- Department of Biological Sciences and Bioinformatics, Myongji University, Yongin, South Korea
A defining characteristic of Gram-negative bacteria is the presence of an outer membrane, which functions as an additional barrier inhibiting the penetration of toxic chemicals, such as antibiotics. Porins are outer membrane proteins associated with the modulation of cellular permeability and antibiotic resistance. Although there are numerous studies regarding porins, a systematic approach about the roles of porins in bacterial physiology and antibiotic resistance does not exist yet. In this study, we constructed mutants of all porins in Escherichia coli and examined the effect of porins on antibiotic resistance and membrane integrity. The OmpF-defective mutant was resistant to several antibiotics including β-lactams, suggesting that OmpF functions as the main route of outer membrane penetration for many antibiotics. In contrast, OmpA was strongly associated with the maintenance of membrane integrity, which resulted in the increased susceptibility of the ompA mutant to many antibiotics. Notably, OmpC was involved in both the roles. Additionally, our systematic analyses revealed that other porins were not involved in the maintenance of membrane integrity, but several porins played a major or minor role in the outer membrane penetration for a few antibiotics. Collectively, these results show that each porin plays a distinct role in antibiotic resistance and membrane integrity, which could improve our understanding of the physiological function and clinical importance of porins.
Introduction
The outer membrane (OM) of Gram-negative bacteria is a unique architecture which is composed of phospholipids, lipopolysaccharides (LPS), lipoproteins, and β-barrel porins (Henderson et al., 2016). The OM functions as an additional barrier for blocking the transport of toxic compounds, such as bile acid and antibiotics (O’Shea and Moser, 2008; Pages et al., 2008). Therefore, chemicals with a molecular weight of more than 600 Da cannot generally penetrate the envelope of the Gram-negative bacteria (O’Shea and Moser, 2008). Therefore, antibiotics with a molecular weight more than 1400 Da, such as vancomycin and daptomycin, cannot pass through the OM of Gram-negative bacteria. This feature of the OM is one of the main obstacles in the development of a novel antimicrobial agent targeting the Gram-negative pathogens (Lee et al., 2013).
OM porins are transmembrane pore-forming proteins with a β-barrel structure, which forms a water-filled open channel and allows the passive transport of hydrophilic compounds (Schulz, 2002; Nikaido, 2003; Pages et al., 2008). The porins are the most abundant proteins of the OM in Gram-negative bacteria, with various existing types. They can be classified as non-specific or specific porins based on their activity. Additionally, they are classified into monomeric, dimeric, or trimeric porins based on their functional structure (Koebnik et al., 2000; Pages et al., 2008). In addition to the passive transport of various molecules, porins seem to play an important role in maintaining the envelope integrity of the Gram-negative bacteria. For example, outer membrane protein A (OmpA) is a non-specific porin which allows the passive transport of many small chemicals (Sugawara and Nikaido, 1992; Iyer et al., 2018). It is also a peptidoglycan-associated protein with a flexible periplasmic domain that is involved in the non-covalent interaction with peptidoglycan (Samsudin et al., 2016). Because porins mediate the passive diffusion of antibiotics across the OM, they are closely associated with antibiotic resistance in the Gram-negative bacteria. For example, β-lactams and fluoroquinolones were known to penetrate the OM through the non-specific porin OmpF (Mach et al., 2008; Delcour, 2009). Therefore, the ompF mutant was resistant to several β-lactam antibiotics in some Gram-negative pathogens, including Escherichia coli (Nikaido et al., 1983; Ziervogel and Roux, 2013), Klebsiella pneumoniae (Sugawara et al., 2016), Serratia marcescens (Moya-Torres et al., 2014), Pseudomonas aeruginosa (Okamoto et al., 2001), and Enterobacter aerogenes (Bornet et al., 2000). On the other hand, the deletion of OmpA resulted in increased susceptibility to several antibiotics including β-lactams in Acinetobacter baumannii (Smani et al., 2014). This result may be caused by the effect of OmpA on the maintenance of membrane integrity, because impaired membrane integrity can increase the intracellular diffusion of antibiotics. Although these results suggest that porins affect antibiotic resistance in different ways, a systematic analysis of the relationship between porins and antibiotic resistance has not been performed yet. In particular, the role of specific porins in antibiotic resistance remains unclear.
In this study, we analyzed how all porins of E. coli affect the resistance to various antibiotics of different classes, and the maintenance of membrane integrity. These analyses showed that porins can be classified into three groups according to their roles in antibiotic transport and membrane integrity: antibiotic transport-related specific porins (LamB, YddB, etc.), membrane integrity-related non-specific porin (OmpA), and non-specific porins involved in both antibiotic transport and membrane integrity (OmpC and OmpF). OmpF and OmpA play a major role in antibiotic transport and the maintenance of membrane integrity, respectively. OmpC is important for both of the two functions. These results suggest the functional diversity of porins and explain why the effect of porins on antibiotic resistance is diverse depending on the kind of porin.
Materials and Methods
Bacterial Strains, Plasmids, and Culture Conditions
The bacterial strains and plasmids used in this study are listed in Supplementary Table S1. Bacterial cells were grown as described previously (Lee et al., 2015; Choi et al., 2016). Unless otherwise indicated, Luria-Bertani broth (LB broth) was used for the general growth of cells. The antibiotics kanamycin (Kan) and chloramphenicol (Cm) were used at concentrations of 50 μg/ml and 5 μg/ml, respectively. All porin deletion mutants were constructed by replacing the entire or partial open reading frame region of a target gene with the kanamycin-resistance gene using the λ red recombinase as described previously (Datsenko and Wanner, 2000). The kanamycin-resistance gene was amplified from pKD13 using the primer sets listed in Supplementary Table S2. The purified PCR product was electroporated into MG1655 cells harboring a pKD46 plasmid, and the deletion mutant was selected on LB plates with kanamycin at 37°C. The kanamycin-resistance gene was removed by using a pCP20 plasmid expressing the FLP recombinase as described previously (Datsenko and Wanner, 2000).
The ompAΔC mutant was constructed by replacing theregion between 197th codon and the stop codon of the ompA gene with the kanamycin-resistance gene containing its promoter region. The kanamycin-resistance gene was amplified using a forward primer with a synthetic linker (underlined) and FLAG-tag (in boldface type), 5′-ACGGCATGCTGAGCCTGGGTGTTTCCTACCGTTTCGGTC AGGGCGAAGCAGGCAGCGGC GACTACAAAGACGATGAC GACAAGTAGCTTAGACGTCAGGTGGCACT-3′ and a reverse primer, 5′-AAAGGCAAAAAAAACCCCGCAGCAGCGGG GTTTTTCTACCAGACGAGAACACGCTCAGTGGAACGAA AAC-3′. The purified PCR product was electroporated into MG1655 cells harboring the pKD46 plasmid, and the deletion mutant was selected on LB plates with kanamycin at 37°C.
Determination of Minimal Inhibitory Concentration (MIC) Values
The MIC values were examined on agar plates with the agar dilution method according to the Clinical Laboratory Standards Institute guidelines (Wikler and CLSI, 2018). The Müller-Hinton agar (Difco, United States) plates were prepared by adding antimicrobial agents in two-fold serial dilutions, resulting in plates containing final concentrations of 512 μg/ml to 7.8 ng/ml. E. coli cells were grown in the Mueller-Hinton broth to a McFarland turbidity standard of 0.5 (approximately 1.5 × 108 cells/ml). Cultures were diluted 10-fold with Mueller-Hinton broth to reach a final concentration of 107 cells/ml. A 10 μl volume was spotted on the plates. After incubation at 37°C for 20 h, the MIC value was determined. The MIC is defined as the lowest concentration of an antibiotic preventing the lawn growth of cells.
Measurement of Bacterial Growth
Stationary phase cells grown in LB medium were serially diluted from 108 to 104 cells/ml in 10-fold dilutions. Aliquots of 2 μl were spotted onto LB plates supplemented with 2% SDS, 6% ethanol, 750 mM NaCl, 64 μg/ml vancomycin, or 20 μg/ml chlorophenyl red-β-d-galactopyranoside (CPRG). To determine the effect of the C-terminal domain of OmpA on antibiotic sensitivity, cells were spotted onto LB plates containing 6 μg/ml ampicillin, 2.5 μg/ml cefalotin, 6 μg/ml choramphenicol, 150 μg/ml clindamycin, or 5 μg/ml rifampicin. After 8–20 h incubation at 37°C, photographs of the plates were taken with a digital camera EOS 100D (Canon Inc., Tokyo, Japan). Isopropyl-β-d-thiogalactopyranoside of 50 μM was added when experiments using CPRG were performed.
Determination of Membrane Integrity Using Fluorescent Chemicals
For fluorescence imaging, stationary phase cells of wild-type and ompA ompC ompF triple mutants were inoculated into LB medium. When the A600 reached 2, cells were stained with FM4-64 [N-(3-triethylammoniumpropyl)-4-(p-diethylaminophenylhexatrienyl)-pyridinium dibromide], propidium iodide (red), DAPI (4′,6-diamidino-2-phenylindole) (blue), and SYTOX green (green), and then spotted on a 1% agarose pad prepared in phosphate buffered saline (PBS). Cells were visualized using a Nikon Eclipse Ni microscope (Nikon, Japan).
Results
The Effect of Porins on Antibiotic Resistance
To perform a systematic analysis of the effects of porins on antibiotic resistance in E. coli, we constructed the deletion mutants for all porin genes of E. coli and measured the corresponding MIC values of various antibiotics from different classes (Supplementary Table S3). The ompA mutant exhibited an increased susceptibility to many antibiotic classes, including β-lactams, glycopeptides, amphenicols, and licosamides (Figure 1A). There was no antibiotic with an increased MIC for the ompA mutant compared to that of wild-type cells. On the other hand, the ompF mutant was resistant to many antibiotics belonging to various classes, including β-lactams, amphenicols, tetracyclines, licosamides, steroides, and quinolones (Figure 1A). The ompF mutant did not decrease the MIC value of any antibiotic compared to that for the wild-type background. These results suggest that many antibiotics could be transported into the periplasm via OmpF. Notably, the ompC mutant was sensitive to some antibiotics, such as imipenem, vancomycin, and furomycin, but it was resistant to other antibiotics, such as streptomycin, fusidic acid, and nitrofurantoin (Figure 1A), suggesting that OmpC is involved in the transport of antibiotics, but has various sensitivity to different classes of these compounds. Therefore, these results indicate that non-specific porins (OmpF, OmpA, and OmpC) play a distinct role in antibiotic resistance.
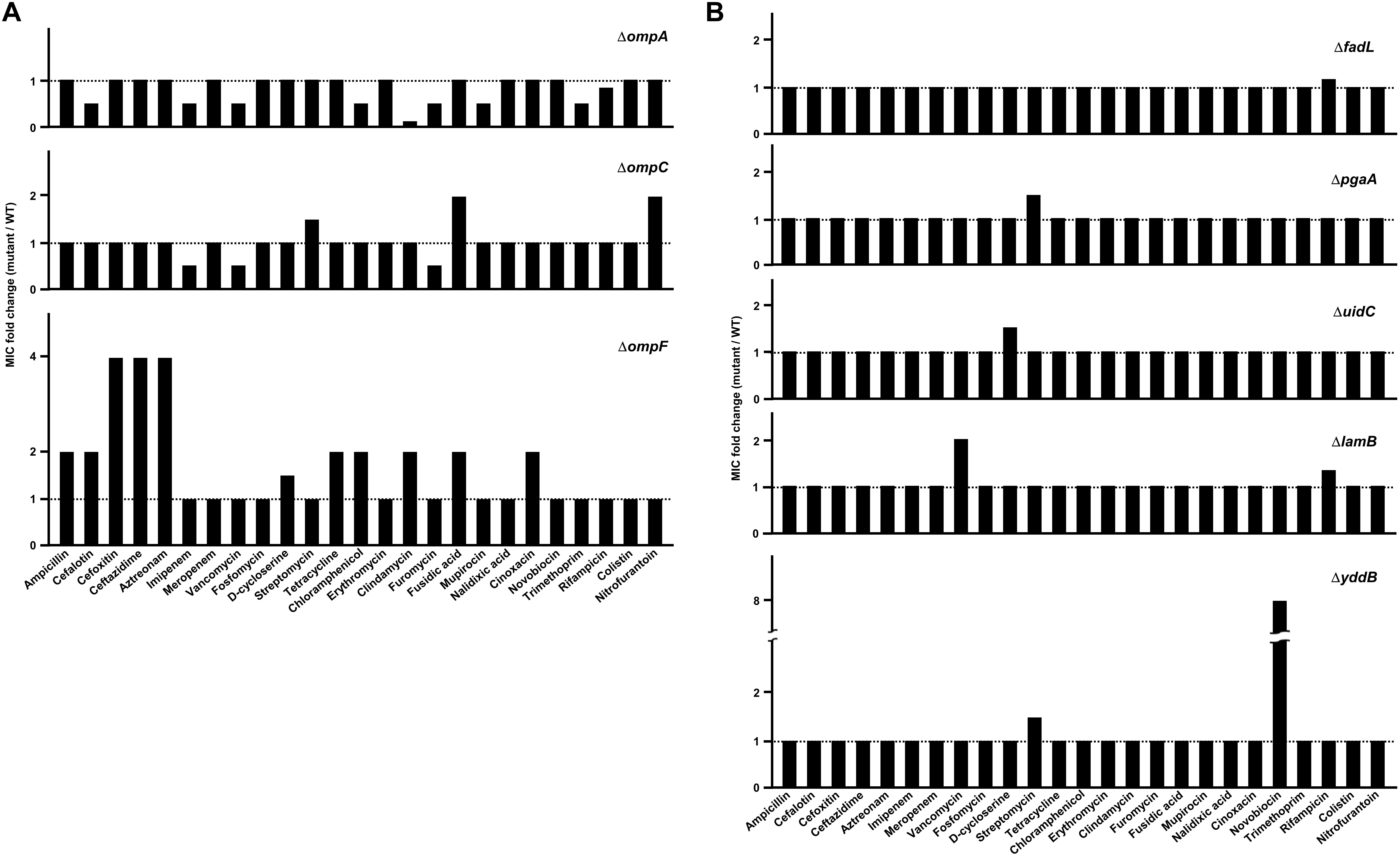
Figure 1. A systematic examination of the effects of porins on antibiotic resistance. (A) The MICs of various antibiotics were measured in the wild-type or mutant strain defective for OmpA, OmpC, or OmpF. The relative MIC value in the mutant cells compared to the wild-type cells is shown. (B) The MICs of various antibiotics were measured in the wild-type or mutant strain defective for the indicated specific porin. The relative MIC value in the mutant cells compared to the wild-type cells is shown.
Most specific porins did not affect the MICs of the tested antibiotics (Supplementary Table S1), but several specific porins are associated with the passive transport of some antibiotics (Figure 1B). The fadL, pgaA, uidC, or lamB mutant was resistant to rifampicin, streptomycin, d-cycloserine, or vancomycin, respectively. In particular, the yddB mutant showed an eight-fold increase in the MIC of novobiocin (Figure 1B). The ectopic expression of the yddB gene using the pBAD plasmid with an arabinose-inducible promoter restored the MIC value to the wild-type level (Figure 2A). Similarly, the MIC of vancomycin for the lamB mutant was restored to the wild-type level by ectopic expression of the lamB gene in the lamB mutant (Figure 2B), suggesting that YddB and LamB are the specific porins that are involved in the passive transport of novobiocin and vancomycin, respectively. Therefore, our systematic analyses of the mutants of all specific porins of E. coli revealed that most specific porins are not involved in antibiotic resistance. However, several specific porins were shown to be related to the outer membrane penetration of several antibiotics. Specifically, the novel specific porin YddB, playing a major role in the passive transport of novobiocin, was identified.
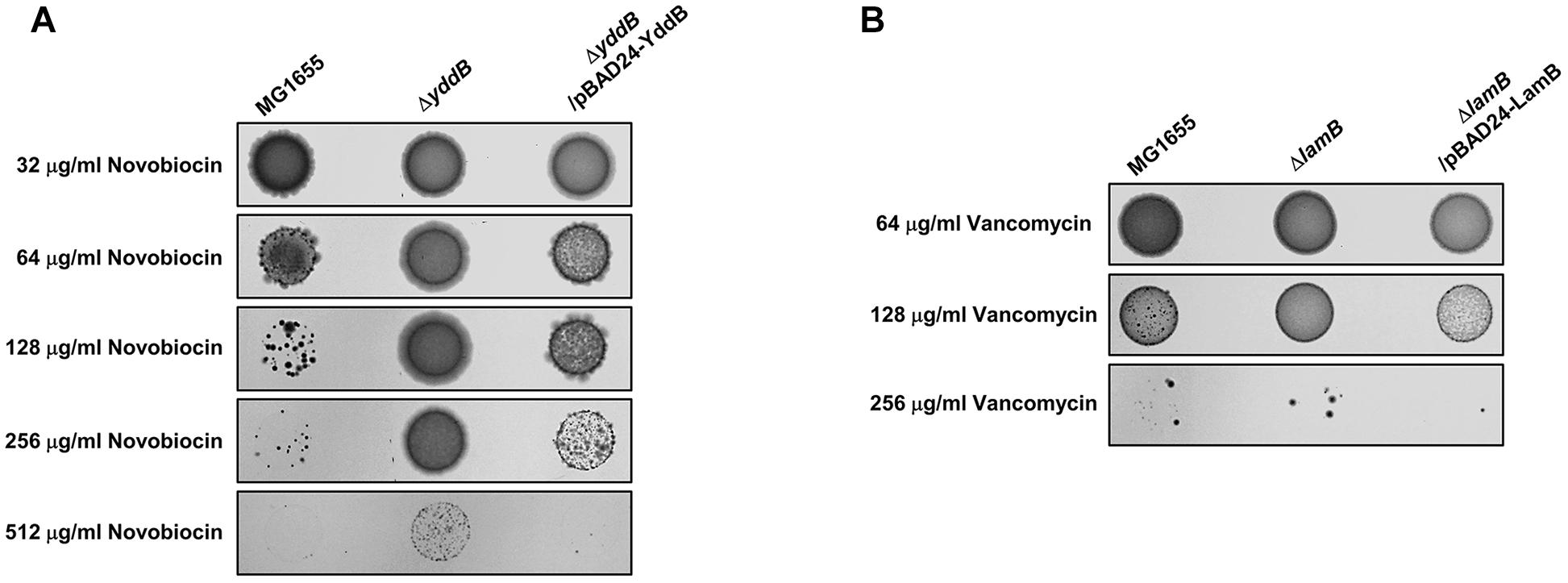
Figure 2. Complemetation of the antibiotic-resistant phenotype of the yddB or lamB mutant. (A) The MICs of novobiocin were examined in 0.01% arabinose-containing agar plates with the agar dilution method according to the Clinical Laboratory Standards Institute guidelines in the wild-type or yddB mutant with or without a pBAD-YbbB plasmid. After incubation at 37°C for 20 h, photographs of the plates were taken with a digital camera. (B) The MICs of vancomycin were examined in 0.01% arabinose-containing agar plates with the agar dilution method in the wild-type or lamB mutant with or without a pBAD-LamB plasmid.
The Effect of Porins on the Envelope Stress Response
To elucidate the mechanism of the ompA mutant conferring sensitivity to various antibiotics, we examined the effect of porins on envelope stress responses. Although the specific porin mutants were not influenced by envelope stresses, including SDS, ethanol, and salt stresses (Supplementary Figure S1), the non-specific porins, especially OmpA and OmpC, were strongly associated with the envelope stress response (Figure 3). The ompA mutant showed a significantly enhanced sensitivity to salt stress. Furthermore, the ompC mutant showed slightly increased sensitivity to salt stress. Under ethanol stress, only the ompC mutant showed a strong retardation of cell growth (Figure 3A). Three mutant strains did not show any significant difference in growth compared to the wild type strain under SDS stress. These results indicate that OmpA and OmpC play an important role in the envelope stress response.
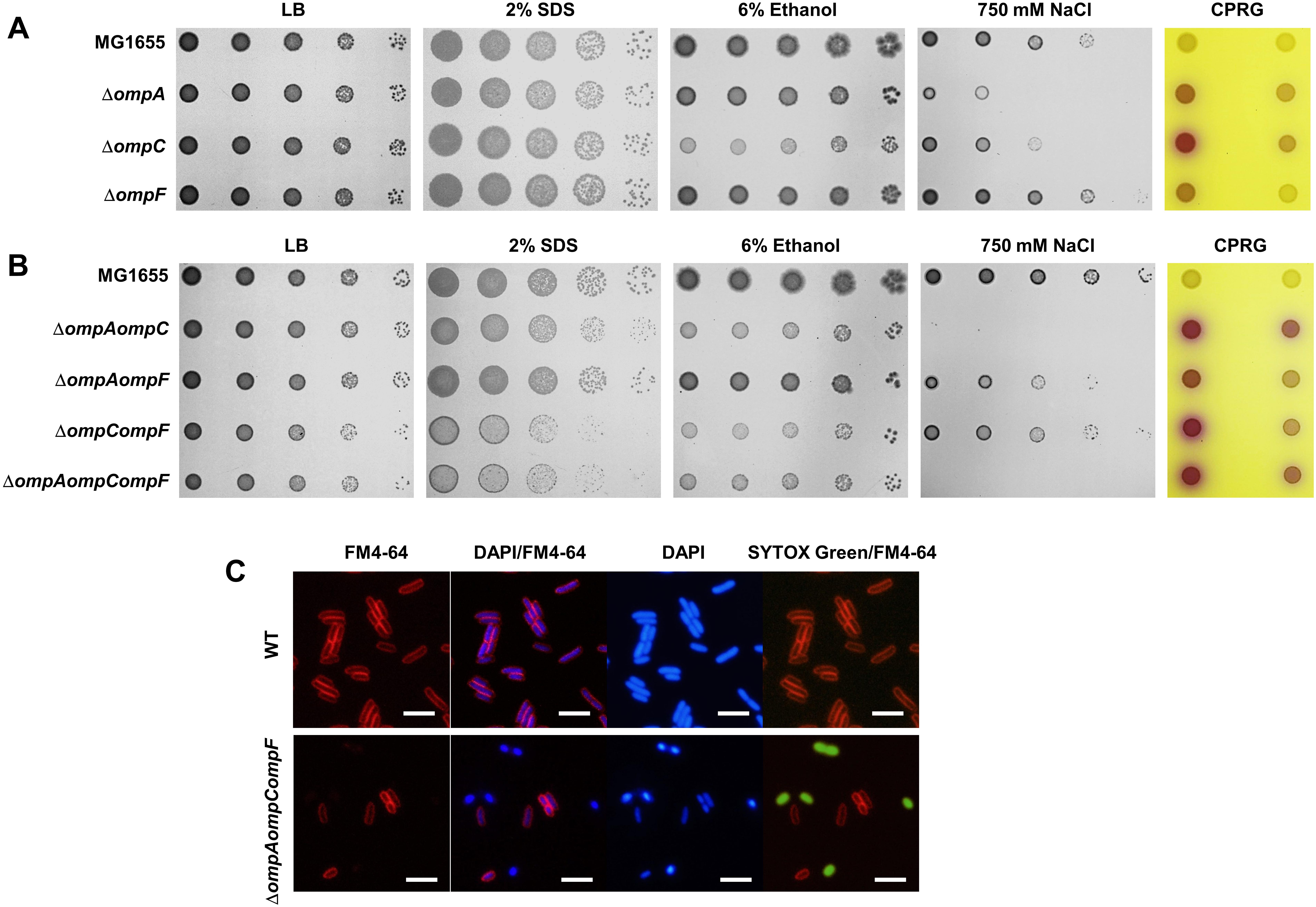
Figure 3. The effect of non-specific porins on envelope stress responses and membrane integrity. (A) The wild-type, ompA, ompC, or ompF mutant cells were serially diluted from 108 to 104 cells/ml in 10-fold steps and spotted onto a LB plate or LB plates with the addition of 2% SDS, 6% ethanol, 750 mM NaCl, or 20 μg/ml CPRG as indicated. (B) The wild-type, ompA ompC, ompA ompF, ompC ompF, or ompA ompC ompF mutant cells were serially diluted 10-fold from 108 to 104 cells/ml and spotted onto a LB plate or LB plates with the addition of 2% SDS, 6% ethanol, 750 mM NaCl, or 20 μg/ml CPRG as indicated. (C) The wild-type or ompA ompC ompF triple mutant cells grown in LB medium were stained with FM4-64 (red), DAPI (blue), and SYTOX green (green), and then spotted on a 1% agarose pad. Cells were visualized using a Nikon Eclipse Ni microscope. Bars, 0.5 μm.
To analyze the roles of non-specific porins in the envelope stress response in more detail, we constructed double and triple mutants of non-specific porins. Under salt stress, the ompA ompC double mutant showed a strong growth defect similar to that of the ompA ompC ompF triple mutant (Figure 3B). Additionally, the growth inhibition of the ompA or ompC mutant under salt stress was not enhanced by an additional deletion of the ompF gene. These results imply that OmpA and OmpC are of significant importance for the salt stress response. In ethanol stress, the ethanol-sensitive phenotype of the ompC mutant was not increased by the additional deletion of the ompA or ompF gene (Figure 3B), suggesting that only OmpC is associated with the ethanol stress response. Under SDS stress, the ompC ompF double mutant showed an enhanced sensitivity to this condition, although the single deletion mutants of non-specific porins were not affected by this stress. The growth defect was not increased by the additional deletion of the ompA gene (Figure 3B). Therefore, OmpC and OmpF seem to be associated with the SDS stress response. However, because the ompA ompC double mutant was slightly more sensitive to SDS stress than the ompC mutant, OmpA also seems to play a minor effect. In summary, these results show that all of the non-specific porins are involved in the envelope stress response, but their roles are different. OmpC seems to play an important role in all stress responses tested, whereas OmpA and OmpF seem to be mainly associated with salt stress and SDS stress responses, respectively. Consistent with the results on antibiotic resistance, these results show distinct roles between non-specific porins.
The Effect of Porins on Membrane Permeability
The importance of non-specific porins on the envelope stress response imply that non-specific porins may affect the membrane permeability. To test this assumption, we performed a LacZ assay using CPRG, a substrate of LacZ that cannot penetrate the E. coli envelope (Paradis-Bleau et al., 2014; Choi et al., 2017). CPRG is able to penetrate the membrane of the cells with increased membrane permeability, where cytoplasmic LacZ degrades it to chlorophenyl red. These cells form red colonies by chlorophenyl red. Expectedly, the membrane permeability test using CPRG showed that only three mutants defective for non-specific porins exhibited increased membrane permeability (Figure 3 and Supplementary Figure S2). The ompC mutant that is involved in all envelope stress responses tested, showed the strongest red phenotype. We also experimentally examined the membrane permeability through experiments using the cell-impermeable, fluorescent DNA dye SYTOX green (Roth et al., 1997). The wild-type cells were not stained with the green fluorescent SYTOX green dye, whereas the ompA ompC ompF triple mutant showed a large increase in the intracellular SYTOX green signal (Figure 3C), indicating an increase of membrane permeability. Interestingly, the SYTOX green-stained cells of the ompA ompC ompF triple mutant were not stained with the amphiphilic membrane dye FM4-64. This result implies that the big change at the OM happens in the triple mutant. We examined the presence of dead cells for this mutant strain, because the ompA ompC ompF triple mutant exhibited a growth defect even in LB medium (Figure 3B). Live–dead staining using the cell viability stain propidium iodide (Meeske et al., 2016), showed decreased cell viability in the ompA ompC ompF triple mutant cells compared to the wild-type cells (Supplementary Figure S3). Therefore, these results indicate that non-specific porins affect the membrane permeability and bacterial viability.
The Importance of the C-Terminal Domain of OmpA in Its Function
OmpA has a flexible C-terminal domain that non-covalently interacts with peptidoglycan (Samsudin et al., 2016). To determine whether this domain is important for the effect of OmpA on antibiotic resistance and the envelope stress response, we constructed the mutant strain (ompAΔC) that is chromosomally deleted for the C-terminal domain of OmpA. Notably, all of the phenotypes of the ompA mutant were phenocopied by the ompAΔC mutant (Figure 4). For example, the ompAΔC mutant was sensitive to salt stress to an extent similar to the ompA mutant and showed a phenotype almost identical to the ompA mutant in the antibiotic susceptibility test. These results suggest that the effect of OmpA on antibiotic resistance and the envelope stress response is entirely dependent on the C-terminal domain of OmpA. The loss of the interaction with peptidoglycan might weaken the turgor of the bacterial envelope, which can induce the increased membrane permeability and antibiotic penetration.
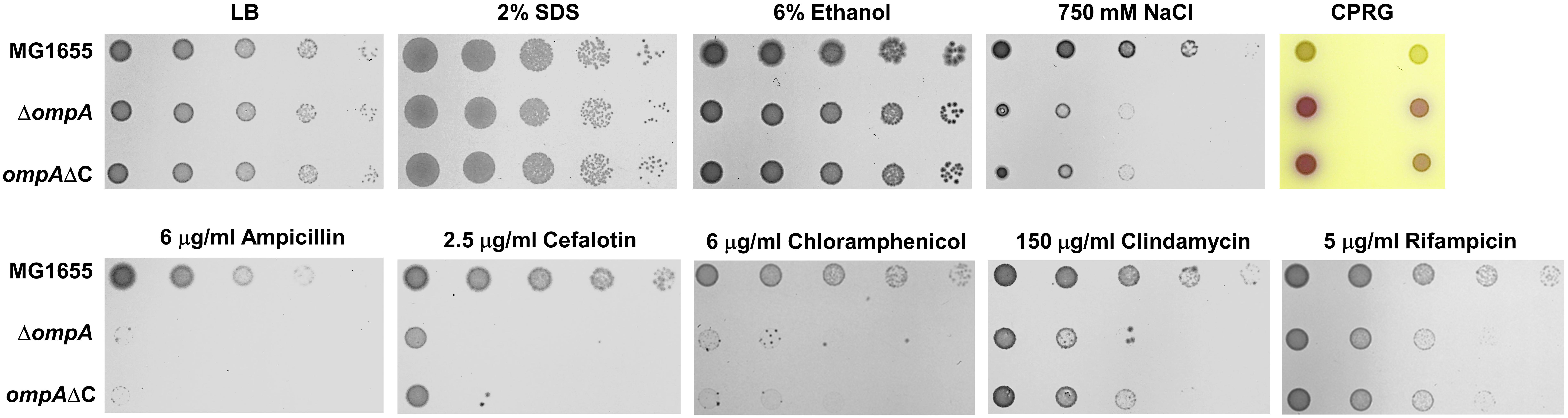
Figure 4. The importance of the C-terminal region of OmpA on its function. The wild-type, ompA, or ompAΔC mutant cells were serially diluted from 108 to 104 cells/ml in 10-fold steps and spotted onto a LB plate or LB plates with the addition of 2% SDS, 6% ethanol, 750 mM NaCl, 20 μg/ml CPRG, 6 μg/ml ampicillin, 2.5 μg/ml cefalotin, 6 μg/ml choramphenicol, 150 μg/ml clindamycin, or 5 μg/ml rifampicin as indicated.
The Effect of Double and Triple Mutants of Non-specific Porins on Antibiotic Resistance
Our results showed that specific porins are only associated with the passive transport of antibiotics, whereas non-specific porins are involved in membrane permeability as well as antibiotic transport, indicating the functional diversity of non-specific porins. To understand the various functions of non-specific porins in more detail, we analyzed the antibiotic susceptibility of double and triple mutants of the ompA, ompC, and ompF genes (Figure 5). Notably, phenotype patterns of these mutants could be divided into two groups: β-lactams and other antibiotics. Besides ampicillin and imipenem, the susceptibilities to most β-lactam antibiotics were strongly affected by OmpF. The MIC of most β-lactams was the lowest in the ompA ompC double mutant and the additional deletion of the ompF gene strongly increased the MIC in the ompA ompC double mutant; consequently, the MICs in the ompA ompC ompF triple mutant were 2- to 8-fold higher than those in the wild-type strain (Figure 5A). OmpC also seems to be important for the transport of β-lactam antibiotics. The additional deletion of the ompC gene increased the MICs in the ompF mutant up to four times. Therefore, these results suggest that the transport of β-lactam antibiotics by OmpF and OmpC is the most important factor in the bacterial susceptibility to most β-lactam antibiotics. Ampicillin and imipenem are exceptional cases. Although OmpF is a porin that plays an important role in the transport of ampicillin (Delcour, 2009), the MIC in the ompA ompC ompF triple mutant was 2-fold lower than that in the wild-type strain (Figure 5A). The MIC of imipenem was also 4-fold lower in the triple mutant than in the wild-type strain. These results imply that the effect of non-specific porins on membrane permeability may be a more important factor in the bacterial susceptibility to ampicillin and imipenem.
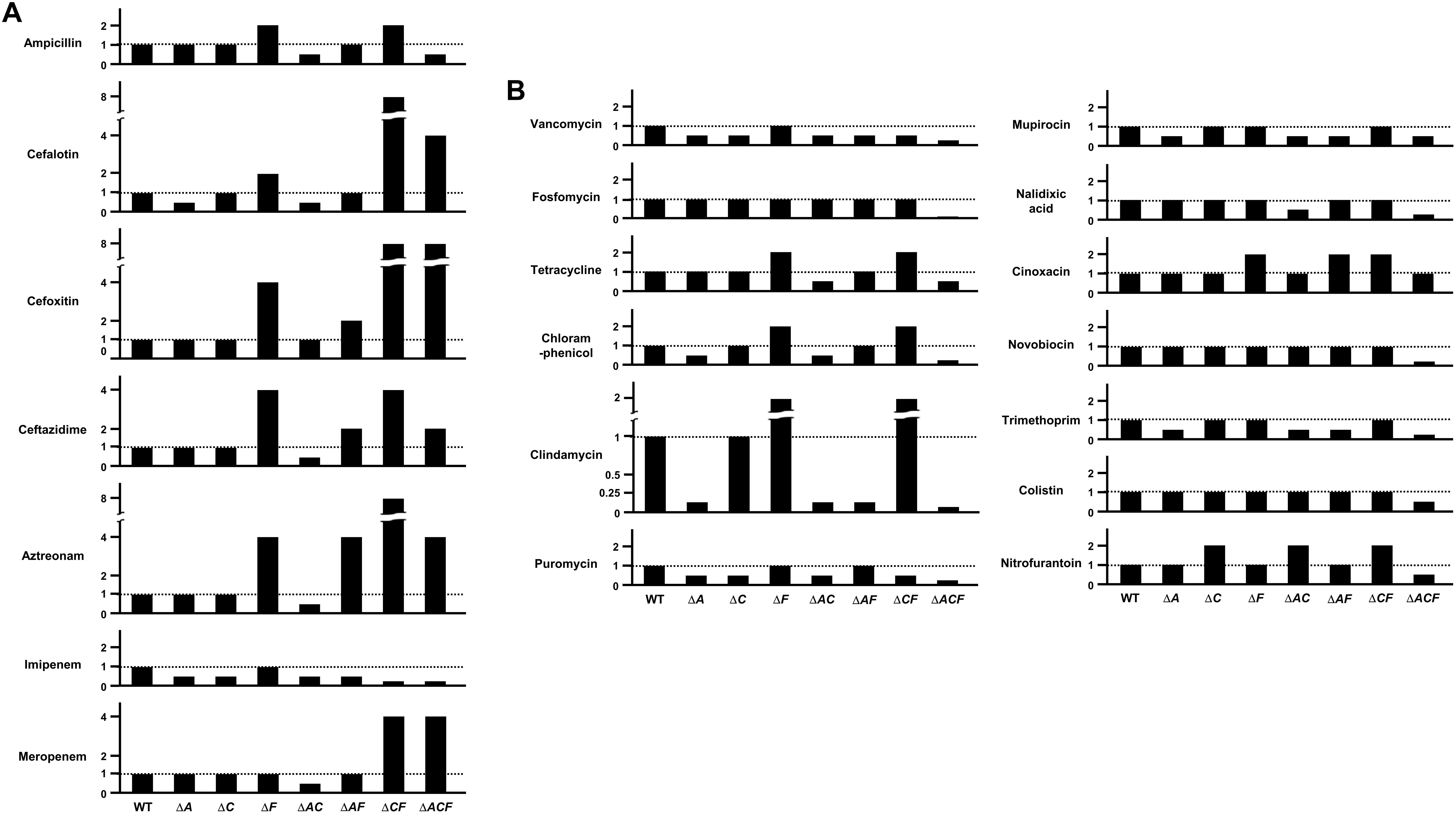
Figure 5. The effects of single-, double-, and triple-mutants of non-specific porins on antibiotic resistance. (A) The MICs of β-lactam antibiotics were measured in the wild-type strain or single-, double-, and triple-mutants of OmpA, OmpC, and OmpF. The relative MIC value in the mutant cells compared to the wild-type cells was shown. (B) The MICs of non-β-lactam antibiotics were measured in the wild-type strain or single-, double-, and triple-mutants of OmpA, OmpC, and OmpF. The relative MIC value in the mutant cells compared to the wild-type cells was shown: WT, MG1655; ΔA, ΔompA; ΔC, ΔompC; ΔF, ΔompF, ΔAC, ΔompA ompC; ΔAF, ΔompA ompF; ΔCF, ΔompC ompF; ΔACF, ΔompA ompC ompF.
In all non-β-lactam antibiotics tested, the strain with the lowest MIC value was the ompA ompC ompF triple mutant (Figure 5B). Even in the case of antibiotics exhibiting higher MICs in the ompF ompC double mutant than in the wild-type strain, the additional deletion of the ompA gene decreased the MICs up to 32 times. The example of clindamycin demonstrates this pattern clearly. Although the MIC of clindamycin was 2-fold higher in the ompF or ompF ompC double mutant than in the wild-type strain, the MICs in the ompF ompA double or ompF ompC ompA triple mutant was 16- or 32-fold lower than those of the wild-type strain (Figure 5B). Therefore, these results suggest that in non-β-lactam antibiotics, the effect of non-specific porins, particularly OmpA, on membrane integrity is more important for antibiotic susceptibility than their roles in antibiotic transport. In conclusion, analyses of the effects of double and triple mutants on antibiotic resistance revealed that two roles of non-specific porins, antibiotic transport and the maintenance of membrane integrity, differently affect the antibiotic susceptibility depending on the kind of antibiotic. The transport of antibiotics via non-specific porins, particularly OmpF and OmpC, strongly affects the susceptibility to most β-lactam antibiotics, whereas the maintenance of the membrane integrity by non-specific porins seems to be more important for the susceptibility to non-β-lactam antibiotics.
Discussion
In this study, we performed a systematic analysis examining the roles of all porins of E. coli in antibiotic susceptibility. Despite the importance of porins for antibiotic resistance, a systematic study about porins has not been attempted previously. Our study shows the complexity of porin-mediated antibiotic resistance. OmpA plays an important role in the maintenance of membrane integrity, rather than the transport of antibiotics (Figure 1). The C-terminal domain is essential for these OmpA functions (Figure 4). Although OmpF is associated with both antibiotic transport and membrane permeability, the ompF mutant exhibits antibiotic resistance phenotypes (Figure 1A). Increased susceptibility to antibiotic was not found. These results may be due to a minor effect of OmpF on membrane integrity (Figure 3). OmpF is known to be a major porin for the OM permeability for β-lactams (Delcour, 2009), which is also supported by our results. The ompF mutant showed significantly increased resistance to several β-lactam antibiotics (Figure 1A). Additionally, in many β-lactam antibiotics, the ompA ompC mutant had the lowest MICs. However, the MICs were dramatically increased with the additional deletion of the ompF gene (Figure 5). These results show the importance of OmpF in the passive transport of β-lactam antibiotics. OmpC seems to play an important role in both antibiotic transport and membrane permeability (Figures 1, 4, 5). Deletion of the ompC gene increased the MICs of some antibiotics, but it decreased the MICs of other antibiotics (Figure 1). OmpC seems to be associated with the transport of many β-lactam antibiotics (Figure 5), which is consistent with previous results (Nikaido et al., 1983; Lou et al., 2011; Kojima and Nikaido, 2014). Furthermore, OmpC plays an important role in the adaptation to various envelope stresses (Figure 3). Therefore, these results demonstrate the distinct roles of three non-specific porins. A previous study showed that the expression level of OmpF was lower than those of OmpA and OmpC (Sato et al., 2000). Our results show that OmpA and OmpC plays an important role in the maintenance of membrane integrity, whereas OmpF is not strongly involved in the maintenance of membrane integrity. Therefore, there is a possibility that E. coil differentially expresses three non-specific porins based on their distinct roles in the maintenance of membrane integrity. All specific porins do not seem to be involved in the envelope stress response (Supplementary Figure S1). Therefore, there is no case with increased antibiotic susceptibility in the mutant defective for specific porin (Figure 1B and Supplementary Table S3). Several specific porins seem to be involved in the passive transport of several antibiotics (Figure 1B). Therefore, E. coli porins can be classified into three groups according to their roles in antibiotic transport and membrane integrity: antibiotic transport-related specific porins (LamB, YddB, etc.), membrane integrity-related non-specific porin (OmpA), and porins associated with both antibiotic transport and membrane integrity (OmpC and OmpF). The functional diversity of porins might indicate why the effects of porins on antibiotic resistance differ so widely depending on the kind of porin. The efflux systems strongly affect antibiotic resistance (Li et al., 2015). Therefore, further studies are required to analyze of the roles of porins in the absence of efflux systems and examine the effects of porins on expression of the efflux systems.
Through our systematic study on E. coli specific porins, we identified a novel porin YddB which seems to be involved in the passive transport of novobiocin across the OM. YddB is an outer membrane protein that is predicted to have a β-barrel structure, but its function is unknown. The yddB mutant displays a dramatically decreased susceptibility to novobiocin compared to the wild type strain (Figure 1). The MIC of novobiocin was not altered by the deletion of any porin gene (Supplementary Table S3); therefore, YddB seems to be a major porin that is responsible for the OM penetration of novobiocin. Besides particular examples, such as YddB and LamB, the role of specific porins for antibiotic transport is of minor importance. Most β-lactam antibiotics are transported with non-specific porins, especially OmpF and OmpC, and most non-β-lactam antibiotics seem to penetrate the OM in a porin-independent manner. However, because a synergistic effect among specific porins cannot be excluded, further experiments are required to analyze whether antibiotic resistance is affected by double or triple deletions of specific porins.
In this study, we find that all non-specific porins, OmpA, OmpC, and OmpF, are involved in the maintenance of membrane integrity, despite a large diversity of their roles and influences. OmpA is known to affect membrane integrity through non-covalent interaction of its C-terminal periplasmic domain with peptidoglycan (Samsudin et al., 2016). The role of the C-terminal domain seems to be critical for the role of OmpA in antibiotic resistance as well as the maintenance of membrane integrity (Figure 4). OmpC is known as osmoporin and the expression of ompC increases under high osmolarity conditions (Pratt et al., 1996). The ompC mutant was sensitive to various envelope stresses (Kaeriyama et al., 2006; Wang et al., 2007; Figure 3), but the exact reason for this phenotype is unknown. A recent study showed that OmpC forms a complex with MlaA and may function in the removal of phospholipids from the OM (Chong et al., 2015). MlaA is a component of the Mla pathway mediating the retrograde transport of phospholipids mislocalized in the outer leaflet of the OM for the maintenance of OM lipid asymmetry (Choi and Lee, 2019). Because the mislocalization of phospholipids in the outer leaflet of the OM weakens the OM integrity (Sutterlin et al., 2016), the role of OmpC in the retrograde transport of phospholipids can be one of the reasons for the diverse OmpC-associated phenotypes. Our study showed that OmpF is also associated with membrane integrity regulation (Figure 3). Because there is no report showing the relationship between OmpF and membrane integrity, the mechanism of this effect remains to be elucidated.
Conclusion
In conclusion, our systematic study shows two possible roles of porins, the transport of antibiotics and the membrane integrity regulation, which differently affect antibiotic resistance, and the distinct role of each porin. This provides an important insight to understand not only the role of each porin as a functionally important component of the outer membrane, but also its specific role in the survival of the pathogen under the action of antibiotics.
Data Availability
All datasets generated for this study are included in the manuscript and/or the Supplementary Files.
Author Contributions
C-RL contributed to the conception and design of the experiments. C-RL and UC researched and wrote the manuscript.
Funding
This work was supported by research grants from the NRF funded by the Ministry of Science and ICT (No. NRF-2018R1A1A1A05023049) and the Bio Medical Technology Development Program of the NRF funded by the Korean Government, MSIT (NRF-2017M3A9E4078017).
Conflict of Interest Statement
The authors declare that the research was conducted in the absence of any commercial or financial relationships that could be construed as a potential conflict of interest.
Supplementary Material
The Supplementary Material for this article can be found online at: https://www.frontiersin.org/articles/10.3389/fmicb.2019.00953/full#supplementary-material
References
Bornet, C., Davin-Regli, A., Bosi, C., Pages, J. M., and Bollet, C. (2000). Imipenem resistance of Enterobacter aerogenes mediated by outer membrane permeability. J. Clin. Microbiol. 38, 1048–1052.
Choi, U., and Lee, C. R. (2019). Antimicrobial agents that inhibit the outer membrane assembly machines of gram negative bacteria. J. Microbiol. Biotechnol. 29, 1–10. doi: 10.4014/jmb.1804.03051
Choi, U., Park, Y. H., Kim, Y. R., Seok, Y. J., and Lee, C. R. (2016). Increased expression of genes involved in uptake and degradation of murein tripeptide under nitrogen starvation in Escherichia coli. FEMS Microbiol. Lett. 363:fnw136. doi: 10.1093/femsle/fnw136
Choi, U., Park, Y. H., Kim, Y. R., Seok, Y. J., and Lee, C. R. (2017). Effect of the RNA pyrophosphohydrolase RppH on envelope integrity in Escherichia coli. FEMS Microbiol. Lett. 364:fnx152. doi: 10.1093/femsle/fnx152
Chong, Z. S., Woo, W. F., and Chng, S. S. (2015). Osmoporin OmpC forms a complex with MlaA to maintain outer membrane lipid asymmetry in Escherichia coli. Mol. Microbiol. 98, 1133–1146. doi: 10.1111/mmi.13202
Datsenko, K. A., and Wanner, B. L. (2000). One-step inactivation of chromosomal genes in Escherichia coli K-12 using PCR products. Proc. Natl. Acad. Sci. U.S.A. 97, 6640–6645. doi: 10.1073/pnas.120163297
Delcour, A. H. (2009). Outer membrane permeability and antibiotic resistance. Biochim. Biophys. Acta 1794, 808–816. doi: 10.1016/j.bbapap.2008.11.005
Henderson, J. C., Zimmerman, S. M., Crofts, A. A., Boll, J. M., Kuhns, L. G., Herrera, C. M., et al. (2016). The power of asymmetry: architecture and assembly of the Gram-negative outer membrane lipid bilayer. Annu. Rev. Microbiol. 70, 255–278. doi: 10.1146/annurev-micro-102215-095308
Iyer, R., Moussa, S. H., Durand-Reville, T. F., Tommasi, R., and Miller, A. (2018). Acinetobacter baumannii OmpA is a selective antibiotic permeant porin. ACS Infect. Dis. 4, 373–381. doi: 10.1021/acsinfecdis.7b00168
Kaeriyama, M., Machida, K., Kitakaze, A., Wang, H., Lao, Q., Fukamachi, T., et al. (2006). OmpC and OmpF are required for growth under hyperosmotic stress above pH 8 in Escherichia coli. Lett. Appl. Microbiol. 42, 195–201. doi: 10.1111/j.1472-765X.2006.01845.x
Koebnik, R., Locher, K. P., and Van Gelder, P. (2000). Structure and function of bacterial outer membrane proteins: barrels in a nutshell. Mol. Microbiol. 37, 239–253. doi: 10.1046/j.1365-2958.2000.01983.x
Kojima, S., and Nikaido, H. (2014). High salt concentrations increase permeability through OmpC channels of Escherichia coli. J. Biol. Chem. 289, 26464–26473. doi: 10.1074/jbc.M114.585869
Lee, C. R., Cho, I. H., Jeong, B. C., and Lee, S. H. (2013). Strategies to minimize antibiotic resistance. Int. J. Environ. Res. Public Health 10, 4274–4305. doi: 10.3390/ijerph10094274
Lee, J., Park, Y. H., Kim, Y. R., Seok, Y. J., and Lee, C. R. (2015). Dephosphorylated NPr is involved in an envelope stress response of Escherichia coli. Microbiology 161, 1113–1123. doi: 10.1099/mic.0.000056
Li, X. Z., Plesiat, P., and Nikaido, H. (2015). The challenge of efflux-mediated antibiotic resistance in Gram-negative bacteria. Clin. Microbiol. Rev. 28, 337–418. doi: 10.1128/CMR.00117-14
Lou, H., Chen, M., Black, S. S., Bushell, S. R., Ceccarelli, M., Mach, T., et al. (2011). Altered antibiotic transport in OmpC mutants isolated from a series of clinical strains of multi-drug resistant E. coli. PLoS One 6:e25825. doi: 10.1371/journal.pone.0025825
Mach, T., Neves, P., Spiga, E., Weingart, H., Winterhalter, M., Ruggerone, P., et al. (2008). Facilitated permeation of antibiotics across membrane channels-interaction of the quinolone moxifloxacin with the OmpF channel. J. Am. Chem. Soc. 130, 13301–13309. doi: 10.1021/ja803188c
Meeske, A. J., Riley, E. P., Robins, W. P., Uehara, T., Mekalanos, J. J., Kahne, D., et al. (2016). SEDS proteins are a widespread family of bacterial cell wall polymerases. Nature 537, 634–638. doi: 10.1038/nature19331
Moya-Torres, A., Mulvey, M. R., Kumar, A., Oresnik, I. J., and Brassinga, A. K. (2014). The lack of OmpF, but not OmpC, contributes to increased antibiotic resistance in Serratia marcescens. Microbiology 160, 1882–1892. doi: 10.1099/mic.0.081166-0
Nikaido, H. (2003). Molecular basis of bacterial outer membrane permeability revisited. Microbiol. Mol. Biol. Rev. 67, 593–656. doi: 10.1128/MMBR.67.4.593-656.2003
Nikaido, H., Rosenberg, E. Y., and Foulds, J. (1983). Porin channels in Escherichia coli: studies with β-lactams in intact cells. J. Bacteriol. 153, 232–240.
Okamoto, K., Gotoh, N., and Nishino, T. (2001). Pseudomonas aeruginosa reveals high intrinsic resistance to penem antibiotics: penem resistance mechanisms and their interplay. Antimicrob. Agents Chemother. 45, 1964–1971. doi: 10.1128/AAC.45.7.1964-1971.2001
O’Shea, R., and Moser, H. E. (2008). Physicochemical properties of antibacterial compounds: implications for drug discovery. J. Med. Chem. 51, 2871–2878. doi: 10.1021/jm700967e
Pages, J. M., James, C. E., and Winterhalter, M. (2008). The porin and the permeating antibiotic: a selective diffusion barrier in Gram-negative bacteria. Nat. Rev. Microbiol. 6, 893–903. doi: 10.1038/nrmicro1994
Paradis-Bleau, C., Kritikos, G., Orlova, K., Typas, A., and Bernhardt, T. G. (2014). A genome-wide screen for bacterial envelope biogenesis mutants identifies a novel factor involved in cell wall precursor metabolism. PLoS Genet. 10:e1004056. doi: 10.1371/journal.pgen.1004056
Pratt, L. A., Hsing, W., Gibson, K. E., and Silhavy, T. J. (1996). From acids to osmZ: multiple factors influence synthesis of the OmpF and OmpC porins in Escherichia coli. Mol. Microbiol. 20, 911–917. doi: 10.1111/j.1365-2958.1996.tb02532.x
Roth, B. L., Poot, M., Yue, S. T., and Millard, P. J. (1997). Bacterial viability and antibiotic susceptibility testing with SYTOX green nucleic acid stain. Appl. Environ. Microbiol. 63, 2421–2431.
Samsudin, F., Ortiz-Suarez, M. L., Piggot, T. J., Bond, P. J., and Khalid, S. (2016). OmpA: a flexible clamp for bacterial cell wall attachment. Structure 24, 2227–2235. doi: 10.1016/j.str.2016.10.009
Sato, M., Machida, K., Arikado, E., Saito, H., Kakegawa, T., and Kobayashi, H. (2000). Expression of outer membrane proteins in Escherichia coli growing at acid pH. Appl. Environ. Microbiol. 66, 943–947. doi: 10.1128/AEM.66.3.943-947.2000
Schulz, G. E. (2002). The structure of bacterial outer membrane proteins. Biochim. Biophys. Acta 1565, 308–317. doi: 10.1016/S0005-2736(02)00577-1
Smani, Y., Fabrega, A., Roca, I., Sanchez-Encinales, V., Vila, J., and Pachon, J. (2014). Role of OmpA in the multidrug resistance phenotype of Acinetobacter baumannii. Antimicrob. Agents Chemother. 58, 1806–1808. doi: 10.1128/AAC.02101-13
Sugawara, E., Kojima, S., and Nikaido, H. (2016). Klebsiella pneumoniae major porins OmpK35 and OmpK36 allow more efficient diffusion of β-lactams than their Escherichia coli homologs OmpF and OmpC. J. Bacteriol. 198, 3200–3208. doi: 10.1128/JB.00590-16
Sugawara, E., and Nikaido, H. (1992). Pore-forming activity of OmpA protein of Escherichia coli. J. Biol. Chem. 267, 2507–2511.
Sutterlin, H. A., Shi, H., May, K. L., Miguel, A., Khare, S., Huang, K. C., et al. (2016). Disruption of lipid homeostasis in the Gram-negative cell envelope activates a novel cell death pathway. Proc. Natl. Acad. Sci. U.S.A. 113, E1565–E1574. doi: 10.1073/pnas.1601375113
Wang, Y., Wang, L., Sun, Y., Chen, Y., Zhu, L., Guo, L., et al. (2007). Disrupted OmpC causes osmosis sensitivity of Escherichia coli in alkaline medium. J. Genet. Genomics 34, 1131–1138. doi: 10.1016/S1673-8527(07)60129-5
Wikler, M. A., and CLSI (2018). Methods for Dilution Antimicrobial Susceptibility Tests for Bacteria That Grow Aerobically; Approved Standard, 11th Edn. Wayne, PA: Clinical and Laboratory Standards Institute.
Keywords: antibiotic resistance, porins, membrane integrity, OmpA, OmpC, OmpF
Citation: Choi U and Lee C-R (2019) Distinct Roles of Outer Membrane Porins in Antibiotic Resistance and Membrane Integrity in Escherichia coli. Front. Microbiol. 10:953. doi: 10.3389/fmicb.2019.00953
Received: 06 March 2019; Accepted: 15 April 2019;
Published: 30 April 2019.
Edited by:
Helen Zgurskaya, The University of Oklahoma, United StatesReviewed by:
Matteo Ceccarelli, University of Cagliari, ItalyOlga Novikova, G.B. Elyakov Pacific Institute of Bioorganic Chemistry (RAS), Russia
Copyright © 2019 Choi and Lee. This is an open-access article distributed under the terms of the Creative Commons Attribution License (CC BY). The use, distribution or reproduction in other forums is permitted, provided the original author(s) and the copyright owner(s) are credited and that the original publication in this journal is cited, in accordance with accepted academic practice. No use, distribution or reproduction is permitted which does not comply with these terms.
*Correspondence: Chang-Ro Lee, Y3JsZWVAbWp1LmFjLmty