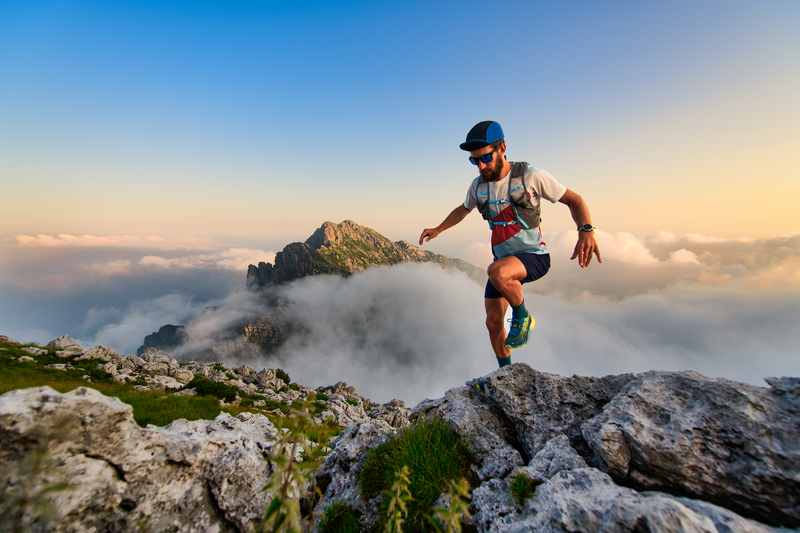
94% of researchers rate our articles as excellent or good
Learn more about the work of our research integrity team to safeguard the quality of each article we publish.
Find out more
ORIGINAL RESEARCH article
Front. Microbiol. , 24 April 2019
Sec. Extreme Microbiology
Volume 10 - 2019 | https://doi.org/10.3389/fmicb.2019.00839
This article is part of the Research Topic Living with Salt: Genetics and Ecology of Halophiles View all 17 articles
Halophilic methanogens play an important role in the carbon cycle in hypersaline environments, but are under-represented in culture collections. In this study, we describe a novel Methanohalophilus strain that was isolated from the sulfide-rich brine-seawater interface of Kebrit Deep in the Red Sea. Based on physiological and phylogenomic features, strain RSK, which is the first methanogenic archaeon to be isolated from a deep hypersaline anoxic brine lake of the Red Sea, represents a novel species of this genus. In order to compare the genetic traits underpinning the adaptations of this genus in diverse hypersaline environments, we sequenced the genome of strain RSK and compared it with genomes of previously isolated and well characterized species in this genus (Methanohalophilus mahii, Methanohalophilus halophilus, Methanohalophilus portucalensis, and Methanohalophilus euhalobius). These analyses revealed a highly conserved genomic core of greater than 93% of annotated genes (1490 genes) containing pathways for methylotrophic methanogenesis, osmoprotection through salt-out strategy, and oxidative stress response, among others. Despite the high degree of genomic conservation, species-specific differences in sulfur and glycogen metabolisms, viral resistance, amino acid, and peptide uptake machineries were also evident. Thus, while Methanohalophilus species are found in diverse extreme environments, each genotype also possesses adaptive traits that are likely relevant in their respective hypersaline habitats.
Hydrogenotrophic, methylotrophic and acetoclastic pathways are the major pathways for methanogenesis – the production of methane by microbial cells (Ferry and Kastead, 2007). Methanogenic species thriving in hypersaline environments have only been reported from the order Methanosarcinales and the recently described “Methanonatronarchaeia” (Sorokin et al., 2017) that branch deeply within the Euryarchaeota. Halophilic methylotrophic methanogens belonging to the order Methanosarcinales use the classical methylotrophic pathway where methylated compounds are dismutated to methane and CO2, whereas “Methanonatronarchaeia” use a heterotrophic methylotrophic pathway in which methylated compounds are used as electron acceptors and formate or hydrogen are being used as electron donors (Sorokin et al., 2017).
In hypersaline environments, the salinity and additional factors such as redox potential, permanency of anaerobic conditions, and the concentrations of other terminal electron acceptors determine the distribution and energy-efficiency of the prevailing methanogenic pathways. In these habitats, methylotrophic methanogens are more successful compared to hydrogenotrophic and acetoclastic methanogens due to their higher energy yield and the use of non-competitive substrates, such as methylamines and methanol (McGenity, 2010; Lazar et al., 2011; Oren, 2011; Kelley et al., 2012). In order to keep the cell cytoplasm iso-osmotic at elevated salt concentrations, both organic osmolytes (“salt-out” strategy) as well as the accumulation of potassium ion (“salt-in” strategy) are used for osmotic balance in Methanosarcinales (Lai and Gunsalus, 1992; Sowers and Gunsalus, 1995), while accumulation of potassium alone (“salt-in” strategy) is used to achieve an osmotic equilibrium in the “Methanonatronarchaeia” (Sorokin et al., 2017).
The majority of cultivated halophilic methylotrophic methanogens within the order Methanosarcinales belong to the genus Methanohalophilus, which currently comprises five described species: Methanohalophilus halophilus isolated from Shark Bay in Australia (Zhilina, 1983), Methanohalophilus euhalobius (Obraztsova et al., 1987), Methanohalophilus mahii isolated from the Great Salt Lake in Utah, United States (Paterek and Smith, 1988), Methanohalophilus portucalensis isolated from a salt pan in Portugal (Boone et al., 1993), and Methanohalophilus levihalophilus isolated from a natural gas-bearing deep aquifer (Katayama et al., 2014). The methanogens of the genus Methanohalophilus are unique among the halophilic methanogens with respect to their ability to grow in a wide range of salinities (1.5–20% NaCl). All known members of this genus are mesophilic, neutrophilic, obligate methylotrophic methanogens, except for the recently described M. levihalophilus, which appears to be only slightly halophilic, growing optimally at NaCl concentration around 2%.
Deep-sea hypersaline anoxic basins (DHABs) are polyextreme brines that so far have been found only on the seafloors of the Mediterranean Sea, the Gulf of Mexico, and the Red Sea. Biologically produced methane is an important metabolite in the DHABs (Borin et al., 2009) and marker gene sequences that are closely related to those of Methanohalophilus have been commonly found in deep-sea hypersaline environments (La Cono et al., 2011; Yakimov et al., 2013; Guan et al., 2015). One methanogenic strain, Methanohalophilus sp. TA21, has been isolated from Lake Thetis in the Eastern Mediterranean Sea (Cono et al., 2015). This strain performs methanogenesis from methylotrophic substrates arising from the catabolism of glycine betaine by other microbial consortia in the brine.
In several deep-sea brine habitats of the Red Sea, Methanosarcinales have been identified as the main methanogens based on genetic markers (Guan et al., 2015), but our knowledge of Methanohalophilus in the Red Sea is limited to the presence, distribution and relative abundance of marker gene sequences (Guan et al., 2015). In this study, we describe the isolation and the physiological characteristics of the first strain affiliated with this genus from the brine-sea water interface of Kebrit Deep together with its genome sequence to obtain a better understanding of its metabolic potential. Additionally, we describe comparative genome analyses with multiple, closely related Methanohalophilus species, providing insights into their life styles and adaptive strategies to different harsh environments.
The samples of the brine-seawater interface (BSI) and the brine were collected by Dr. André Antunes from of the Kebrit Deep (24° 43′ 28″N, 36° 16′ 36″E) by means of a rosette sampler at depths of 1,467 and 1,490 m below the sea surface during the R/V Aegaeo Red Sea Expedition in November 2011. At the time of sampling, the methane concentration was 1293 μM and temperatures ranged between 21.9 and 23.4°C, while salinity levels were between 18.2 and 26% (Guan et al., 2015; Ngugi et al., 2015).
Enrichments were initiated by adding 5 ml of Kebrit Deep samples to anaerobic serum vials that contained 45 ml of medium containing 50 μM trimethylamine (TMA) and a bicarbonate buffer system previously used to isolate marine methanogens (Sowers and Ferry, 1983; Sowers et al., 1984). The cultures were incubated in the dark at either 22, 30, or 37°C. Enrichment cultures were screened after approximately 4 weeks of incubation for methane production (using Agilent 6890 Gas Chromatography/Mass Spectrometry, Agilent, Santa Clara, CA, United States) and increase in turbidity (measuring optical density at 600 nm, SpectraMax, Molecular Devices, San Jose, CA, United States), and, if positive, further isolation of single colonies on agar plates (using the same medium, incubated under anoxic conditions) resulted in the isolation of strain RSK. The purity of the culture was tested by ensuring cells of uniform morphology with light microscopy as well as sequencing of 16S rRNA genes, and finally confirmed by the analyses of the genome sequences of the cultures.
The effect of different salinity ranges on growth of strain RSK was tested in the above-mentioned enrichment media containing NaCl in 1% increments ranging from 0 to 30%. Tests were performed in triplicate in Hungate anaerobic culture tubes as described previously (Sowers et al., 1984; L’Haridon et al., 2014), and growth was evaluated by measuring absorbance of the cultures at 600 nm. The range of temperatures supporting growth and the optimal growth temperature of strain RSK was tested at 4, 15, 22, 30, 33, 35, 37, 40, 45, and 50°C. The pH range for growth was examined between pH 5 and pH 9 with increments of 0.5. Formate (50 mM), acetate (90 mM), methanol (150 mM), H2:CO2 (4:1 v/v), and methylated substrates (mono-, di-, and tri-methylamine at 50 mM each, betaine at 5 mM each) were tested as potential growth substrates. The effects of the addition of vitamins biotin, folic acid, vitamin B12, pyridoxine-HCl, thiamine-HCl, riboflavin, nicotinic acid, pantothenate, p-aminobenzoic acid, lipoic acid with concentrations used previously (Sowers et al., 1984; Wolfe, 2011), and stimulatory compounds (e.g., yeast extract, peptone tested in three final concentrations 0.05, 1, and 2 g/L) on growth parameters of strain RSK were also tested. Inhibition of growth by antibiotics (ampicillin, kanamycin, carbenicillin, penicillin G, chloramphenicol, rifampicin, and vancomycin at final concentrations of 100 μg per ml) was examined with 50 μM TMA as the substrate.
For imaging using Transmission Electron Microscopy (TEM), cells in exponential phase growing with TMA as substrate were fixed by adding 2.5% glutaraldehyde directly to the growth medium. Transmission electron micrographs were obtained in the Imaging and Characterization Core Lab at King Abdullah University of Science and Technology (KAUST) using a Titan G2 80–300 kV TEM (FEI). The TEM was equipped with a 4 k × 4 k CCD camera (US4000) and an energy filter, model GIF Tridiem (Gatan, Inc., Germany). Cyro-scanning electron microscopy was performed using a PP2000T cryo-transfer system (Qurorum Technologies) attached to an FEI Nova Nano630 scanning electron microscope equipped with a field emission electron source and through-the-lens electron detectors.
Genomic DNA of strain RSK grown with TMA was extracted and purified using the DNeasy Blood & Tissue Kit (Qiagen, Hilden, Germany) following the manufacturer’s protocols. Genomic DNA of M. euhalobius (DSM 10369), M. halophilus (DSM 3094), and M. portucalensis (DSM 7471) was provided by DSMZ (German Collection of Microorganisms and Cell Cultures). Sequencing libraries were prepared with 1 μg genomic DNA using the Illumina TruSeqTM DNA sample preparation kit. Sequencing was done using an Illumina MiSeq sequencer at KAUST’s Bioscience Core Laboratory following Illumina’s protocols. Reads were quality filtered, trimmed, and assembled into contigs using the de novo assembler SPAdes, version 2.5.1 (Bankevich et al., 2012).
Putative coding sequences (CDSs) of all draft genomes were predicted using the automated annotation pipeline INDIGO1 (Alam et al., 2013). Clustered Regularly Interspaced Short Palindromic Repeats (CRISPR) loci, CRISPR direct repeat elements (DR), and spacers were detected by CRISPRFinder online server (Grissa et al., 2007). Small CRISPRs (e.g., CRISPR loci with less than three DRs) were marked as questionable CRISPRs. CRISPRmap (Lange et al., 2013) was used to classify the class assignment and structure motifs of DRs.
The relatedness of the organisms at the genome level was assessed using the average nucleotide identity (ANI) metric and the overall gene order conservation (synteny) as described in Ngugi et al. (2015). The complete genome of M. mahii (Spring et al., 2010) was used as a reference for the comparative analysis. To synchronize the genome annotation, the published genome of M. mahii was re-annotated using the INDIGO pipeline (Alam et al., 2013). Comparative genomics was conducted using the phylogenomic pipeline EDGAR (Blom et al., 2009), as described in Ngugi et al. (2015).
Publicly available genomes representing all methanogenic archaea as well as a few Thermoplasmata species were selected for a comprehensive phylogenetic analysis of the genus Methanohalophilus and downloaded from GenBank2. Subsequently, AMPHORA2 (Wu and Scott, 2012) was used to identify a total of 77 single conserved marker genes for phylogenomic reconstruction. The phylogeny was inferred by a maximum likelihood approach with Le Gascuel’s substitution model under 100 bootstrap replicates implemented in PHYML as implemented in Geneious R9 Biomatters, Ltd.; (Kearse et al., 2012).
The draft genome sequences of strain RSK, M. portucalensis, M. halophilus, and M. euhalobius are deposited in Genbank under BioProject identifier PRJNA500754. These genome drafts of M. portucalensis and M. halophilus presented here were used in the publications by L’Haridon et al. (2017, 2018).
At the time of sampling, remarkably high concentrations of methane (1293 μM), H2S (149.8 μM), and CO2 (4630 μM) were observed in Kebrit Deep BSI relative to other deep-sea brines of the Red Sea (Guan et al., 2015). Marker gene profiling suggested that sulfate-reducing bacteria and methylotrophic methanogenic Methanohalophilus populations co-inhabit this location, possibly because they do not compete for energy substrates (Lovley et al., 1982; Oremland and Polcin, 1982; McGenity, 2010; Guan et al., 2015). Strain RSK was isolated from an enrichment culture inoculated with brine-seawater interface samples from Kebrit Deep (1,468 meters below the sea surface) in the Red Sea. This strain represents the first methanogenic culture from the deep-sea brines of the Red Sea.
Strain RSK utilizes mono-, di-, and trimethylamine as well as methanol as energy sources, but no growth was observed when hydrogen (head space H2:CO2, 4:1 v/v), formate or acetate were used as substrates. Strain RSK was able to grow in a broad salinity range (2–20% NaCl), which is the widest among all described Methanohalophilus species so far (Table 1). Methane was still produced at 25% NaCl although growth was not observed. The temperature range for growth was 15–40°C. No growth was observed at 45°C and above over a period of 6 months. Optimal growth was observed in a medium containing 5 to 10% NaCl, 33°C and a pH of 6.5. Vitamins, yeast extract and trypticase did not stimulate growth. RSK was resistant to ampicillin, kanamycin, carbenicillin, penicillin G, and vancomycin, while chloramphenicol and rifampicin inhibited its growth (as determined by OD600, Table 1).
RSK cells growing with trimethylamine were non-motile, mostly regular cocci ranging from 0.3 to 2.5 μm in diameter. Single cells, pairs, or small aggregates were detected. Small nubs on the cell surface were observed, which could be extracellular vesicles or buddings due to their sizes and bud-like morphological characteristics (Choi et al., 2015; Figure 1). Compared to previously described Methanohalophilus species (Spring et al., 2010; Katayama et al., 2014), regular cocci-shaped cells and nubs are two distinct cellular features of strain RSK that have not been reported from other strains.
Figure 1. Transmission electron microscopy (TEM) micrographs of cell aggregates of strain RSK. (A) Magnification 30844 x, (B) Magnification 15759 x.
An overview of genomic characteristics of five Methanohalophilus species studied here is summarized in Table 2. Based on one complete genome and four high-quality draft genomes with >99.5% estimated genome completeness, the average genome length of Methanohalophilus species is around 2.0 Mbp, making them the smallest genomes in the family Methanosarcinaceae [ranging from around 2.0 to 5.75 Mbp (Galagan et al., 2002)]. The DNA G+C content of the genomes of Methanohalophilus were almost identical at around 42%, while the numbers of predicted open-reading frames ranged from 1,990 to 2,124. All tRNAs for 20 common proteinogenic amino acids and pyrrolysine are present. Species of this genus are also very similar to each other at the 16S rRNA gene level (>99.4%), in their tetranucleotide signatures (>0.99%), and based on their genomic average nucleotide identities (ANI; 91.1–93.7%; Supplementary Table S1). Thus, based on an ANI threshold of ∼95%, which is currently being operationally used for species delineation (Konstantinidis and Tiedje, 2005), our results indicate that strain RSK represents a novel species in the genus Methanohalophilus. M. euhalobius is the most closely related species, based on phylogenomic analysis of 77 single conserved marker genes (Figure 2).
Figure 2. Phylogenomic analysis of strain RSK in relation to other Methanohalophilus spp. and other methanogens. The tree is rooted with sequences of Methanopyrus kanderi. Significance of branch support inferred using maximum likelihood (n = 100 bootstraps). Strain RSK is in bold. Methanosarcinales are shown in blue, Methanocellales are shown in gray, Methanomicrobiales are shown in green, Methanomassiliicoccales are shown in light blue, Methanobacteriales are shown in purple, Methanococcales are shown in ochre.
Complementary synteny-based comparative genomics analysis (Yelton et al., 2011) comparing the predicted proteome of strain RSK with that obtained from the completed genome of M. mahii shows that ∼77% of their core genes are syntenic, implying a high degree of functional conservation between these two species. We estimate that the core genome of strain RSK and closely related species of Methanohalophilus contains 1,490 protein-coding genes, accounting for 70 to 75% of their predicted proteomes (Figure 3), which is significantly larger than the core genome formed by including representatives of M. euhalobius recovered from hydraulically fractured rocks in unconventional reservoirs (Borton et al., 2018). Only 6.5–13.4% of all predicted proteins are unique to the five individual species compared in this study.
Figure 3. Comparative analysis of the predicted proteomes of Methanohalophilus species analyzed in EDGAR. The Venn diagram shows the core, unique, and flexible genome components among strains RSK (Red Sea Kebrit BSI isolate), M. mahii, M. portucalensis, M. euhalobius, and M. halophilus.
The genome of Methanohalophilus strain RSK is the first genome of methanogen from DHABs. It shares very similar metabolic capacities with closely related of this genus with respect to energy metabolism and strategies for oxidative and osmotic stress response. These genomes encode the complete set of enzymes for methanogenesis from methyl compounds but lack the hydrogenotrophy-associated hydrogenases, acetate kinase and acetate thiokinase encoding genes necessary growth with H2-CO2 and acetate, respectively (Ferry, 2010).
Methanogens inhabit a diverse array of ecosystems with a broad range of physicochemical conditions, including some habitats where oxygen is constantly present at low concentrations or is present in measurable concentrations at certain times, e.g., the BSIs of the deep-sea brines of the Red Sea and soil crusts (Antunes et al., 2011; Guan et al., 2015; Ngugi et al., 2015). However, high oxygen concentrations are usually restrictive to their anaerobic lifestyle and thus their distribution (Hoehler et al., 2010). Interestingly, Methanohalophilus species are commonly found in brine-seawater transition zones (La Cono et al., 2011; Yakimov et al., 2013, 2014; Guan et al., 2015), where micro-oxic conditions exist (Ngugi et al., 2015). Strain RSK was isolated from the BSI of Kebrit Deep, where the concentration of dissolved oxygen (DO2) was 4.6 μM. Thus, it must have developed mechanisms to protect themselves from the toxicity of DO2 and reactive oxygen species (ROS) and to repair damaged cellular compounds and enzymes. Accordingly, the genomes of strain RSK and type strains of Methanohalophilus encode up to eight copies of thioredoxin, up to six copies of different isozymes of catalase peroxidase, peroxiredoxin, alkyl-hydroperoxide reductase, and alkyl-hydroperoxidase, and three copies of rubrerythrin. Thioredoxin reductase, catalase, and superoxide reductase were also detected in each genome. Among the above mentioned enzymes encoded by Methanohalophilus genomes, thioredoxin reductase and thioredoxin together form the thioredoxin system which constitutes a key function in defense against oxidative stress in providing thioredoxin-based oxidative redox regulation (Lu and Holmgren, 2014; Susanti et al., 2014). The Methanohalophilus genomes encode alkyl-hydroperoxide reductase, peroxiredoxin, catalase, catalase peroxidase that could reduce hydrogen peroxide (Seaver and Imlay, 2001; Sakai et al., 2011), alkyl-hydroperoxidase and rubrerythrin, which could reduce peroxides (Sztukowska et al., 2002; Guimarães et al., 2005), and superoxide reductase, which could detoxify superoxide.
Glutaredoxins are small ubiquitous thiol-disulfide redox enzymes (Iversen et al., 2010). In the domain Bacteria, glutaredoxin is reduced by glutathione and diverse low-molecular-weight thiols (Holmgren, 1989; Van Laer et al., 2013). Prevailing evidence indicates that glutathione and glutaredoxins are unlikely participants in the oxidative stress response of methanogens (McCarver et al., 2017; Prakash et al., 2018). In Methanosarcina acetivorans, the annotated glutaredoxin enzyme (AAM05066.1, renamed methanoredoxin) utilizes coenzyme M and ferredoxin:disulfide reductase (Yenugudhati et al., 2015; Prakash et al., 2018). A glutaredoxin homolog, which is more similar to glutaredoxin from the domain Bacteria (30% identity, 71% coverage to glutaredoxin under the accession number Q9HU55.1 from Pseudomonas aeruginosa PAO1), is also present in each genome of Methanohalophilus reported here. Further biochemical characterization of this enzyme from Methanohalophilus would be necessary to identify its actual function.
Osmotic adaptation is one of the main factors that allow halophilic methanogens to thrive in hypersaline environments. All Methanohalophilus species compared in this study have the genomic capacity to perform both the “salt-in” and “compatible solute” strategies (Oren, 2011) to combat osmotic stress as described for M. mahii (Spring et al., 2010). The presence of a K+/Na+ symporter Ktr as well as multiple copies of the potassium uptake system Trk and the glycine betaine/L-proline transport system permease (ProW) in all analyzed genomes of Methanohalophilus indicate that the “salt-in” strategy is widely used by these methanogens to cope with salinity. Notably, all strains possess the potential to synthesize betaine (unique to this genus and Methanohalobium) by methylation of glycine (Chen et al., 2009; Lai and Lai, 2011; Borton et al., 2018) and to synthesize Nε-acetyl-β-lysine from lysine; the later trait was also detected in the genome of closely related halophilic methanogens of genus Methanococcoides (Guan et al., 2014) and several other methanogens (Schlegel and Müller, 2011).
Sulfite is toxic to methanogens as it inactivates the essential enzyme for methanogenesis, methyl-coenzyme M reductase (Mahlert et al., 2002). In the brine-seawater interface of Kebrit Deep, where strain RSK was isolated from, sulfite is likely generated due to the presence of oxygen (4.6 μM) and high sulfide concentrations (149.8 μM) (Ngugi et al., 2015). Methanohalophilus species have one to two copies of nitrite and sulfite reductase (anaerobic sulfite reductase, MhpRSK_00883) containing a dissimilatory sulfite reductase subunit A domain (DsrA, COG2221), which presumably helps to protect halophilic methanogens in sulfidic environments by reducing sulfite.
Methanohalophilus portucalensis it is the only genome predicted to have the complete set of genes to assimilate sulfate catalyzed by sulfate adenylyltransferase (CysD and CysN/C), 3′-phosphoadenosine 5′-phosphosulfate synthase (adenylylsulfate kinase, PAPSS) and phosphoadenosine phosphosulfate reductase, although this would require additional experimental verification. The other four Methanohalophilus species lack PAPSS, while sulfate adenylyltransferase was not detected in M. halophilus and M. euhalobius.
Members of the genus Methanohalophilus appear to have different genomic capacities for the uptake of amino acids and peptides. A predicted operon for peptide transport (Opp) (Goodell and Higgins, 1987) is found only in M. mahii, M. halophilus, and strain RSK, indicating that they can directly take up peptides as a nutrient source.
The livK genes, encoding a high-affinity branched-chain amino acid transport system, are only present in the genomes of M. mahii, M. portucalensis, and M. halophilus (M. euhalobius has only a truncated version of this gene). This periplasmic Leu/Ile/Val-binding protein serves as the primary receptor for the leucine transport system in Escherichia coli (Anderson and Oxender, 1977; Magnusson et al., 2004). In turn, this suggests variability in the ability of different Methanohalophilus members to accumulate exogenous branched-chain amino acids.
Glycogen is a reserve polysaccharide common in methanogens (Simpson and Whitman, 1993). Enzymes for glycogen metabolism (glycogen synthase, alpha-amylase, and glycogen debranching protein) are predicted to be present only in M. mahii and M. halophilus, suggesting the capacity of these species to synthesize and store glycogen.
As the most abundant biological entities on Earth, viruses are also present in the Deep-sea Anoxic Brines of the Red Sea (Antunes et al., 2015). Clustered regularly interspaced short palindromic repeats (CRISPR)-Cas systems provide prokaryotes with acquired, adaptive, and heritable resistance against foreign invading genetic elements such as viruses, phages, and plasmids (Barrangou et al., 2007; Horvath and Barrangou, 2010). Genes related to viral defense were previously detected in Methanohalophilus (Borton et al., 2018). In agreement with this report, we also found that strain RSK possesses complete CRISPR/Cas systems. Genomes of strain RSK and M. euhalobius carry two different Cas arrays – Type I and Type III-U, based on the classification proposed by Makarova et al. (2011). Both Type III-U Cas arrays exhibit high sequence similarities and high synteny. Similar Cas arrays could only be found in the genomes of Candidatus Methanoperedens nitroreducens and Methanospirillum hungatei so far, suggesting a novel variant of subtype III-U. This further indicates the uniqueness of this subtype in anaerobic methane metabolizing microbes. The CRISPR locus 2 in strain RSK and M. euhalobius share the same direct repeat element (GTTACCATTCCCTATTTTCTCGGGAGCTACTTTCAAC) (Supplementary Table S2), suggesting close evolutionary relationships in viral defense systems between these two species.
The 260 predicted species-specific genes in the genome of RSK encompass 15 COG categories and are overrepresented in categories “Replication, recombination and repair” (22 genes), “Cell wall/membrane/envelope biogenesis” (17 genes), “Inorganic ion transport and metabolism” (14 genes), “General function prediction only” (14 genes), and “coenzyme transport and metabolism” (12 genes; Supplementary Table S3).
The genome of RSK encodes an aquaporin homolog (RSK_01503) that is 54% identical (coverage 96%) to the aquaporin characterized from Methanothermobacter marburgensis str. Marburg (Kozono et al., 2003). This archaeal type of aquaporin showed characteristics of both, aquaporin and aquaglyceroporin, the two existing classes of this protein family (Araya-Secchi et al., 2011). The presence of this gene implies a role for water channel mediated osmotically driven water flux in strain RSK that might aid cells to cope with sudden changes in salinity in their habitat.
The genome of RSK carries putative genes that encode for membrane components of the ssu system, which is involved in sulfur assimilation from aliphatic sulfonates during sulfur-starvation conditions. In this system, the ssuABC gene products are involved in the import of aliphatic sulfonates while the ssuDE gene products form an oxygenase system responsible for releasing sulfur from aliphatic sulfonates (van Der Ploeg et al., 2001). Strain RSK also encodes for a putative aliphatic sulfonate-binding protein SsuA (RSK_00452), a probable aliphatic sulfonate transport permease SsuC (RSK_00453), and an aliphatic sulfonate import ATP-binding protein SsuB (RSK_00454). This suggests that RSK could take up organic sulfur, although the exact mechanism of desulfonation remains unknown.
Furthermore, a partial arsenite efflux protein ArsB that shares 59% amino acid sequence identity to that of Alkaliphilus metalliredigens QYMF (acc. A6TP80.1) (Fu et al., 2009) is also encoded in the genome of RSK (RSK_01501). If functional, it would allow RSK to confer resistance to toxic arsenite by pumping it out of the cells.
Methanogens are important players in the carbon cycle of hypersaline habitats. However, not much is known about the characteristics of methanogens from the deep-sea brine habitats of the Red Sea and no cultures existed. In this study, we describe a novel isolate (strain RSK) from the BSI of Kebrit Deep of the Red Sea representing a novel species within the genus Methanohalophilus. In order to understand why species of the genus Methanohalophilus are successful in chemically diverse hypersaline environments, we analyzed and compared the genomic inventory among five Methanohalophilus species to elucidate genomically conserved traits and underlying mechanisms for oxidative and osmotic adaptation. Their genomic toolboxes encompass versatile metabolic strategies potentially contributing to their osmo-adaptation in polyextreme hypersaline environments. We suggest that niche adaptation of Methanohalophilus populations is driven by their different genomic capacities in sulfur and glycogen metabolisms, viral resistance, amino acid and peptide uptake.
US conceived and designed the study. YG, DN, and MV, performed the analysis of the sequence data and bioinformatics tasks. JB and IA developed and maintained EDGAR and INDIGO bioinformatic platforms. JF designed the archaeal growth strategy. YG and SG performed the laboratory work. YG and US wrote the manuscript. YG, DN, JF, and US critically reviewed and edited the manuscript. All authors approved the final version of the manuscript.
This research was supported by baseline funding provided by King Abdullah University of Science and Technology and from the SEDCO Research Excellence Award to US. The funders had no role in study design, data collection, and analysis, decision to publish, or preparation of the manuscript.
The authors declare that the research was conducted in the absence of any commercial or financial relationships that could be construed as a potential conflict of interest.
The Supplementary Material for this article can be found online at: https://www.frontiersin.org/articles/10.3389/fmicb.2019.00839/full#supplementary-material
Alam, I., Antunes, A., Kamau, A. A., Ba alawi, W., Kalkatawi, M., Stingl, U., et al. (2013). INDIGO – integrated data warehouse of MIcrobial GenOmes with examples from the Red Sea extremophiles. PLoS One 8:e82210. doi: 10.1371/journal.pone.0082210
Anderson, J. J., and Oxender, D. L. (1977). Escherichia coli transport mutants lacking binding protein and other components of the branched-chain amino acid transport systems. J. Bacteriol. 130, 384–392.
Antunes, A., Alam, I., Simões, M. F., Daniels, C., Ferreira, A. J. S., Siam, R., et al. (2015). First Insights into the viral communities of the deep-sea aoxic brines of the Red Sea. Genom. Proteom. Bioinform. 13, 304–309. doi: 10.1016/j.gpb.2015.06.004
Antunes, A., Ngugi, D. K., and Stingl, U. (2011). Microbiology of the Red Sea (and other) deep-sea anoxic brine lakes. Environ. Microbiol. Rep. 3, 416–433. doi: 10.1111/j.1758-2229.2011.00264.x
Araya-Secchi, R., Garate, J. A., Holmes, D. S., and Perez-Acle, T. (2011). Molecular dynamics study of the archaeal aquaporin AqpM. BMC Genomics 12(Suppl. 4):S8. doi: 10.1186/1471-2164-12-S4-S8
Bankevich, A., Nurk, S., Antipov, D., Gurevich, A. A., Dvorkin, M., Kulikov, A. S., et al. (2012). SPAdes: a new genome assembly algorithm and its applications to single-cell sequencing. J. Comput. Biol. 19, 455–477. doi: 10.1089/cmb.2012.0021
Barrangou, R., Fremaux, C., Deveau, H., Richards, M., Boyaval, P., Moineau, S., et al. (2007). CRISPR provides acquired resistance against viruses in prokaryotes. Science 315:1712. doi: 10.1126/science.1138140
Blom, J., Albaum, S. P., Doppmeier, D., Pühler, A., Vorhölter, F.-J., Zakrzewski, M., et al. (2009). EDGAR: a software framework for the comparative analysis of prokaryotic genomes. BMC Bioinformatics 10:154. doi: 10.1186/1471-2105-10-154
Boone, D. R., Mathrani, I. M., Liu, Y., Menaia, J. A. G. F., Mah, R. A., and Boone, J. E. (1993). Isolation and characterization of Methanohalophilus portucalensis sp. nov. and DNA reassociation study of the genus Methanohalophilus. Int. J. Syst. Bacteriol. 43, 430–437. doi: 10.1099/00207713-43-3-430
Borin, S., Brusetti, L., Mapelli, F., D’Auria, G., Brusa, T., Marzorati, M., et al. (2009). Sulfur cycling and methanogenesis primarily drive microbial colonization of the highly sulfidic Urania deep hypersaline basin. Proc. Natl. Acad. Sci. U.S.A. 106, 9151–9156. doi: 10.1073/pnas.0811984106
Borton, M. A., Daly, R. A., O’Banion, B., Hoyt, D. W., Marcus, D. N., Welch, S., et al. (2018). Comparative genomics and physiology of the genus Methanohalophilus, a prevalent methanogen in hydraulically fractured shale. Environ. Microbiol. 20, 4596–4611. doi: 10.1111/1462-2920.14467
Chen, S.-Y., Lai, M.-C., Lai, S.-J., and Lee, Y.-C. (2009). Characterization of osmolyte betaine synthesizing sarcosine dimethylglycine N-methyltransferase from Methanohalophilus portucalensis. Arch. Microbiol. 191, 735–743. doi: 10.1007/s00203-009-0501-z
Choi, D. H., Kwon, Y. M., Chiura, H. X., Yang, E. C., Bae, S. S., Kang, S. G., et al. (2015). Extracellular vesicles of the hyperthermophilic archaeon “Thermococcus onnurineus” NA1T. Appl. Environ. Microbiol. 81, 4591–4599. doi: 10.1128/AEM.00428-15
Cono, V., Arcadi, E., Spada, G., Barreca, D., Laganà, G., Bellocco, E., et al. (2015). A three-component microbial consortium from deep-sea salt-saturated anoxic lake Thetis links anaerobic glycine betaine degradation with methanogenesis. Microorganisms 3, 500–517. doi: 10.3390/microorganisms3030500
Ferry, J. G. (2010). How to make a living by exhaling methane. Annu. Rev. Microbiol. 64, 453–473. doi: 10.1146/annurev.micro.112408.134051
Ferry, J. G., and Kastead, K. A. (2007). “Methanogenesis,” in Archaea: Molecular and Cellular Biology, ed. R. Cavicchioli (Washington, DC: ASM Press), 288–314. doi: 10.1128/9781555815516.ch13
Fu, H.-L., Meng, Y., Ordóñez, E., Villadangos, A. F., Bhattacharjee, H., Gil, J. A., et al. (2009). Properties of arsenite efflux permeases (Acr3) from Alkaliphilus metalliredigens and Corynebacterium glutamicum. J. Biol. Chem. 284, 19887–19895. doi: 10.1074/jbc.M109.011882
Galagan, J. E., Nusbaum, C., Roy, A., Endrizzi, M. G., Macdonald, P., FitzHugh, W., et al. (2002). The genome of M. acetivorans reveals extensive metabolic and physiological diversity. Genome Res. 12, 532–542. doi: 10.1101/gr.223902
Goodell, E. W., and Higgins, C. F. (1987). Uptake of cell wall peptides by Salmonella typhimurium and Escherichia coli. J. Bacteriol. 169, 3861–3865. doi: 10.1128/jb.169.8.3861-3865.1987
Grissa, I., Vergnaud, G., and Pourcel, C. (2007). CRISPRFinder: a web tool to identify clustered regularly interspaced short palindromic repeats. Nucleic Acids Res. 35, W52–W57. doi: 10.1093/nar/gkm360
Guan, Y., Hikmawan, T., Antunes, A., Ngugi, D., and Stingl, U. (2015). Diversity of methanogens and sulfate-reducing bacteria in the interfaces of five deep-sea anoxic brines of the Red Sea. Res. Microbiol. 166, 688–699. doi: 10.1016/j.resmic.2015.07.002
Guan, Y., Ngugi, D. K., Blom, J., Ali, S., Ferry, J. G., and Stingl, U. (2014). Draft genome sequence of an obligately methylotrophic methanogen, Methanococcoides methylutens, isolated from marine sediment. Genome Announc. 2:e1184-14. doi: 10.1128/genomeA.01184-14
Guimarães, B. G., Souchon, H., Honoré, N., Saint-Joanis, B., Brosch, R., Shepard, W., et al. (2005). Structure and mechanism of the alkyl hydroperoxidase AhpC, a key element of the Mycobacterium tuberculosis defense system against oxidative stress. J. Biol. Chem. 280, 25735–25742. doi: 10.1074/jbc.M503076200
Hoehler, T., Gunsalus, R. P., and McInerney, M. J. (2010). “Environmental constraints that limit methanogenesis,” in Handbook of Hydrocarbon and Lipid Microbiology, eds T. McGenity, J. R. van der Meer, and V. de Lorenzo (Berlin: Springer), 635–654. doi: 10.1007/978-3-540-77587-4_51
Horvath, P., and Barrangou, R. (2010). CRISPR/Cas, the immune system of bacteria and archaea. Science 327, 167–170. doi: 10.1126/science.1179555
Iversen, R., Andersen, P. A., Jensen, K. S., Winther, J. R., and Sigurskjold, B. W. (2010). Thiol-disulfide exchange between glutaredoxin and glutathione. Biochemistry 49, 810–820. doi: 10.1021/bi9015956
Katayama, T., Yoshioka, H., Mochimaru, H., Meng, X.-Y., Muramoto, Y., Usami, J., et al. (2014). Methanohalophilus levihalophilus sp. nov., a slightly halophilic, methylotrophic methanogen isolated from natural gas-bearing deep aquifers, and an emended description of the genus Methanohalophilus. Int. J. Syst. Evol. Microbiol. 64, 2089–2093. doi: 10.1099/ijs.0.063677-0
Kearse, M., Moir, R., Wilson, A., Stones-Havas, S., Cheung, M., Sturrock, S., et al. (2012). Geneious basic: an integrated and extendable desktop software platform for the organization and analysis of sequence data. Bioinformatics 28, 1647–1649. doi: 10.1093/bioinformatics/bts199
Kelley, C. A., Poole, J. A., Tazaz, A. M., Chanton, J. P., and Bebout, B. M. (2012). Substrate limitation for methanogenesis in hypersaline environments. Astrobiology 12, 89–97. doi: 10.1089/ast.2011.0703
Konstantinidis, K. T., and Tiedje, J. M. (2005). Genomic insights that advance the species definition for prokaryotes. Proc. Natl. Acad. Sci. U.S.A. 102, 2567–2572. doi: 10.1073/pnas.0409727102
Kozono, D., Ding, X., Iwasaki, I., Meng, X., Kamagata, Y., Agre, P., et al. (2003). Functional expression and characterization of an archaeal aquaporin. AqpM from Methanothermobacter marburgensis. J. Biol. Chem. 278, 10649–10656. doi: 10.1074/jbc.M212418200
La Cono, V., Smedile, F., Bortoluzzi, G., Arcadi, E., Maimone, G., Messina, E., et al. (2011). Unveiling microbial life in new deep-sea hypersaline Lake Thetis. Part I: prokaryotes and environmental settings. Environ. Microbiol. 13, 2250–2268. doi: 10.1111/j.1462-2920.2011.02478.x
Lai, M. C., and Gunsalus, R. P. (1992). Glycine betaine and potassium ion are the major compatible solutes in the extremely halophilic methanogen Methanohalophilus strain Z7302. J. Bacteriol. 174, 7474–7477. doi: 10.1128/jb.174.22.7474-7477.1992
Lai, S.-J., and Lai, M.-C. (2011). Characterization and regulation of the osmolyte betaine synthesizing enzymes GSMT and SDMT from halophilic methanogen Methanohalophilus portucalensis. PLoS One 6:e25090. doi: 10.1371/journal.pone.0025090
Lange, S. J., Alkhnbashi, O. S., Rose, D., Will, S., and Backofen, R. (2013). CRISPRmap: an automated classification of repeat conservation in prokaryotic adaptive immune systems. Nucleic Acids Res. 41, 8034–8044. doi: 10.1093/nar/gkt606
Lazar, C. S., Parkes, R. J., Cragg, B. A., L’Haridon, S., and Toffin, L. (2011). Methanogenic diversity and activity in hypersaline sediments of the centre of the Napoli mud volcano, Eastern Mediterranean Sea. Environ. Microbiol. 13, 2078–2091. doi: 10.1111/j.1462-2920.2011.02425.x
L’Haridon, S., Chalopin, M., Colombo, D., and Toffin, L. (2014). Methanococcoides vulcani sp. nov., a marine methylotrophic methanogen that uses betaine, choline and N,N-dimethylethanolamine for methanogenesis, isolated from a mud volcano, and emended description of the genus Methanococcoides. Int. J. Syst. Evol. Microbiol. 64, 1978–1983. doi: 10.1099/ijs.0.058289-0
L’Haridon, S., Corre, E., Guan, Y., Vinu, M., La Cono, V., Yakimov, M., et al. (2017). Complete genome sequence of methanohalophilus halophilus DSM 3094T, isolated from a cyanobacterial mat and bottom deposits at Hamelin Pool, Shark Bay, Northwestern Australia. Genome. Announc. 5:e1604-16. doi: 10.1128/genomeA.01604-16
L’Haridon, S., Corre, E., Guan, Y., Vinu, M., La Cono, V., Yakimov, M., et al. (2018). Complete genome sequence of the halophilic methylotrophic methanogen archaeon Methanohalophilus portucalensis strain FDF-1T. Genome Announc. 6:431. doi: 10.1128/genomeA.01482-17
Lovley, D. R., Dwyer, D. F., and Klug, M. J. (1982). Kinetic analysis of competition between sulfate reducers and methanogens for hydrogen in sediments. Appl. Environ. Microbiol. 43, 1373–1379.
Lu, J., and Holmgren, A. (2014). The thioredoxin antioxidant system. Free Radic. Biol. Med. 66, 75–87. doi: 10.1016/j.freeradbiomed.2013.07.036
Magnusson, U., Salopek-Sondi, B., Luck, L. A., and Mowbray, S. L. (2004). X-ray structures of the leucine-binding protein illustrate conformational changes and the basis of ligand specificity. J. Biol. Chem. 279, 8747–8752. doi: 10.1074/jbc.M311890200
Mahlert, F., Bauer, C., Jaun, B., Thauer, R. K., and Duin, E. C. (2002). The nickel enzyme methyl-coenzyme M reductase from methanogenic archaea: in vitro induction of the nickel-based MCR-ox EPR signals from MCR-red2. J. Biol. Inorgan. Chem. 7, 500–513. doi: 10.1007/s00775-001-0325-z
Makarova, K. S., Haft, D. H., Barrangou, R., Brouns, S. J. J., Charpentier, E., Horvath, P., et al. (2011). Evolution and classification of the CRISPR–Cas systems. Nat. Rev. Microbiol. 9, 467–477. doi: 10.1038/nrmicro2577
McCarver, A. C., Lessner, F. H., Soroeta, J. M., and Lessner, D. J. (2017). Methanosarcina acetivorans utilizes a single NADPH-dependent thioredoxin system and contains additional thioredoxin homologues with distinct functions. Microbiology 163, 62–74. doi: 10.1099/mic.0.000406
McGenity, T. J. (2010). “Methanogens and methanogenesis in hypersaline environments,” in Handbook of Hydrocarbon and Lipid Microbiology, eds T. McGenity, J. R. van der Meer, and V. de Lorenzo (Berlin: Springer), 665–680. doi: 10.1007/978-3-540-77587-4_53
Ngugi, D. K., Blom, J., Alam, I., Rashid, M., Ba-Alawi, W., Zhang, G., et al. (2015). Comparative genomics reveals adaptations of a halotolerant thaumarchaeon in the interfaces of brine pools in the Red Sea. ISME J. 9, 396–411. doi: 10.1038/ismej.2014.137
Obraztsova, A. Y., Bezrukova, V., Belyaev, S. S., and Shipin, O. V. (1987). Properties of the coccoid methylotrophic methanogen, Methanococcoides euhalobius sp. Mikrobiologiia 56, 523–527.
Oremland, R. S., and Polcin, S. (1982). Methanogenesis and sulfate reduction: competitive and noncompetitive substrates in estuarine sediments. Appl. Environ. Microbiol. 44, 1270–1276.
Oren, A. (2011). Thermodynamic limits to microbial life at high salt concentrations. Environ. Microbiol. 13, 1908–1923. doi: 10.1111/j.1462-2920.2010.02365.x
Parks, D. H., Imelfort, M., Skennerton, C. T., Hugenholtz, P., and Tyson, G. W. (2015). CheckM: assessing the quality of microbial genomes recovered from isolates, single cells, and metagenomes. Genome Res. 25, 1043–1055. doi: 10.1101/gr.186072.114
Paterek, J. R., and Smith, P. H. (1988). Methanohalophilus mahii gen nov., sp. nov., a methylotrophic halophilic methanogen. Int. J. Syst. Bacteriol. 38, 122–123. doi: 10.1099/00207713-38-1-122
Prakash, D., Walters, K. A., Martinie, R. J., McCarver, A. C., Kumar, A. K., Lessner, D. J., et al. (2018). Toward a mechanistic and physiological understanding of a ferredoxin:disulfide reductase from the domains archaea and bacteria. J. Biol. Chem. 293, 9198–9209. doi: 10.1074/jbc.RA118.002473
Sakai, S., Takaki, Y., Shimamura, S., Sekine, M., Tajima, T., Kosugi, H., et al. (2011). Genome sequence of a mesophilic hydrogenotrophic methanogen Methanocella paludicola, the first cultivated representative of the order Methanocellales. PLoS One 6:e22898. doi: 10.1371/journal.pone.0022898
Schlegel, K., and Müller, D. V. (2011). “Osmoadaptation in methanogenic archaea: physiology, genetics, and regulation in methanosarcina mazei gö1,” in Extremophiles Handbook, eds K. Horikoshi, G. Antranikian, A. T. Bull, F. T. Robb, and K. O. Stetter (Tokyo: Springer), 327–342.
Seaver, L. C., and Imlay, J. A. (2001). Alkyl hydroperoxide reductase is the primary scavenger of endogenous hydrogen peroxide in Escherichia coli. J. Bacteriol. 183, 7173–7181. doi: 10.1128/JB.183.24.7173-7181.2001
Simpson, P. G., and Whitman, W. B. (1993). “Anabolic pathways in methanogens,” in Methanogenesis: Chapman & Hall Microbiology Series (Physiology / Ecology / Molecular Biology / Biotechnology), ed. J. G. Ferry (Boston, MA: Springer). doi: 10.1007/978-1-4615-2391-8_11
Sorokin, D. Y., Makarova, K. S., Abbas, B., Ferrer, M., Golyshin, P. N., Galinski, E. A., et al. (2017). Discovery of extremely halophilic, methyl-reducing euryarchaea provides insights into the evolutionary origin of methanogenesis. Nat. Microbiol. 2:17081. doi: 10.1038/nmicrobiol.2017.81
Sowers, K. R., Baron, S. F., and Ferry, J. G. (1984). Methanosarcina acetivorans sp. nov., an acetotrophic methane-producing bacterium isolated from marine sediments. Appl. Environ. Microbiol. 47, 971–978.
Sowers, K. R., and Ferry, J. G. (1983). Isolation and characterization of a methylotrophic marine methanogen, Methanococcoides methylutens gen. nov., sp. nov. Appl. Environ. Microbiol. 45, 684–690.
Sowers, K. R., and Gunsalus, R. P. (1995). Halotolerance in Methanosarcina spp.: role of N(sup(epsilon))-acetyl-(beta)-lysine, (alpha)-glutamate, glycine betaine, and K+ as compatible solutes for osmotic adaptation. Appl. Environ. Microbiol. 61, 4382–4388.
Spring, S., Scheuner, C., Lapidus, A., Lucas, S., Glavina Del Rio, T., Tice, H., et al. (2010). The genome sequence of Methanohalophilus Mahii SLPT reveals differences in the energy metabolism among members of the Methanosarcinaceae inhabiting freshwater and saline environments. Archaea 2010:690737. doi: 10.1155/2010/690737
Susanti, D., Wong, J. H., Vensel, W. H., Loganathan, U., DeSantis, R., Schmitz, R. A., et al. (2014). Thioredoxin targets fundamental processes in a methane-producing archaeon, Methanocaldococcus jannaschii. Proc. Natl. Acad. Sci. U.S.A. 111, 2608–2613. doi: 10.1073/pnas.1324240111
Sztukowska, M., Bugno, M., Potempa, J., Travis, J., and Kurtz, D. M. (2002). Role of rubrerythrin in the oxidative stress response of Porphyromonas gingivalis. Mol. Microbiol. 44, 479–488. doi: 10.1046/j.1365-2958.2002.02892.x
van Der Ploeg, J. R., Eichhorn, E., and Leisinger, T. (2001). Sulfonate-sulfur metabolism and its regulation in Escherichia coli. Arch. Microbiol. 176, 1–8. doi: 10.1007/s002030100298
Van Laer, K., Hamilton, C. J., and Messens, J. (2013). Low-molecular-weight thiols in thiol-disulfide exchange. Antioxid. Redox Signal. 18, 1642–1653. doi: 10.1089/ars.2012.4964
Wilharm, T., Zhilina, T. N., and Hummel, P. (1991). DNA-DNA hybridization of methylotrophic halophilic methanogenic bacteria and transfer of Methanococcus halophilus vp to the genus Methanohalophilus as Methanohalophilus halophilus comb. nov. Int. J. Syst. Bacteriol. 41, 558–562. doi: 10.1099/00207713-41-4-558
Wolfe, R. S. (2011). “Techniques for cultivating methanogens,” in Methods in Enzymology, ed. J. Abelson (Amsterdam: Elsevier), 1–22.
Wu, M., and Scott, A. J. (2012). Phylogenomic analysis of bacterial and archaeal sequences with AMPHORA2. Bioinformatics 28, 1033–1034. doi: 10.1093/bioinformatics/bts079
Yakimov, M. M., La Cono, V., La Spada, G., Bortoluzzi, G., Messina, E., Smedile, F., et al. (2014). Microbial community of the deep-sea brine Lake Kryos seawater-brine interface is active below the chaotropicity limit of life as revealed by recovery of mRNA. Environ. Microbiol. 17, 364–382. doi: 10.1111/1462-2920.12587
Yakimov, M. M., La Cono, V., Slepak, V. Z., La Spada, G., Arcadi, E., Messina, E., et al. (2013). Microbial life in the Lake Medee, the largest deep-sea salt-saturated formation. Sci. Rep. 3:3554. doi: 10.1038/srep03554
Yelton, A. P., Thomas, B. C., Simmons, S. L., Wilmes, P., Zemla, A., Thelen, M. P., et al. (2011). A semi-quantitative, synteny-based method to improve functional predictions for hypothetical and poorly annotated bacterial and archaeal genes. PLoS. Comp. Biol. 7:e1002230. doi: 10.1371/journal.pcbi.1002230
Yenugudhati, D., Prakash, D., Kumar, A. K., Kumar, R. S. S., Yennawar, N. H., Yennawar, H. P., et al. (2015). Structural and biochemical characterizations of Methanoredoxin from Methanosarcina acetivorans, a glutaredoxin-like enzyme with coenzyme M-dependent protein disulfide reductase activity. Biochemistry 55, 313–321. doi: 10.1021/acs.biochem.5b00823
Keywords: deep-sea brine, red sea, methanogenic archaea, Methanohalophilus, comparative genomics
Citation: Guan Y, Ngugi DK, Vinu M, Blom J, Alam I, Guillot S, Ferry JG and Stingl U (2019) Comparative Genomics of the Genus Methanohalophilus, Including a Newly Isolated Strain From Kebrit Deep in the Red Sea. Front. Microbiol. 10:839. doi: 10.3389/fmicb.2019.00839
Received: 14 January 2019; Accepted: 02 April 2019;
Published: 24 April 2019.
Edited by:
Peter Dunfield, University of Calgary, CanadaReviewed by:
Mohamed Jebbar, Université de Bretagne Occidentale, FranceCopyright © 2019 Guan, Ngugi, Vinu, Blom, Alam, Guillot, Ferry and Stingl. This is an open-access article distributed under the terms of the Creative Commons Attribution License (CC BY). The use, distribution or reproduction in other forums is permitted, provided the original author(s) and the copyright owner(s) are credited and that the original publication in this journal is cited, in accordance with accepted academic practice. No use, distribution or reproduction is permitted which does not comply with these terms.
*Correspondence: Ulrich Stingl, dXN0aW5nbEB1ZmwuZWR1
†Present address: David K. Ngugi, Leibniz Institute DSMZ, Braunschweig, Germany
Disclaimer: All claims expressed in this article are solely those of the authors and do not necessarily represent those of their affiliated organizations, or those of the publisher, the editors and the reviewers. Any product that may be evaluated in this article or claim that may be made by its manufacturer is not guaranteed or endorsed by the publisher.
Research integrity at Frontiers
Learn more about the work of our research integrity team to safeguard the quality of each article we publish.