabolites of molecular oxygen (i.e., superoxide radical, hydroxyl radical, or hydrogen peroxide) that are constantly generated in the cells as by-products of normal aerobic metabolism, but whose levels can increase under certain stress situations (for example, alcohol consumption, smoking, or exposure to environmental pollutants) and become harmful for the cell (Al-Gubory, 2014; Rahal et al., 2014; Zorov et al., 2014; Chen et al., 2015). In humans, chronic oxidative stress has been associated on many occasions with the initiation and progression of a variety of diseases, including Alzheimer’s and cardiovascular diseases (such as hypertension and atherosclerosis) or cancer (Chen and Zhong, 2014; Milkovic et al., 2014; Dandekar et al., 2015; Siti et al., 2015).
The discovery of new antioxidants from natural sources (i.e., plants or microorganisms) is of high interest for the pharmacological and food industries (Finley et al., 2011; Lin et al., 2014). The search for novel natural molecules with biotechnological applications is known as bioprospecting and, in the past, microorganisms have proved to be rich sources of natural products that have been used for the fabrication of commercial products (antibiotics, probiotics, sustainable agriculture, fermentation processes, etc.) with a wide range of applications (Mahajan and Balachandran, 2012; Kanchiswamy et al., 2015; Katz and Baltz, 2016; Choudhary et al., 2017; Gupta and Bajaj, 2017). Microorganisms living in harsh environments typically exhibit strategies to cope with the environmental stresses they are exposed to. In the case of microbial communities exposed to sunlight (i.e., to radiation and desiccation), these adaptations include efficient DNA repair systems, pigment production and protection from oxidative stress (Lebre et al., 2017), suggesting that highly-irradiated environments may be good sources of novel antioxidant-producing microorganisms. In fact, tolerances to desiccation and radiation are mechanistically correlated (Mattimore and Battista, 1996; Ragon et al., 2011; Slade and Radman, 2011), particularly through protection strategies against protein oxidation (Fredrickson et al., 2008; Fagliarone et al., 2017). For these reasons, in the present research we selected a highly-irradiated environment as a potential source of antioxidant-producing microorganisms: solar panels. Solar panels are man-made structures that are exposed to desiccation and high amounts of solar radiation. These harsh conditions shape the surface-inhabiting microbiome toward a highly diverse microbial community with many drought-, heat-, and radiation-resistant bacteria (Dorado-Morales et al., 2016; Porcar et al., 2018; Tanner et al., 2018a). The cultivable microorganisms isolated from solar panels typically display red, orange, or yellow pigmentation, which is assumed to be linked to the production of carotenoids (CRTs), natural pigments that may play a role in the protection of these microorganisms against harmful ionizing radiation and oxidative stress (Britton, 1995; Sandmann, 2015; Dorado-Morales et al., 2016).
Taking into account the need of screening a large number of pigment-producing bacteria isolated from the solar panels, Caenorhabditis elegans was chosen as an experimental organism, as it is suitable for these high-throughput screenings. C. elegans is a nematode which has previously been used for testing potential antioxidant compounds such as selenite (Li et al., 2014), cocoa products (Martorell et al., 2013), tyrosol (Cañuelo et al., 2012), or CRTs such as astaxanthin (Yazaki et al., 2011) or β-carotene (Lashmanova et al., 2015). The use of C. elegans as an experimental model has many advantages, such as the low cost, simplicity, and quickness of the methods. Nevertheless, there is one more advantage that is of particular interest in this study: the fact that this nematode is naturally a bacteria eater, worms can directly be fed with selected bacterial strains. Laboratory C. elegans have a basal diet of Escherichia coli, but it is possible to supplement the growth medium with many ingredients of interest, including other bacteria, in order to analyze their biological activity. This functional screening method has previously been used in order to identify new antioxidant probiotic strains, such as Lactobacillus rhamnosus CNCM I-3690 strain (Grompone et al., 2012) or Bifidobacterium animalis subsp. lactis CECT 8145 strain (Martorell et al., 2016).
The research we present here aimed at establishing a collection of pigmented bacteria isolated from solar panels in order to select those with promising biological activities as antioxidants. For this, bacterial isolates with no record of opportunistic infections were subjected to a high-throughput antioxidant screening in C. elegans using the tracking device WMicrotracker-OneTM (PhylumTech, Santa Fé, Argentina), which uses photo-beam interruptions to assess movement of nematodes in multi-well plates. Specifically, the WMicrotracker-OneTM (WT) device was used to quantify the survival of the worms after the addition of hydrogen peroxide to the medium. Isolates with the highest antioxidant activity were then selected for further characterization through oxidative stress and UV-protection assays. Finally, a preliminary identification of the CRTs from the selected isolates was performed. This is the first study focused on bioprospecting the solar panel microbiome aiming at obtaining microorganisms with high potential as antioxidants.
Materials and Methods
Sampling
Samples were collected from six solar panels located on the rooftop of the Faculty of Economics of the University of Valencia on the 30th November 2015. Sampling was performed by washing the solar panels with sterile Phosphate-Buffered Saline (PBS) and by scraping the surface with sterile glass wipers as previously described (Dorado-Morales et al., 2016). The resulting liquid was collected using sterile pipettes and stored in 50 mL Falcon tubes, which were then transported to the laboratory on ice, where cultivation, isolation, and identification of the strains was performed.
Cultivation and Isolation of Pigmented Bacterial Strains
Solar panel samples were cultivated on Luria-Bertani medium (LB), Reasoner’s 2A (R2A) agar (Reasoner and Geldereich, 1985), and Marine Agar (MA) medium, by spreading 50 μL of the collected liquid to each plate. Then, samples were left to settle for 30 min, allowing the larger sized particles – including many fungi – to sediment, and 50 μL of the supernatant were plated on LB, R2A agar, and MA. By allowing the samples to settle, fungal growth was reduced when cultivating the samples on the different culture media. Plates were incubated at room temperature for 1 week and, after incubation, individual colonies were selected and isolated in pure culture by re-streaking on fresh medium. Morphological characteristics of the colonies (color, texture, and size) were taken into account in order to isolate as many different microorganisms as possible. The pure isolates were conserved at -80°C in 20% glycerol for future use.
16S rDNA Sequencing
For 16S rDNA sequencing, a 500-bp fragment of the hypervariable region V1-V3 of the isolates was amplified by colony PCR, using universal primers 28F (5′-GAG TTT GAT CNT GGC TCA G-3′) and 519R (5′-GTN TTA CNG CGG CKG CTG-3′). Isolates whose 16S rDNA failed to amplify from colony templates were amplified again with the same PCR program plus an initial step of incubation for 10 min at 100°C. Amplicons were checked in 1.4% agarose gel and then precipitated overnight in isopropanol 1:1 (vol:vol) and potassium acetate 3 M pH 5 1:10 (vol:vol). Precipitated DNA was washed with 70% ethanol, resuspended in Milli-Q water (Merck Millipore Ltd, Tullagreen, Cork, Ireland) and quantified with a Nanodrop-1000 Spectrophotometer (Thermo Fisher Scientific, Wilmington, DE, United States). Amplicons were tagged using BigDye®Terminator v3.1 Cycle Sequencing Kit (Applied Biosystems, Carlsbad, CA, United States) and sequenced with the Sanger method by the Sequencing Service (SCSIE) of the University of Valencia (Spain). The resulting sequences were manually edited using Pregap4 (Staden Package, 2002) to eliminate low-quality base calls. The EzBioCloud online tool (Yoon et al., 2017) was used to determine the closest neighbor with valid name for each isolate. The partial 16S rDNA sequence of the isolates was deposited in the GenBank/EMBL/DDBJ databases, under accession numbers MK621939-MK622006.
Oxidative Stress Assays With Worm Tracker
Experiments were carried out with the wild-type C. elegans strain N2 (Bristol), which was routinely propagated at 20°C on Nematode Growth Medium (NGM) plates supplemented with E. coli strain OP50 as the regular food source. Worms were synchronized by isolating eggs from gravid adults at 20°C. Synchronization was performed on NGM plates with E. coli OP50 as a negative control, E. coli OP50 plus vitamin C (vitC) at 20 μg/mL as a positive control (Supplementary Figure 2A), or E. coli OP50 plus the pigmented isolates in order to test antioxidant properties of the bacteria. The isolates were grown overnight in liquid LB medium at 28°C and 180 rpm, optical density at 600 nm (OD600) was adjusted to 30 and to 60, and 50 μL of the bacterial suspension was added to the plates. The synchronized worms were incubated for a total of 3 days on the previously described plates, until reaching young adult stage.
Young adult worms were collected and washed three times with M9 buffer, and finally resuspended in 100–200 μL of the buffer. Worms were then transferred by pipetting to 96-well plates (10–30 worms per well) containing M9 buffer. After transferring all the worms, hydrogen peroxide was added to the wells, reaching a final concentration of 1.2 mM of hydrogen peroxide (Supplementary Figure 2B). Mobility of the worms was measured with the WT device during 60 min (four measurements of 15 min). This device detects the movement of organisms through the interference they cause in an array of microbeams of infrared light (patented technology, #US12515723, EP208640881). In this experiment, data was collected in the form of “worm activity” (or relative locomotive activity), and was normalized by the number of worms in each well. All assays were performed with two biological replicates.
Manual Oxidative Stress Assays
Manual assays were also carried out with the wild-type C. elegans strain N2 (Bristol), routinely propagated and synchronized as previously described (on NGM with E. coli OP50 as a negative control, and supplemented with pigmented isolates at an OD600 of 30 for biological assays), except for the positive control, which in this case was vitC at 10 μg/mL. Young adult worms were transferred to fresh plates once every 2 days, until reaching 5-day adult stage. Then, these worms were transferred to plates containing basal medium supplemented with 2 mM hydrogen peroxide and incubated for 5 h at 20°C. After incubation, the survival rate of the worms for each condition (negative control, positive control and fed with pigmented bacteria) was calculated by manually assessing survival of the worms. Two biological replicates were performed for every condition.
UV-Protection Assays
Wild-type C. elegans strain N2 (Bristol) worms were synchronized on NGM plates with E. coli OP50 as a negative control, E. coli OP50 plus vitC (0.1 μg/mL) or plus chlorogenic acid (CGA) (0.1 μg/mL) as positive controls, or E. coli OP50 plus the pigmented isolates (50 μL of an over-night culture adjusted to OD 30) in order to test the UV light protection properties of the bacteria.
Synchronized worms were propagated for 15 days on the different types of medium, irradiated daily for 45 s in the laminar flow hood with UV light and transferred to new medium every 2 days, as previously described (Iriondo-DeHond et al., 2016). Survival rate of the worms was manually recorded every day and the assay was performed with biological duplicates.
Pigment Extraction
Carotenoid extraction was performed with two types of bacterial cultures: grown on solid (S) and in liquid (L) medium for 1 week and 12 h (overnight), respectively. For CRTs extraction from isolates grown on solid medium, bacterial cells were collected from solid LB medium after 1 week of incubation at room temperature. Cells were resuspended in PBS and concentrated through centrifugation at 13000 rpm for 3 min. The supernatant was discarded and pellets were dried completely with a vacuum-connected centrifuge (DNA Speed Vac, DNA120, Savant). Then, dry weight was determined. For the exponential phase samples, overnight cultures of selected isolates were collected and the wet weight was determined for each sample.
Bacterial pellets were resuspended and washed in Tris-Buffered Saline (TBS) solution, and centrifuged. Pelleted cells were frozen in liquid nitrogen (N2) three times, followed by addition of methanol (Sharlau, HPLC grade) (ten times the volume of the pellet) and sonication in a XUBA3 ultrasonic water bath (35 W; Grant Instruments, Cambridge, United Kingdom) for 5 min, in order to break the bacterial cells. Samples were vigorously shaken and centrifuged, and then the upper layer of colored methanol was transferred to a clean tube. This step was performed several times until a non-colored pellet was obtained.
Dicloromethane (HPLC grade) and water (Milli Q grade) (both at ten times the volume of the original pellet) were added to the methanol extract in order to separate organic and aqueous phases. Samples were vigorously shaken, centrifuged, and the aqueous phase was discarded. This step was performed twice, finally yielding CRT extracts in dicloromethane. Samples were then dried under N2 and kept at -20°C until analysis by HPLC-PDA. All steps were performed under dim light to avoid CRTs modifications such as photodegradation, isomerizations or structural changes.
HPLC-PDA Analysis
Carotenoid composition of each sample was analyzed by using an HPLC with a Waters liquid chromatography system (Waters, Barcelona, Spain) equipped with a 600E pump and a 2998 photodiode array detector (PDA). Empower software (Waters, Barcelona, Spain) was used for HPLC program set up and chromatogram analysis. A C30 CRT column (250 mm × 4.6 mm, 5 μm) coupled to a C30 guard column (20 mm × 4.0 mm, 5 μm) (YMC GmbH, Germany) was used. Samples were prepared for HPLC analysis by dissolving the CRT extracts in CHCl3:MeOH:acetone (3:2:1, v:v:v), followed by centrifugation for 2 min at 13000 rpm in order to discard any solid residues. CRT separation was performed with a ternary gradient elution, with an initial solvent composition of 90% methanol (MeOH), 5% water and 5% methyl tert-butyl ether (MTBE). Solvent composition changed during the analysis as described by Carmona et al. (2012) and Alquezar et al. (2008). After each analysis, the initial conditions were re-established and equilibrated before the next injection. The flow rate was 1 mL min-1 and column temperature was 25°C. A volume of 20 μL of each sample was injected and the PDA was set to scan from 250 to 540 nm. A Maxplot chromatogram was obtained for each sample that plots each CRT peak at its corresponding maximum absorbance wavelength.
Carotenoids were identified by comparison of the absorption spectra and retention times with the available standards or with data obtained in similar experimental conditions and described in the literature (Britton et al., 1998). For quantification, the chromatographic peaks of each CRT were integrated in their maximum wavelength and the resulting area of the peak was interpolated in different calibration curves that were already set up in the laboratory. The available calibration curves were: canthaxanthin (Sigma), lutein (Sigma), β-carotene (Sigma), β-cryptoxanthin (Extrasynthese). Standards of phytoene and phytofluene were obtained from peel extracts of orange fruits (Rodrigo et al., 2003) and HPLC purified. Quantification of adonirubin, astaxanthin, and echineone was performed using the calibration curve of β-carotene, with values expressed as equivalents of β-carotene. As for the non-identified CRTs, they were quantified using either the β-carotene or the lutein calibration curves depending on their retention times and spectra.
This article had been previously published as a preprint (Tanner et al., 2018b).
Results
Isolation of Pigmented Bacteria
Culturing of the solar panel samples yielded a high amount of colony-forming pigmented microorganisms on all three media (LB, R2A, and MA) as previously described (Dorado-Morales et al., 2016), although the isolates growing on LB media displayed more intense pigmentation. On the other hand, fungal growth was much lower on LB medium than on R2A or MA, facilitating the isolation of pure bacterial cultures from samples grown on LB medium rather than from the other two media. A total of 87 isolates were selected, obtained in pure culture, cryo-preserved in 20% glycerol and subjected to taxonomic identification through 16S rDNA sequencing, with 68 isolates being successfully identified and comprising a wide range of species belonging to the following genera: Agrococcus, Arthrobacter, Bacillus, Cellulosimicrobium, Curtobacterium, Frigoribacterium, Glutamicibacter, Kocuria, Leucobacter, Microbacterium, Pantoea, Paracoccus, Pedobacter, Planomicrobium, Plantibacter, Pontibacter, Pseudoclavibacter, Rhodobacter, Sanguibacter, and Sphingomonas (Supplementary Table 1).
Oxidative Stress Assays
After identification, 14 isolates with no record of opportunistic infections were selected for biological activity assays in C. elegans. For example, Erwinia persicina was not selected for these assays due to its capacity of infecting plants, causing chlorosis and necrosis in leaves (González et al., 2007). Isolates from the Kocuria genus were not selected due to increasing incidence of different types of Kocuria infection, mostly in immunocompromised hosts or hosts with severe underlying diseases, causing infections such as peritonitis, bacteremia or endocarditis (Purty et al., 2013). Finally, isolation from clinical specimens of bacteria from the genera Microbacterium, Cellulosimicrobium, and Curtobacterium have been reported, therefore isolates from these species were not selected for biological activity assays (Gneiding et al., 2008; Francis et al., 2011; Zamora and Camps, 2018).
The selected isolates for high-throughput biological assays were the following (Table 1): PS1 (Planomicrobium sp.), PS83 (Bacillus sp.), PS75 (Bacillus sp.), PS21 (Rhodobacter sp.), PS20 (Curtobacterium sp.), PS13 (Sanguibacter sp.), PS19 (Sanguibacter sp.), PS30 (Arthrobacter sp.), PS17 (Arthrobacter sp.), PS47 (Arthrobacter sp.), PS63 (Arthrobacter sp.), PS10 (Glutamicibacter sp.), PS66 (Agrococcus sp.) and PS57 (Sphingomonas sp.). All these isolates were individually tested with an oxidative stress assay using the WT device, which is able to automatically assess survival of the worms through the detection of omega bends and reversals in the worm’s locomotion (Huang et al., 2006). Survival under oxidative stress conditions was measured after incubation of the worms for 3 days on NGM supplemented with each bacterial isolate at OD600 of 30 or of 60. Isolate PS57 did not grow well in liquid culture and was therefore discarded from the assay. After 3 days of incubation with the selected pigmented isolates, some worms had not reached young adult phase and were de-synchronized. Specifically, this was the case of worms incubated with PS66, PS47, PS19, and PS20. The most extreme case was PS66, so this one was not measured in the WT device. Nevertheless, PS47, PS19, and PS20 displayed only slight differences in growth and were therefore tested. Worm activity after oxidative stress was best measured at 30 min after addition of hydrogen peroxide to the medium, as it is at this point when larger differences could be observed between the positive and negative controls (Figure 1).
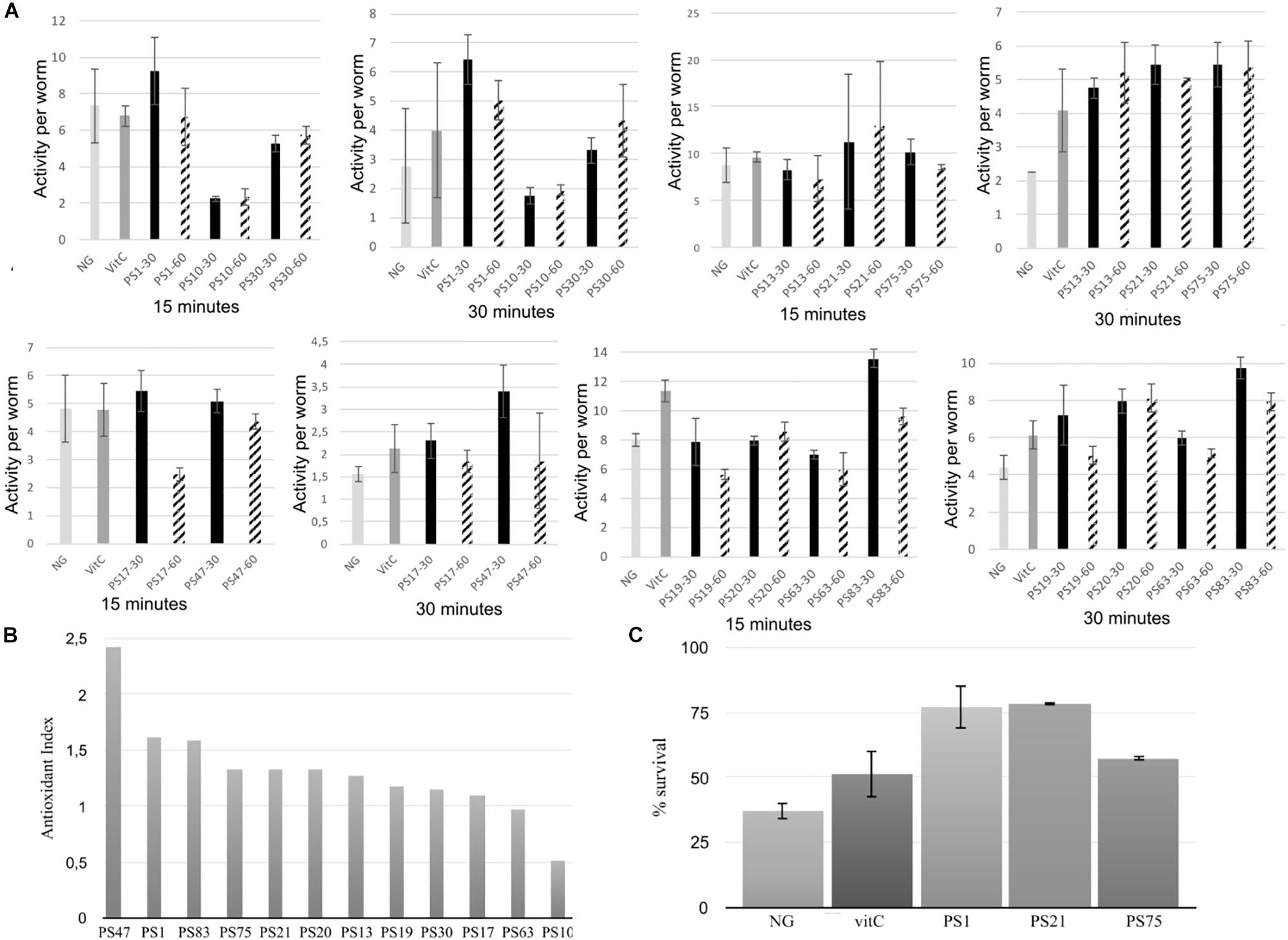
Figure 1. (A) Oxidative stress assays of the selected isolates using the WT device. Worms were fed with the selected isolates at either an OD600 of 30 or 60. Survival rate is represented in the Y-axis in the form of activity per worm, and results are shown after 15 and 30 min of incubation with hydrogen peroxide. Error bars indicate standard deviation. (B) Antioxidant index (AI) of the pigmented bacterial isolates from solar panels. AI was calculated by dividing the highest activity average (at an OD600 of 30 or 60) of each isolate by the average activity of the positive control (vitC) after 30 min of incubation with hydrogen peroxide. (C) Manual oxidative stress assay results. Y-axis indicates percentage of survival of the worms after 5 h of incubation in nematode growth medium supplemented with 20 mM hydrogen peroxide. NG (Nematode growth), negative control. VitC (vitamin C), positive control. Error bars indicate standard deviation.
In general, there was no significant differences in antioxidant activity between the worms incubated with the isolates at an OD of 30 or of 60 (Figure 1A), although a lower OD was beneficial for worm movement and, therefore, was the OD of choice for further experiments. After 30 min of incubation, PS30 did not display significant differences in activity per worm in comparison to the negative control, and PS10 displayed lower mobility than the negative control, indicating more worm mortality. On the other hand, incubation of the worms with PS1, PS13, PS21, PS75, PS17, PS47, PS19, PS20, PS63, and PS83 resulted in a higher protection of these worms against oxidative stress, with significant differences with respect to the negative control, and in some cases, with significantly higher protection in comparison to the positive control (Figure 1A). In order to compare all experiments, an antioxidant index (AI) was calculated for each isolate by dividing the average activity per worm at 30 min when incubated with the isolate at OD 30 or 60 (the highest activity was used) by the average activity per worm of the positive control (Figure 1B). Nine out of the ten tested isolates displayed higher antioxidant activity than the positive control (AI > 1), although three of these (PS47, PS19, and PS20) could not be compared to the rest due to the worms being smaller and, in some cases, not correctly synchronized.
The WT is a device that measures survival of the worms through their mobility, although this is not the most precise way to measure survival due to the fact that worms tend to have reduced mobility in liquid culture in comparison to solid medium. Therefore, the device may detect false negative results. For this reason, the best isolates according to results with the WT were selected for further, in depth characterization with the manual oxidative stress assay in order to confirm the results. Specifically, PS1, PS75, and PS21 were selected. For the manual assays, oxidative stress is applied to 5-day old adult worms instead of young adult worms, in accordance with the protocol described by Martorell et al. (2013).
Incubation with hydrogen peroxide resulted in a survival of approximately 37% of the worms grown on NGM with E. coli, whereas the survival of worms grown on NGM with E. coli supplemented with vitamin C (vitC) was higher, with approximately 51% survival (Figure 1C), confirming the antioxidant effect of the positive control (vitC). Furthermore, the selected isolates also displayed a high antioxidant effect: incubation with PS75 resulted in around 57% survival, whereas incubation with PS1 and PS21 resulted in a survival rate of as much as 78%. These results confirm that isolates PS1, PS21, and PS75 confer a very high protection against oxidative stress in C. elegans and, therefore, validate the WT protocol that was designed for this project.
UV-Protection Assays
The photo-protective effects of the isolated pigmented bacteria were tested in vivo in C. elegans using a UV-protection assay (Figure 2).
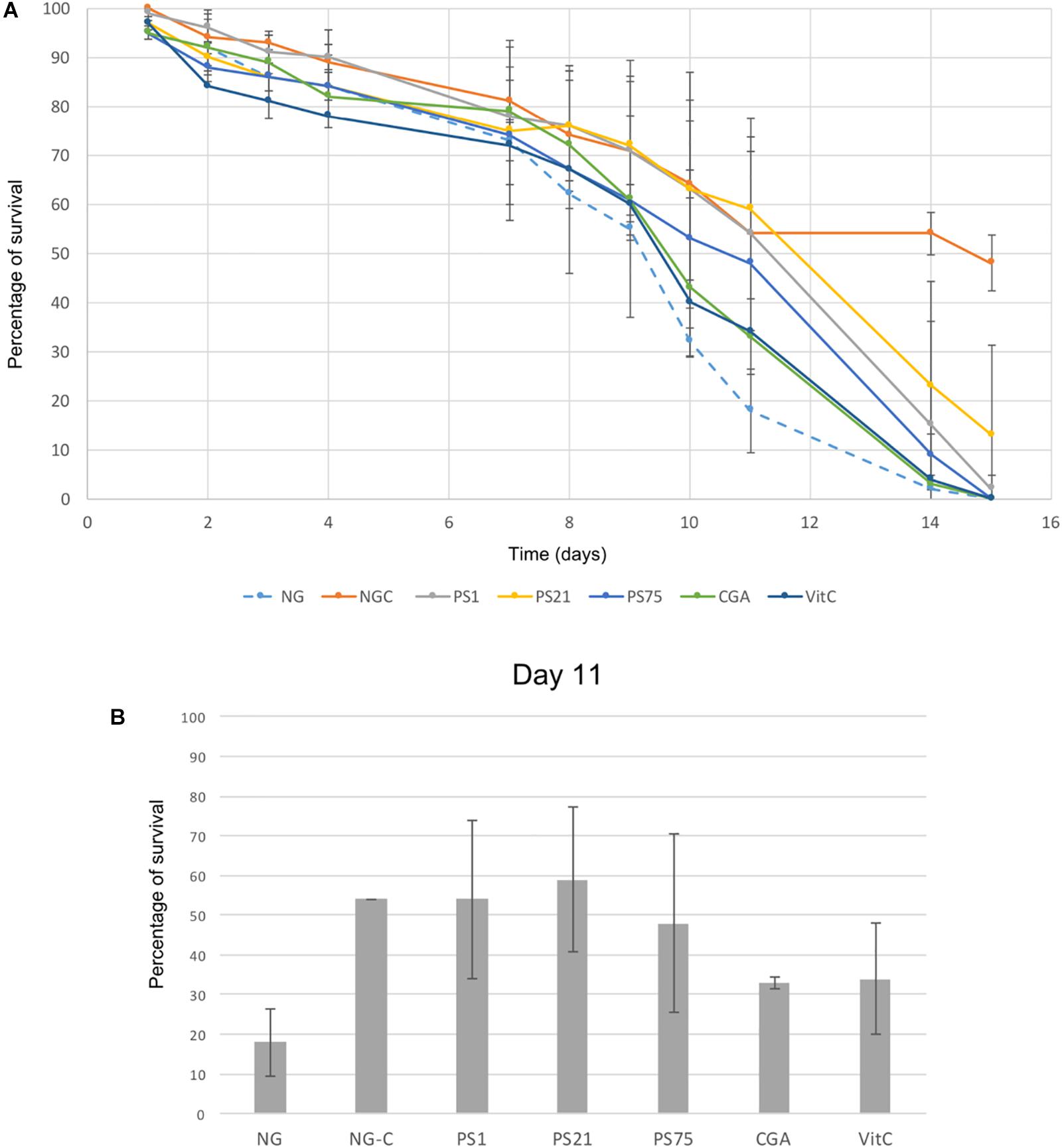
Figure 2. UV-light protection assay. Error bars indicate standard deviation. (A) Y-axis indicates percentage of survival of C. elegans irradiated with UV-light for 45 s every day over a period of 15 days (X-axis). NG-C indicates the non-irradiated controls: the basal survival rate of the worms over the 15-day period. NG refers to the negative control: worms incubated in NGM with no supplements and irradiated during the 15 days. CGA and VitC are two positive controls: worms incubated with antioxidant compounds (chlorogenic acid and vitamin C) and irradiated during 15 days. Finally, PS1, PS21, and PS75 (Planomicrobium sp., Rhodobacter sp., and Bacillus sp., respectively) indicate worms incubated with pigmented solar panel isolates and irradiated over the 15-day period in order to test the protective effect of these isolates against UV-light. (B) Results at day 11, in which the largest differences between the negative control and the worms fed with the pigmented isolates were observed.
There was a natural decrease in survival rate over time in the non-irradiated control (NG-C), with a survival rate at day 14 of 54% (Figure 2A). Despite a general decrease of survival rate over the first 9 days (Figure 2A), day 11 showed the largest decrease of the negative control survival rate (worms grown on NGM with E. coli and subjected to irradiation) in comparison to the survival rate of the positive controls and of the worms fed with the selected isolates (Figure 2B). Worms fed with PS1 and PS21 displayed a survival rate of around 55% at day 11, suggesting that these isolates are able to confer resistance against UV irradiation. On the other hand, although PS75 is also able to confer protection to UV-light, the survival rates are lower than the ones obtained with PS1 and PS21 (Figure 2A). These results correlate with the previous ones regarding effectiveness of the strains in protecting C. elegans against oxidative stress: PS1 and PS21 are the isolates which confer the highest resistance, followed by PS75.
Preliminary Characterization of the Carotenoid Content of Selected Isolates
The three selected isolates (PS1, PS21, and PS75) were further studied in two different types of bacterial culture: liquid culture and solid culture. For this, pigments were extracted and analyzed by HPLC-PDA. The resulting chromatogram of each sample, together with examples of characteristic absorption spectra for CRTs peaks can be seen in Supplementary Figure 1. For each sample, the peaks with a characteristic CRT spectrum were integrated at their maximum wavelength and, if possible, their probable identities were assigned according to the absorbance spectrum and retention time compared to commercial standards or reported in similar chromatographic conditions. Peaks with a characteristic CRT spectrum but without assigned identity were reported as “not identified” (NI) in the profile description. Peaks were quantified by interpolating the area of the peaks into calibration curves, as explained in Materials and Methods. The relative abundance of each carotenoid can be seen in Figure 3, and all details (identification, peaks, maximum wavelengths, numeric indication of the spectral shape, and quantification) of the CRTs tentatively identified (TI) of each sample can be found in Supplementary Table 2.
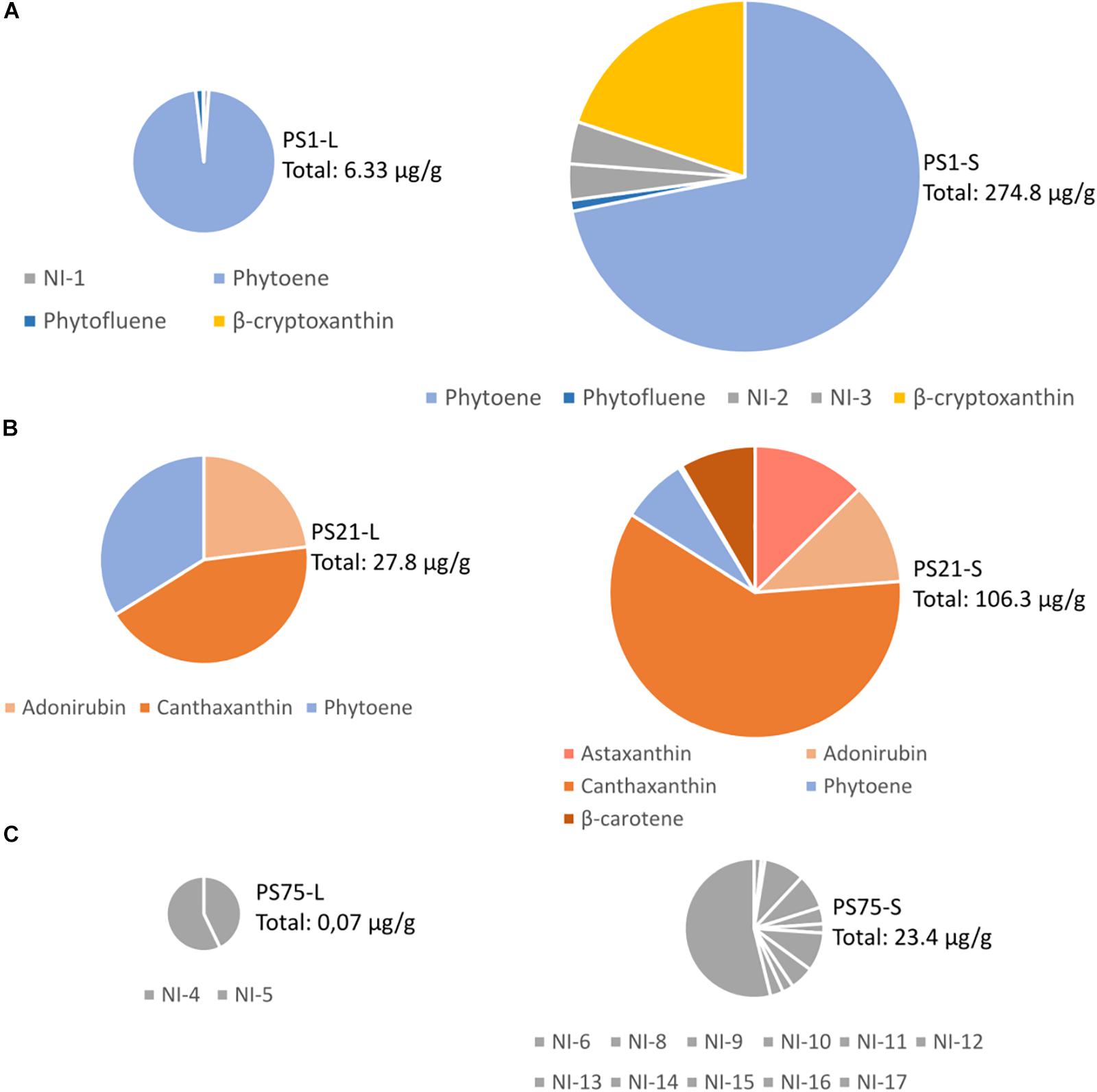
Figure 3. Tentative identification and quantification of the carotenoid content from the three selected isolates (A) PS1, (B) PS21, (C) PS75) after harvesting from liquid (L) or solid (S) culture. The total amount of CRTs is indicated next to each chart in μg per gram of cellular pellet (dry pellet in the samples harvested from solid culture, and wet pellet in the samples harvested from liquid culture). Further details on the concentration of each carotenoid can be found in Supplementary Table 2.
Discussion
Despite the harsh conditions, solar panels harbor a wide range of pigmented bacteria that are also shared by other harsh environments. Microbacterium radiodurans is a UV radiation-tolerant bacterium that was isolated for the first time from the upper sand layers of the Gobi desert in China (Zhang et al., 2010). Other isolates are characteristic of polar environments, such as Planomicrobium glaciei, a psychrotolerant bacterium that was first isolated from a glacier in China (Zhang et al., 2009), Arthrobacter agilis (Brambilla et al., 2001) or Sphingomonas aerolata (Busse et al., 2003); and others are characteristic of soil environments, such as P. agri (Roh et al., 2008) or many species of the Frigoribacterium (Kämpfer et al., 2000; Dastager et al., 2008), Arthrobacter (Park et al., 2014; Siddiqi et al., 2014) and Curtobacterium genera (Kim et al., 2008). Pigmentation of the bacterial isolates may play a protective role in their survival in environments with extreme temperature fluctuations and subjected to large amounts of irradiation. An intensification in the pigmentation was observed after the plates were incubated in the refrigerator for several days. In fact, previous studies suggest that pigments such as CRTs not only play an important role in radiation protection but also in cryoprotection (Dieser et al., 2010) due to their ability to modulate membrane fluidity in bacteria when grown under low temperature conditions (Jagannadham et al., 2000).
The diversity of genera found on the solar panel surfaces is consistent with other studies focusing on naturally irradiated environments. For example, several members of the Bacillus, Micrococcus, and Pseudomonas genera that proved to be resistant to UV-B irradiation have previously been isolated from high-altitude Andean wetlands, an environment that is characterized to have high UV radiation (Dib et al., 2008). On the other hand, UV-C resistant microorganisms including Arthrobacter sp. and Curtobacterium sp. have been isolated from sun-exposed rock varnish from the hot desert of the Whipple Mountains (Kuhlman et al., 2005). Furthermore, it is important to stress that 48 of the 68 isolates identified in the present study were Actinobacteria, a class that has been previously associated to UV-exposed environments including soil and high-altitude freshwater lakes (Warnecke et al., 2005; Rasuk et al., 2017; Bull et al., 2018).
Oxidative-stress assays with C. elegans revealed the antioxidant properties of these isolates, making them of great interest for the pharmacological and food industries: extracts of these isolates or even the bacteria themselves could be used as promising treatments for conditions in which oxidative stress plays an important role. On the other hand, the UV-protection assays suggest that the pigmented bacteria isolated from solar panels could also play a protecting role in this type of stress, which is of high interest for the cosmetic industry, specifically in the fabrication of products that protect against sunlight-induced skin damage. The three isolates selected for UV-light protection assays due to the promising results obtained in the oxidative-stress tests (Planomicrobium sp. or PS1, Rhodobacter sp. or PS21 and Bacillus sp. or PS75) were further tested through HPLC-PDA analysis to shed light on their CRTs composition.
PS1 was found to be 97,38% similar to Planomicrobium glaciei, a species that was first described by Zhang et al. (2009), who indicated that it displayed yellow-to-orange pigmentation. Our results suggest that the main CRTs present in PS1 may be phytoene and β-cryptoxanthin, and previous studies have demonstrated the antioxidant and free radical scavenging properties of β-cryptoxanthin, phytoene and phytofluene (Martínez et al., 2014; Ni et al., 2014). It would be interesting to consider whether the high antioxidant capacity of this isolate could be related to the presence of phytoene together with the colored CRT, β-cryptoxanthin.
PS21 was found to be 98.89% similar to Rhodobacter maris, a bacteria previously isolated from a marine habitat and described to produce CRTs (Ramana et al., 2008). Interestingly, although Paracoccus PS21 harvested from liquid culture was seen to be rich in pigments probably corresponding to adonirubin (TI), canthaxanthin, and phytoene, when harvested from solid medium CRT composition included also astaxanthin (T) and β-carotene. The CRTs present in PS1 and PS21 could be commercially valuable as they have many applications (Sandmann, 2015): β-carotene and canthaxanthin are used as food colorants and feed additives, especially in aquaculture, whereas astaxanthin and phytoene are widely used in the cosmetic industry.
Finally, the closest neighbor of PS75 was identified as B. megaterium (100% similarity), a spore-forming species (Mitchell et al., 1986). Although no identity was assigned to CRT peaks in PS75 extracts, the absorbance spectrum and retention time in the used chromatographic conditions of NI-17 and other minor peaks (NI-14 to -16) in solid culture, and NI-4 and 5 in liquid culture, are compatible with methyl esters of glycosyl-apo-8′-lycopene, orange colored derivatives of a C30 apo-8′-carotenoid pathway that occurs in certain Bacillus species (Pérez-Fons et al., 2011). Moreover, the NI-6 to NI-13 compounds and phytoene-like may also correspond to glycosyl-3-4-dehydro-8′-apolycopene esters and apo-8-phytoene which have been identified in vegetative cells and spores of Bacillus sp. species (Pérez-Fons et al., 2011). In relation to the oxidative stress and UV-resistant assays, this isolate had less antioxidant activity in comparison to PS1 and PS21.
Conclusion
In conclusion, after selecting a number of pigmented isolates from solar panels according to their low biological risk and testing them in vivo in order to elucidate their biological activity, nine out of the ten selected isolates displayed a higher antioxidant activity than the positive control. The isolates with highest antioxidant activity, PS1 (Planomicrobium sp.), PS21 (Rhodobacter sp.), and PS75 (Bacillus sp.) were validated with a manual oxidative stress assay, confirming the previous results and validating the protocol designed and used for oxidative stress assay in the WT device. Furthermore, the three selected strains also displayed UV-protection properties, with values once again higher than the positive control in the case of PS1 and PS21. The high antioxidant properties of these isolates are promising from a pharmacological point of view. Specifically, extracts of these bacteria or artificial combinations of their active compounds, could be useful for the design of new treatments against diseases in which oxidative stress plays a crucial role.
Taken together, our results provide new data on the biological activity of bacterial strains from solar panels with very high antioxidant and UV-protection properties. This is the first report describing the biotechnological potential of pigmented bacterial strains from solar panels using a C. elegans-based model.
Author Contributions
The project was designed by MP, JP, and DR. Sampling and isolation/identification of the strains was performed by MP, JP, and KT. Experiments involving C. elegans were performed by SG, PM, DR, and KT. Characterization of the pigment content was performed by MR, LZ, and KT. Manuscript has been written and revised by all the authors.
Funding
Financial support from the Spanish Government (grant Helios, reference: BIO2015-66960-C3-1-R co-financed by FEDER funds and Ministerio de Ciencia, Innovación y Universidades) and from the Regional Government of Valencia (grant MICROBIOSOL, reference: IFIDUA/2015/10 financed by IVACE) are acknowledged. Furthermore, KT is a recipient of a Doctorado Industrial fellowship from the Ministerio de Ciencia, Innovación y Universidades (Spain), with reference DI-16-08976. MR and LZ are members of the CaRed Excellence Network (BIO2017-90877-REDT) and EUROCAROTEN European COST Action (CA15113).
Conflict of Interest Statement
KT was employed at the time of research by the company Darwin Bioprospecting Excellence S.L., whereas JP and MP are co-founders of the company Darwin Bioprospecting Excellence S.L. PM, SG, and DR were employed by the company ADM Biopolis S.L.
The remaining authors declare that the research was conducted in the absence of any commercial or financial relationships that could be construed as a potential conflict of interest.
Acknowledgments
We are very grateful to Cristina Vilanova for her kind assistance in the taxonomic identification of the isolates.
Supplementary Material
The Supplementary Material for this article can be found online at: https://www.frontiersin.org/articles/10.3389/fmicb.2019.00986/full#supplementary-material
References
Al-Gubory, K. H. (2014). Environmental pollutants and lifestyle factors induce oxidative stress and poor prenatal development. Reprod. Biomed. 29, 17–31. doi: 10.1016/j.rbmo.2014.03.002
Alquezar, B., Rodrigo, M. J., and Zacarías, L. (2008). Regulation of carotenoid biosynthesis during fruit maturation in the red-fleshed orange mutant Cara cara. Phytochemistry 69, 1997–2007. doi: 10.1016/j.phytochem.2008.04.020
Brambilla, E., Hippe, H., Hagelstein, A., Tindal, B. J., and Stackerbrandt, E. (2001). 16S rRNA diversity of cultured and uncultured prokaryotes of a mat sample from Lake Fryxell, McMurdo Dry Valleys, Antarctica. Extremophiles 5, 23–33. doi: 10.1007/s007920000169
Britton, G. (1995). Structure and properties of carotenoids in relation to function. FASEB J. 9, 1551–1558. doi: 10.1096/fasebj.9.15.8529834
Britton, G., Liaaen-Jensen, S., and Pfander, H. (1998). Carotenoids: Biosynthesis and Metabolism, Vol. 3. Basel: Birkhäuser.
Bull, A. T., Idris, H., Sanderson, R., Asenjo, J., Andrews, B., and Goodfellow, M. (2018). High altitude, hyper-arid soils of the Central-Andes harbor mega-diverse communities of Actinobacteria. Extremophiles 22, 47–57. doi: 10.1007/s00792-017-0976-5
Busse, H. J., Denner, E. B., Buczolits, S., Salkinoja-Salonen, M., Bennasar, A., and Kämpfer, P. (2003). Sphingomonas aurantiaca sp. nov., Sphingomonas aerolata sp. nov. and Sphingomonas faeni sp. nov., air- and dustborne and Antarctic, orange-pigmented, psychrotolerant bacteria, and emended description of the genus Sphingomonas. Int. J. Syst. Evol. Microbiol. 53, 1253–1260. doi: 10.1099/ijs.0.02461-0
Cañuelo, A., Gilbert-López, B., Pacheco-Liñán, P., Martínez-Lara, E., Siles, E., and Miranda-Vizuete, A. (2012). Tyrosol, a main phenol present in extra virgin olive oil, increases lifespan and stress resistance in Caenorhabditis elegans. Mech. Ageing Dev. 133, 563–574. doi: 10.1016/j.mad.2012.07.004
Carmona, L., Zacarías, L., and Rodrigo, M. J. (2012). Stimulation of coloration and carotenoid biosynthesis during postharvest storage of ‘Navelina’ orange fruit at 12°C. Postharvest Biol. Technol. 74, 108–117. doi: 10.1016/j.postharvbio.2012.06.021
Chen, Z., Wang, D., Liu, X., Pei, W., Li, J., Cao, Y., et al. (2015). Oxidative DNA damage is involved in cigarette smoke-induced lung injury in rats. Environ. Health. Prev. Med. 20, 318–324. doi: 10.1007/s12199-015-0469-z
Chen, Z., and Zhong, C. (2014). Oxidative stress in Alzheimer’s disease. Neurosci. Bull. 30, 271–281.
Choudhary, J., Singh, S., and Nain, L. (2017). Bioprospecting thermotolerant ethanologenic yeasts for simultaneous saccharification and fermentation from diverse environments. J. Biosci. Bioeng. 123, 342–346. doi: 10.1016/j.jbiosc.2016.10.007
Dandekar, A., Mendez, R., and Zhang, K. (2015). “Cross talk between ER stress, oxidative stress, and inflammation in health and disease,” in Stress Responses. Methods in Molecular Biology, ed. C. Oslowski (New York, NY: Humana Press), 205–214. doi: 10.1007/978-1-4939-2522-3_15
Dastager, S. G., Lee, J. C., Ju, Y. J., Park, D. J., and Kim, C. J. (2008). Frigoribacterium mesophilum sp. nov., a mesophilic actinobacterium isolated from Bigeum Island, Korea. Int. J. Syst. Evol. Microbiol. 58, 1869–1872. doi: 10.1099/ijs.0.65637-0
Dib, J., Motok, J., Zenoff, V. F., Ordoñez, O., and Farías, M. E. (2008). Occurrence of resistance to antibiotics, UV-B, and arsenic in bacteria isolated from extreme environments in high-altitude (above 4400 m) Andean wetlands. Curr. Microbiol. 56, 510–517. doi: 10.1007/s00284-008-9103-2
Dieser, M., Greenwood, M., and Foreman, C. M. (2010). Carotenoid pigmentation in Antarctic heterotrophic bacteria as a strategy to withstand environmental stresses. Arct. Antarct. Alp. Res. 42, 396–405. doi: 10.1657/1938-4246-42.4.396
Dorado-Morales, P., Vilanova, C., Peretó, J., Codoñer, F. M., Ramón, D., and Porcar, M. (2016). A highly diverse, desert-like microbial biocenosis on solar panels in a Mediterranean city. Sci. Rep. 6, 29235. doi: 10.1038/srep29235
Fagliarone, C., Mosca, C., Ubaldi, I., Verseux, C., Baqué, M., Wilmotte, A., et al. (2017). Avoidance of protein oxidation correlates with the desiccation and radiation resistance of hot and cold desert strains of the cyanobacterium Chroococcidiopsis. Extremophiles 21, 981–991. doi: 10.1007/s00792-017-0957-8
Finley, J. W., Kong, A. N., Hintze, K. J., Jeffery, E. H., Ji, L. L., and Lei, X. G. (2011). Antioxidants in foods: state of the science important to the food industry. J. Agric. Food Chem. 59, 6837–6846. doi: 10.1021/jf2013875
Francis, M. J., Doherty, R. R., Patel, M., Hamblin, J. F., Ojaimi, S., and Korman, T. M. (2011). Curtobacterium flaccumfaciens septic arthritis following puncture with a Coxspur Hawthorn thorn. J. Clin. Microbiol. 49, 2759–2760. doi: 10.1128/JCM.00340-11
Fredrickson, J. K., Shu-mei, W. L., Gaidamakova, E. K., Matrosova, V. Y., Zhai, M., Sulloway, H. M., et al. (2008). Protein oxidation: key to bacterial desiccation resistance? ISME J. 2, 393–403. doi: 10.1038/ismej.2007.116
Gneiding, K., Frodl, R., and Funke, G. (2008). Identities of Microbacterium spp. encountered in human clinical specimens. J. Clin. Microbiol. 46, 3646–3652. doi: 10.1128/JCM.01202-08
González, A. J., Tello, J. C., and Rodicio, M. R. (2007). Erwinia persicina causing chlorosis and necrotic spots in leaves and tendrils of Pisum sativum in southeastern Spain. Plant Dis. 91:460. doi: 10.1094/PDIS-91-4-0460A
Grompone, G., Martorell, P., Llopis, S., González, N., Genovés, S., Mulet, A. P., et al. (2012). Anti-inflammatory Lactobacillus rhamnosus CNCM I-3690 strains protects against oxidative stress and increases lifespan in Caenorhabditis elegans. PLoS One 7:e52493. doi: 10.1371/journal.pone.0052493
Gupta, M., and Bajaj, B. K. (2017). Functional characterization of potential probiotic lactic acid bacteria isolated from Kalarei and development of probiotic fermented oat flour. Probiotics Antimicrob. Proteins 10, 654–661. doi: 10.1007/s12602-017-9306-6
Huang, K., Cosman, P., and Schafer, W. R. (2006). Machine vision based detection of omega bends and reversals in C. elegans. J. Neurosci. Methods 158, 323–336. doi: 10.1016/j.jneumeth.2006.06.007
Iriondo-DeHond, A., Martorell, P., Genovés, S., Ramón, D., Stamatakis, K., Fresno, M., et al. (2016). Coffee silverskin extract protects against accelerated aging caused by oxidative agents. Molecules 21:721. doi: 10.3390/molecules21060721
Jagannadham, M. V., Chattopadhyay, M. K., Subbalakshmi, C., Vairamani, M., Narayanan, K., Rao, C. M., et al. (2000). Carotenoids of an Antarctic psychrotolerant bacterium, Sphingomonas antarticus, and a mesophilic bacterium, Sphingobacterium multivorum. Arch. Microbiol. 173, 418–424. doi: 10.1007/s002030000163
Kämpfer, P., Rainey, F. A., Andersson, M. A., Nurmiaho, L. E. L., Ulrych, U., Busse, H. J., et al. (2000). Frigoribacterium faeni gen. nov., sp. nov., a novel psychrophilic genus of the family Microbacteriaceae. Int. J. Syst. Evol. Microbiol. 50, 355–363. doi: 10.1099/00207713-50-1-355
Kanchiswamy, C. N., Malnoy, M., and Maffei, M. E. (2015). Bioprospecting bacterial and fungal volatiles for sustainable agriculture. Trends Plant Sci. 20, 206–211. doi: 10.1016/j.tplants.2015.01.004
Katz, L., and Baltz, R. H. (2016). Natural product discovery: past, present and future. J. Ind. Microbiol. Biotechnol. 43, 155–176. doi: 10.1007/s10295-015-1723-5
Kim, M. K., Kim, Y. J., Kim, H. B., Kim, S. Y., Yi, T. H., and Yang, D. C. (2008). Curtobacterium ginsengisoli sp. nov., isolated from soil of a ginseng field. Int. J. Syst. Evol. Microbiol. 58, 2393–2397. doi: 10.1099/ijs.0.65591-0
Kuhlman, K. R., Allenbach, L. B., Ball, C. L., Fusco, W. G., La Duc, M. T., Kuhlman, G. M., et al. (2005). Enumeration, isolation, and characterization of ultraviolet (UV-C) resistant bacteria from rock varnish in the Whipple Mountains, California. Icarus 174, 585–595. doi: 10.1016/j.icarus.2004.11.022
Lashmanova, E., Proshkina, E., Zhikrivetskaya, S., Shevchenko, O., Marusich, E., Leonov, S., et al. (2015). Fucoxanthin increases lifespan of Drosophila melanogaster and Caenorhabditis elegans. Pharmacol. Res. 100, 228–241. doi: 10.1016/j.phrs.2015.08.009
Lebre, P. H., De Maayer, P., and Cowan, D. A. (2017). Xerotolerant bacteria: surviving through a dry spell. Nat. Rev. Microbiol. 15, 285–296. doi: 10.1038/nrmicro.2017.16
Li, W. H., Shi, Y. C., Chang, C. H., Huang, C. W., and Hsiu-Chuan Liao, V. (2014). Selenite protects Caenorhabditis elegans from oxidative stress via DAF-16 and TRXR-1. Mol. Nutr. Food Res. 58, 863–874. doi: 10.1002/mnfr.201300404
Lin, Y., Jain, R., and Yan, Y. (2014). Microbial production of antioxidant food ingredients via metabolic engineering. Curr. Opin. Biotechnol. 26, 71–78. doi: 10.1016/j.copbio.2013.10.004
Mahajan, G. B., and Balachandran, L. (2012). Antibacterial agents from actinomycetes – a review. Front. Biosci. 4:240–253. doi: 10.2741/e373
Martínez, A., Stinco, C. M., and Meléndez-Martínez, A. J. (2014). Free radical scavenging properties of phytofluene and phytoene isomers as compared to lycopene: a combined experimental and theoretical study. J. Phys. Chem. 118, 9819–9825. doi: 10.1021/jp503227j
Martorell, P., Bataller, E., Llopis, S., Gonzalez, N., Álvarez, B., Montón, F., et al. (2013). A cocoa peptide protects Caenorhabditis elegans from oxidative stress and β-amyloid peptide toxicity. PLoS One 8:e63283. doi: 10.1371/journal.pone.0063283
Martorell, P., Llopis, S., González, N., Chenoll, E., López-Carreras, N., Aleixandre, A., et al. (2016). Probiotic strain Bifidobacterium animalis subsp. lactis CECT 8145 reduces fat content and modulates lipid metabolism and antioxidant response in Caenorhabditis elegans. J. Agric. Food Chem. 64, 3462–3472. doi: 10.1021/acs.jafc.5b05934
Mattimore, V., and Battista, J. R. (1996). Radioresistance of Deinococcus radiodurans: functions necessary to survive ionizing radiation are also necessary to survive prolonged desiccation. J. Bacteriol. 178, 633–637. doi: 10.1128/jb.178.3.633-637.1996
Milkovic, L., Siems, W., Siems, R., and Zarkovic, N. (2014). Oxidative stress and antioxidants in carcinogenesis and integrative therapy of cancer. Curr. Pharm. Des. 20, 6529–6542. doi: 10.2174/1381612820666140826152822
Mitchell, C., Lyer, S., Skomurski, J. F., and Vary, J. C. (1986). Red pigment in Bacillus megaterium spores. Appl. Environ. Microbiol. 52, 64–67.
Ni, Y., Nagashimada, M., Zhan, L., Nagata, N., Kobori, M., Sugiura, M., et al. (2014). Prevention and reversal of lipotoxicity-induced hepatic insulin resistance and steatohepatitis in mice by an antioxidant carotenoid, β-cryptoxanthin. Endocrinology 156, 987–999. doi: 10.1210/en.2014-1776
Park, Y., Kook, M., Ngo, H. T., Kim, K. Y., Park, S. Y., Mavlonov, G. T., et al. (2014). Arthrobacter bambusae sp. nov., isolated from soil of a bamboo grove. Int. J. Syst. Evol. Microbiol. 64, 3069–3074. doi: 10.1099/ijs.0.064550-0
Pérez-Fons, L., Steiger, S., Khaneja, R., Bramley, P. M., Cutting, S. M., Sandmann, G., et al. (2011). Identification and the developmental formation of carotenoid pigments in the yellow/orange Bacillus spore-formers. Biochem. Biophys. Acta 1811, 177–185. doi: 10.1016/j.bbalip.2010.12.009
Porcar, M., Louie, K. B., Kosina, S. M., Van Goethem, M. W., Bowen, B. P., Tanner, K., et al. (2018). Microbial ecology on solar panels in Berkeley, CA, United States. Front. Microbiol. 9:3043. doi: 10.3389/fmicb.2018.03043
Purty, S., Saranathan, R., Prashanth, K., Narayanan, K., Asir, J., Devi, C. S., et al. (2013). The expanding spectrum of human infections caused by Kocuria species: a case report and literature review. Emerg. Microbes Infect. 2:e71.
Ragon, M., Restoux, G., Moreira, D., Møller, A. P., and López-García, P. (2011). Sunlight-exposed biofilm microbial communities are naturally resistant to chernobyl ionizing-radiation levels. PLoS One 6:e21764. doi: 10.1371/journal.pone.0021764
Rahal, A., Kumar, A., Singh, V., Yadav, B., Tiwari, R., Charkraborty, S., et al. (2014). Oxidative stress, prooxidants and antioxidants: the interplay. Biomed. Res. Int. 2014:761264. doi: 10.1155/2014/761264
Ramana, V. V., Sasikala, C., and Ramana, C. V. (2008). Rhodobacter maris sp. nov., a phototrophic alphaproteobacterium isolated from a marine habitat of India. Int. J. Syst. Evol. Microbiol. 58, 1719–1722. doi: 10.1099/ijs.0.65638-0
Rasuk, M. C., Ferrer, G. M., Kurth, D., Portero, L. R., Farías, M. E., and Albarracín, V. H. (2017). UV-resistant Actinobacteria from high-altitude Andean lakes: isolation, characterization and antagonistic activities. Photochem. Photobiol. 93, 865–880. doi: 10.1111/php.12759
Reasoner, D. J., and Geldereich, E. E. (1985). A new medium for the enumeration and subculture of bacteria from potable water. Appl. Environ. Microbiol. 49, 1–7.
Rodrigo, M., Marcos, J., Alferez, F., Mallent, M. D., and Zacarias, L. (2003). Characterization of Pinalate, a novel Citrus sinensis mutant with a fruit-specific alteration that results in yellow pigmentation and decreased ABA content. J. Exp. Bot. 54, 727–738. doi: 10.1093/jxb/erg083
Roh, S. W., Quan, Z. X., Nam, Y. D., Chang, H. W., Kim, K. H., Kim, M. K., et al. (2008). Pedobacter agri sp. nov., from soil. Int. J. Syst. Evol. Microbiol. 58, 1640–1643. doi: 10.1099/ijs.0.65783-0
Sandmann, G. (2015). Carotenoids of biotechnological importance. Adv. Biochem. Eng. Biotechnol. 148, 449–467. doi: 10.1007/10_2014_277
Siddiqi, M. Z., Kim, Y. J., Hoang, V. A., Siddiqi, M. H., Huq, M. A., and Yang, D. C. (2014). Arthrobacter ginsengisoli sp. nov., isolated from soil of a ginseng field. Arch. Microbiol. 496, 863–870. doi: 10.1007/s00203-014-1025-8
Siti, H. N., Kamisah, Y., and Kamsiah, J. (2015). The role of oxidative stress, antioxidants and vascular inflammation in cardiovascular disease (a review). Vascul. Pharmacol. 71, 40–56. doi: 10.1016/j.vph.2015.03.005
Slade, D., and Radman, M. (2011). Oxidative stress resistance in Deinococcus radiodurans. Microbiol. Mol. Biol. Rev. 75, 133–191. doi: 10.1128/MMBR.00015-10
Tanner, K., Martí, J. M., Belliure, J., Fernández-Méndez, M., Molina-Menor, E., Peretó, J., et al. (2018a). Polar solar panels: arctic and Antarctic microbiomes display similar taxonomic profiles. Environ. Microbiol. Rep. 10, 75–79. doi: 10.1111/1758-2229.12608
Tanner, K., Martorell, P., Genovés, S., Ramón, D., Zacarías, L., Rodrigo, M. J., et al. (2018b). Bioprospecting the solar panel microbiome: high-throughput screening for antioxidant bacteria in a Caenorhabditis elegans model. bioRxiv [Preprint]. doi: 10.1101/423731
Warnecke, F., Sommaruga, R., Sekar, R., Hofer, J. S., and Pernthaler, J. (2005). Abundances, identity, and growth state of Actinobacteria in mountain lakes of different UV transparency. Appl. Environ. Microbiol. 71, 5551–5559. doi: 10.1128/aem.71.9.5551-5559.2005
Yazaki, K., Yoshikoshi, C., Oshiro, S., and Yanase, S. (2011). Supplemental cellular protection by a carotenoid extends lifespan via Ins/IGF-1 signaling in Caenorhabditis elegans. Oxid. Med. Cell. Longev. 2011:596240. doi: 10.1155/2011/596240
Yoon, S. H., Ha, S. M., Kwon, S., Lim, J., Kim, Y., Seo, H., et al. (2017). Introducing EzBioCloud: a taxonomically united database of 16S rRNA and whole genome assemblies. Int. J. Syst. Evol. Microbiol. 67, 1613–1617. doi: 10.1099/ijsem.0.001755
Zamora, J. A. G., and Camps, N. (2018). Bacteremia caused by cellulosimicrobium in a bone marrow transplant patient: a case report and literature review. IDCases 11, 64–66. doi: 10.1016/j.idcr.2018.01.007
Zhang, D. C., Liu, H. C., Xin, Y. H., Yu, Y., Zhou, P. J., and Zhou, Y. G. (2009). Planomicrobium glaciei sp. nov., a Psychrotolerant bacterium isolated from a glacier. Int. J. Syst. Evol. Microbiol. 59, 1387–1390. doi: 10.1099/ijs.0.002592-0
Zhang, W., Zhu, H. H., Yuan, M., Yao, Q., Tang, R., Lin, M., et al. (2010). Microbacterium radiodurans sp. nov., a UV radiation-resistant bacterium isolated from soil. Int. J. Syst. Evol. Microbiol. 60(Pt 11), 2665–2670. doi: 10.1099/ijs.0.017400-0
Keywords: microbiome, sun-exposed environment, bioprospecting, antioxidant, Caenorhabditis elegans
Citation: Tanner K, Martorell P, Genovés S, Ramón D, Zacarías L, Rodrigo MJ, Peretó J and Porcar M (2019) Bioprospecting the Solar Panel Microbiome: High-Throughput Screening for Antioxidant Bacteria in a Caenorhabditis elegans Model. Front. Microbiol. 10:986. doi: 10.3389/fmicb.2019.00986
Received: 20 December 2018; Accepted: 18 April 2019;
Published: 07 May 2019.
Edited by:
Davide Zannoni, University of Bologna, ItalyReviewed by:
Virginia Helena Albarracín, Center for Electron Microscopy (CIME), ArgentinaBirinchi Kumar Sarma, Banaras Hindu University, India
Copyright © 2019 Tanner, Martorell, Genovés, Ramón, Zacarías, Rodrigo, Peretó and Porcar. This is an open-access article distributed under the terms of the Creative Commons Attribution License (CC BY). The use, distribution or reproduction in other forums is permitted, provided the original author(s) and the copyright owner(s) are credited and that the original publication in this journal is cited, in accordance with accepted academic practice. No use, distribution or reproduction is permitted which does not comply with these terms.
*Correspondence: Manuel Porcar, bWFudWVsLnBvcmNhckB1di5lcw==