- 1Department of Biomedical Sciences, Cardiff School of Health Sciences, Cardiff Metropolitan University, Cardiff, United Kingdom
- 2Swansea University Medical School, Swansea University, Swansea, United Kingdom
- 3School of Dentistry, College of Biomedical and Life Sciences, Cardiff University, Cardiff, United Kingdom
Pseudomonas aeruginosa causes problematic chronic lung infections in those suffering from cystic fibrosis. This is due to its antimicrobial resistance mechanisms and its ability to form robust biofilm communities with increased antimicrobial tolerances. Using novel antimicrobials or repurposing current ones is required in order to overcome these problems. Manuka honey is a natural antimicrobial agent that has been used for many decades in the treatment of chronic surface wounds with great success, particularly those infected with P. aeruginosa. Here we aim to determine whether the antimicrobial activity of manuka honey could potentially be repurposed to inhibit pulmonary P. aeruginosa infections using two ex vivo models. P. aeruginosa isolates (n = 28) from an international panel were tested for their susceptibility to manuka honey and clinically relevant antibiotics (ciprofloxacin, ceftazidime, and tobramycin), alone and in combination, using conventional antimicrobial susceptibility testing (AST). To increase clinical applicability, two ex vivo porcine lung (EVPL) models (using alveolar and bronchiolar tissue) were used to determine the anti-biofilm effects of manuka honey alone and in combination with antibiotics. All P. aeruginosa isolates were susceptible to manuka honey, however, varying incidences of resistance were seen against antibiotics. The combination of sub-inhibitory manuka honey and antibiotics using conventional AST had no effect on activity against the majority of isolates tested. Using the two ex vivo models, 64% (w/v) manuka honey inhibited many of the isolates where abnormally high concentrations of antibiotics could not. Typically, combinations of both manuka honey and antibiotics had increased antimicrobial activity. These results highlight the potential of manuka honey as a future antimicrobial for the treatment of pulmonary P. aeruginosa isolates, clearing potential infection reservoirs within the upper airway.
Introduction
Pseudomonas aeruginosa is an opportunistic pathogen, capable of causing infections in various immunocompromised patient groups (de Bentzmann and Plésiat, 2011). Patients suffering with Cystic Fibrosis (CF) are at a heightened risk of P. aeruginosa infection (Lyczak et al., 2002). Amongst the many complications that affect the body of CF patients, mucus build-up within the lung results in chronic pulmonary colonization, with the nasal cavity and upper respiratory tract acting as reservoirs for recurrent infections (Siegel and Weiser, 2015). Up to 80% of CF patients will acquire a pulmonary P. aeruginosa infection, an event that is linked to poor patient prognosis and infection chronicity (Schelstraete et al., 2013). This link between P. aeruginosa and persistence, is due, in part, to the formation of biofilms by mucoid strains (Høiby et al., 2010).
Biofilm formation in P. aeruginosa leads to increased antimicrobial tolerance (AMT), an extra defensive strategy on top of the innate and acquired antimicrobial resistance (AMR) mechanisms it possesses (El Zowalaty et al., 2015). Eradication of P. aeruginosa from the CF lung is therefore not always possible, even when aggressive antimicrobial treatment regimens are used. There are few effective therapeutic options for pulmonary P. aeruginosa infections, with many patients harboring multi- drug resistant strains (López-Causapé et al., 2017). In order to provide respite care for these patients, novel antimicrobial agents with effective anti-biofilm effects must be identified (Worthington and Melander, 2013).
Recently, researchers have sought innovative antimicrobial strategies, utilizing natural products in order to combat AMR (Halstead et al., 2015; Harrison et al., 2015; Moloney, 2016). An antimicrobial agent which has demonstrable antimicrobial and anti-biofilm activity is manuka honey (Molan, 2016; White, 2016). It is well documented in wound microbiology that manuka honey is an effective treatment for chronic infections with exceptional activity against a range of AMR organisms (Mandal and Mandal, 2011; Maddocks and Jenkins, 2013). To date, two mechanisms of action have been identified: impairment of the cell division process described in Staphylococcus aureus, (Jenkins et al., 2011) and disruption of the cell envelope in P. aeruginosa (Roberts et al., 2012).
Previous manuka honey research has focussed on pathogens associated with wounds. However, many species found in wounds are synonymous with pulmonary infection. A recent study showed that manuka honey is capable of inhibiting the growth of multiple P. aeruginosa isolates (n = 56) of pulmonary origin (Jenkins et al., 2015), however, the methodologies used better represented acute infections as opposed to chronic biofilm-infections (European Committee for Antimicrobial Susceptibilty Testing, 2003). To ensure the best translational applicability for novel treatments, models that more accurately represent in vivo conditions are required (Roberts et al., 2015).
Recently, two ex vivo porcine lung (EVPL) models for the study of pulmonary P. aeruginosa biofilms have been described (Harrison et al., 2015; Harrison and Diggle, 2016). These provide a spatially structured in vivo environment with similar nutrient cues to those identified in CF sputum, allowing bacteria to form biofilms similar to those found in chronic lung infection (Palmer et al., 2005). This study is the first to utilize these more relevant models to determine the inhibitory efficacy of relevant antibiotics and medical grade manuka honey against a wide range of pulmonary P. aeruginosa isolates selected from an international reference panel (De Soyza et al., 2013). This focussed panel of isolates allows a wide range of pheno- and geno-types to be extensively tested, covering the wide diversity of P. aeruginosa isolates observed in the CF lung from commonly studied clones, to highly transmissible strains.
Materials and Methods
Ethics Statement
Materials required for the EVPL model were sourced from a local butcher as waste products of the food industry.
Manuka Honey
Gamma-irradiated medical grade manuka honey (Medihoney; Integra Life Sciences) with a Unique Manuka Factor (UMF) rating of 12+ was used throughout.
Bacterial Strains and Culture Conditions
Twenty eight P. aeruginosa Reference Panel (PaRP) isolates (De Soyza et al., 2013), were selected and covered a wide range of phenotypically and genotypically diverse isolates. Isolates were recovered from frozen stocks on Cation Adjusted (10 mg/L Mg2+ and 20 mg/L Ca2+) Mueller Hinton Agar (CA-MHA) for 24 h at 37°C. Log-phase and stationary phase cells were created by transferring single colonies to aliquots of Cation Adjusted Mueller Hinton Broth (CA-MHB) for 6 and 24 h, respectively, at 37°C.
Minimum Inhibitory Concentrations (MICs) for Antimicrobial Agents
The Minimum Inhibitory Concentration (MIC) of manuka honey and clinically relevant antibiotics (Cystic Fibrosis Trust, 2009), was determined in accordance with microbroth dilution standards set out by the European Committee on Antimicrobial Susceptibility Testing (EUCAST; European Committee for Antimicrobial Susceptibilty Testing, 2003). Various doubling dilutions of manuka honey (1–32% w/v), ciprofloxacin (0.002–128 μg/ml), ceftazidime 0.03–2048 μg/ml), and tobramycin (0.016–1024 μg/ml) were used to determine the MICs. All results were derived from triplicate biological replicas.
Determining Potential Interaction Between Antimicrobial Agents
To test for interactions between manuka honey and antibiotics, sub-inhibitory concentrations of honey (0.5, 0.25, and 0.125 of the MIC) were supplemented with various concentrations of antibiotics using a checkerboard methodology. Fractional Inhibitory Concentration Indices (FICI), which determine interplay between two antimicrobial agents were calculated. Mean FICI values from each of the sub-inhibitory manuka honey concentrations were used to allow a comprehensive dose-response analyses of total inhibition between the two antimicrobial agents and interpreted as: “synergistic” (FICI ≤ 0.5), “antagonistic” (FICI > 4.0), and “no interaction” (FICI > 0.5–4.0) (Odds, 2003). All results were derived from triplicate biological replicas for each of the sub-inhibitory manuka honey concentrations.
Efficacy of Manuka Honey and Antibiotics Against PaRP Biofilms Grown in an ex vivo Porcine Lung (EVPL) Alveolar Model
The anti-biofilm effects of manuka honey and antibiotics were identified using an EVPL alveolar model (Harrison et al., 2014). Briefly, stationary growth phase bacterial cells were washed twice with Phosphate Buffered Saline (PBS) and re-suspended in an Artificial Sputum Media (ASM; Palmer et al., 2007) supplemented with 50 μg/ml ampicillin (ASMAmp) to a final cell density of ∼2 × 105 cells/ml. Triplicate cubes (5 mm3) of EVPL alveolar tissue were dissected from the ventral surface of the left caudal lobe, washed three times with 1 ml Cell Culture Media supplemented with 50 μg/ml ampicillin (50% v/v RPMI 1640 and Dulbecco’s modified Eagle medium [DMEM]), and once with 1 ml ASMAmp. EVPL alveolar tissue cubes were placed on a bed of solidified ASMAmp (using 0.8% agarose) in 24-well microtitre plates, submerged in 0.5 ml ASMAmp and inoculated with 50 μl of cells using a 30-gauge needle. Cubes of EVPL alveolar tissue were incubated at 37°C. After 24 h, the cubes were rinsed with 1 ml PBS, removing loosely adhered cells, and treated with 0.5 ml of 0% (ASMAmp), 32%, or 64% w/v manuka honey supplemented with/without antibiotics (128 μg/ml ciprofloxacin, 2048 μg/ml ceftazidime, or 1024 μg/ml tobramycin) for 16 h. Increased concentrations of antimicrobial solutions were selected due to biofilms being inherently more tolerant of antimicrobial agents. Cubes of EVPL alveolar tissue were then rinsed with 1 ml PBS, and homogenized in 500 μl PBS with 7 mm × 2.8 mm metal beads using a Beadbug Microtube homogeniser (Benchmark Scientific) at 4000 rpm for 40 s. Total viable counts (TVCs) were completed for biofilm cells within in the homogenized samples. Biological replica data was obtained using triplicate independent porcine lungs obtained from the local butcher on separate occasions.
Efficacy of Manuka Honey and Antibiotics Against Certain PaRP Biofilms Grown in an EVPL Bronchiole Model
In addition to the anti-biofilm effects studied using an EVPL alveolar model (simulating lower respiratory tract infections), the effect of manuka honey and antibiotics was studied against PaRP isolate #1 (LES B58, a representative transmissible strain), #17 (PAO1; a representative reference strain), and #28 (NH57388A; a mucoid phenotypically diverse strain) in an EVPL model using bronchiole tissue (simulating upper respiratory tract infections) (Harrison and Diggle, 2016). The transition from alveolar tissue to bronchiole tissue was to aid visualization of the tissue surface, as infection with P. aeruginosa isolates was highly destructive to alveolar tissue. Methodologies similar to the EVPL alveolar model were used, however, bronchiole sections were cleaned of alveolar tissue and dissected into triplicate 5 mm2 sections. These were washed, inoculated, and treated as above. TVCs of cells surviving treatment with each of the antimicrobial agents were determined; parallel samples for PaRP isolate #1 were prepared for Scanning Electron Microscopy (SEM) by fixing overnight in 2.5% [v/v] glutaraldehyde (4°C). Samples were rinsed in distilled water (x3) prior to critical-point drying, employing a gradual ascending series of ethanol dehydration steps. Finally, the samples underwent three changes in hexamethyldisilazane until the solution had evaporated. Samples were then sputter-coated with gold and imaged using the Tescan Vega SEM at 4 kV. SEM images were then processed in ImageJ software. Biological replica data was obtained using triplicate independent porcine lungs obtained from the local butcher on separate occasions.
Statistical Analysis
Analysis of variance (ANOVA) was used to test the main effects of manuka honey and antibiotics on bacterial load with Dunnett’s post hoc test applied where applicable.
Results
All PaRP isolates, using conventional antimicrobial susceptibility testing (AST), were inhibited by 16% or 32% (w/v) manuka honey (n = 22 and 6, respectively; Figure 1A). The majority of isolates with Minimum inhibitory concentration values of 32% w/v manuka honey (n = 5) were paired and/or sequential isolates taken at varying times from the same source (De Soyza et al., 2013). MIC values for ciprofloxacin, ceftazidime, and tobramycin varied above and below the EUCAST breakpoints (Figures 1B–D, respectively). The majority of isolates were sensitive to tobramycin (n = 20) and resistant to ciprofloxacin (n = 17) and ceftazidime (n = 15). PaRP isolate #1, 4, 15, and 41 were shown to be resistant to all tested antibiotics, however, they were inhibited with a lower manuka honey concentration [16% (w/v)].
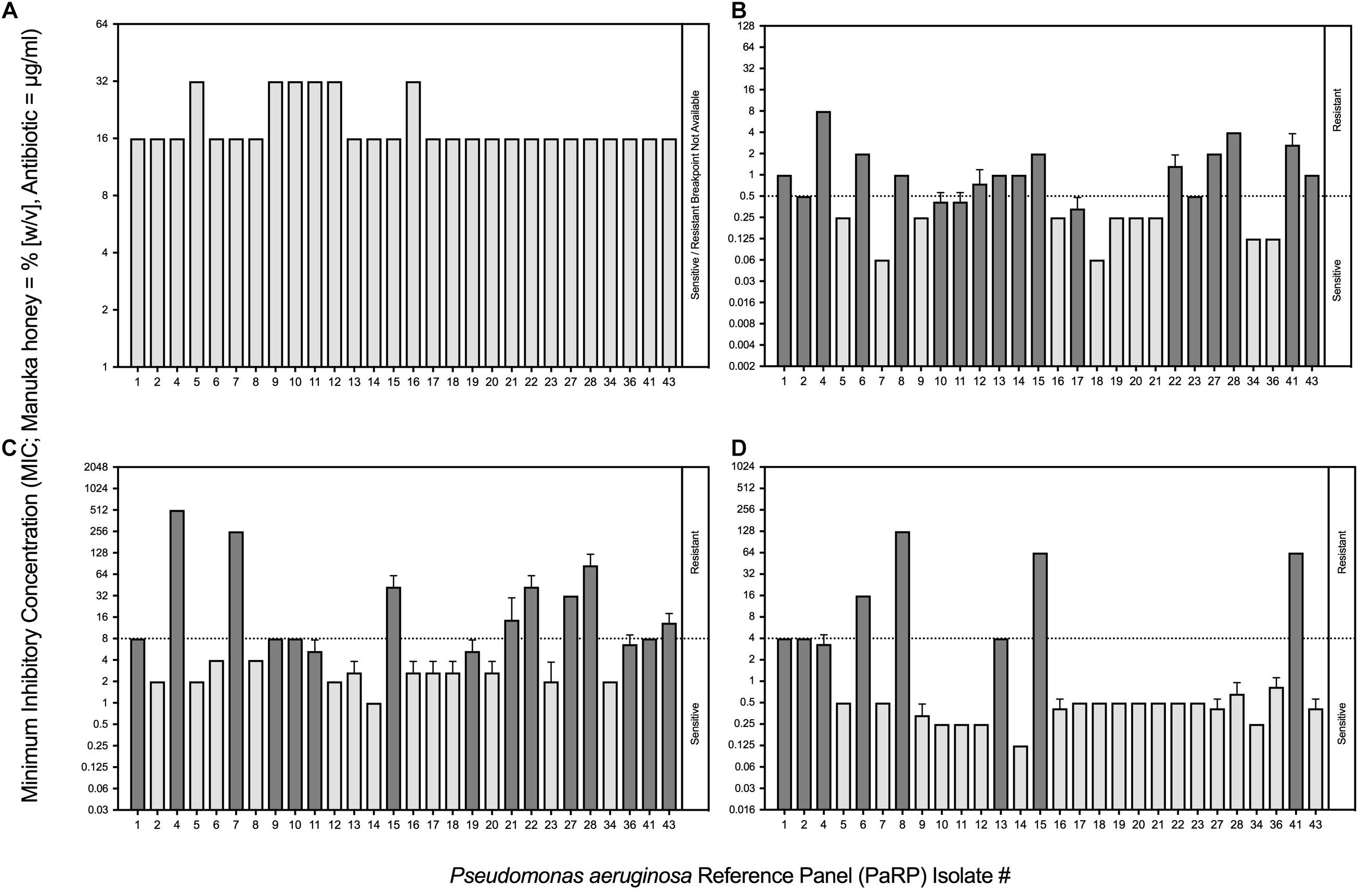
Figure 1. Minimum Inhibitory Concentrations (MICs) of P. aeruginosa Reference Panel (PaRP) isolates associated with Cystic Fibrosis (CF) to manuka honey (expressed as % [w/v]; A) and three clinically relevant antibiotics (expressed as μg/ml); ciprofloxacin (B), ceftazidime (C) and tobramycin (D). PaRP strains found to be sensitive/resistant to the antibiotics tested (B–D), based on EUCAST breakpoints (dashed horizontal line), are colored light/dark gray, respectively (this does not apply to manuka honey (A) where there is no EUCAST breakpoint).
Fractional inhibitory concentration indices values showed that manuka honey and ciprofloxacin had no interactive effects against the majority of PaRP isolates (n = 25; Figure 2A). Some isolate-specific interactions, dependent upon manuka honey concentration, were observed when FICI values crossed interaction “breakpoints” indicative of antagonism and synergism. As an example; various manuka honey and ciprofloxacin combinations worked synergistically against PaRP isolate #41 (Supplementary Figure 1) which was deemed resistant to ciprofloxacin (Figure 1B). FICI values for manuka honey and ceftazidime indicated no interaction between the two antimicrobial agents for the majority of isolates tested (n = 24; Figure 2B). Strain-specific interactions were again observed with some FICI values crossing interaction “breakpoints” indicating antagonism and synergism. As an example; 0.125 MIC manuka honey and ceftazidime worked synergistically against PaRP isolate #4 (Supplementary Figure 2) which was deemed resistant to ceftazidime (Figure 1C). Interactions between manuka honey and tobramycin were more varied, despite there being no discernible interaction against the majority of PaRP isolates tested (n = 19; Figure 2C). This was because FICI values for a larger proportion of isolates crossed antagonistic breakpoints due to two of the sub-inhibitory concentrations of manuka honey having antagonistic FICI values (Supplementary Figure 3). Synergism between tobramycin and 0.25 MIC manuka honey was observed against the tobramycin resistant isolate PaRP #41 (Supplementary Figure 3). Interestingly instances of synergy and antagonism, pushing the mean FICI value over the respective breakpoints were observed when low and high sub-inhibitory concentrations of manuka honey (0.125 and 0.5 MIC, respectively) were used (Supplementary Figure 3).
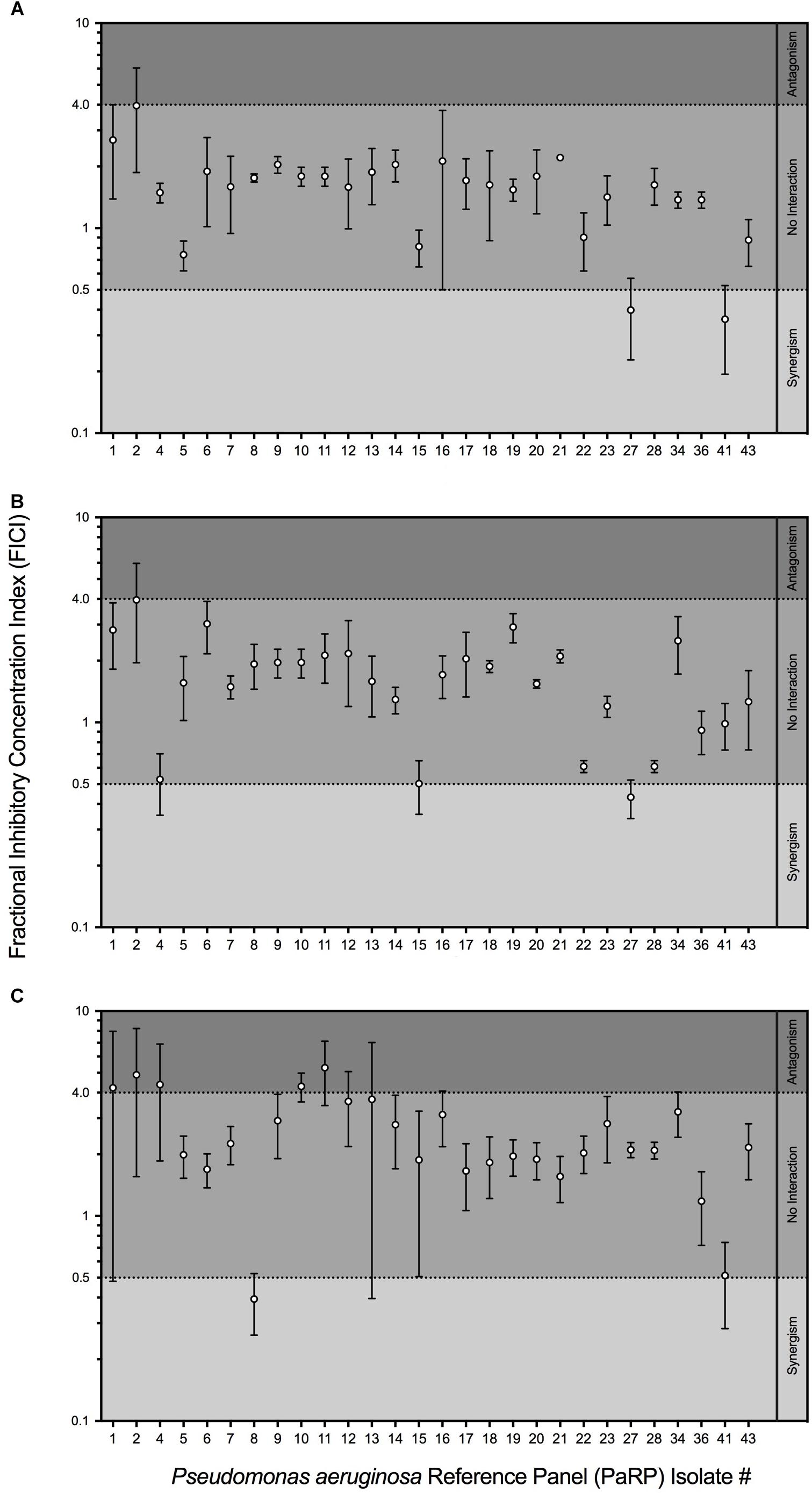
Figure 2. Fractional Inhibitory Concentration Index (FICI) of sub-inhibitory manuka honey concentrations supplemented with one of three clinically relevant antibiotics; ciprofloxacin (A), ceftazidime (B) and tobramycin (C). The interaction between manuka honey and antibiotics were interpreted as ’synergistic (FICI < 0.5), “antagonistic” (FICI > 4.0), and “no interaction” (FICI > 0.5–4.0).
In the EVPL alveolar tissue, all PaRP isolates produced high cell density biofilms (>1 × 107 cfu/5mm3; Figure 3A) with varying visible degrees of exopolysaccharide production (Supplementary Figure 4). Overall, manuka honey demonstrated significant inhibition of multiple PaRP isolates [F(2,168) = 116.3, p < 0.0001], with a range of log-fold reductions being observed in the presence of 32% w/v manuka honey (strain dependent), in addition to the complete inhibition of multiple (n = 12) isolates with 64% w/v manuka honey (Figure 3A). This observation was not seen when]ciprofloxacin or ceftazidime were used alone (despite the high concentrations), however, tobramycin was effective at inhibiting the majority of isolates (n = 17; Figure 3D). When used in combination with ciprofloxacin, ceftazidime or tobramycin, manuka honey induced further statistically significant reductions in cellular viability [F(2,168) = 27.68, p < 0.0001, F(2,168) = 27.42, p < 0.0001, and F(2,168) = 24.61, p < 0.0001, respectively], with an increased incidence rate of total inhibition increased (n = 21, 27, and 28, respectively). It was noted that against some PaRP strains, the addition of various antibiotics had a negative effect on 32% (w/v) manuka honey, slightly reducing its inhibitory efficacy, however, viability remained below that seen in control conditions with no treatment (Figure 3; e.g., ceftazidime and 32% w/v manuka honey had reduced efficacy against PaRP #1 compared to manuka honey alone, however, this activity was better than control).
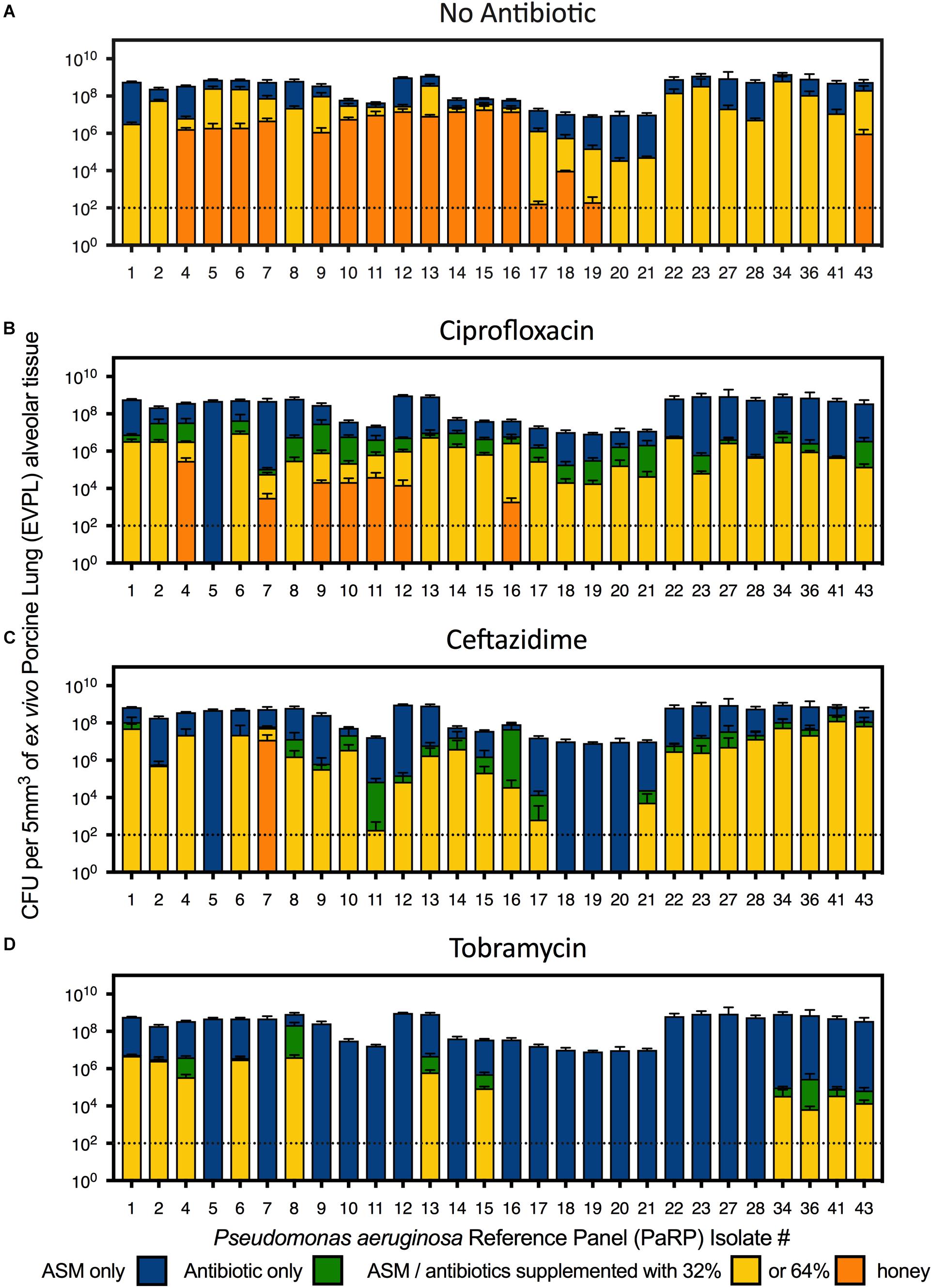
Figure 3. Viability of P. aeruginosa Reference Panel (PaRP) isolates grown in ex vivo Porcine Lung (EVPL) alveolar tissue for 24 h and treated with ASM (A), ciprofloxacin (B), ceftazidime (C), and tobramycin (D) supplemented with 32 and 64% w/v manuka honey for 16 h. Dashed horizontal lines indicate the detection level of the EVPL model. Error bars denote Standard Deviation.
Three representative PaRP isolates grown on EVPL bronchiole tissue sections with ASM demonstrated dense biofilm growth (>1 × 108 cfu/5 mm2; Figure 4). Manuka honey at 64% w/v completely inhibited cellular viability with and without antibiotics, with 3–4 log reductions observed in samples treated with 32% w/v manuka honey (Figure 4). Combinations of ciprofloxacin and manuka honey (32% w/v) caused a marginal (less than 1 log-fold difference), yet significant (P < 0.05) increase in cellular numbers compared to untreated samples (Figure 4). Conversely, ceftazidime and 32% (w/v) manuka honey caused significant reductions in viability (P < 0.001), however, the extent of inhibition observed was strain-dependent, with only marginal reductions against PaRP #17 (Figure 4). As tobramycin completely inhibited two of the three isolates tested, only one isolate could be studied. The viability of PaRP#1 was reduced when 64% manuka honey and tobramycin were combined (Figure 4).
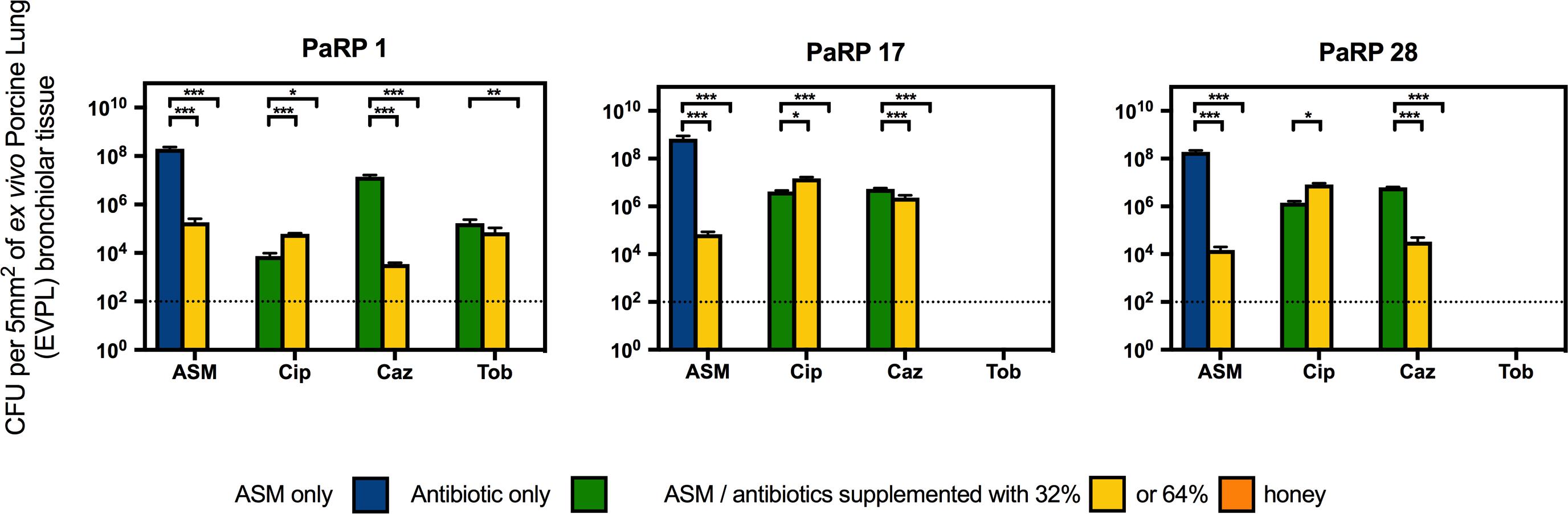
Figure 4. Viability of three P. aeruginosa Reference Panel (PaRP) isolates; #1, #17, and #28, grown in ex vivo Porcine Lung (EVPL) bronchiolar tissue for 24 h and treated with ASM or clinically relevant antibiotics (Cip; Ciprofloxacin, Tob; Tobramycin, and Caz; Ceftazidime) supplemented with 32% and 64% (w/v) manuka honey for 16 h. Dashed horizontal lines indicate the detection level of the model. Error bars denote Standard Deviation. Varying levels of statistical significance denoted by ∗, ∗∗, and ∗∗∗ (P≤0.05, 0.001, and 0.0001 respectively).
Comparative SEM imaging of the EVPL bronchiole tissue infected with PaRP isolate #1 was employed (Figure 5), with concurrent growth of biofilm cells (compared to TVC data) under control conditions (ASM only). In the presence of both concentrations of manuka honey cells were not observed on the surface of bronchioles. Ceftazidime was selected as a representative antibiotic for testing here due to the different effects observed against the selected isolates. In the presence of ceftazidime, bacterial cells were present, however, the addition of varying manuka honey concentrations resulted in the loss of cells from the tissues imaged. It should also be noted that in the presence of 64% (w/v) manuka honey, differences in tissue structure were observed.
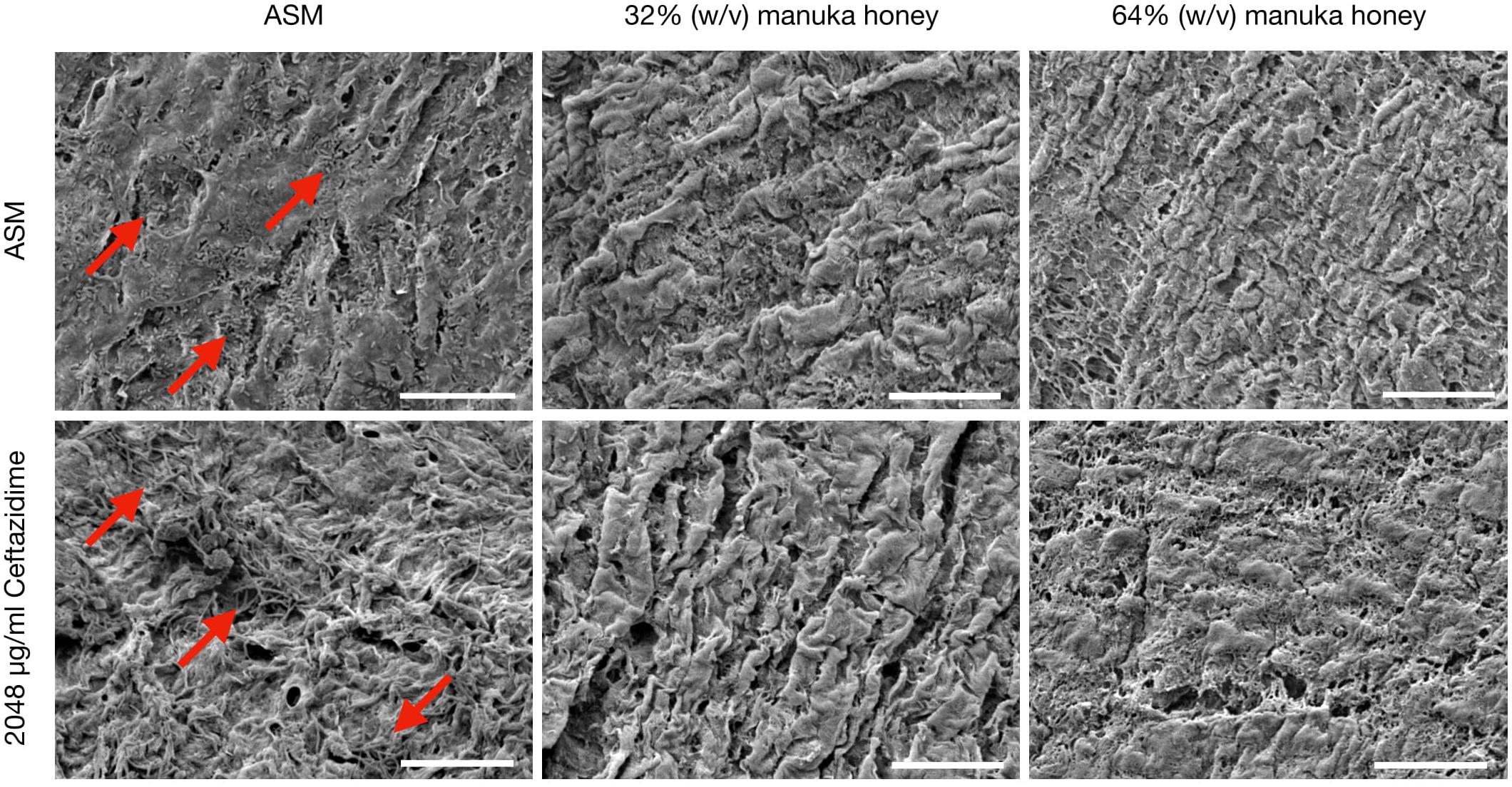
Figure 5. Scanning Electron Microscopy (SEM) images of P. aeruginosa Reference Panel (PaRP) isolate #11 (LES B58) recovered from ex vivo Porcine Lung (EVPL) bronchiole tissue samples grown in Artificial Sputum Media (ASM) with/without 2048 μg/ml ceftazidime and supplemented with/without 32/65% (w/v) manuka honey for 16 h. Images show bacteria cells (red arrows) and their subsequent inhibition with increasing antimicrobial concentrations. Scale bar = 20 μm.
Discussion
Pulmonary P. aeruginosa infections are difficult to treat, due to its various AMR and AMT capabilities and the constraints surrounding the effective delivery of antimicrobial agents (Lee and Yoon, 2017). It is therefore crucial that novel antimicrobial agents are identified, especially those with anti-biofilm activity. This study demonstrates that manuka honey was effective at inhibiting a wide range of P. aeruginosa isolates (22) associated with CF infection; building on the findings of a previous study (Jenkins et al., 2015). For the first time, it was established that manuka honey reduced the viability of various P. aeruginosa isolates in an ex vivo infection model (where clinically relevant antibiotics could not), allowing a realistic assessment of the ability of manuka honey to inhibit biofilms in more realistic tissue types. Previous studies (Maddocks et al., 2012; Lu et al., 2014; Liu et al., 2018) have shown antimicrobial efficacy of manuka honey against biofilms grown using conventional in vitro methods, however, biofilms grown using ex vivo models and ASM are thought to be more representative of clinical biofilms, unlike those formed using conventional in vitro methods and microbiological media (Roberts et al., 2015).
Minimum inhibitory concentration testing was completed using methods that are typical in a clinical setting, and whilst it is believed that these methods (which reply on the use of planktonic cells) do not convey what is seen during chronic infection (biofilms) (Roberts et al., 2015), they represent gold standard practice. Whilst methods looking into the Minimum Biofilm Inhibition Concentration (MBIC) could have been used, they are not done routinely in clinic and would not provide any additional information as the MIC and MBIC of manuka honey have been previously observed to be similar (Liu et al., 2015). Conventional MIC values were higher than those seen previously, and is thought to be due to a change in the methodologies, whereby doubling dilutions were used (so as to be in line with EUCAST methodologies) instead of percentage increments (Jenkins et al., 2015). Combining manuka honey with antibiotics yielded varied results based on the sub-inhibitory concentration of manuka honey used, the combined antibiotic, the strain of P. aeruginosa and the method used (conventional methods vs. the use of the ex vivo model). For the majority of PaRP isolates tested via conventional methods it appeared that manuka honey was non-interactive with the three antibiotics tested, however, some strain specific antagonism and synergism was observed, a stark contrast to previous studies where synergism was widespread (Mandal and Mandal, 2011; Jenkins and Cooper, 2012; Jenkins et al., 2015). The absence of antagonism in the majority of isolates suggests manuka honey could be considered for use alongside conventional antibiotic treatment regimens. In the ex vivo models, combinations of manuka honey and clinically relevant antibiotics had anti-biofilm efficacy, reducing viability for the majority of isolates tested, with total inhibition observed in many instances. This puts manuka honey in a strong position as a potential novel therapeutic for chronic upper airway infections, especially where AMR is observed, however, there are some limitations to the ex vivo models. Despite similar nutrient cues being used, nutrient availability may differ due to the lack of thick mucus. The physical surface structures of the tissue may differ slightly, especially if it were to degrade during infection which might not be observed during in vivo infection of a cystic fibrosis lung.
When considering the use of manuka honey for the treatment of pulmonary infections, ease of application, subsequent reapplications and potential diluting effects are difficult to predict. During interaction studies, multiple sub-inhibitory concentrations of manuka honey were used, allowing dilution effects to be identified. Not only does this allow the efficacy of the manuka honey to be determined at sub-inhibitory concentrations when combined with antibiotics, but identifies any potential problems arising from these combinations. It is critical to take such observations into consideration before progressing into a clinical setting as many of these patients will be undergoing extensive antimicrobial therapy. The specific instances of antagonism at low sub-inhibitory concentrations, is most likely due to the sugars acting as a carbon source, therefore inhibitory concentrations must be maintained. The use of doubling dilutions might have exacerbated this, with previous studies (Roberts et al., 2012; Jenkins et al., 2015) opting for minor percentage reductions in concentrations which does not necessarily convey the range of dilutions that may be encountered in vivo. As the reference panel used within this study covers the wide strain diversity seen for P. aeruginosa from those that are highly transmissible to those that are extremely virulent, effects against individual strains should also be considered in addition to the overarching effects on the species as a whole. For instance, the synergism of some manuka honey and antibiotic combinations against specific antimicrobial resistant strains (e.g., ciprofloxacin and tobramycin against PaRP isolate #41) suggests combinational therapy could have potential therapeutic benefit, albeit under stringent conditions and may be of benefit where other treatment options fail.
In light of increasing AMR, repurposing antimicrobial agents may provide new treatment options for difficult to treat infections. In this study we have demonstrated the antibacterial and anti-biofilm effects of manuka honey (above the effect seen with antibiotics when used alone) against P. aeruginosa grown in two ex vivo models, suggesting potential for manuka honey to be re-purposed from its original use as a wound dressing. During routine clinical practice, biofilm-based methods for antimicrobial testing are not used, primarily due to a lack of relevance when it comes to patient prognosis (Coenye et al., 2018), however, they are useful in academic research for the identification of anti-biofilm effects, especially when they better replicate chronic infections and their associated challenges (Waters and Ratjen, 2017). Whilst the anti-bacterial efficacy of manuka honey in an ex vivo biofilm model simulating chronic lung infections has been demonstrated, an obvious limitation when it comes to clinical applicability of manuka honey for the treatment of pulmonary infection is delivery. Firstly, Nebulisation of antimicrobials can be both difficult and inefficient, with lower final concentrations (13–64% of the original dose) in the lung environment (Bassetti et al., 2016), therefore manuka honey is unlikely to reach the lung at 64% (w/v) in its current formulation. Concentrations in the region of 40% (w/v) could be achievable; however, efficacy at this concentration needs to be experimentally tested. This potentially brings its own challenges as manuka honey can exert in vitro toxicity on human cells (Yabes et al., 2017). The differences between EVPL bronchiole tissue in the presence of 64% (w/v) manuka honey may be subject to these similar “in vitro” effects, however, the negative effects observed in vitro do not necessarily occur in vivo whereby 100% manuka honey is used on open wounds with positive effects, increasing wound healing (Mohamed et al., 2015). Alternatively higher concentration of manuka honey could be readily incorporated into a sinus rinse solution and be used to clear sino-nasal cavity infection reservoirs, preventing the migration of bacteria from the upper airway into the lung (Siegel and Weiser, 2015), and affording terminal CF patients the opportunity to undergo lifesaving lung transplantations.
Author Contributions
AR and RJ conceived the design of the study, analyzed the data, and wrote the manuscript. AR, LP, and MP carried out the experimental work. LP, MP, DT, and RJ contributed to the final preparation of the manuscript.
Funding
This work was funded by the (Jane) Hodge Foundation (Awarded to RJ in November 2014) and Cardiff Metropolitan University’s Research and Enterprise Investment Fund (Awarded to AR and RJ in November 2015).
Conflict of Interest Statement
The authors declare that the research was conducted in the absence of any commercial or financial relationships that could be construed as a potential conflict of interest.
Acknowledgments
We thank Integra Lifesciences for the gift of Medihoney used within this study, Eshwar Mahenthiralingam (Cardiff University) for the donation of strains, and Freya Harrison (Warwick University) for advice with the EVPL model systems.
Supplementary Material
The Supplementary Material for this article can be found online at: https://www.frontiersin.org/articles/10.3389/fmicb.2019.00869/full#supplementary-material
References
Bassetti, M., Luyt, C., Nicolau, D. P., and Pugin, J. (2016). Characteristics of an ideal nebulized antibiotic for the treatment of pneumonia in the intubated patient. Ann. Intensive Care 6, 1–8. doi: 10.1186/s13613-016-0140-x
Coenye, T., Goeres, D., Van Bambeke, F., and Bjarnsholt, T. (2018). Should standardized susceptibility testing for microbial biofilms be introduced in clinical practice? Clin. Microbiol. Infect. 24, 570–572. doi: 10.1016/j.cmi.2018.01.003
Cystic Fibrosis Trust (2009). Antibiotic Treatment for Cystic Fibrosis, 3rd Edn. London: Cystic Fibrosis Trust.
de Bentzmann, S., and Plésiat, P. (2011). The Pseudomonas aeruginosa opportunistic pathogen and human infections. Environ. Microbiol. 13, 1655–1665. doi: 10.1111/j.1462-2920.2011.02469.x
De Soyza, A., Hall, A. J., Mahenthiralingam, E., Drevinek, P., Kaca, W., Drulis-Kawa, Z., et al. (2013). Developing an international Pseudomonas aeruginosa reference panel. Microbiologyopen 2, 1010–1023. doi: 10.1002/mbo3.141
El Zowalaty, M. E., Al Thani, A. A., Webster, T. J., El Zowalaty, A. E., Schweizer, H. P., Nasrallah, G. K., et al. (2015). Pseudomonas aeruginosa: arsenal of resistance mechanisms, decades of changing resistance profiles, and future antimicrobial therapies. Future Microbiol. 10, 1683–1706. doi: 10.2217/fmb.15.48
European Committee for Antimicrobial Susceptibilty Testing (2003). Determination of minimum inhibitory concentrations (MICs) of antibacterial agents by broth dilution. Clin. Microbiol. Infect. 9, ix–xv. doi: 10.1046/j.1469-0691.2003.00790.x
Halstead, F. D., Rauf, M., Moiemen, N. S., Bamford, A., Wearn, C. M., Fraise, A. P., et al. (2015). The antibacterial activity of acetic acid against biofilm-producing pathogens of relevance to burns patients. PLoS One 10:e0136190. doi: 10.1371/journal.pone.0136190
Harrison, F., and Diggle, S. P. (2016). An ex vivo lung model to study bronchioles infected with Pseudomonas aeruginosa biofilms. Microbiology 162, 1755–1760. doi: 10.1099/mic.0.000352
Harrison, F., Muruli, A., Higgins, S., and Diggle, S. P. (2014). Development of an ex vivo porcine lung model for studying growth, virulence, and signaling of Pseudomonas aeruginosa. Infect. Immun. 82, 3312–3323. doi: 10.1128/IAI.01554-14
Harrison, F., Roberts, A. E. L., Gabrilska, R., Rumbaugh, K. P., Lee, C., and Diggle, S. P. (2015). A 1,000-year-old antimicrobial remedy with antistaphylococcal activity. mBio 6:e1129-15. doi: 10.1128/mBio.01129-15
Høiby, N., Ciofu, O., and Bjarnsholt, T. (2010). Pseudomonas aeruginosa biofilms in cystic fibrosis. Future Microbiol. 5, 1663–1674. doi: 10.2217/fmb.10.125
Jenkins, R. E., Burton, N., and Cooper, R. A. (2011). Manuka honey inhibits cell division in methicillin-resistant Staphylococcus aureus. J. Antimicrob. Chemother. 66, 2536–2542. doi: 10.1093/jac/dkr340
Jenkins, R. E., and Cooper, R. A. (2012). Improving antibiotic activity against wound pathogens with manuka honey in vitro. PLoS One 7:e45600. doi: 10.1371/journal.pone.0045600
Jenkins, R. E., Wootton, M., Howe, R., and Cooper, R. A. (2015). A demonstration of the susceptibility of clinical isolates obtained from cystic fibrosis patients to manuka honey. Arch. Microbiol. 197, 597–601. doi: 10.1007/s00203-015-1091-6
Lee, K., and Yoon, S. S. (2017). Pseudomonas aeruginosa biofilm, a programmed bacterial life for fitness. J. Microbiol. Biotechnol. 27, 1053–1064. doi: 10.4014/jmb.1611.11056
Liu, M., Lu, J., Müller, P., Turnbull, L., Burke, C. M., Schlothauer, R. C., et al. (2015). Antibiotic-specific differences in the response of Staphylococcus aureus to treatment with antimicrobials combined with manuka honey. Front. Microbiol. 5:779. doi: 10.3389/fmicb.2014.00779
Liu, M. Y., Cokcetin, N. N., Lu, J., Turnbull, L., Carter, D. A., Whitchurch, C. B., et al. (2018). Rifampicin-manuka honey combinations are superior to other antibiotic-manuka honey combinations in eradicating Staphylococcus aureus biofilms. Front. Microbiol. 8:2653. doi: 10.3389/fmicb.2017.02653
López-Causapé, C., de Dios-Caballero, J., Cobo, M., Escribano, A., Asensio,Ó, Oliver, A., et al. (2017). Antibiotic resistance and population structure of cystic fibrosis Pseudomonas aeruginosa isolates from a Spanish multi-centre study. Int. J. Antimicrob. Agents 50, 334–341. doi: 10.1016/j.ijantimicag.2017.03.034
Lu, J., Turnbull, L., Burke, C. M., Liu, M., Carter, D. A., Schlothauer, R. C., et al. (2014). Manuka-type honeys can eradicate biofilms produced by Staphylococcus aureus strains with different biofilm-forming abilities. PeerJ 2:e326. doi: 10.7717/peerj.326
Lyczak, J. B., Cannon, C. L., and Pier, G. B. (2002). Lung infections associated with cystic fibrosis. Clin. Microbiol. Rev. 15, 194–222. doi: 10.1128/CMR.15.2.194-222.2002
Maddocks, S. E., and Jenkins, R. E. (2013). Honey: a sweet solution to the growing problem of antimicrobial resistance? Future Microbiol. 8, 1419–1429. doi: 10.2217/fmb.13.105
Maddocks, S. E., Lopex, M. S., Rowlands, R. S., and Cooper, R. A. (2012). Manuka honey inhibits the development of Streptococcus pyogenes biofilms and causes reduced expression of two fibronectin binding proteins. Microbiology 158, 781–790. doi: 10.1099/mic.0.053959-0
Mandal, M. D., and Mandal, S. (2011). Honey: its medicinal property and antibacterial activity. Asian Pac. J. Trop. Biomed. 1, 154–160. doi: 10.1016/S2221-1691(11)60016-6
Mohamed, H., Salma, M. A., Lenjawi Al, B., Abdi, S., Gouda, Z., Barakat, N., et al. (2015). The efficacy and safety of natural honey on the healing of foot ulcers: a case series. Wounds 27, 103–114.
Molan, P. C. (2016). The evidence supporting the use of honey as a wound dressing. Int. J. Low. Extrem. Wounds 5, 40–54. doi: 10.1177/1534734605286014
Moloney, M. G. (2016). Natural products as a source for novel antibiotics. Trends Pharmacol. Sci. 37, 689–701. doi: 10.1016/j.tips.2016.05.001
Odds, F. C. (2003). Synergy, antagonism, and what the chequerboard puts between them. J. Antimicrob. Chemother. 52:1. doi: 10.1093/jac/dkg301
Palmer, K. L., Aye, L. M., and Whiteley, M. (2007). Nutritional cues control Pseudomonas aeruginosa multicellular behavior in cystic fibrosis sputum. J. Bacteriol. 189, 8079–8087. doi: 10.1128/JB.01138-07
Palmer, K. L., Mashburn, L. M., Singh, P. K., and Whiteley, M. (2005). Cystic fibrosis sputum supports growth and cues key aspects of Pseudomonas aeruginosa physiology. J. Bacteriol. 187, 5267–5277. doi: 10.1128/JB.187.15.5267-5277.2005
Roberts, A. E. L., Kragh, K. N., Bjarnsholt, T., and Diggle, S. P. (2015). The limitations of in vitro experimentation in understanding biofilms and chronic infection. J. Mol. Biol. 427, 3646–3661. doi: 10.1016/j.jmb.2015.09.002
Roberts, A. E. L., Maddocks, S. E., and Cooper, R. A. (2012). Manuka honey is bactericidal against Pseudomonas aeruginosa and results in differential expression of oprF and algD. Microbiology 158, 3005–3013. doi: 10.1099/mic.0.062794-0
Schelstraete, P., Haerynck, F., Van daele, S., Deseyne, S., and De Baets, F. (2013). Eradication therapy for Pseudomonas aeruginosa colonization episodes in cystic fibrosis patients not chronically colonized by P. aeruginosa. J. Cyst. Fibros. 12, 1–8. doi: 10.1016/j.jcf.2012.07.008
Siegel, S. J., and Weiser, J. N. (2015). Mechanisms of bacterial colonization of the respiratory tract. Annu. Rev. Microbiol. 69, 425–444.
Waters, V., and Ratjen, F. (2017). Standard Versus Biofilm Antimicrobial Susceptibility Testing to Guide Antibiotic Therapy in Cystic Fibrosis. Hoboken, NJ: John Wiley & Sons Ltd.
White, R. (2016). Manuka honey in wound management: greater than the sum of its parts? J. Wound Care 25, 539–543. doi: 10.12968/jowc.2016.25.9.539
Worthington, R. J., and Melander, C. (2013). Combination approaches to combat multidrug-resistant bacteria. Trends Biotechnol. 31, 177–184. doi: 10.1016/j.tibtech.2012.12.006
Keywords: Pseudomonas aeruginosa, biofilms, antimicrobial susceptibility testing, manuka honey, ex vivo model, cystic fibrosis
Citation: Roberts AEL, Powell LC, Pritchard MF, Thomas DW and Jenkins RE (2019) Anti-pseudomonad Activity of Manuka Honey and Antibiotics in a Specialized ex vivo Model Simulating Cystic Fibrosis Lung Infection. Front. Microbiol. 10:869. doi: 10.3389/fmicb.2019.00869
Received: 12 September 2018; Accepted: 04 April 2019;
Published: 24 April 2019.
Edited by:
Yuji Morita, Meiji Pharmaceutical University, JapanReviewed by:
Freya Harrison, University of Warwick, United KingdomDee Carter, University of Sydney, Australia
Copyright © 2019 Roberts, Powell, Pritchard, Thomas and Jenkins. This is an open-access article distributed under the terms of the Creative Commons Attribution License (CC BY). The use, distribution or reproduction in other forums is permitted, provided the original author(s) and the copyright owner(s) are credited and that the original publication in this journal is cited, in accordance with accepted academic practice. No use, distribution or reproduction is permitted which does not comply with these terms.
*Correspondence: Aled E. L. Roberts, YWxlZC5yb2JlcnRzQHN3YW5zZWEuYWMudWs=