- State Key Laboratory of Animal Nutrition, College of Animal Science and Technology, China Agricultural University, Beijing, China
Microbial exposure during early life plays a pivotal role in modulating the health and intestinal development of the host. Our recent study showed that the low-birth-weight (LBW) piglets harbored a different fecal microbiota compared to normal-birth-weight (NBW) piglets during early life with a lower abundance of the genus Lactobacillus. Considering the spatial variations in gut microbiota at distinct gut locations, this study was designed to further investigate the differences in the microbiota composition and predominant Lactobacillus species in the ileum and colon between LBW and NBW piglets during early life, including day 7 (D7), day 21 (D21, before weaning), and day 35 (D35, 2 weeks after weaning). Compared with the normal group, LBW piglets harbored a significantly lower proportion of short-chain fatty acids producing microbes, such as Ruminococcaceae and Prevotellaceae in the ileum on D7, Alistipes and Lachnospiraceae in the colon on D7, Blautia in the colon on D21, and Ruminiclostridium 9 in the colon on D35. The relative abundance of the phylum Bacteroidetes was also declined in both ileum and colon of LBW piglets on D7. Meanwhile, the levels of total SCFAs on D7, D21, and D35, acetate and valerate on D7 and D21, propionate on D21, and lactate on D21 and D35, were also declined in the colon of LBW piglets. Moreover, functional alterations in the gut microbiota of LBW piglets were characterized by differentially abundant microbial genes involved in multiple pathways such as amino acid metabolism, energy metabolism, replication and repair, and metabolism of cofactors and vitamins in the colon. Additionally, lower numbers of L. salivarius on D7 and L. amylovorus on D21 resided in the colon of LBW piglets compared to those in the normal ones. Collectively, LBW piglets have altered bacterial communities, microbial metabolism and gene functions in the ileum and colon during early life, especially the colonic community. This work will help to develop novel ideas in identifying the reliable biomarkers affecting the gut microbiota development in LBW piglets during early life and facilitate the development of new nutritional interventions.
Introduction
Increased litter size by genetic selection for high-prolific sows in recent years has been accompanied by an increasing occurrence of piglets born with LBW (Li et al., 2017). LBW pigs refer to those pigs with a birth weight less than 1.1 kg, accounting for 15–25% of the newborn piglets (Wang et al., 2017). LBW piglets are more susceptible to high postnatal morbidity and mortality, postnatal growth restriction (Wu et al., 2006), as well as malfunction in vital organs like the GIT (Li et al., 2017; Wang et al., 2018). Previous studies have revealed a significant alteration of the small intestinal development in LBW fetuses (Wang et al., 2014) as well as LBW piglets at birth (Wang et al., 2008) and during lactation (Wang et al., 2010).
A highly diverse microbial consortium inhabits in the mammalian GIT and plays a vital role for their host (Rooks and Garrett, 2016). Early life colonization of microbes in the gut of the piglet sets the stages for adult microbiota and has lifelong influences on the development of the GIT and immune system (Matamoros et al., 2013; Houghteling and Walker, 2015). On the other hand, the gut microbiota of newborns is extremely unstable due to the fast growth of the GIT and its dysbiosis is closely connected with a higher risk of gut diseases and infections (Houghteling and Walker, 2015).
Several studies have investigated the differences in the early establishment of gut microbiota between LBW and NBW piglets. LBW piglets present a significantly distinct fecal bacterial community structure compared with NBW piglets during suckling and weaning periods (Li et al., 2018). D’Inca et al. (2010, 2011) showed that intrauterine growth restricted (IUGR) pigs with LBW had higher counts of adherent bacteria in ileal and colonic mucosa compared with the normal ones via a traditional colony-counting method. The distribution of gut microbes in pigs exhibits spatial heterogeneity across different intestinal segments, with Firmicutes and Proteobacteria dominating in the small intestine, while Firmicutes and Bacteroidetes dominate in the large intestine (Zhao et al., 2015; Mu et al., 2017). Therefore, it will be necessary to disclose the differences in the bacterial community between LBW and NBW piglets at distinct gut locations by using high-throughput 16S rRNA gene sequencing. In addition, the gut microbiota, particularly the microbiota in the hindgut, can ferment the carbohydrates to produce SCFAs, which are beneficial to the intestinal physiological functions (Koh et al., 2016). Our recent study showed the population of SCFAs-producing bacteria, including Prevotella spp. and Faecalibacterium spp., were markedly diminished in feces of the LBW piglets (Li et al., 2018), however, whether production of SCFAs in different gut segments of the LBW piglets is distinct from normal pigs requires further investigations.
Historically, Lactobacillus species, such as Lactobacillus amylovorus (L. amylovorus), Lactobacillus johnsonii (L. johnsonii), Lactobacillus mucosae (L. mucosae), and Lactobacillus salivarius (L. salivarius), have been widely considered to be the predominant endogenous probiotics in the GIT of pigs (Leser et al., 2002; Pieper et al., 2006; Houghteling and Walker, 2015). Our previous study has demonstrated that the proportion of the genus Lactobacillus was continuously lower in feces of the LBW piglets than that in NBW piglets during the first 35 days (Li et al., 2018). Further studies are needed to figure out the dynamic changes in dominant Lactobacillus species along the GIT in LBW and NBW piglets during their early life.
Therefore, the current study was designed to further characterize the differences in the development of microbiota assembly and predominant Lactobacillus species at distinct intestinal locations between LBW and NBW piglets during their early life, including the pre- and post-weaning periods. Moreover, dynamic shifts for production of the bacterial metabolites, including SCFAs and lactic acid, in these pigs were also clarified. The findings of this study are supposed to provide novel evidence for altered development of the gut microbiota in LBW piglets and promote the development of new probiotics for newborns with LBW.
Materials and Methods
Animals and Sample Collection
A total of 18 litters of full-term piglets (Landrace × Yorkshire) were selected from a commercial pig breeding farm in Mianyang city, Sichuan province, China. Only sows with 12∼14 live-born piglets were included and no cross-fostering was conducted in the present study. A NBW piglet was selected as a pig having a birth weight within 1 standard deviation (SD) of the mean birth weight of the whole litter, whereas a piglet with a birth weight 2 SD below the mean was labeled as a LBW littermate as we described previously (Wang et al., 2014). Average birth weights for all LBW and NBW piglets in the current study were 0.878 ± 0.044 and 1.434 ± 0.034 kg, respectively. Neonatal piglets were able to suckle the sow and drink water ad libitum, as well as started to receive a commercial creep feed from day 3 to 5 postpartum. All the piglets were weaned at day 21 and transferred into the nursery pens with free access to solid feed and water. No antibiotics or other drugs were used during the experiment. On day 7 (D7), day 21 (D21, before weaning), and day 35 (D35, 2 weeks after weaning) after birth, piglets (6 LBW and 6 NBW piglets) from randomly selected 6 litters were killed after anesthesia for sample collection. The digesta from the ileum and colon were immediately collected on ice, placed in liquid nitrogen, and then stored at -80°C until analysis.
DNA Extraction, 16S rRNA Gene Amplification and Sequencing
Total genomic DNA was extracted from 0.5 g of each specimen using the QIAamp® Fast DNA Stool Mini Kit (Qiagen Ltd., Germany) in accordance with the manufacturer’s protocol. The V3-V4 region of the 16S rRNA gene was amplified using universal primers 338F (ACTCCTACGGGAGGCAGCAG) and 806R (GGACTACHVGGGTWTCTAAT) (Ren et al., 2017). The amplified products were detected using 2% agarose gel electrophoresis, purified using AxyPrep DNA Gel Extraction Kit (Axygen Biosciences, United States), and quantified by Qubit 2.0 Fluorometer (Thermo Fisher Scientific, United States). Purified PCR products were pooled into equimolar amounts and sequenced on the Illumina MiSeq platform to generate paired-end reads of 300 bp (Caporaso et al., 2012).
Analysis of Sequencing Data
Raw paired-end reads were strictly analyzed using QIIME (version 1.9) (Caporaso et al., 2012). In brief, the low-quality sequences with a length of <220 nt or >500 nt, an average quality score of <20, and sequences containing > 3 nitrogenous bases, were removed (Masella et al., 2012). The remaining high-quality sequences were clustered into OTUs at a 97% similarity using UPARSE (version 7.0) (Edgar, 2013) and chimeric sequences were removed using UCHIME (Edgar et al., 2011). Taxonomy assignment of OTUs was conducted with the RDP classifier1 (Bacci et al., 2015) against the SILVA 16S rRNA gene database (Release128)2 (Pruesse et al., 2007) with a confidence threshold of 0.70.
Alpha-diversity was evaluated by calculating the Shannon diversity index and number of OTUs per sample with the MOTHUR program (version v.1.30.1)3 (Schloss et al., 2009). Bar plots and Heat maps were obtained using the “vegan” package in R (version 3.3.1). For beta-diversity analysis, PCoA was performed based on Bray-Curtis and Unweighted Unifrac distances using QIIME (version 1.9). ANOSIM (1,000 Monte Carlo permutations) based on Bray-Curtis and Unweighted Unifrac distances was performed to compare the similarity of microbial community between groups using the “vegan” package of R (version 3.3.1). In addition, the prediction of the microbial gene functions were done using PICRUSt software (version 1.0) against the KEGG database (Langille et al., 2013).
Determination of the Bacterial Metabolites
Short-chain fatty acids including formate, acetate, propionate, butyrate, isobutyrate, valerate, and isovalerate, as well as lactate in luminal contents were quantified with Ion Chromatograph as previously described (He et al., 2018). In brief, 0.5 g of digesta samples were weighed, dissolved with 8 mL ultrapure water, homogenized, and then centrifuged at 3000 × g for 10 min. The supernatant was diluted (1:50), filtered through a 0.22 μm membrane, kept in a 2 mL screw-cap vial, and then subjected for SCFAs analysis with an Ion Chromatography System (DIONEX ICS-3000, Thermo Fisher Scientific, United States).
Quantification of Predominant Lactobacillus Species
Total DNA was extracted from the luminal digesta samples as mentioned above. The primers for the species L. amylovorus, L. johnsonii, L. mucosae, and L. salivarius, total Lactobacillus, and total bacteria are shown in Supplementary Table S1 as reported previously. The qPCR was conducted with the Roche LightCycler® 96 Real-time PCR system (Roche, Sweden). The reaction mixture (25 μL) contained 1.5 μL forward and 1.5 μL reverse primers, 12.5 μL 2 × TB GreenTM Premix Ex TaqTM II (Takara, Japan), 1 μL template DNA, and 8.5 μL ddH2O. The reaction protocol consisted of one initial denaturation at 95°C for 10 min, 40 cycles of denaturation at 95°C for 10 s, 60 s at the appropriate annealing temperature (Supplementary Table S1), and extension at 72°C for 10 s. All samples were analyzed in duplicates. Standard curves were generated by constructing standard plasmids containing the 16S rRNA genes as previously described (Han et al., 2012). The copy numbers of each target bacteria were calculated using the corresponding standard curves. Briefly, a series of 10-fold dilution (109 to 101 copies/μL) of plasmid DNA were used to generate their respective standard curves with the logarithm of target copy numbers as the abscissa and the Ct values as the ordinate. The gene copy numbers were calculated using the following equation: [DNA concentration (μg/μL) × 6.0233 × 1023 copies/mol]/[DNA size (bp) × 660 × 106].
Statistical Analysis
Non-parametric Mann–Whitney U-test (SPSS 20.0) was used to compare the difference between LBW and NBW piglets as well as between the ileal and colon at each time-point (Ren et al., 2016). P-values were adjusted with a FDR (below 5%) as described by Benjamini and Hochberg (1995). The differential bacteria taxa between LBW and NBW piglets were identified using discriminant analysis (LDA) effect size (LEfSe) analysis. Moreover, STAMP software (version 2.1.3) was applied to detect the differentially abundant KEGG pathways between groups using the Wilcoxon rank-sum test with FDR correction (Parks et al., 2014). Only taxa with an average relative abundance greater than 0.01% were considered. The corrected P-values below 0.05 were considered as statistically significant.
Results
Summary of Sequencing Data and Alpha Diversities Across All Samples
A total of 2,683,338 high-quality sequences were obtained from 72 digesta samples, with an average of 37,268 sequences per sample. Rarefaction curves implied that almost all the bacterial species were captured in luminal contents of all piglets (Supplementary Figure S1). We randomly subsampled per sample to 23,185 sequences for subsequent analysis. These sequences were clustered into 1,250 OTUs based on 97% sequence similarity, and then assigned to 21 phyla, 40 classes, 85 orders, 167 families, and 441 genera.
Alpha diversity parameters, including Numbers of OTUs and Shannon diversity index, were substantially increased in the colon compared with the ileum at each time-point (Figures 1E,F). However, birth weight had no significant influence on the bacterial richness and diversity of ileum and colon at any time-point (Figures 1A–D).
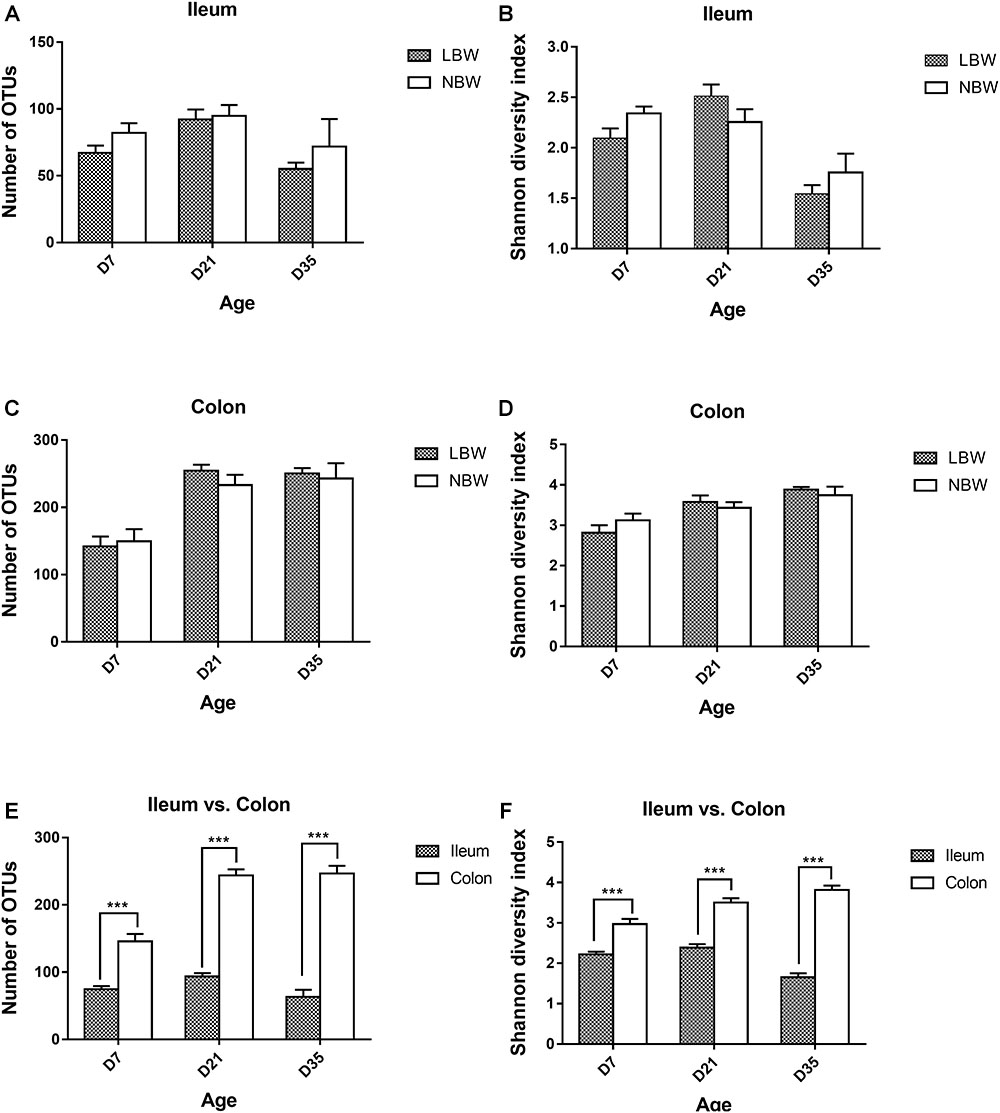
Figure 1. Alpha diversity of the gut bacterial community of LBW and NBW piglets. Number of OTUs (A) and shannon diversity index (B) in ileal samples of LBW and NBW piglets at each time point. Number of OTUs (C) and shannon diversity index (D) in colonic samples of LBW and NBW piglets at each time point. Number of OTUs (E) and Shannon diversity index (F) in the colon and ileum of all piglets at each time point. Data are shown as mean ± SEM. ∗∗∗P < 0.001. n = 6 per group. LBW, low birth weight; NBW, normal birth weight.
Spatial Variations in the Ileal and Colonic Microbiota
The overall bacterial composition of the ileum was significantly different from the colon throughout this experiment, irrespective of birth weight (Figure 2). A PCoA plot based upon Bray-Curtis distances (Supplementary Figure S2A) and Unweighted Unifrac distances (Figure 3A) further confirmed that ileal samples were clearly separated from colonic samples at all time-points. An ANOSIM of these distances also showed that the gut microbiota structure of piglets was strongly affected by gut segments (Supplementary Table S2, P < 0.01). At the phylum level (Figure 2A), Firmicutes and Proteobacteria were predominant in the ileum, while Firmicutes and Bacteriodetes were dominant in the colon. At the family level (Figure 2B), Lactobacillaceae, Clostridiaceae 1, Pasteurellaceae, Peptostreptococcaceae, and Streptococcaceae were the main bacterial families in ileal samples. In contrast, Prevotellaceae, Ruminococcaceae, Bacteriodaceae, Fusobacteriaceae, as well as Lactobacillaceae were dominant in colonic contents. At the genus level (Figure 2C), ileal microbiota was dominated by Lactobacillus, Actinobacillus, Terrisporobacter, Streptococcus, and Clostridium sensu strico 1. In the colon, Lactobacillus was still dominant but significantly lower than that in the ileum. Meanwhile, the relative proportions of Bacteroides, five genera of Prevotellaceae (Prevotella 2, Prevotella 1, Prevotella 9, Alloprevotella, Prevotellaceae NK3B31 group), Fusobacterium, and Ruminococcus 2 were greater compared with the ileal microbiota. The relative abundances of the top 20 most abundant genera are presented in Supplementary Figure S3 for visualization.
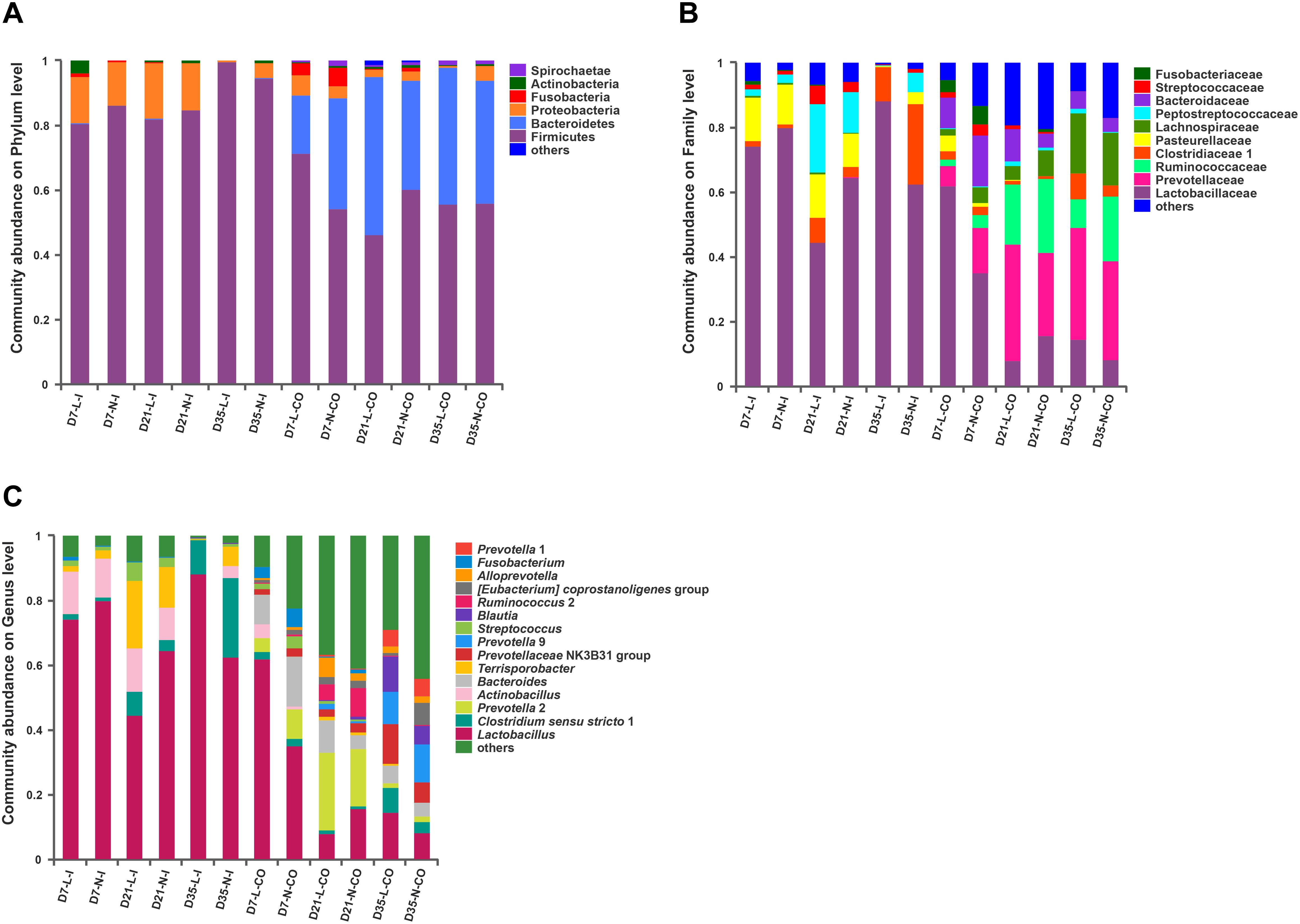
Figure 2. Gut microbiota composition of LBW and NBW piglets. Abundant phyla (A), families (B), and genera (C) in the gut microbiota of LBW and NBW piglets. Only families and genera with average relative abundance greater than 5% were shown. Data are shown as means. n = 6 per group. L, low birth weight; N, normal birth weight; I, ileum; CO, colon.
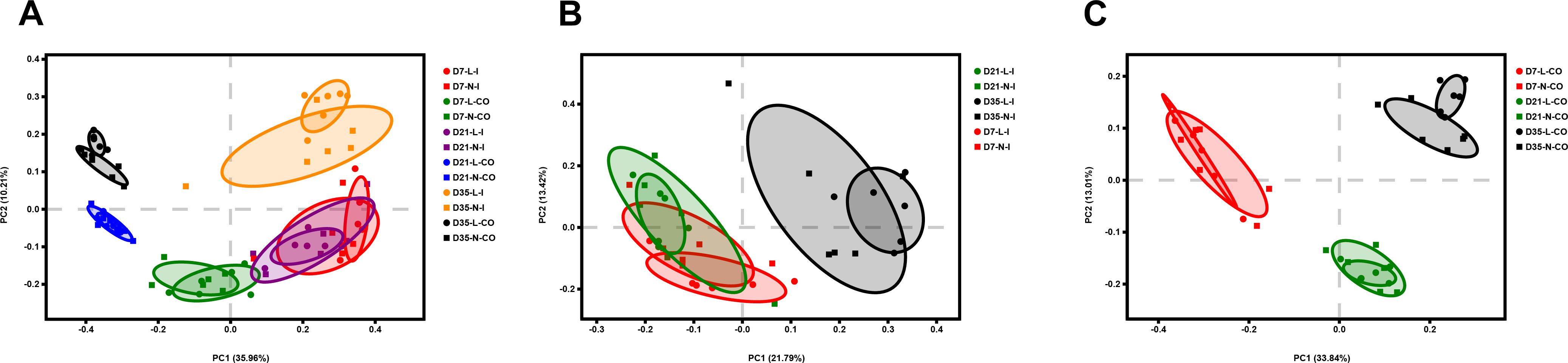
Figure 3. Principal coordinate analysis (PCoA) of all samples (A), ileal samples (B), and colonic samples (C) of LBW and NBW piglets, based on Unweighted UniFrac distances. n = 6 per group. L, low birth weight; N, normal birth weight; I, ileum; CO, colon.
Differences in the Ileal and Colonic Microbiota Between LBW and NBW Piglets
Principal coordinates analysis plots based on Bray-Curtis distances (Supplementary Figures S2B,C) and Unweighted Unifrac distances (Figures 3B,C) showed no clear separation of the overall bacterial community structure between LBW and NBW piglets on D7, D21, and D35, which was confirmed by ANOSIM (Supplementary Table S2, P > 0.05).
Furthermore, we applied the Mann–Whitney U-test and LEfSe analysis to identify differentially abundant phyla, families, and genera in the ileal and colonic microbiota between LBW and NBW piglets at each certain age. The results revealed that the influence of LBW on the bacterial community composition were focused on the colonic digesta (Figure 4 and Supplementary Table S3). At the phylum level, the relative abundance of Bacteroidetes was significantly higher in the ileum (0.006 vs. 0.0 51%, P < 0.05) and colon (17.480 vs. 33.860%, P < 0.05) of LBW piglets than that in NBW piglets on D7. On D35, LBW piglets had significantly lower population of Proteobacteria in the colon (0.311 vs. 4.418%, P < 0.01) compared with the normal ones. At the family level, 7-day-old LBW piglets showed dramatically lower relative abundances of Prevotellaceae (0.006 vs. 0.037%, P < 0.05), Ruminococcaceae (0.002 vs. 0.022%, P < 0.05), and Bacteroidaceae (0.000% vs. 0.010%, P < 0.05) in the ileum as well as Lachnospiraceae (1.924% vs. 4.952%, P < 0.05) in the colon than NBW piglets. However, the relative abundance of Lactobacillaceae (62.850% vs. 35.720%, P < 0.05) was significantly increased in the colon of LBW piglets compared with the normal group. In the colon on D35, LBW piglets presented markedly lower proportions of Campylobacteraceae (0.000% vs. 3.400%, P = 0.01), Desulfovibrionaceae (0.028% vs. 0.343%, P < 0.05), and Erysipelotrichaceae (0.384% vs. 0.828%, P < 0.05) but higher Peptostreptococcaceae (1.627% vs. 0.423%, P < 0.05) than NBW piglets. At the genus level, the lower proportions of Bacteroides (0.000% vs. 0.010%, P < 0.05) in the ileum, Alistipes (0.050% vs. 0.235%, P < 0.05), Lachnoclostridium (0.556% vs. 2.440%, P < 0.05), and Lachnospiraceae FCS020 group (0.000% vs. 0.039%, P < 0.05) were observed in LBW piglets on D7 compared to normal ones. But a higher proportion of Lactobacillus (62.850% vs. 35.720%, P < 0.05) resided in the colon of LBW piglets. Moreover, the population of Peptostreptococcus (0.010% vs. 0.000%, P < 0.05) and Coprococcus 1 (0.225% vs. 0.024%, P < 0.05) on D21, and Terrisporobacter (0.669% vs. 0.118%, P < 0.05) on D35 were significantly higher in the colon LBW piglets than those in NBW piglets. In the contrary, Blautia (0.291% vs. 0.721%, P < 0.05) and Eubacterium nodatum group (0.116% vs. 0.525%, P < 0.05) had obviously lower proportions in the colon of 21-day-old LBW piglets compared with the NBW group. Meanwhile, relative abundances of Campylobacter (0.000% vs. 0.340%, P < 0.01), Desulfovibrio (0.026% vs. 0.336%, P < 0.05), Eubacterium oxidoreducens group (0.000% vs. 0.021%, P < 0.05), Howardella (0.006% vs. 0.043%, P < 0.01), and Ruminiclostridium 9 (0.325% vs. 1.205%, P < 0.05) were obviously reduced in the colon of 35-day-old LBW piglets.
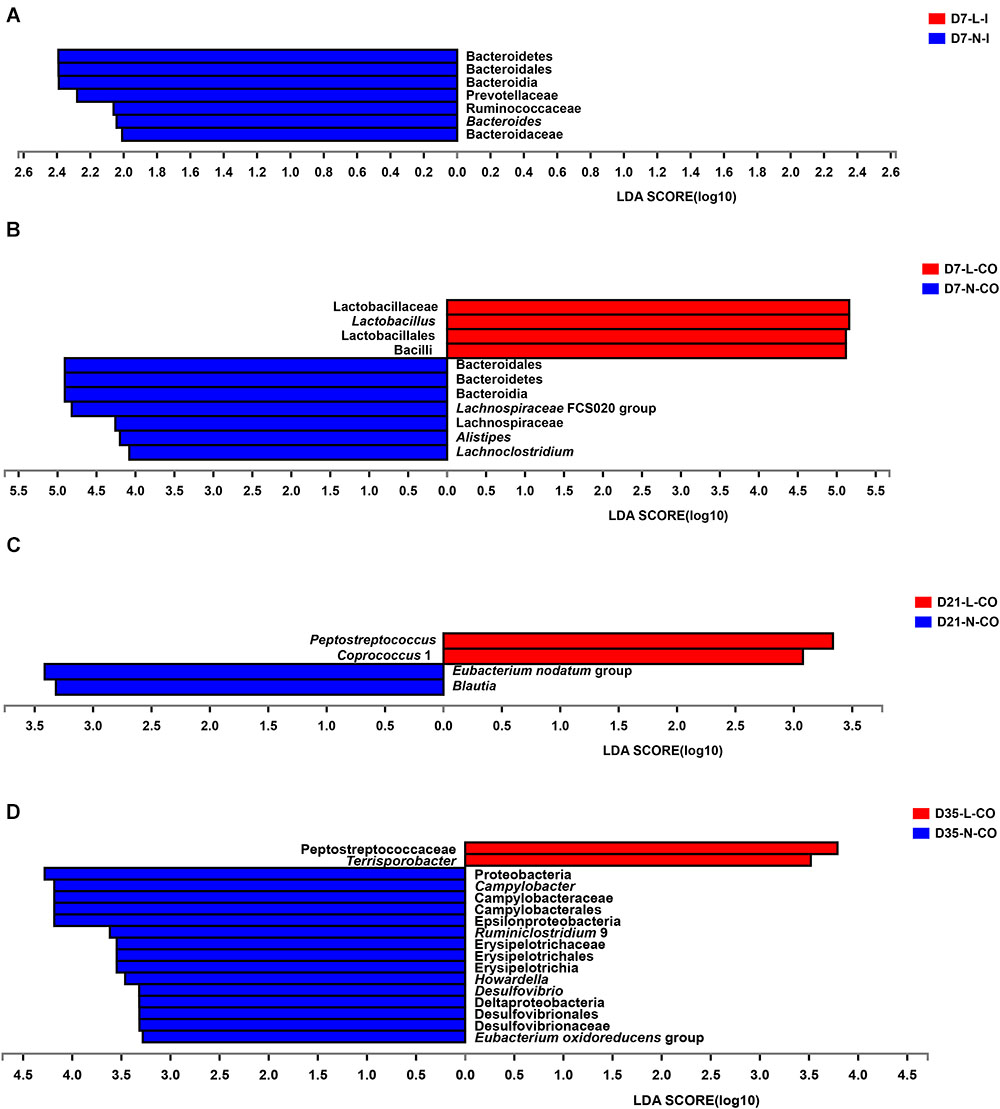
Figure 4. Differentially abundant taxa between LBW and NBW piglets. Histograms of a linear discriminant analysis (LDA) score (threshold: ≥ 2) in ileal samples on D7 (A), colonic samples on D7 (B), colonic samples on D21 (C), and colonic samples on D35 (D) are plotted. n = 6 per group. L, low birth weight; N, normal birth weight; I, ileum; CO, colon.
Differences in the Predicted Microbial Gene Functions Between LBW and NBW Piglets
To figure out the functional differences between microbiome residing in LBW and NBW piglets, we performed functional analysis of microbiota using PICRUST. A total of 41 categories of gene functions were successfully obtained. The most abundant gene functions included membrane transport (10.260∼13.880%), carbohydrate metabolism (10.120∼11.210%), replication and repair (9.458∼11.510%), amino acid metabolism (6.969∼9.649%), translation (6.089∼7.202%), energy metabolism (4.934∼6.151%), poorly characterized (4.802∼5.308%), and nucleotide metabolism (4.309∼5.357%). The results of STAMP demonstrated that the functional capability of LBW and NBW piglets significantly differed at each time-point (Figure 5 and Supplementary Table S4). Compared with NBW piglets, the ileal bacterial community in LBW piglets had higher relative abundances of the genes associated with lipid metabolism (0.325 vs. 1.205%, P < 0.05) and nervous system (0.130 vs. 0.119%, P < 0.05) on D35. Meanwhile, the colonic community of 35-day-old LBW piglets also have the increased proportion of genes involved in nervous system (0.121 vs. 0.113%, P < 0.05). In addition, a total of 12 differentially metabolic pathways were detected in the colonic microbiota between 7-day-old LBW and NBW piglets. The relative abundances of the genes involved in replication and repair (10.920 vs. 9.926%, P < 0.05), translation (6.818 vs. 6.244%, P < 0.05), poorly characterized (5.097 vs. 4.949%, P < 0.05), nucleotide metabolism (4.967 vs. 4.570%, P < 0.05), genetic information processing (2.806 vs. 2.674%, P < 0.05), and immune system diseases (0.088 vs. 0.069%, P < 0.05) were dramatically enriched in the colonic community of LBW piglets on D7. However, the proportions of the genes related with amino acid metabolism (7.635 vs. 8.589%, P < 0.05), energy metabolism (5.376 vs. 5.673%, P < 0.05), metabolism of cofactors and vitamins (3.560 vs. 4.023%, P < 0.01), biosynthesis of other secondary metabolites (0.704 vs. 0.861%, P < 0.05), endocrine system (0.222 vs. 0.284%, P < 0.05), and immune system (0.042 vs. 0.066%, P < 0.05) were significantly lower in the colonic microbiota of 7-day-old LBW piglets than those in the NBW ones. Genes related to membrane transport, as the most abundant pathway, were also decreased in the colonic community of 21-day-old LBW piglets (10.260 vs. 11.610%, P < 0.05).
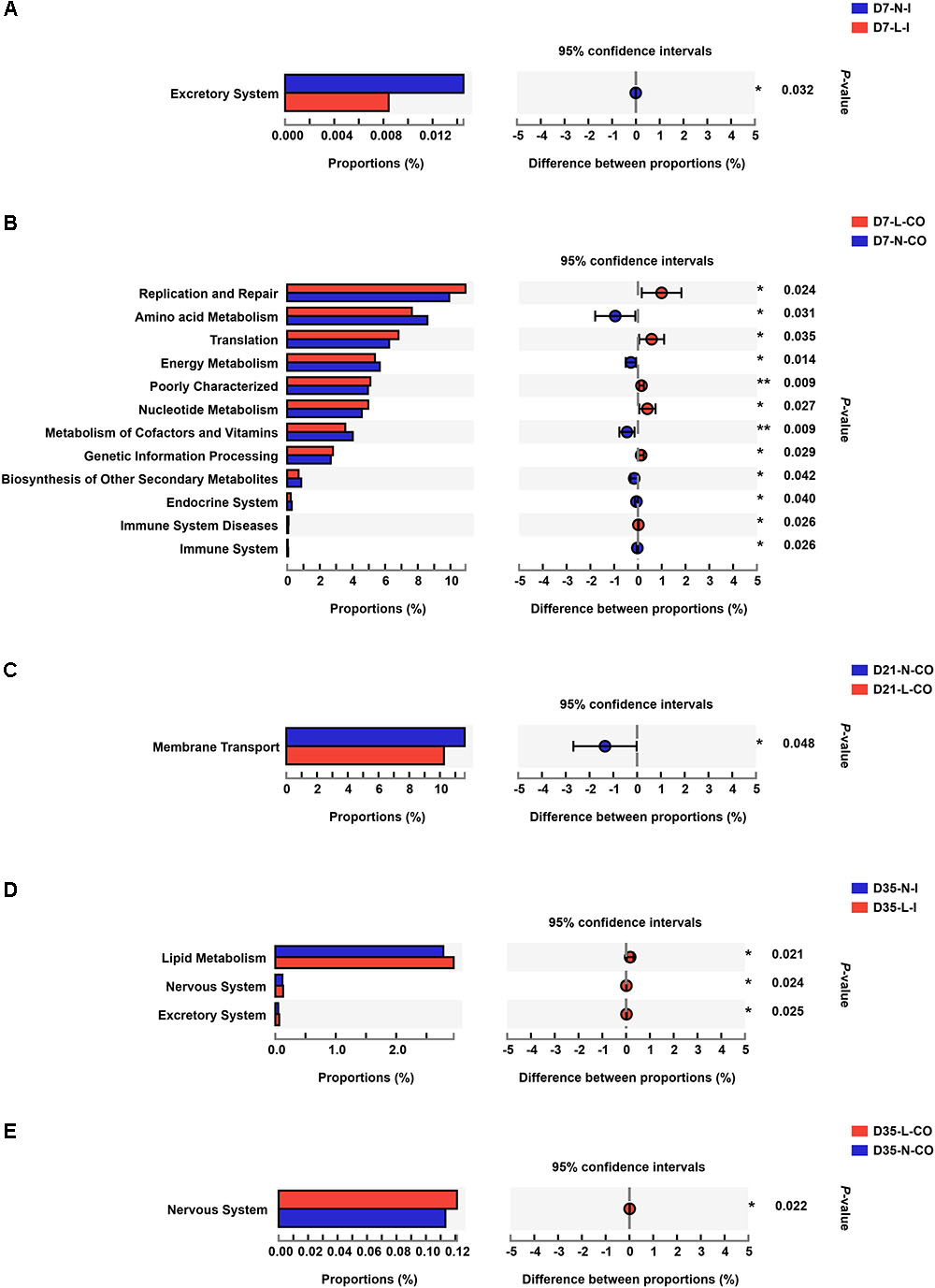
Figure 5. Differentially functional profiles of the gut bacterial community between LBW and NBW piglets. Differential abundant KEGG pathways in ileal samples on D7 (A), colonic samples on D7 (B), colonic samples on D21 (C), ileal samples on D35 (D), and colonic samples on D35 (E) are plotted. Data are shown as means. ∗∗P < 0.01; ∗P < 0.05. n = 6 per group. L, low birth weight; N, normal birth weight; I, ileum; CO, colon.
Differences in Production of the Bacterial Metabolites Between LBW and NBW Piglets
Total concentration of organic acids in the ileum was continuously lower than that of the colon from D7 to D35, with a significant decrease in 7- and 35-day-old LBW piglets (Supplementary Figure S4A, P < 0.05) and in 21- and 35-day-old NBW piglets (Supplementary Figure S4B, P < 0.01). In the ileum, the main organic acids consisted of lactate, formate, and acetate (Supplementary Figure S4A). The concentrations of acetate and propionate were greatly increased in the colon, while the proportion of formate was decreased (Supplementary Figure S4B). Compared with NBW piglets, LBW piglets had significantly decreased total concentration of SCFAs in the ileum from D7 and D21 (Figure 6F, P < 0.01) and in the colon at all three time-points (Figure 7I, P < 0.05). In the ileum, the concentrations of acetate on D7 and D35, propionate on D21, and butyrate on D35 were markedly reduced in LBW piglets (Figures 6C–E and Supplementary Table S5, P < 0.01). No difference was observed in the levels of lactate and formate between LBW and NBW piglets (Figures 6A,B and Supplementary Table S5, P > 0.05). In the colon, the concentrations of valerate on D7 and D21, lactate on D21 and D35, and isovalerate at each time-point were also lower in LBW piglets than those in the NBW ones (Figures 7A,G,H and Supplementary Table S5, P < 0.05). Additionally, the lower concentrations of acetate on D7 and D35 as well as propionate on D21 were detected again in the colon of LBW piglets compared with the normal group (Figures 7C,D and Supplementary Table S5, P < 0.05). No significant effect was seen in the levels of formate, butyrate, and isobutyrate, between LBW and NBW piglets (Figures 7B,E,F and Supplementary Table S5, P > 0.05).
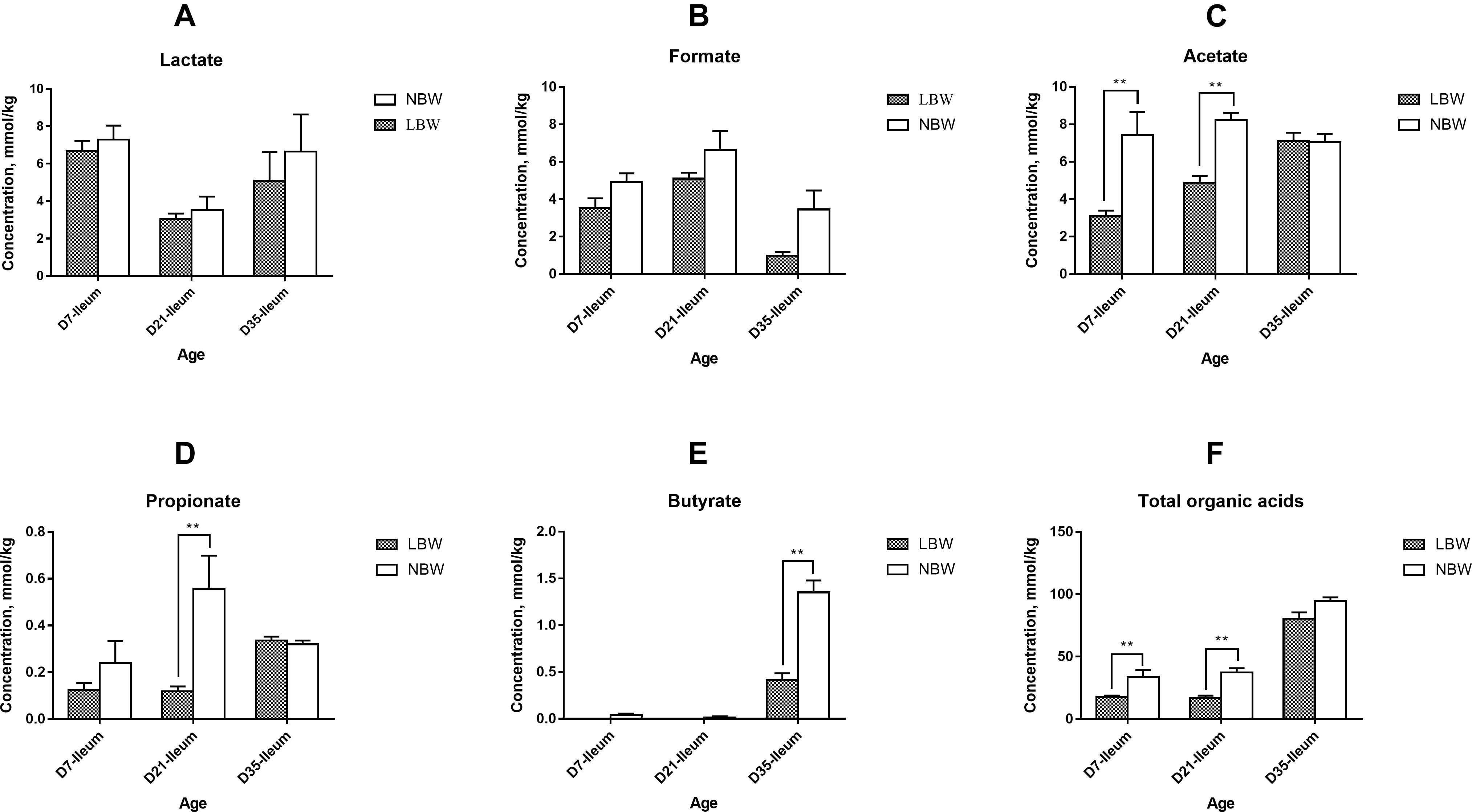
Figure 6. Concentrations of organic acids in ileal samples of piglets. Concentration of lactate (A), formate (B), acetate (C), propionate (D), butyrate (E), and total organic acids (F) of LBW and NBW piglets. Data are shown as mean ± SEM. ∗∗P < 0.01. n = 6 per group. LBW, low birth weight; NBW, normal birth weight.
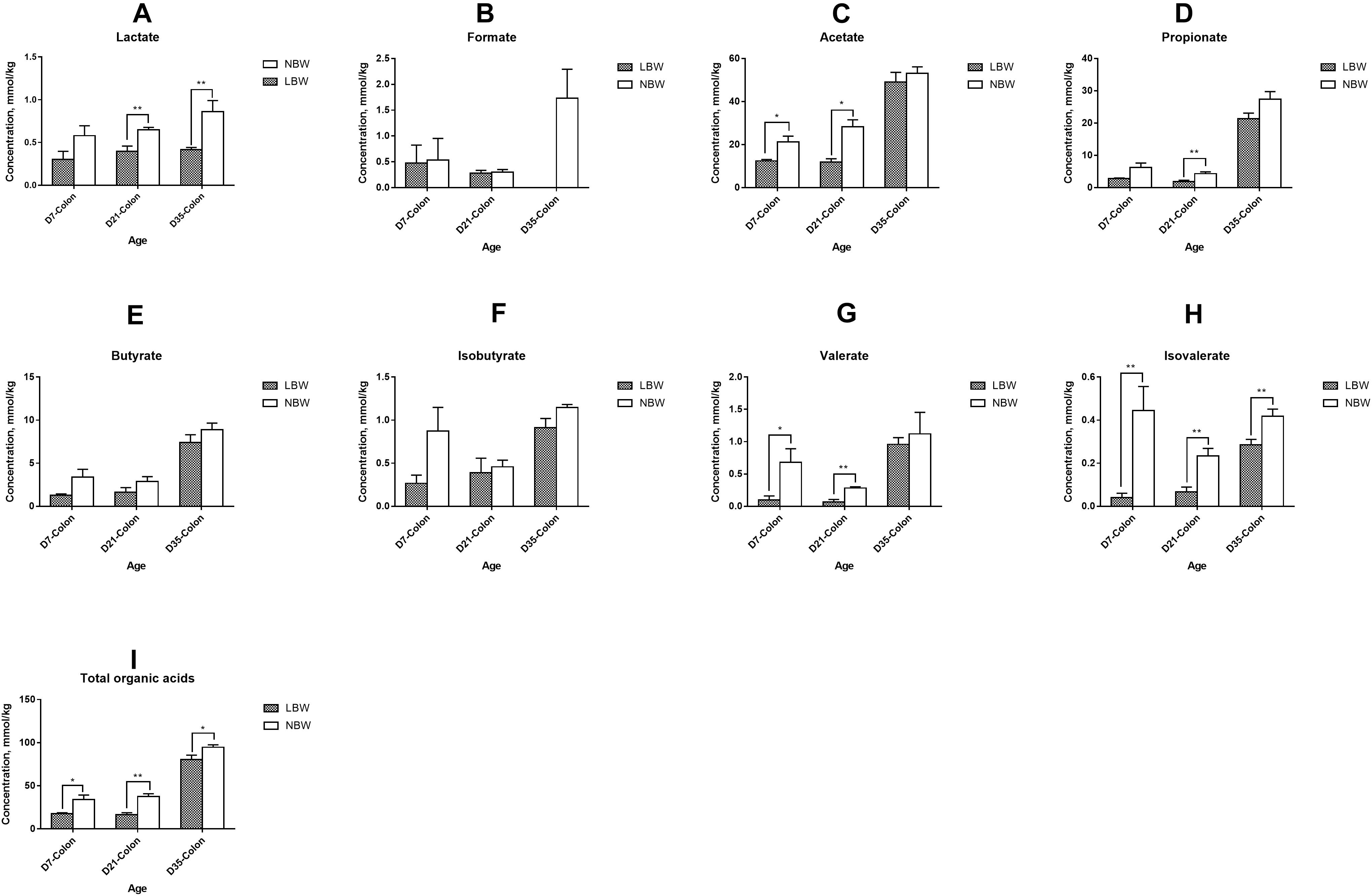
Figure 7. Concentrations of organic acids in colonic samples of piglets. The concentration of lactate (A), formate (B), acetate (C), propionate (D), butyrate (E), isobutyrate (F), valerate (G), isovalerate (H), and total organic acids (I) of LBW and NBW piglets. Data are shown as mean ± SEM. ∗∗P < 0.01; ∗P < 0.05. n = 6 per group. LBW, low birth weight; NBW, normal birth weight.
Differences in the Dominant Lactobacillus Species Between LBW and NBW Piglets
For four predominant Lactobacillus species, results from qPCR analysis revealed that L. johnsonii had the highest copy number in both of the ileal and colonic samples, closely followed by L. mucosae and L. amylovorus, with L. salivarius the least (Figure 8 and Supplementary Table S6). Birth weight had no significant influence on the copy numbers of total bacteria, total Lactobacillus, L. johnsonii, L. mucosae, and L. salivarius in the ileal digesta (Figures 8A,B,D–F and Supplementary Table S6, P > 0.05). However, the copy number of L. amylovorus in the ileum of LBW piglets on D21 were significantly declined compared with the NBW ones (Figure 8C and Supplementary Table S6, P < 0.05). In the colon, 21-day-old LBW piglets had the less copy number of total bacteria and total Lactobacillus compared to NBW piglets (Figures 9A,B and Supplementary Table S6, P < 0.05). Moreover, the population of L. amylovorus was significantly reduced again in the colon of LBW piglets on D21 (Figure 9C and Supplementary Table S6, P < 0.01). Furthermore, the less copy number of L. salivarius were also observed in the colon of LBW piglets than that in the NBW ones on D7 (Figure 9F and Supplementary Table S6, P < 0.01). No significant influence was observed in the copy numbers of L. johnsonii and L. mucosae between LBW and NBW piglets (Figures 9D,E and Supplementary Table S6, P > 0.05).
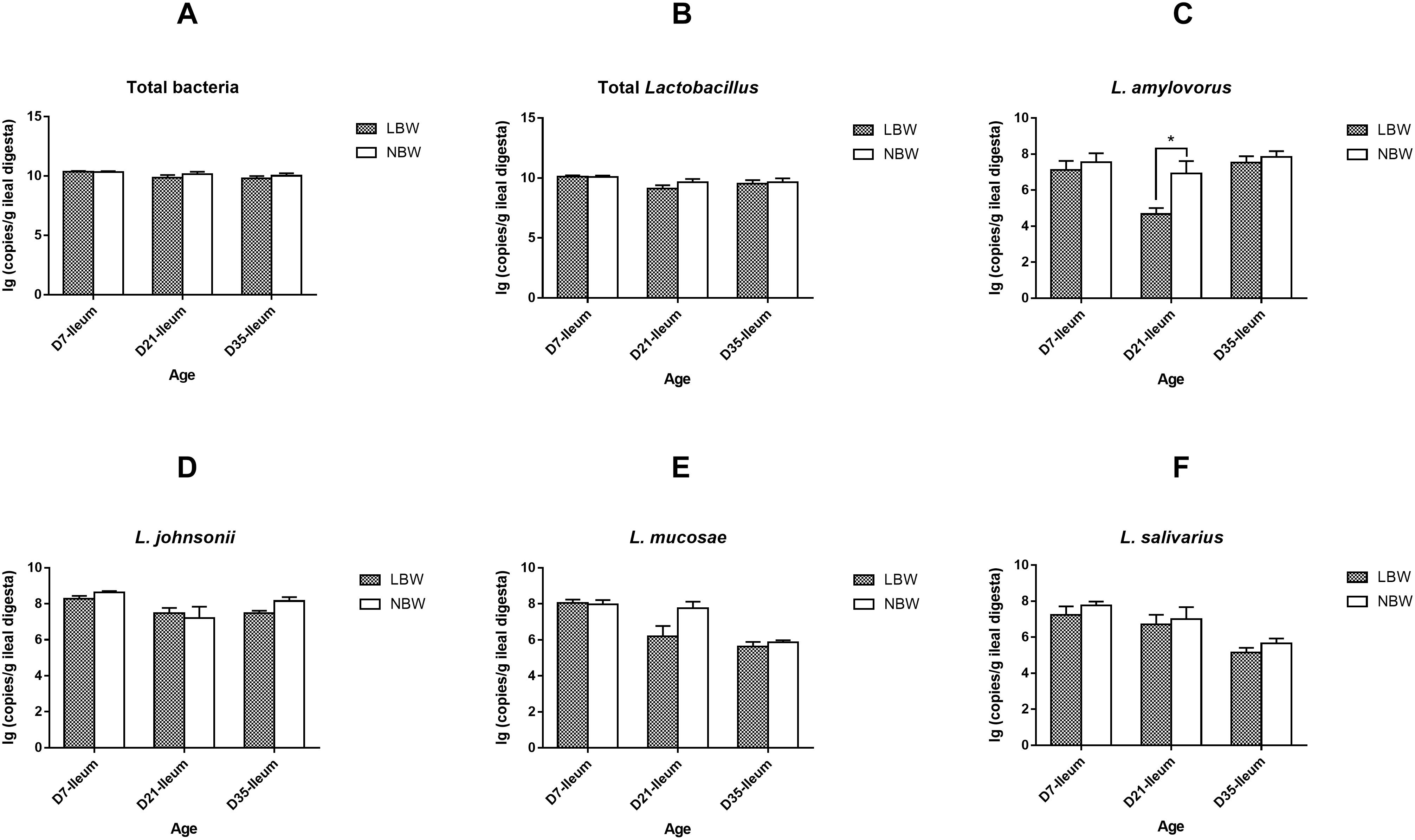
Figure 8. Copy numbers of predominant Lactobacillus species in ileal samples of piglets. The copy number of total bacteria (A), total Lactobacillus (B), L. amylovorus (C), L. johnsonii (D), L. mucosae (E), and L. salivarius (F) of LBW and NBW piglets. Data are shown as mean ± SEM. ∗P < 0.05. n = 6 per group. LBW, low birth weight; NBW, normal birth weight.
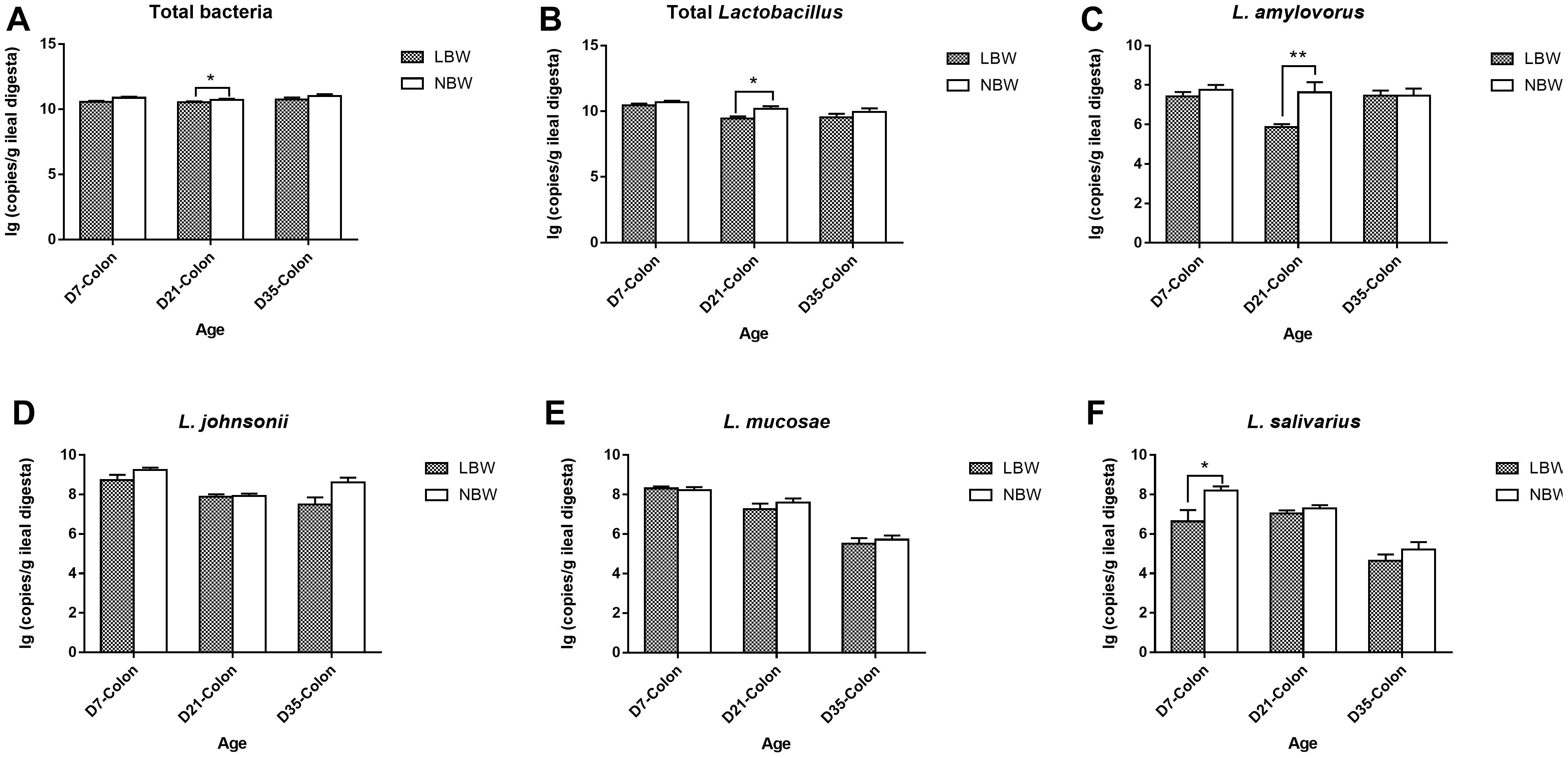
Figure 9. Copy numbers of predominant Lactobacillus species in colonic samples of piglets. The copy number of total bacteria (A), total Lactobacillus (B), L. amylovorus (C), L. johnsonii (D), L. mucosae (E), and L. salivarius (F) of LBW and NBW piglets. Data are shown as mean ± SEM. ∗∗P < 0.01; ∗P < 0.05. n = 6 per group. LBW, low birth weight; NBW, normal birth weight.
Discussion
Low-birth-weight piglets are at high risk for postnatal mortality, reduced growth rates and poor carcass quality (Wu et al., 2006; Berends et al., 2013). Our previous study has shown a significant alteration in the fecal bacterial community structure of LBW piglets during suckling and weaning periods (Li et al., 2018). Considering the segmented distribution of the gut microflora, the present investigation extended this work to the ileal and colonic bacterial community and further characterized differentially abundant taxa and microbial gene functions. In addition, we clarified spatial changes in the production of SCFAs and the colonization of predominant Lactobacillus species in ileum and colon between the LBW and NBW piglets. Our findings suggested that LBW piglets presented significantly altered bacterial communities, microbial metabolism and gene functions in ileum and colon from 7 to 35 days of age, especially the colonic microbiota.
Numerous studies have indicated the spatial heterogeneity in the bacterial community composition across the swine intestinal tract (Looft et al., 2014; Zhao et al., 2015; Kelly et al., 2017; Mu et al., 2017; Gao et al., 2018; Zhang et al., 2018). In our study, we found that the microbiota of ileal digesta samples from all the piglets showed lower diversity than that of colonic samples. Firmicutes and Proteobacteria were primarily colonized in the ileum, while Firmicutes and Bacteriodetes were the most prevalent phyla in the colon, which are consistent with other studies (Looft et al., 2014; Zhao et al., 2015). The spatial distribution of gut microbes may be derived from the difference in oxygen availability, pH gradient, and nutrient substrate along the intestinal lumen (Zhang et al., 2018). It has been well-known that the higher numbers of microorganisms residing in the large intestine are primarily correlated to the bacterial fermentation (DiBaise et al., 2008). In our study, microbes responsible for degrading indigestible carbohydrates, including Bacteroidetes (Arumugam et al., 2011), Prevotellaceae (Zhang et al., 2018), and some families of Firmicutes such as Lachnospiraceae (Rode et al., 1981) and Ruminococcaceae (Duncan et al., 2007), were significantly increased in the colon. The enrichment of these carbohydrate degraders, thereafter, resulted in the higher concentrations of SCFAs in the colon compared with the ileum. These observations are similar to the previous studies in piglets (Mu et al., 2017; Gao et al., 2018; Zhang et al., 2018).
Available information has shown that the gut microbiota establishment is altered in preterm infants born with LBW during early life (Fanca-Berthon et al., 2010; Arboleya et al., 2012a,b). A recent study in piglets has clarified that a significantly distinct bacterial community resides in the feces of LBW piglets during suckling and weaning periods (Li et al., 2018). Moreover, results focused on the ileum and colon have indicated greater counts of adherent bacteria in the intestinal mucosa of 2- to 5-day-old piglets born with LBW (D’Inca et al., 2010, 2011). In the current study, our findings further demonstrated that LBW significantly affected the bacterial composition in ileum and colon of the piglets from D7 to D35, especially in the colon. This suggests that selectively intervening the microflora of the hindgut may be an effective therapy to restore the altered gut microbiota of LBW piglets. There are a number of specific bacterial taxa declined in the gut of LBW piglets in this study. Compared to NBW piglets, the LBW piglets harbored lower relative abundances of Prevotellaceae, Ruminococcaceae, and Bacteroidaceae in the ileum as well as Lachnospiraceae in the colon on D7. Additionally, we observed that the proportions of Alistipes within Rikenellaceae, Lachnospiraceae FCS020 group, Lachnostridium, and Eubacterium oxidoreducens group within Lachnospiraceae, as well as Ruminiclostridium 9 within Ruminococcaceae, were also decreased in the colon of LBW piglets. Bacteria within these families were considered to own great abilities in degrading refractory carbohydrates to produce SCFAs (Zhang et al., 2018). Blautia within Lachnospiraceae and Eubacterium nodatum group in Clostridales are also recognized as SCFAs producers (Levine et al., 2013; Louis et al., 2014), which were less abundant in the colon of 21-day-old LBW piglets. Desulfovibrio spp. are generally considered as sulfate-reducing bacteria with the potential to utilize the sulfated mucins (Earley et al., 2015) and have a positive correlation with dietary fiber degradation (Luo et al., 2018). In the current study, the genus Desulfovibrio was discovered to have the lower prevalence in the colonic digesta of 35-day-old piglets. Therefore, the observed reduction in members of these taxa might lead to the lower production of SCFAs in the hindgut of LBW piglets. As expected, for the first time, we found that LBW piglets had lower levels of total SCFAs, acetate, propionate, valerate, and isovalerate in the colonic content at different ages compared with the normal ones. Collectively, these findings reflect an attenuated capacity to metabolize dietary fiber in LBW piglets. Besides, LBW piglets have been well recognized as being more susceptible to impaired intestinal development and various gut infections (Li et al., 2017; Wang et al., 2017). SCFAs have broad impacts on improving intestinal barrier function and reducing inflammation in the gut (Turroni et al., 2018). The reduction of SCFAs production, therefore, might partly explain the high morbidity of LBW piglets. Also, the genus Howardella presented a reduced relative abundance in the colon of LBW piglets at 35 days of age in our study, which is in agreement with the observation in the feces of LBW piglets (Li et al., 2018). The family Erysipelotrichaceae, which has recently been positively associated with higher feed intake (Buzoianu et al., 2012), had a decreased proportion in the colon of 35-day-old LBW piglets.
Compared to NBW piglets, the LBW piglets had higher relative abundances of Peptostreptococcus and Terrisporobacter within the family Peptostreptococcaceae in the colon on D21 and D35, respectively. Previous studies reported that Peptostreptococcaceae were more prevalent in subjects with colorectal cancer compared to controls (Chen et al., 2012; Zhu et al., 2014). This suggests that increased microbes belonging to Peptostreptococcaceae as commensal bacteria may have the potential to cause the intestinal infections of the host. Another interesting observation in the present study is that Campylobacter spp. of the phylum Proteobacteria, some of which are associated with the occurrence of diarrhea in piglets, were less abundant in the colonic digesta of 35-day-old LBW piglets than those in NBW piglets. However, an opposite observation was found in the feces of LBW piglets during nursing period (Li et al., 2018). These contradictory findings might result from the difference in intestinal regions and time-points sampled. Campylobacter spp. detected in the current study might not be pathogenic as diarrhea was not observed in any piglet born with either LBW or NBW.
Species from Lactobacillus, exhibiting excellent probiotic properties in improving health and disease resistance, are widely used as probiotics (Naito et al., 2011; Wang et al., 2012). Results from qPCR in this study showed that the LBW piglets had a decreased copy number of total Lactobacillus in the colon on D21, in agreement with previous studies in placenta, vagina, and feces of premature LBW infants (Sakata et al., 1985; Hillier et al., 1995; Zheng et al., 2015). Similar results were also seen in the feces of LBW rodents and piglets (Wang et al., 2016; Li et al., 2018). In contrast, sequencing data in this study presented a higher relative abundance of the genus Lactobacillus colonized in the colon of 7-day-old LBW piglets. These inconsistent results could be explained by the difference in analytical tools and targeting primers for quantification and need further investigation. Moreover, Lactobacillus spp. can produce lactate as a major microbial metabolite, which confers beneficial effects to the host such as inhibiting pathogen adhesion (Makras et al., 2006). In the present study, the declined concentration of lactic acid in the colonic digesta of 21-day-old LBW piglets might be partly attributed to the decrease in lactate-producing bacteria. L. amylovorus, widely considered as the predominant endogenous species in the gut of pigs (Pieper et al., 2006), exhibited a lower number in ileum and colon of the 21-day-old LBW piglets than that in the NBW ones. Another Lactobacillus species, L. salivarius, was also reduced in the colon of 7-day-old LBW piglets. Strains within L. amylovorus and L. salivarius as dietary probiotics can protect against infections by competitive exclusion against pathogens through bacteriocins excretion and inflammatory cytokine modulation (Messaoudi et al., 2013; Finamore et al., 2014). On basis of the above results, Lactobacillus spp., especially the dominant species L. amylovorus and L. salivarius, can serve as promising probiotic candidates for improving the health and growth of LBW offspring.
Beyond alterations in the microbial composition of LBW piglets, we found that the microbial gene functions of LBW piglets also differed from those of NBW piglets. Differential functional pathways between LBW and NBW piglets were mainly presented in the colonic microbiota compared with the ileum, which was consistent with the observations in the bacterial composition. Our data revealed that the functional alterations of the colonic bacterial community in LBW piglets were characterized by significantly decreased abundances of functions associated with amino acid metabolism, energy metabolism, metabolism of cofactors and vitamins, and biosynthesis of secondary metabolites on D7. Accumulating evidence indicates that the gut microbiota plays pivotal roles in amino acid catabolism and energy harvest from the diet for the generation of various bacterial metabolites including ammonia, SCFAs, and biogenic amines (Cani and Delzenne, 2009; Tremaroli and Backhed, 2012; Neis et al., 2015). Moreover, a vast array of microorganisms in the gut, such as Lactobacilli (Kleerebezem and Vaughan, 2009), can also act as important suppliers of various vitamins (LeBlanc et al., 2013). Furthermore, Zhang et al. (2018) have reported that these microbial pathways were positively associated with bacteria within Bacteroidetes, Lachnospiraceae, and Ruminococcaceae. Therefore, reduced proportions of these microbial pathways may reflect an impaired microbiota-mediated metabolic and biosynthetic capacity of nutrients in the intestine of LBW piglets. Nevertheless, we observed that microbial genes associated with lipid metabolism were dramatically enriched in the ileal bacterial community of 21-day-old LBW piglets. It has been evidenced that LBW newborns have a higher risk for developing adult metabolic and cardiovascular diseases due to the abnormality of fat storage and lipid metabolism (Wu et al., 2006). Therefore, the observed alteration in microbiota-associated lipid metabolism may be an important factor in the development of the metabolic disorders in later life of the LBW neonates. Moreover, genes functions involved in replication and repair, translation, poorly characterized, nucleotide metabolism, and genetic information processing were overrepresented in the colonic microbiome of 7-day-old LBW piglets compared to the NBW ones, which might cause aberrant genetic information transmission and expression in LBW piglets. Overall, perturbations in functional profiles of the gut microbiota of LBW piglets might have a long-term side effect on their physiology and health.
Conclusion
In summary, the results of this study provide novel evidence for an alteration of the microbiome in ileum and colon of the LBW piglets. Compared with their normal littermates, LBW piglets had significantly different bacterial communities, microbial metabolism, and microbial gene functions in the ileum and colon from 7 to 35 days of age, especially in the colon. Relative abundances of some SCFAs-producing microbes, which belong to the families Bacteroidaceae, Ruminococcaceae, Prevotellaceae, and Lachnospiraceae, were dramatically decreased in LBW piglets. Reduction of these bacteria led to decreased production of SCFAs, thereby reflecting a poorer ability to ferment dietary fiber in the hindgut of LBW piglets than that of NBW piglets. Moreover, decreased numbers of L. amylovorus and L. salivarius in the gut of LBW piglets implies that these two Lactobacillus species could be used as potential probiotics to improve the growth and development of LBW piglets. Moreover, a clear alteration in gut microbial functionality of the LBW piglets was characterized by the altered proportions of microbial genes involved in multiple pathways such as amino acid metabolism, energy metabolism, replication and repair, and metabolism of cofactors and vitamins. This work will provide new directions in identifying the reliable biomarkers affecting early colonization of gut microbiota in LBW piglets and facilitate the development of new nutritional interventions.
Data Availability
The datasets generated for this study can be found in NCBI Sequence Read Archive (SRA) database, SRP181998.
Ethics Statement
This study was carried out in accordance with the recommendations of the guidelines for the Institutional Animal Care and Use Committee of China Agricultural University. The protocol was approved by the Institutional Animal Ethical Committee of China Agricultural University (CAU20170114-1, Beijing, China).
Author Contributions
JW, ZD, and NL designed the experiments. NL, SH, and DH conducted the experiments. NL, SH, and LJ collected the samples. NL and SH performed the analysis of samples. NL, TL, and ZD analyzed the data. NL and JW wrote and revised the manuscript. All authors read and approved the final manuscript.
Funding
This work was supported by the National Natural Science Foundation of China (31630074), the Beijing Municipal Natural Science Foundation (S170001), the National Key Research and Development Program of China (2016YFD0500506 and 2018YDF0501002), the 111 Project (B16044), and Jinxinnong Animal Science Developmental Foundation.
Conflict of Interest Statement
The authors declare that the research was conducted in the absence of any commercial or financial relationships that could be construed as a potential conflict of interest.
Acknowledgments
We would like to thank the Mianyang New-hope Livestock Farming Co. Ltd. in Sichuan Province, China, for their support in the pig management.
Supplementary Material
The Supplementary Material for this article can be found online at: https://www.frontiersin.org/articles/10.3389/fmicb.2019.00797/full#supplementary-material
Abbreviations
ANOSIM, the analysis of similarities; FDR, false discovery rate; GIT, gastrointestinal tract; IUGR, intrauterine growth restriction; LBW, low birth weight; LDA, linear discriminant analysis; LEfSe, linear discriminant analysis effect size; NBW, normal birth weight; OTUs, operational taxonomic units; PCoA, principal coordinates analysis; SCFAs, short-chain fatty acids; SEM, standard error of the mean.
Footnotes
References
Arboleya, S., Binetti, A., Salazar, N., Fernandez, N., Solis, G., Hernandez-Barranco, A., et al. (2012a). Establishment and development of intestinal microbiota in preterm neonates. FEMS Microbiol. Ecol. 79, 763–772. doi: 10.1111/j.1574-6941.2011.01261.x
Arboleya, S., Solis, G., Fernandez, N., de los Reyes-Gavilan, C. G., and Gueimonde, M. (2012b). Facultative to strict anaerobes ratio in the preterm infant microbiota: a target for intervention? Gut Microbes 3, 583–588. doi: 10.4161/gmic.21942
Arumugam, M., Raes, J., Pelletier, E., Le Paslier, D., Yamada, T., Mende, D. R., et al. (2011). Enterotypes of the human gut microbiome. Nature 473, 174–180. doi: 10.1038/nature09944
Bacci, G., Bani, A., Bazzicalupo, M., Ceccherini, M. T., Galardini, M., Nannipieri, P., et al. (2015). Evaluation of the performances of Ribosomal Database Project (RDP) classifier for taxonomic assignment of 16S rRNA metabarcoding sequences generated from Illumina-solexa NGS. J. Genomics 3, 36–39. doi: 10.7150/jgen.9204
Benjamini, Y., and Hochberg, Y. (1995). Controlling the false discovery rate: a practical and powerful approach to multiple testing. J. R. Stat. Soc. Ser. B. 57, 289–300. doi: 10.1111/j.2517-6161.1995.tb02031.x
Berends, L. M., Fernandez-Twinn, D. S., Martin-Gronert, M. S., Cripps, R. L., and Ozanne, S. E. (2013). Catch-up growth following intra-uterine growth-restriction programmes an insulin-resistant phenotype in adipose tissue. Int. J. Obes. 37, 1051–1057. doi: 10.1038/ijo.2012.196
Buzoianu, S. G., Walsh, M. C., Rea, M. C., O’Sullivan, O., Cotter, P. D., Ross, R. P., et al. (2012). High-throughput sequence-based analysis of the intestinal microbiota of weanling pigs fed genetically modified MON810 maize expressing Bacillus thuringiensis Cry1Ab (Bt maize) for 31 days. Appl. Environ. Microbiol. 78, 4217–4224. doi: 10.1128/AEM.00307-12
Cani, P. D., and Delzenne, N. M. (2009). The role of the gut microbiota in energy metabolism and metabolic disease. Curr. Pharm. Des. 15, 1546–1558. doi: 10.2174/138161209788168164
Caporaso, J. G., Lauber, C. L., Walters, W. A., Berg-Lyons, D., Huntley, J., Fierer, N., et al. (2012). Ultra-high-throughput microbial community analysis on the Illumina HiSeq and MiSeq platforms. ISME J. 6, 1621–1624. doi: 10.1038/ismej.2012.8
Chen, W., Liu, F., Ling, Z., Tong, X., and Xiang, C. (2012). Human intestinal lumen and mucosa-associated microbiota in patients with colorectal cancer. PLoS One 7:e39743. doi: 10.1371/journal.pone.0039743
DiBaise, J. K., Zhang, H., Crowell, M. D., Krajmalnik-Brown, R., Decker, G. A., and Rittmann, B. E. (2008). Gut microbiota and its possible relationship with obesity. Mayo Clin. Proc. 83, 460–469. doi: 10.4065/83.4.460
D’Inca, R., Gras-Le Guen, C., Che, L., Sangild, P. T., and Le Huerou-Luron, I. (2011). Intrauterine growth restriction delays feeding-induced gut adaptation in term newborn pigs. Neonatology 99, 208–216. doi: 10.1159/000314919
D’Inca, R., Kloareg, M., Gras-Le Guen, C., and Le Huerou-Luron, I. (2010). Intrauterine growth restriction modifies the developmental pattern of intestinal structure, transcriptomic profile, and bacterial colonization in neonatal pigs. J. Nutr. 140, 925–931. doi: 10.3945/jn.109.116822
Duncan, S. H., Louis, P., and Flint, H. J. (2007). Cultivable bacterial diversity from the human colon. Lett. Appl. Microbiol. 44, 343–350. doi: 10.1111/j.1472-765X.2007.02129.x
Earley, H., Lennon, G., Balfe, A., Kilcoyne, M., Clyne, M., Joshi, L., et al. (2015). A preliminary study examining the binding capacity of Akkermansia muciniphila and Desulfovibrio spp., to colonic mucin in health and ulcerative colitis. PLoS One 10:e0135280. doi: 10.1371/journal.pone.0135280
Edgar, R. C. (2013). UPARSE: highly accurate OTU sequences from microbial amplicon reads. Nat. Methods 10, 996–998. doi: 10.1038/nmeth.2604
Edgar, R. C., Haas, B. J., Clemente, J. C., Quince, C., and Knight, R. (2011). UCHIME improves sensitivity and speed of chimera detection. Bioinformatics 27, 2194–2200. doi: 10.1093/bioinformatics/btr381
Fanca-Berthon, P., Hoebler, C., Mouzet, E., David, A., and Michel, C. (2010). Intrauterine growth restriction not only modifies the cecocolonic microbiota in neonatal rats but also affects its activity in young adult rats. J. Pediatr. Gastroenterol. Nutr. 51, 402–413. doi: 10.1097/MPG.0b013e3181d75d52
Finamore, A., Roselli, M., Imbinto, A., Seeboth, J., Oswald, I. P., and Mengheri, E. (2014). Lactobacillus amylovorus inhibits the TLR4 inflammatory signaling triggered by enterotoxigenic Escherichia coli via modulation of the negative regulators and involvement of TLR2 in intestinal Caco-2 cells and pig explants. PLoS One 9:e94891. doi: 10.1371/journal.pone.0094891
Gao, K., Pi, Y., Peng, Y., Mu, C. L., and Zhu, W. Y. (2018). Time-course responses of ileal and fecal microbiota and metabolite profiles to antibiotics in cannulated pigs. Appl. Microbiol. Biotechnol. 102, 2289–2299. doi: 10.1007/s00253-018-8774-2
Han, G. Q., Xiang, Z. T., Yu, B., Chen, D. W., Qi, H. W., Mao, X. B., et al. (2012). Effects of different starch sources on Bacillus spp. in intestinal tract and expression of intestinal development related genes of weanling piglets. Mol. Biol. Rep. 39, 1869–1876. doi: 10.1007/s11033-011-0932-x
He, B., Bai, Y., Jiang, L., Wang, W., Li, T., Liu, P., et al. (2018). Effects of oat bran on nutrient digestibility, intestinal microbiota, and inflammatory responses in the hindgut of growing pigs. Int. J. Mol. Sci. 19:2407. doi: 10.3390/ijms19082407
Hillier, S. L., Nugent, R. P., Eschenbach, D. A., Krohn, M. A., Gibbs, R. S., Martin, D. H., et al. (1995). Association between bacterial vaginosis and preterm delivery of a low-birth-weight infant. N. Engl. J. Med. 333, 1737–1742. doi: 10.1056/Nejm199512283332604
Houghteling, P. D., and Walker, W. A. (2015). Why is initial bacterial colonization of the intestine important to infants’ and children’s health? J. Pediatr. Gastroenterol. Nutr. 60, 294–307. doi: 10.1097/MPG.0000000000000597
Kelly, J., Daly, K., Moran, A. W., Ryan, S., Bravo, D., and Shirazi-Beechey, S. P. (2017). Composition and diversity of mucosa-associated microbiota along the entire length of the pig gastrointestinal tract; dietary influences. Environ. Microbiol. 19, 1425–1438. doi: 10.1111/1462-2920.13619
Kleerebezem, M., and Vaughan, E. E. (2009). Probiotic and gut lactobacilli and bifidobacteria: molecular approaches to study diversity and activity. Annu. Rev. Microbiol. 63, 269–290. doi: 10.1146/annurev.micro.091208.073341
Koh, A., De Vadder, F., Kovatcheva-Datchary, P., and Backhed, F. (2016). From dietary fiber to host physiology: short-chain fatty acids as key bacterial metabolites. Cell 165, 1332–1345. doi: 10.1016/j.cell.2016.05.041
Langille, M. G. I., Zaneveld, J., Caporaso, J. G., McDonald, D., Knights, D., Reyes, J. A., et al. (2013). Predictive functional profiling of microbial communities using 16S rRNA marker gene sequences. Nat. Biotechnol. 31, 814–821. doi: 10.1038/nbt.2676
LeBlanc, J. G., Milani, C., de Giori, G. S., Sesma, F., van Sinderen, D., and Ventura, M. (2013). Bacteria as vitamin suppliers to their host: a gut microbiota perspective. Curr. Opin. Biotechnol. 24, 160–168. doi: 10.1016/j.copbio.2012.08.005
Leser, T. D., Amenuvor, J. Z., Jensen, T. K., Lindecrona, R. H., Boye, M., and Moller, K. (2002). Culture-independent analysis of gut bacteria: the pig gastrointestinal tract microbiota revisited. Appl. Environ. Microbiol. 68, 673–690. doi: 10.1128/aem.68.2.673-690.2002
Levine, U. Y., Looft, T., Allen, H. K., and Stanton, T. B. (2013). Butyrate-producing bacteria, including mucin degraders, from the swine intestinal tract. Appl. Environ. Microbiol. 79, 3879–3881. doi: 10.1128/AEM.00589-13
Li, N., Huang, S., Jiang, L., Wang, W., Li, T., Zuo, B., et al. (2018). Differences in the gut microbiota establishment and metabolome characteristics between low- and normal-birth-weight piglets during early-life. Front. Microbiol. 9:1798. doi: 10.3389/fmicb.2018.01798
Li, N., Wang, W., Wu, G., and Wang, J. (2017). Nutritional support for low birth weight infants: insights from animal studies. Br. J. Nutr. 117, 1390–1402. doi: 10.1017/S000711451700126X
Looft, T., Allen, H. K., Cantarel, B. L., Levine, U. Y., Bayles, D. O., Alt, D. P., et al. (2014). Bacteria, phages and pigs: the effects of in-feed antibiotics on the microbiome at different gut locations. ISME J. 8, 1566–1576. doi: 10.1038/ismej.2014.12
Louis, P., Hold, G. L., and Flint, H. J. (2014). The gut microbiota, bacterial metabolites and colorectal cancer. Nat. Rev. Microbiol. 12, 661–672. doi: 10.1038/nrmicro3344
Luo, Y., Chen, H., Yu, B., He, J., Zheng, P., Mao, X., et al. (2018). Dietary pea fibre alters the microbial community and fermentation with increase in fibre degradation-associated bacterial groups in the colon of pigs. J. Anim. Physiol. Anim. Nutr. 102, e254–e261. doi: 10.1111/jpn.12736
Makras, L., Triantafyllou, V., Fayol-Messaoudi, D., Adriany, T., Zoumpopoulou, G., Tsakalidou, E., et al. (2006). Kinetic analysis of the antibacterial activity of probiotic lactobacilli towards Salmonella enterica serovar Typhimurium reveals a role for lactic acid and other inhibitory compounds. Res. Microbiol. 157, 241–247. doi: 10.1016/j.resmic.2005.09.002
Masella, A. P., Bartram, A. K., Truszkowski, J. M., Brown, D. G., and Neufeld, J. D. (2012). PANDAseq: paired-end assembler for Illumina sequences. BMC Bioinformatics 13:31. doi: 10.1186/1471-2105-13-31
Matamoros, S., Gras-Leguen, C., Le Vacon, F., Potel, G., and de La Cochetiere, M. F. (2013). Development of intestinal microbiota in infants and its impact on health. Trends Microbiol. 21, 167–173. doi: 10.1016/j.tim.2012.12.001
Messaoudi, S., Manai, M., Kergourlay, G., Prevost, H., Connil, N., Chobert, J. M., et al. (2013). Lactobacillus salivarius: bacteriocin and probiotic activity. Food Microbiol. 36, 296–304. doi: 10.1016/j.fm.2013.05.010
Mu, C., Yang, Y., Su, Y., Zoetendal, E. G., and Zhu, W. (2017). Differences in microbiota membership along the gastrointestinal tract of piglets and their differential alterations following an early-life antibiotic Intervention. Front. Microbiol. 8:797. doi: 10.3389/fmicb.2017.00797
Naito, E., Yoshida, Y., Makino, K., Kounoshi, Y., Kunihiro, S., Takahashi, R., et al. (2011). Beneficial effect of oral administration of Lactobacillus casei strain Shirota on insulin resistance in diet-induced obesity mice. J. Appl. Microbiol. 110, 650–657. doi: 10.1111/j.1365-2672.2010.04922.x
Neis, E. P., Dejong, C. H., and Rensen, S. S. (2015). The role of microbial amino acid metabolism in host metabolism. Nutrients 7, 2930–2946. doi: 10.3390/nu7042930
Parks, D. H., Tyson, G. W., Hugenholtz, P., and Beiko, R. G. (2014). STAMP: statistical analysis of taxonomic and functional profiles. Bioinformatics 30, 3123–3124. doi: 10.1093/bioinformatics/btu494
Pieper, R., Janczyk, P., Schumann, R., and Souffrant, W. B. (2006). The intestinal microflora of piglets around weaning - with emphasis on lactobacilli. Archiva Zootechnica 9, 28–40.
Pruesse, E., Quast, C., Knittel, K., Fuchs, B. M., Ludwig, W., Peplies, J., et al. (2007). SILVA: a comprehensive online resource for quality checked and aligned ribosomal RNA sequence data compatible with ARB. Nucleic Acids Res. 35, 7188–7196. doi: 10.1093/nar/gkm864
Ren, W., Wang, P., Yan, J., Liu, G., Zeng, B., Hussain, T., et al. (2017). Melatonin alleviates weanling stress in mice: involvement of intestinal microbiota. J. Pineal. Res. 64:e12448. doi: 10.1111/jpi.12448
Ren, W., Yin, J., Xiao, H., Chen, S., Liu, G., Tan, B., et al. (2016). Intestinal microbiota-derived GABA mediates interleukin-17 expression during enterotoxigenic Escherichia coli infection. Front. Immunol. 7:685. doi: 10.3389/fimmu.2016.00685
Rode, L. M., Genthner, B. R., and Bryant, M. P. (1981). Syntrophic association by cocultures of the methanol- and CO2-H2-utilizing species Eubacterium limosum and pectin-fermenting Lachnospira multiparus during growth in a pectin medium. Appl. Environ. Microbiol. 42, 20–22.
Rooks, M. G., and Garrett, W. S. (2016). Gut microbiota, metabolites and host immunity. Nat. Rev. Immunol. 16, 341–352. doi: 10.1038/nri.2016.42
Sakata, H., Yoshioka, H., and Fujita, K. (1985). Development of the intestinal flora in very low birth-weight infants compared to normal full-term newborns. Eur. J. Pediatr. 144, 186–190. doi: 10.1007/Bf00451911
Schloss, P. D., Westcott, S. L., Ryabin, T., Hall, J. R., Hartmann, M., Hollister, E. B., et al. (2009). Introducing mothur: open-source, platform-independent, community-supported software for describing and comparing microbial communities. Appl. Environ. Microbiol. 75, 7537–7541. doi: 10.1128/AEM.01541-09
Tremaroli, V., and Backhed, F. (2012). Functional interactions between the gut microbiota and host metabolism. Nature 489, 242–249. doi: 10.1038/nature11552
Turroni, S., Brigidi, P., Cavalli, A., and Candela, M. (2018). Microbiota-host transgenomic metabolism, bioactive molecules from the inside. J. Med. Chem. 61, 47–61. doi: 10.1021/acs.jmedchem.7b00244
Wang, J., Chen, L., Li, D., Yin, Y., Wang, X., Li, P., et al. (2008). Intrauterine growth restriction affects the proteomes of the small intestine, liver, and skeletal muscle in newborn pigs. J. Nutr. 138, 60–66. doi: 10.1093/jn/138.1.60
Wang, J., Feng, C., Liu, T., Shi, M., Wu, G., and Bazer, F. W. (2017). Physiological alterations associated with intrauterine growth restriction in fetal pigs: causes and insights for nutritional optimization. Mol. Reprod. Dev. 84, 897–904. doi: 10.1002/mrd.22842
Wang, J., Tang, H., Wang, X., Zhang, X., Zhang, C., Zhang, M., et al. (2016). The structural alteration of gut microbiota in low-birth-weight mice undergoing accelerated postnatal growth. Sci. Rep. 6:27780. doi: 10.1038/srep27780
Wang, Q., Dong, J., and Zhu, Y. (2012). Probiotic supplement reduces risk of necrotizing enterocolitis and mortality in preterm very low-birth-weight infants: an updated meta-analysis of 20 randomized, controlled trials. J. Pediatr Surg. 47, 241–248. doi: 10.1016/j.jpedsurg.2011.09.064
Wang, X., Lin, G., Liu, C., Feng, C., Zhou, H., Wang, T., et al. (2014). Temporal proteomic analysis reveals defects in small-intestinal development of porcine fetuses with intrauterine growth restriction. J. Nutr. Biochem. 25, 785–795. doi: 10.1016/j.jnutbio.2014.03.008
Wang, X., Wu, W., Lin, G., Li, D., Wu, G., and Wang, J. (2010). Temporal proteomic analysis reveals continuous impairment of intestinal development in neonatal piglets with intrauterine growth restriction. J. Proteome Res. 9, 924–935. doi: 10.1021/pr900747d
Wang, X., Zhu, Y., Feng, C., Lin, G., Wu, G., Li, D., et al. (2018). Innate differences and colostrum-induced alterations of jejunal mucosal proteins in piglets with intra-uterine growth restriction. Br. J. Nutr. 119, 734–747. doi: 10.1017/S0007114518000375
Wu, G., Bazer, F. W., Wallace, J. M., and Spencer, T. E. (2006). Board-invited review: intrauterine growth retardation: implications for the animal sciences. J. Anim. Sci. 84, 2316–2337. doi: 10.2527/jas.2006-156
Zhang, L., Wu, W., Lee, Y.-K., Xie, J., and Zhang, H. (2018). Spatial heterogeneity and co-occurrence of mucosal and luminal microbiome across swine intestinal tract. Front. Microbiol. 9:48. doi: 10.3389/fmicb.2018.00048
Zhao, W., Wang, Y., Liu, S., Huang, J., Zhai, Z., He, C., et al. (2015). The dynamic distribution of porcine microbiota across different ages and gastrointestinal tract segments. PLoS One 10:e0117441. doi: 10.1371/journal.pone.0117441
Zheng, J., Xiao, X., Zhang, Q., Mao, L., Yu, M., and Xu, J. (2015). The placental microbiome varies in association with low birth weight in full-term neonates. Nutrients 7, 6924–6937. doi: 10.3390/nu7085315
Keywords: low birth weight, gut microbiota, Lactobacillus species, gut segments, piglet
Citation: Li N, Huang S, Jiang L, Dai Z, Li T, Han D and Wang J (2019) Characterization of the Early Life Microbiota Development and Predominant Lactobacillus Species at Distinct Gut Segments of Low- and Normal-Birth-Weight Piglets. Front. Microbiol. 10:797. doi: 10.3389/fmicb.2019.00797
Received: 14 February 2019; Accepted: 28 March 2019;
Published: 16 April 2019.
Edited by:
Benjamin P. Willing, University of Alberta, CanadaReviewed by:
Wenkai Ren, South China Agricultural University, ChinaGang Liu, Institute of Subtropical Agriculture (CAS), China
Copyright © 2019 Li, Huang, Jiang, Dai, Li, Han and Wang. This is an open-access article distributed under the terms of the Creative Commons Attribution License (CC BY). The use, distribution or reproduction in other forums is permitted, provided the original author(s) and the copyright owner(s) are credited and that the original publication in this journal is cited, in accordance with accepted academic practice. No use, distribution or reproduction is permitted which does not comply with these terms.
*Correspondence: Junjun Wang, amt5d2pqQGhvdG1haWwuY29t