- 1Department of Laboratory Medicine, Research Institute of Bacterial Resistance, Yonsei University College of Medicine, Seoul, South Korea
- 2J. Craig Venter Institute, Rockville, MD, United States
- 3Brain Korea 21 PLUS Project for Medical Science, Yonsei University, Seoul, South Korea
- 4Institute for Medical Microbiology, Immunology and Hygiene, University of Cologne, Cologne, Germany
- 5German Centre for Infection Research, Partner site Bonn-Cologne, Germany
- 6Department of Laboratory Medicine, Gyeongsang National University College of Medicine, Jinju, South Korea
Acinetobacter spp. have emerged as significant pathogens causing nosocomial infections. Treatment of these pathogens has become a major challenge to clinicians worldwide, due to their increasing tendency to antibiotic resistance. To address this, much revenue and technology are currently being dedicated toward developing novel drugs and antibiotic combinations to combat antimicrobial resistance. To address this issue, we have constructed a panel of Acinetobacter spp. strains expressing different antimicrobial resistance determinants such as narrow spectrum β-lactamases, extended-spectrum β-lactamases, OXA-type-carbapenemase, metallo-beta-lactamase, and over-expressed AmpC β-lactamase. Bacterial strains exhibiting different resistance phenotypes were collected between 2008 and 2013 from Severance Hospital, Seoul. Antimicrobial susceptibility was determined according to the CLSI guidelines using agar dilution method. Selected strains were sequenced using Ion Torrent PGM system, annotated using RAST server and analyzed using Geneious pro 8.0. Genotypic determinants, such as acquired resistance genes, changes in the expression of efflux pumps, mutations, and porin alternations, contributing to the relevant expressed phenotype were characterized. Isolates expressing ESBL phenotype consisted of blaPER−1 gene, the overproduction of intrinsic AmpC beta-lactamase associated with ISAba1 insertion, and carbapenem resistance associated with production of carbapenem-hydrolyzing Ambler class D β-lactamases, such as OXA-23, OXA-66, OXA-120, OXA-500, and metallo-β-lactamase, SIM-1. We have analyzed the relative expression of Ade efflux systems, and determined the sequences of their regulators to correlate with phenotypic resistance. Quinolone resistance-determining regions were analyzed to understand fluoroquinolone-resistance. Virulence factors responsible for pathogenesis were also identified. Due to several mutations, acquisition of multiple resistance genes and transposon insertion, phenotypic resistance decision scheme for for evaluating the resistance proved inaccurate, which highlights the urgent need for modification to this scheme. This complete illustration of mechanism contributing to specific resistance phenotypes can be used as a target for novel drug development. It can also be used as a reference strain in the clinical laboratory and for the evaluation of antibiotic efficacy for specific resistance mechanisms.
Introduction
Acinetobacter spp. are non-motile, non-fermenting Gram-negative bacteria. Over the years, several species have been identified, and the most common and clinically significant are Acinetobacter baumannii, Acinetobacter pittii, and Acinetobacter nosocomialis (Chen et al., 2014). These bacteria have emerged as the most troublesome pathogens in hospital settings, due to their rapid colonization and infection. Incidence and mortality due to A. nosocomialis and A. pittii are lower than those due to A. baumannii; however, these are frequently isolated from nosocomial infections (Wisplinghoff et al., 2012). Acinetobacter spp. have been implicated in many pathological conditions such as ventilator-associated pneumonia, urinary tract infections, skin and wound infections, infective endocarditis, bacteremia, and secondary meningitis (Fishbain and Peleg, 2010; Garnacho-Montero and Amaya-Villar, 2010; Visca et al., 2011; Chusri et al., 2014). These infections have become challenging to treat due to their widespread multidrug resistance owing to mechanisms such as horizontal gene transfer, increased expression of β-lactamases, alterations of membrane permeability, and increased expression of efflux pumps (Singh et al., 2013); (Blair et al., 2015).
For several decades, numerous research have been conducted to understand the mechanisms of resistance and to control its dissemination in clinical settings. Considering the severity of infections, we have constructed a series of panel strains of Acinetobacter spp. expressing different resistance phenotypes such as narrow spectrum β-lactamase and oxacillinase, extended spectrum β-lactamase (ESBL), OXA-type carbapenemase, metallo-β-lactamase (MBL), and over-expressing AmpC β-lactamase. These strains were characterized genotypically using massive parallel sequencing (MPS) technology to understand the observed phenotypes. In this study, we have performed detailed analysis of the whole genome sequence (WGS) related to multidrug-resistance mechanisms, such as acquisition of β-lactamases, transposon insertions, mutations in porins, and changes in efflux pumps, and interpreted the discrepancy observed in phenotypic changes to relevant antibiotics. These panel strains can be used in hospital settings as reference strains, and also in the pharmaceutical industry to check the efficacy of new antibiotic drugs on pathogens expressing different resistance determinants. These strains can be distributed world-wide to institutions working on discovery of novel antibiotics, aiding in their characterization.
Materials and Methods
Bacterial Strains
All bacterial strains were collected from Severance Hospital, Seoul from 2008 to 2013. Almost 4,000 strains were shortlisted depending on their in-silico resistance prediction from the hospital patient database, according to the resistance determination decision tree to interpret the type of resistance based on phenotypic observation by François et al. (2004) and Richard Bonnet (2010). Strains were categorized according to their resistance phenotype such as narrow spectrum β-lactamase and oxacillinase, ESBL, OXA-type-carbapenemase, MBL and over-expressed AmpC β-lactamase. Bacteria were identified using the direct colony method with MALDI-TOF MS (Bruker Daltonics, Bremen, Germany). In addition, RNA polymerase β-subunit gene (rpoB)-based identification was used to delineate species within the Acinetobacter genus (La Scola et al., 2006).
Susceptibility Tests
Initially, disc diffusion assays were performed on Muller Hinton agar plates with antibiotic discs containing piperacillin, ampicillin, piperacillin-tazobactam, ceftazidime, cefepime, imipenem, meropenem, ciprofloxacin, ceftazidime-clavulanate, ampicillin-sulbactam, and aztreonam to detect antibiotic susceptibility. In addition, the minimum-inhibitory concentrations (MIC) for bacterial strains were determined using agar dilution technique. E-test was used to measure the MIC of levofloxacin, trimethoprim/sulfamethoxazole, tigecycline, tetracycline, gentamicin, rifampicin, clindamycin, erythromycin and chloramphenicol. All of the procedures and results interpretation followed the Clinical and Laboratory Standards Institute (CLSI) guidelines. AmpC β-lactamase-, MBL, and ESBL-producing strains were selected using ertapenem-amino phenylboronic acid (APBA), imipenem-EDTA, and cefepime-clavulanate double disk synergy tests, respectively (Lee et al., 2001). Modified Hodge tests were also performed with cefoxitin disk for AmpC beta-lactamase detection, and imipenem disk for carbapenemase detection (Lee et al., 2010).
Whole Genome Sequencing and Bioinformatics Analysis
A few strains from each phenotypic resistance class were randomly selected and cultured overnight. Genomic DNA extractions were performed using Wizard genomic DNA purification kit (Promega, WI, USA) with a few modifications to the manufacturer's protocol, such as adding 5 μl of RNase solution during cell lysis as well as incubating the supernatant carrying the DNA at −20°C for 1 h after addition of isopropanol. DNA concentration was measured using Qubit dsDNA BR assay kit (Molecular Probes, OR, USA) before sequencing.
Whole genome libraries were prepared using Ion plus fragment library kit, and Emulsion PCR was carried out using Ion one touch 200 Template kit v2 DL (Life technologies, CA, USA). Sequencing of the amplicon libraries was carried out on a 318 chip, using Ion Personal Genome Machine Ion Torrent sequencer through Ion Sequencing 200 kit (Life technologies, CA, USA). The resultant reads were assembled using MIRA plug-in (version 4.0) of Torrent suite software. Genome assemblies were annotated using RAST annotation pipeline, and further validated with Geneious pro 8.0 (Aziz et al., 2008; Kearse et al., 2012). Genes encoding the efflux systems, porins, and virulence factors of the panel strains were aligned using Clustal Omega, and verified for the polymorphisms (Sievers et al., 2011). Resistance genes were identified using Resfinder (Zankari et al., 2012), and manually curated using NCBI BLAST. Multi-locus sequence typing was performed using MLST 1.8 (Zankari et al., 2013) and Acinetobacter baumannii MLST website (Jolley and Maiden, 2010).
Outer Membrane Protein Detection
Bacterial cells were grown in Muller-Hinton broth until logarithmic phase, and centrifuged at 5,000 g for 15 min, washed twice in 10-mM phosphate buffer, and lysed by sonication at 18–20% amplitude for 2 × 30 s cycles, each comprised 6 × 5 s sonication steps separated by 1 s of no sonication, and 30 s of no sonication between the two cycles. Unbroken cells were eliminated using centrifugation at 3,000 g for 5 min, and outer membrane was solubilized with 2% sodium lauroyl sarcosinate. Insoluble outer membrane fraction was recovered by ultracentrifugation at 25,000 g for 1 h, as described previously (Hernandez-Alles et al., 1999). OMP profiles were determined using SDS-PAGE using Mini-Protean TGX gels (12%), followed by Coomassie blue staining (Bio-Rad, CA, USA). Additionally, OMPs were identified using Matrix-Assisted Laser Desorption-Time of Flight Mass Spectrometry on Tinkerbell LT (ASTA, Suwon, Korea), as described (Pinto et al., 2017). All experiments were repeated three times independently to check for reproducibility of the results.
Quantitative Real-Time RT-qPCR
Total RNA of the 12 Acinetobacter spp. isolates were extracted from exponentially grown bacterial cells with optical density at 600 nm of 0.7–0.8, using RNeasy Mini Kit (Qiagen, Hilden, Germany). Quantity and quality of RNA samples were checked using NanoDrop spectrophotometer (ND- 2000 Thermo scientific, USA). RNA samples with 260/280 ratio from 1.9 to 2.1, 260/230 ratio from 2.0 to 2.5 were used for further analysis. All of the RNA samples were adjusted to the same concentration. Then, 1 μg of total RNA was used to synthesize cDNA by reverse transcription using M-MLV cDNA Synthesis Kit (Enzynomics, Korea) in a 20 μl reaction using 50 μM random hexamers. cDNA was further diluted and stored at −20°C until PCR. Real-time PCR was performed with a 20-μl reaction volume containing 2 μl (100ng) of cDNA, 1X iQ SYBR Green Supermix (Bio-Rad, CA, USA), and gene-specific primers, 300 nM each (for adeB, adeG, adeJ, baeSR, carO, 33-36kDa omp, and oprD genes), on StepOne Real-Time PCR System (Life technologies, CA, USA) with the following cycle: 1 cycle at 95°C for 3 min followed by 40 cycles of 95°C for 10 s and 56°C for 1 min. Dissociation curve was generated to check PCR amplification specificity. In each run, 2 μl RNase-free water was used as a no template control (NTC) for each gene. The primers used for RT-qPCR were designed using Primer3web (version 4.1.0) (Untergasser et al., 2012), validated using Geneious pro 8.0. (Kearse et al., 2012), synthesized commercially by Macrogen, Inc., Korea, and are shown in Table S9. Different primers were used for different species due to the polymorphism identified in efflux pumps. Each experiment was performed in triplicates at least twice independently. The changes in expression level for each gene was calculated according to a previous study (Livak and Schmittgen, 2001). In brief, for each sample, the threshold cycle (Ct) of target gene was determined and normalized to Ct value of rpoB gene, and then calculated relatively to the calibrator (strain YMC/2009/2/B2968) using formula 2−ΔΔCt (data is represented as mean ± standard error). Detailed experimental conditions used in RT-qPCR based on MIQE requirements are described in Table S10.
Results and Discussion
Among the 4,000 Acinetobacter spp. screened initially, we selected 26 isolates showing different phenotypic resistances, i.e., two ESBL-, six high-level AmpC β-lactamase-, ten OXA-type-carbapenemase-, five MBL-, two narrow-spectrum β-lactamase-, one narrow-spectrum oxacillinase-producing strains, in addition to a wild type strain, susceptible to all tested antibiotics (Table S1). Among these YMC2003/5/C86, YMC2003/1/R306 in ESBL's; YMC2009/2/B6756, YMC2012/7/R3167 among over-expressed AmpC beta-lactamase; YMC2011/2/C582, YMC2011/7/R812, YMC2012/1/R79, and YMC2012/9/R2209 in OXA-type-carbapenemase; YMC2013/3/R2081 in MBL; YMC2010/8/T346 in narrow spectrum beta-lactamase; and YMC2009/2/B2968 in narrow-spectrum oxacillinase were randomly picked and sequenced to further characterize the phenotypic and genotypic correlation (Tables 1, 2). The draft genome sequences of strains YMC2003/5/C86, YMC2003/1/R306, YMC2009/2/B6756, YMC2012/7/R3167, YMC2011/2/C582, YMC2011/7/R812, YMC2012/1/R79, YMC2012/9/R2209, YMC2013/3/R2081, YMC2010/8/T346, and YMC2009/2/B2968 have been deposited at DDBJ/ENA/GenBank under the accession MKHG00000000, MKHH00000000, MKHI00000000, MKHJ00000000, MKHK00000000, MKHL00000000, MKHM00000000, MKHN00000000, MKHO00000000, and MKHP00000000, respectively.
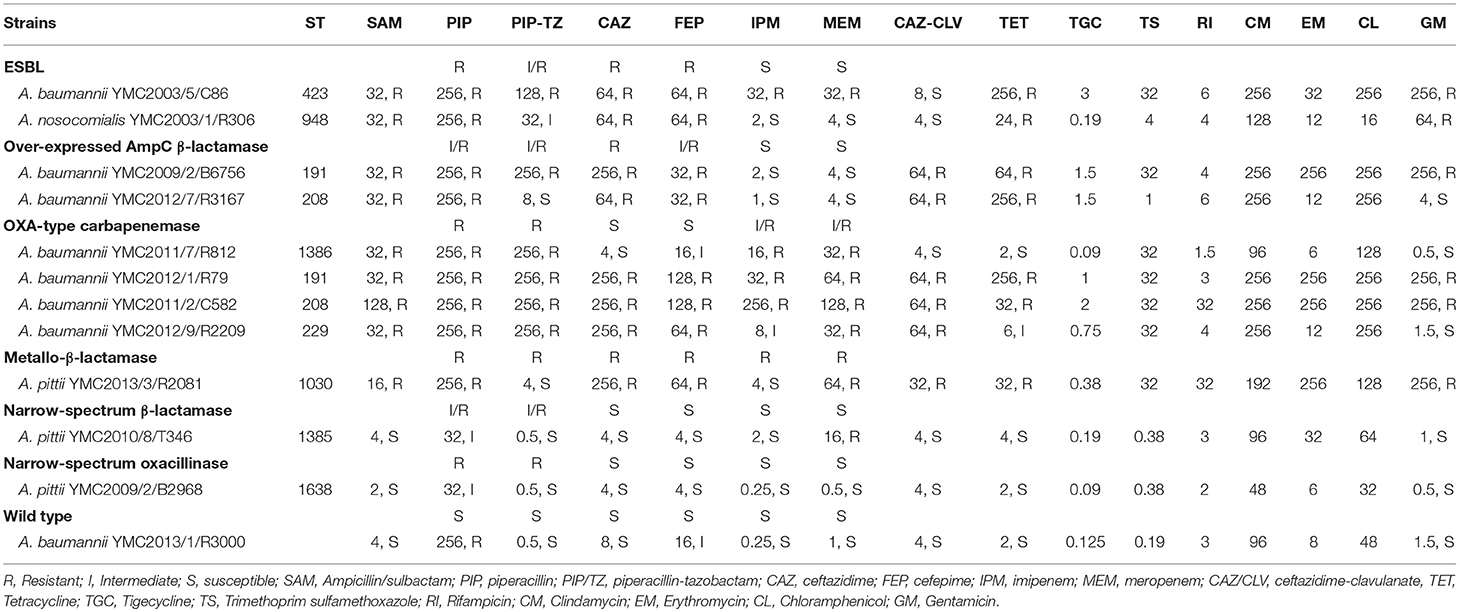
Table 1. Selected list of Acinetobacter spp. panel strains and its minimum inhibitory concentration with its sequence types.
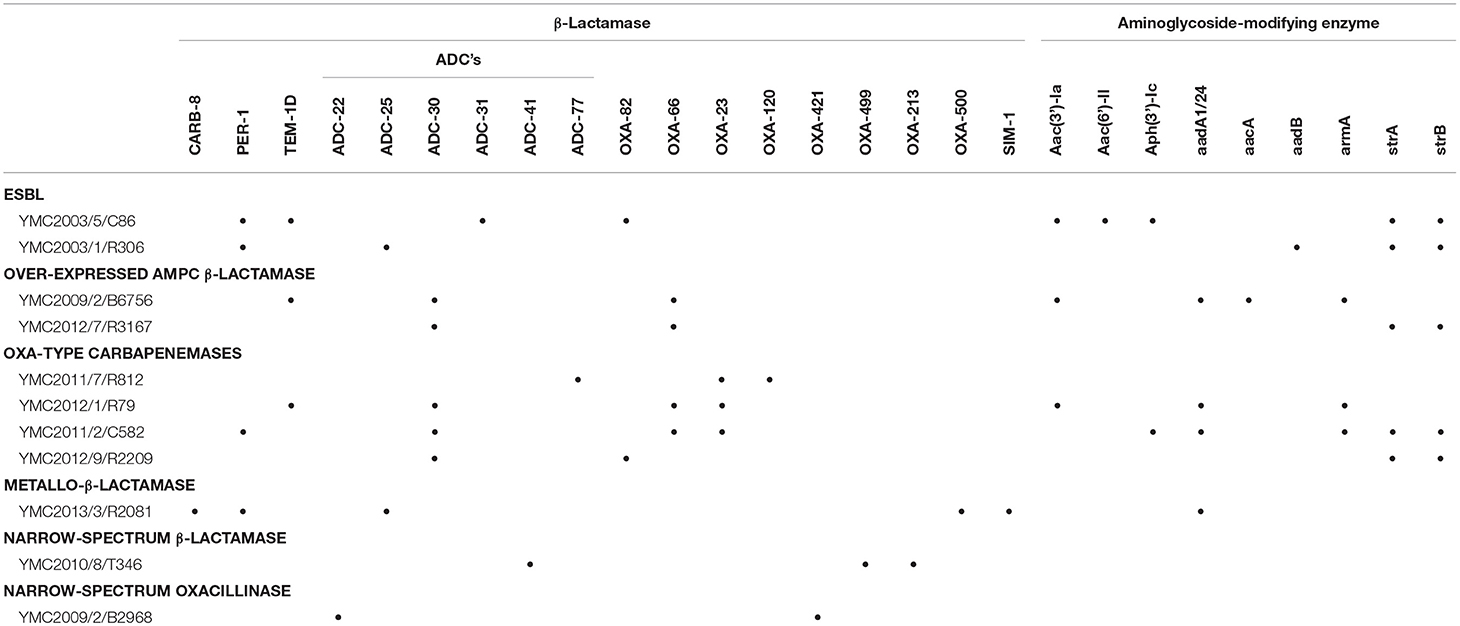
Table 2. Resistome analysis of the Acinetobacter spp. strains representing β-Lactamases and Aminoglycoside-modifying enzymes.
Extended-Spectrum Beta-Lactamases
In Korea a high prevalence of blaPER−1 ESBL-producing Acinetobacter spp. was reported between 2001 and 2005 (Yong et al., 2003), and the level has been reducing over the years. The blaPER−1 belongs to class A extended-spectrum beta-lactamase, which has been detected in P. aeruginosa (Ranellou et al., 2012), P. mirabilis (Pagani et al., 2004), S. enterica (Poirel et al., 2005), and Acinetobacter spp. (Naas et al., 2006), and disseminated worldwide since its first detection in France on 1993 (Nordmann et al., 1993). ESBLs are a class of group A β-lactamases, which hydrolyze third generation cephalosporin's but are inhibited by beta-lactamase inhibitors like clavulanic acid (Bradford, 2001; Jacoby and Munoz-Price, 2005). Antimicrobial susceptibility for beta-lactams is similar in ESBLs and high-level AmpC β-lactamase-producing Acinetobacter spp. We have categorized the strains depending according to the presence of ESBL or AmpC-producing genes, along with IS elements.
a) Acinetobacter baumannii YMC2003/5/C86: This strain was resistant to all antibiotics tested in this study, except ceftazidime-clavulanate. WGS analysis indicated the presence of blaPER−1, blaTEM−1D, blaADC−31, and blaOXA−82. The blaPER−1 gene was flanked by the putative transposase gene tpnA1 and tpnA2 in upstream and downstream region. Insertion sequence ISAba1 was located immediate upstream region of AmpC beta-lactamase gene, blaADC−31 and carbapenemase gene, blaOXA−82 (Zander et al., 2013) (Figure S1). Beta-lactam and cephalosporin resistance of this isolate can be clearly argued by the presence of these encoded β-lactamase genes along with the insertion elements, providing the additional promoters for their increased expression (Lin et al., 2010). Resistance to aminoglycosides and gentamicin are contributed by aac(3′)-Ia, aac(6′)-Il, aph(3′)-Ic, and strAB genes (Tables 1, 2). Levofloxacin resistance was conferred due to the mutations observed in gyrA and parC genes (Table 3). Twenty to seventy-fold up-regulation of adeB and adeJ efflux pumps genes were confirmed, which are assumed to contribute to the resistance of levofloxacin, trimethoprim/sulfamethoxazole, tigecycline, clindamycin, chloramphenicol, and tetracyclines (Table 1, Figure 1).
b) Acinetobacter nosocomialis YMC2003/1/R306 was susceptible to imipenem, meropenem, and ciprofloxacin, intermediate to piperacillin-tazobactam, but resistant to piperacillin, ceftazidime, cefepime, and ampicillin-sulbactam. This isolate is an ideal candidate for ESBL strain, as it carries blaPER−1,which is identified as a part of composite transposon bracketed by two insertion elements ISPa12 and ISPa13, belonging to IS4 family (Figure S2). Expression of this gene was driven by ISPa12 promoter, and its genetic environment is similar to the blaPER−1 found in Providencia stuartii and Pseudomonas aeruginosa isolates, as reported previously (Yong et al., 2003; Poirel et al., 2005). Efflux pumps showed lower expression, which correlated to its increased susceptibility toward fluoroquinolones and tetracyclines (Tables 1, 3, Figure 1).
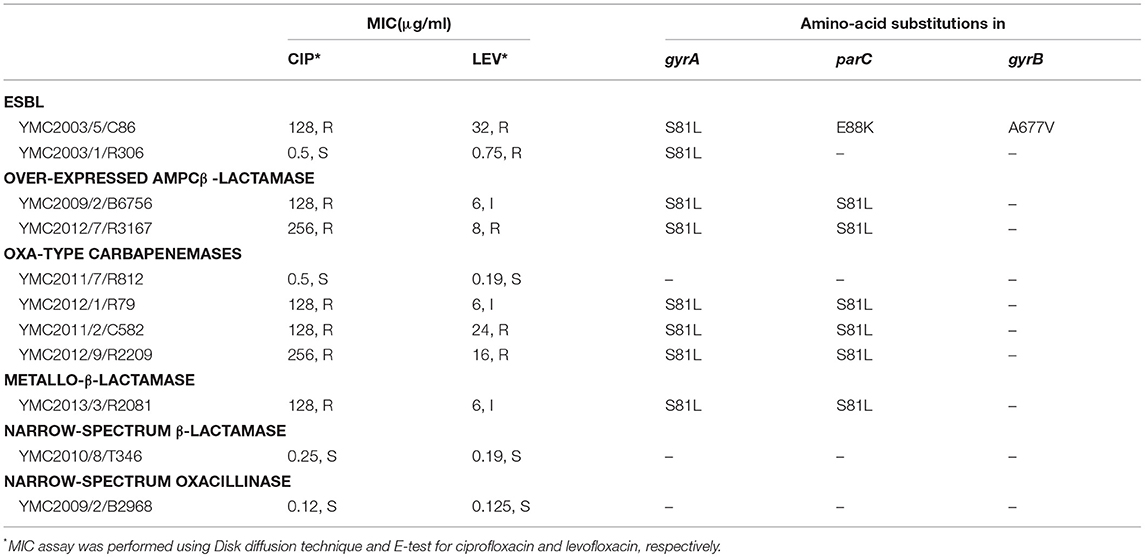
Table 3. MIC of the fluoroquinolone (ciprofloxacin and levofloxacin) and amino-acid substitutions in the QRDR of the gyrA, gyrB, and parC genes of panel strains.
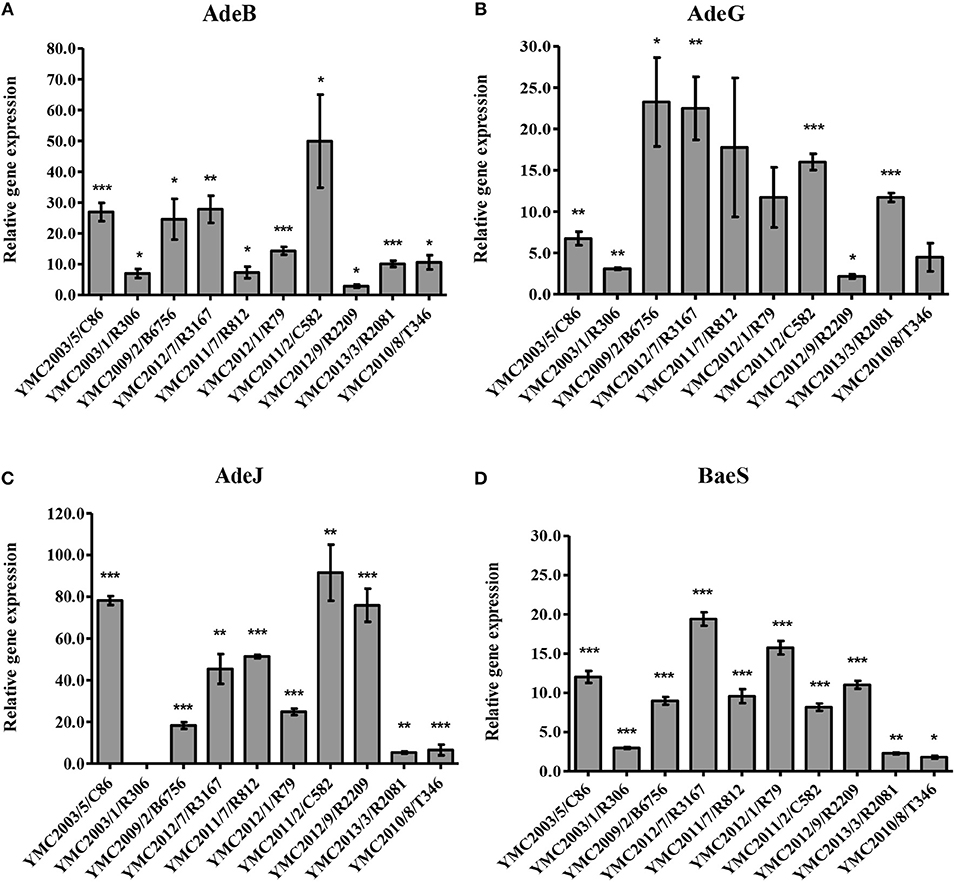
Figure 1. Expression of (A) AdeB, (B) AdeG, (C) AdeJ, and (D) BaeS relative to rpoB. Susceptible strain A. pittii YMC2009/2/B2968 was used as a reference and each isolate was tested in triplicate in two independent experiments. The data represent the mean ± standard error for three independent replicates. The significant difference of expression levels between samples were indicated by bars and asterisks as follows: *p < 0.05, **p < 0.01, and ***p < 0.001 using the Student's t-test.
Over-Expressed AmpC Beta-Lactamase
Overproduction of intrinsic cephalosporinase such as blaADC−25, blaADC−30, or blaADC−56 coupled with insertion elements, such as ISAba1, are responsible for cephalosporin resistance (Lopes and Amyes, 2012).
a) Acinetobacter baumannii YMC2009/2/B6756 was only susceptible to imipenem and meropenem, but resistant to all other antibiotics and beta-lactamase inhibitor combinations used in the study (Table 1). Genomic analysis indicated the presence of blaTEM−1D, blaADC−30, and blaOXA−66 (a blaOXA−51−like gene) (Figure S3). The blaTEM−1D gene in this strain consisted of P3 promoter, which was initially found in Russia contributing to beta-lactam inhibitor-resistance (Edelstein et al., 2000; Leflon-Guibout et al., 2000; Constança and Manuela, 2003). Beta-lactam resistance in this isolate is attributed to the insertion of ISAba1 upstream of AmpC gene, blaADC−30, mediating its over-expression (Li et al., 2015). OXA-66 is the intrinsic OXA-51 variant class D carbapenemase, which does not confer resistance to carbapenems, although it is associated with ISAba1; however, a point mutation converts it into OXA-82, and this variant confers resistance to imipenem and meropenem (Zander et al., 2013) (Figure S3). OXA-82 and OXA-66 are associated with the International clone 2, which is the most prevalent clone found worldwide (Hu et al., 2007; Evans et al., 2008; Evans and Amyes, 2014). Decreased susceptibility toward levofloxacin, tetracycline, trimethoprim/sulfamethoxazole, rifampicin, chloramphenicol, and gentamicin (Tables 1, 3) is contributed by aacA4, aadA1, aac(3)-Ia, armA, and aac(6')Ib-cr genes along with the more than 20-fold increased expression of adeA, adeG, and adeJ efflux pumps compared to the susceptible strain (Magnet et al., 2001; Coyne et al., 2010; Yoon et al., 2013) (Figure 1).
b) Acinetobacter baumannii YMC2012/7/R3167 was susceptible to piperacillin-tazobactam, imipenem, and meropenem, but resistant to ampicillin-sulbactam, piperacillin, ceftazidime, cefepime, ceftazidime-clavulanate (Table 1), and ciprofloxacin (Table 3). Whole genome analysis indicated the presence of β-lactamase genes, blaADC−30, and blaOXA−66. (Hu et al., 2007; Zander et al., 2013) (Figure S4). Further analysis indicated the insertion of ISAba1 upstream of AmpC gene-blaADC−30 which provided a stronger promoter leading to over-expression of AmpC beta-lactamase (Li et al., 2015) leading to multiple beta-lactam resistance. Genetic structure around blaADC−30, and blaOXA−66 of this strain was identical to A. baumannii YMC2009/2/B6756. As opposed to the phenotypic resistance scheme for over-expressed AmpC beta-lactamase class, this strain was susceptible to piperacillin-tazobactam, and we were unable to explain the discrepancy for this phenotype. The expressions of adeB and adeG were similar to A. baumannii YMC2009/2/B6756. High-level resistance to tetracycline was observed due to tet(B) gene (Takahashi et al., 2002).
OXA-Type-Carbapenemases
Carbapenem resistance in Acinetobacter spp. is mediated by various mechanisms such as membrane impermeability due to loss of porins, but it is mostly mediated by enzymatic hydrolysis of antibiotics (Bou et al., 2000; Quale et al., 2003; Bonomo and Szabo, 2006; Poirel and Nordmann, 2006; Nordmann, 2010). Carbapenem-hydrolyzing class D beta-lactamases (CHDLs) or OXA-type-carbapenemases (OXA-51-like, 23-like, -58-like, -143-like, -40-like, and 235-like), often associated with upstream insertion elements, lead to their over-expression resulting in carbapenem resistance (Poirel et al., 2010). Studies have indicated that OXA-40- and OXA-143-type carbapenemases were not associated with insertion sequences nor integrons (Higgins et al., 2009; Evans and Amyes, 2014). Below we have illustrated the mechanism of few strains expressing OXA-type carbapenemases. According to the resistance determination decision tree, these strains were similar to the phenotype observed in metallo-beta lactamase producers, except its susceptibility toward ceftazidime and cefepime. However, due to the complex resistance mechanism involving multiple beta-lactamases and efflux pumps, most of the strains in this class were resistant to these two antibiotics.
a) Acinetobacter baumannii YMC2011/7/R812 was susceptible to ceftazidime, ceftazidime-clavulanate, ciprofloxacin, and levofloxacin, but resistant to ampicillin-sulbactam, piperacillin, piperacillin-tazobactam, imipenem, and meropenem (Tables 1, 3). This strain carried CHDLs such as OXA-120, belonging to OXA-51 family, and OXA-23, along with cephalosporinase ADC-77 (Table 2). There were no insertion sequences located around blaOXA−120 and blaADC−77, keeping their expressions at the basal level (Figure S5-A). However, there was an ISAba1 insertion upstream of blaOXA−23 leading to the overexpression of carbapenemase hydrolyzing activity, along with cefepime resistance (Turton et al., 2006; Lin et al., 2010). As illustrated by Naas and Nordmann (2010) and OXA-type carbapenemase detection scheme, these classes of bacteria are susceptible to ceftazidime and cefepime. This strain was susceptible to fluoroquinolones, tetracyclines, and aminoglycosides (Tables 1, 3) due to absence of adeRS genes, which encode a two-component system regulating AdeABC expression system. In addition, none of the known aminoglycoside and fluoroquinolone resistant genes were present (Tables 1, 3). In addition, adeC gene was also absent, along with truncation of adeA gene (Figure S5-B). The genetic structure around blaOXA−120 from A. baumannii YMC2011/7/R812 and blaOXA−66 from ESBL-producing A. baumannii YMC2009/2/B6756 and YMC2012/7/R3167 were identical, as both the beta-lactamases belongs to OXA-51-like group (Rafei et al., 2015).
b) Acinetobacter baumannii YMC2012/1/R79 was resistant to all of the antibiotics used in this study. This strain carried blaTEM−1D, blaADC−30, blaOXA−66, and CHDL, blaOXA−23. The multi-drug resistant phenotype of this strain was contributed by ISAba1-blaOXA−23 and ISAba1-blaADC−30 genes (Turton et al., 2006; Lin et al., 2010) (Figure S6). Resistance to aminoglycoside were seen due to the presence of aadA1, aadA24, armA, and aac(6′)Ib-cr genes, resistance to fluoroquinolones were due to the mutations in gyrA and parC genes, along with the moderately increased expressions of adeB, adeG, and adeJ efflux pumps (Table 1, Figure 1).
c) Acinetobacter baumannii YMC2011/2/C582 was resistant to all of the antibiotics and beta-lactam inhibitors used in this study for phenotypic screening (Table 1). WGS analysis indicated the presence of ESBL gene, blaPER−1, and wide variety of other β-lactamase genes, such as blaOXA−66, blaOXA−23, and blaADC−30 (Table 2). The blaPER−1 gene and partial glutathione-S-transferase were bracketed by ISPa12 and ISPa13, belonging to the IS4 family, regulating the expression of blaPER−1 gene driven by promoter sequences in ISPa12 (Poirel et al., 2005), similar to A. nosocomialis YMC2003/1/R306 strain (Figures S2, S7). In addition, there was insertion of ISAba1 upstream of blaADC−30 and blaOXA−23, providing additional promoter leading to increased resistance due to overexpressions of AmpC beta-lactamase and carbapenemase, respectively. Increased expression of adeb, adeG, and adeJ, along with aminoglycoside and fluoroquinolones resistance genes such as armA, aph(3′)-Ic, strAB, aph(3′)-VIb, aadA1, and aac(6′)Ib-cr decreased the susceptibility toward gentamicin, tetracycline, trimethoprim/sulfamethoxazole, rifampicin, and chloramphenicol (Table 1, Figure 1). In addition, mutations were observed in gyrA and parC genes, which caused levofloxacin resistance (Table 3).
d) Acinetobacter baumannii YMC2012/9/R2209 was intermediate to imipenem but resistant to all other cephalosporin and carbapenems used in our study (Table 1). This isolate was AmpC beta-lactamase hyper-producer along with CHDL, which was revealed by the presence of ISAba1-blaOXA−82 and ISAba1-blaADC−30 (Figure S8). Increased carbapenem resistance was caused by ISAba1-blaOXA−82 (Zander et al., 2013). Susceptibility toward tigecycline, gentamicin and tetracycline were due to the absence of aminoglycoside resistance genes and lower expressions of adeB and adeG efflux pumps (Table 1, Figure 1). In contrast, increased relative expression of adeJ gene might have increased resistance to fluoroquinolones, such as ciprofloxacin and levofloxacin, along with gyrA and parC genes mutations.
MBL
MBL-producing Acinetobacter spp. have become an emerging therapeutic concern worldwide. Along with CHDLs, carbapenem resistance is attributed to MBLs such as IMP, VIM, GIM, SIM etc. (Kim et al., 2014). According to the resistance detection scheme, Acinetobacter spp. producing MBLs display similar phenotypic resistance as OXA-type carbapenemases, except the latter showing its susceptibility toward ceftazidime and cefepime. MBL producing A. pittii YMC2013/3/R2081 was susceptible to piperacillin-tazobactam and imipenem but resistant to ampicillin-sulbactam, piperacillin, ceftazidime, cefepime, meropenem, ceftazidime-clavulanate, and ciprofloxacin. This bacterium contains blaCARB−8, blaPER−1, blaADC−18, blaOXA−500, and blaSIM−1 (Tables 1, 2). Resistance to most of antibiotics can be explained due to ESBL gene along with IS element, ISCR1-blaPER−1, and MBL gene, blaSIM−1 (Figure S9). Despite SIM-1 production, this bacterium was susceptible to imipenem due to its strong activity against Acinetobacter spp (Lee et al., 2005). Genetic analysis indicated that blaSIM−1 along with aar-3, carB3, and aadA1 genes were encoded by class 1 integron. The blaCARB−8 is carbencillin-hydrolyzing beta-lactamase, which has the same hydrolytic profile as blaCARB−5 (Choury et al., 2000). This enzyme has been previously identified in various species such as Oligella urethralis, Vibrio cholerae, Achromobacter xylosoxidans, A. baumannii, and Salmonella typhimurium, which indicates inter-genus transferability of the gene (Decre et al., 1995; Ridley and Threlfall, 1998; Choury et al., 1999, 2000; Lin et al., 2010). Increased resistance to gentamicin was mediated by the aac(3)-IId gene (Ho et al., 2010), despite lower expression of adeB, adeG, and adeJ efflux pumps (Table 1, Figure 1).
Narrow Spectrum β-Lactamase
Acinetobacter pittii YMC2010/8/T346 belongs to a novel sequence type 1385 (ST 1385), and is susceptible to ampicillin-sulbactam, piperacillin-tazobactam, ceftazidime, cefepime, imipenem, ceftazidime-clavulanate, and ciprofloxacin but resistant to meropenem (Tables 1, 3). Sequence analysis indicated the presence of blaOXA−506, variant of A. pittii intrinsic blaOXA−213−like, blaADC−41, and blaOXA−499, which were not associated with insertion elements (Table 2, Figure S10). The blaOXA−499 is a novel variant of carbapenem hydrolyzing oxacillinase, blaOXA−143. This gene was first found in South Korea, and is the carbapenem hydrolyzing gene which explains its resistance to the meropenem, as reported previously (D'Souza et al., 2017). Wide susceptibility toward aminoglycosides, tetracyclines, and fluoroquinolones was observed due to the lower expression of efflux pumps and absence of any corresponding resistance genes (Tables 1, 3, Figure 1).
Narrow Spectrum Oxacillinase
Acinetobacter pittii YMC2009/2/B2968 belonging to novel ST1638, was not resistant to the antibiotics tested in this study (Table 1). Whole genome analysis revealed blaOXA−421, a CHDL belonging to A. pittii intrinsic blaOXA−213 family and blaADC−22 (Table 2, Figure S11). However, no existing study has yet demonstrated that the carbapenemase activity of blaOXA421. blaADC−22 is a naturally occurring cephalosporinase gene in A. baumannii, which is repressed under normal conditions (Beceiro et al., 2009; Li et al., 2015). This strain exhibited the highest susceptibility toward aminoglycosides, tetracyclines, and fluoroquinolones among all other panel strains, due to the absence of corresponding resistance genes and lowest expression of efflux pumps. Therefore, this was selected as the reference strain to calculate the relative expression of efflux pumps for other strains.
Analysis of QRDRs for gyrA and parC Genes and Fluoroquinolone Resistance
The MICs of ciprofloxacin and levofloxacin were determined (Table 3). Both antibiotics functioned by inhibiting DNA gyrase subunit A (GyrA), DNA gyrase subunit B (GyrB), and toposiomerase IV subunit C (ParC) (Drlica and Zhao, 1997), and hence exhibited similar resistance phenotypes for the panel strains. Resistance to fluoroquinolone in bacteria was mediated by spontaneous mutations in gyrA, gyrB, and parC genes (Park et al., 2011; Ardebili et al., 2015). We identified the substitutions in GyrA (Ser81Leu) and ParC (Ser84Leu) in all fluoroquinolone resistant strains (Table 3). Ser467Gly and Glu88Lys mutation in ParC did not correlate with the resistance phenotypes. As opposed to the previous studies, we found GyrA (Ser81Leu) and ParC (Ser467Gly) mutations in A. nosocomialis YMC2003/1/R306, which were susceptible to fluoroquinolone (Vila et al., 1995). We could not find Glu479Asp, Cys423Ser, Glu479Asp, Leu420Gln, Cys423Ser, Leu433His, Glu479Asp, and D644Y mutations in GyrB which were previously described as novel substitutions (Park et al., 2011), except A677V in A. baumannii YMC2003/5/C86.
Efflux-Mediated Antimicrobial Resistance
Overexpression of efflux pumps are one of the major mechanisms that contribute to the multidrug resistance in Acinetobacter species. Genes encoding these systems are carried by mobile genetic elements or chromosomes, and thus be responsible for acquired or intrinsic resistance (Coyne et al., 2011). Five categories of efflux pump systems have been described, which are responsible for pumping out diverse classes of antibiotics: resistance-nodulation-cell division (RND) family, ATP-binding cassette (ABC) transporters, major facilitator superfamily (MFS), small multidrug resistance (SMR) family, and the recently identified multidrug and toxic compound extrusion (MATE) family (Piddock, 2006; Vila et al., 2007). Considering the broad-range substrate specificity of the three RND-type efflux pump systems, AdeABC, AdeFGH, and AdeIJK, we investigated the expressions of adeB, adeG, and adeJ genes (Figures 1A–C). Reference gene rpoB was used as a control, and susceptible strain A. pittii YMC2009/2/B2968 was used as a reference. Tigecycline appeared to be the best substrate for adeB pump, which correlated with their increased resistance and seven to 50-fold increase in its expression. This was consistent with previous findings (Perez et al., 2007; Ruzin et al., 2007; Hornsey et al., 2010) (Table 1, Figure 1). In addition, decreased susceptibility toward tetracycline, trimethoprim/sulfamethoxazole, and gentamicin also correlated with the increased expression with few exceptions. We screened for mutations in AdeRS, a two-component regulator system that controls the expression of AdeRS. G186V substitution in AdeS and A136V in AdeR was detected in all of the isolates overexpressing adeB gene, which was previously linked to increased tigecycline resistance (Hornsey et al., 2010; Rumbo et al., 2013) (Tables S3, S4). The isolate A. baumannii YMC2011/7/R812 did not contain adeRS, adeA, and adeC genes (Table S2). The adeC gene was also absent from A. baumannii YMC2012/9/R2209 and all A. pittii strains (Table S2). The expressions of adeG and adeJ were variable and strain-specific. Therefore, we could not find the suitable phenotypic marker regulating the pump. Overall, A. baumannii isolates showed increased expression of three RND efflux systems compared to A. pittii and A. nosocomialis. AdeFGH and its LysR-type transcriptional regulator AdeL were present in all strains (Table S5). TetR transcriptional repressor AdeN, controlling AdeIJK were interrupted by ISAba1 insertion sequence in A. baumannii YMC2012/9/R2209, YMC2012/7/R3167, and YMC2011/2/C582, which increased AdeIJK expression (Rosenfeld et al., 2012) (Table S5). In addition, we were unable to correlate the expression of BaeSR two-component system, which was previously known to influence tigecycline susceptibility by regulating adeABC genes (Lin et al., 2014) (Figure 1D). The limitation of our qRT-PCR was using different primers for different species due to the polymorphism identified in efflux pumps. This might have led to different amplicon kinetics resulting in errors in differential expressions. Finally, we could also detect the genes related to non-RND efflux pumps such as cra, amvA, abeM, abeS, and adeXYZ in all of the Acinetobacter strains. The adeDE gene was identified in YMC2003/1/R306 and YMC2013/3/R2081, and cmlA was present only in isolate YMC2013/3/R2081 (Table S6).
Role of Porins in Resistance
Porins play a vital role in the mechanism of carbapenem resistance in Enterobacteriaceae. However, in Acinetobacter spp., their contributions toward resistance are debated, and their functions remain ambiguous (Marti et al., 2006). Previous studies indicated that loss of porins such as CarO, OprD, and 33-36Kda Omp conferred carbapenem resistance (Bou et al., 2000; Fernandez-Cuenca et al., 2003; Mussi et al., 2005; Siroy et al., 2005; Peleg et al., 2008). To determine the potential role of these porins in resistance, we performed SDS-PAGE (data not shown) and MALDI-TOF (Figure S12). All of the panel strains showed identical OMP profiles, which were also confirmed by WGS analysis (Table S7). These results suggested that the porins did not have any role in carbapenem resistance among the panel strains. In addition, qRT-PCR for CarO, oprD, and 33-36Kda Omp did not show any significant correlation to antimicrobial resistance (Figure S13).
Virulence Factors
Understanding the pathogenesis, along with its multi-drug resistance phenotype, is highly essential for infection control and investigation of alternate treatment options. The development of infection, and bacterial survival in the host depends on virulence factors such as biofilm formation, serum resistance, evasion of the host immune response, motility, host cell apoptosis, bacterial dissemination, transfer of genetic material between bacterial cells, and iron acquisition mechanisms (Choi et al., 2005; Jacobs et al., 2010; Luke et al., 2010; Jin et al., 2011; Gaddy et al., 2012; McConnell et al., 2013). Virulence factors capsular polysaccharide (ptk and epsA), phospholipase D, and penicillin-binding protein (pbpG) were present in all of the panel strains (Table S8). Virulent genes associated with biofilm formation, such as OmpA and BfmR, the response regulator component of two-component system BfmRS, were present in all of the strains (Gaddy et al., 2009; Liou et al., 2014). However, another key virulent gene, bap (Biofilm-associated protein), was absent in YMC2011/7/R812, YMC2012/9/R2209, YMC2013/3/R2081, and YMC2009/2/B2968 (Badmasti et al., 2015). Outer membrane proteins, CsuA/B, CsuC, and CsuD were absent from YMC2011/7/R812 and YMC2010/8/T346. Acinetobacter nosocomialis YMC2003/1/R306 did not carry the genes involved in acinetobactin-mediated iron acquisition system such as bauA, bauB, bauC, bauD, bauE, basC, and basD, and we did not find homologs of these systems either.
In summary, all of the panel strains in our study were shortlisted depending on the resistance scheme given by François et al. (2004) and Naas and Nordmann in Antibiogram (Naas and Nordmann, 2010). Similar to our previous study in Klebsiella pneumoniae (Dsouza et al., 2017), we found several discrepancies in the detection scheme. The ESBL strain YMC2003/5/C86 isolated in our study was resistant to carbapenems due to presence of OXA-82, albeit the scheme indicates that ESBL strains should be susceptible to carbapenems. Similarly, it also indicates that OXA-type carbapenemases are susceptible to ceftazidime and cefepime. However, the isolated strains in this study were resistant to both antibiotics. Therefore, we suggest the scheme to be updated and modified considering the novel mutations, acquisition of multiple resistance genes, and transposon insertion, for better detection. The main drawback of this study was characterizing unequal number of strains in each resistance classes. Strains were obtained retrospectively and therefore, limiting the number of strains.
The basic rule in the pharmaceutical industry for developing new antibiotics, or for clinicians prescribing antibacterial therapy, depends on comprehensive understanding of the mechanism(s) of resistance. For some time now, Acinetobacter spp. have been implicated in several pathological conditions, and constant efforts are being undertaken to control the spread of these organisms in hospital and community settings (Maragakis and Perl, 2008; Vila and Pachon, 2008; Metan et al., 2009; Garnacho-Montero and Amaya-Villar, 2010; Evans et al., 2013; Wisplinghoff and Seifert, 2014; Dramowski et al., 2015). There are several mechanisms suggested for Acinetobacter spp. resistance for β-lactams and other antibiotics that we have outlined in this study. In hospital settings and research laboratories, it is quite common to encounter these pathogens with various resistance phenotypes. The genotypic and phenotypic correlations in our study would definitely help clinicians and researchers to better understand the mechanism associated, along with utilizing these pathogens as reference strains. In addition, these panel strains would be highly beneficial for evaluating the efficacy of novel antibiotics or antibiotic kit on Acinetobacter spp. displaying different resistance phenotypes. An in-depth study involving the genetic mechanism conferring resistance can open many opportunities for novel drug target study and ways to control the antimicrobial resistance. We have studied the role of various resistance genes attributing to the specific resistance in detail, by referring to previous publications. Therefore, we believe that we have constructed a single platform consisting of various resistance genes illustrating its role, which can help antimicrobial researchers to understand the basics of antimicrobial resistance. Further, studies could be warranted to determine the lineage analysis on this strain and also understand the expression of virulence factors contributing toward the bacterial pathogenesis.
Author Contributions
DY, JC, and YC designed the study and secured the funding. RD, NP, NLP, and TV performed the experiments. RD, PH, J-HB, and DY analyzed and interpreted the data and wrote the manuscript.
Funding
This work was supported by Korea Institute of Planning and Evaluation for Technology in Food, Agriculture, Forestry and Fisheries (IPET) through Agricultural Microbiome R&D Program, funded by Ministry of Agriculture, Food and Rural Affairs (MAFRA) (918003-4); by Nano Material Technology Development Program through the National Research Foundation of Korea (NRF) funded by the Ministry of Science and ICT (No.2017M3A7B4039936); by the Korea Health Technology R&D Project through the Korea Health Industry Development Institute (KHIDI), funded by the Ministry of Health & Welfare, Republic of Korea (grant number HI17C1807).
Conflict of Interest Statement
The authors declare that the research was conducted in the absence of any commercial or financial relationships that could be construed as a potential conflict of interest.
Supplementary Material
The Supplementary Material for this article can be found online at: https://www.frontiersin.org/articles/10.3389/fmicb.2019.00559/full#supplementary-material
References
Ardebili, A., Lari, A. R., Beheshti, M., and Lari, E. R. (2015). Association between mutations in gyrA and parC genes of Acinetobacter baumannii clinical isolates and ciprofloxacin resistance. Iran. J. Basic Med. Sci. 18, 623–626.
Aziz, R. K., Bartels, D., Best, A. A., DeJongh, M., Disz, T., Edwards, R. A., et al. (2008). The RAST Server: rapid annotations using subsystems technology. BMC Genomics 9:75. doi: 10.1186/1471-2164-9-75
Badmasti, F., Siadat, S. D., Bouzari, S., Ajdary, S., and Shahcheraghi, F. (2015). Molecular detection of genes related to biofilm formation in multidrug-resistant Acinetobacter baumannii isolated from clinical settings. J. Med. Microbiol. 64(Pt 5), 559–564. doi: 10.1099/jmm.0.000058
Beceiro, A., Perez, A., Fernandez-Cuenca, F., Martinez-Martinez, L., Pascual, A., Vila, J., et al. (2009). Genetic variability among ampC genes from Acinetobacter genomic species 3. Antimicrob. Agents Chemother. 53, 1177–1184. doi: 10.1128/AAC.00485-08
Blair, J. M., Webber, M. A., Baylay, A. J., Ogbolu, D. O., and Piddock, L. J. (2015). Molecular mechanisms of antibiotic resistance. Nat. Rev. Microbiol. 13, 42–51. doi: 10.1038/nrmicro3380
Bonomo, R. A., and Szabo, D. (2006). Mechanisms of multidrug resistance in Acinetobacter species and Pseudomonas aeruginosa. Clin. Infect. Dis. 43 (Suppl. 2), S49–S56. doi: 10.1086/504477
Bou, G., Cervero, G., Dominguez, M. A., Quereda, C., and Martinez-Beltran, J. (2000). Characterization of a nosocomial outbreak caused by a multiresistant Acinetobacter baumannii strain with a carbapenem-hydrolyzing enzyme: high-level carbapenem resistance in A. baumannii is not due solely to the presence of beta-lactamases. J. Clin. Microbiol. 38, 3299–3305.
Bradford, P. A. (2001). Extended-spectrum β-lactamases in the 21st century: characterization, epidemiology, and detection of this important resistance threat. Clin. Microbiol. Rev. 14, 933–951. doi: 10.1128/CMR.14.4.933-951.2001
Chen, T. L., Lee, Y. T., Kuo, S. C., Yang, S. P., Fung, C. P., and Lee, S. D. (2014). Rapid identification of Acinetobacter baumannii, Acinetobacter nosocomialis and Acinetobacter pittii with a multiplex PCR assay. J Med Microbiol 63(Pt 9), 1154–1159. doi: 10.1099/jmm.0.071712-0
Choi, C. H., Lee, E. Y., Lee, Y. C., Park, T. I., Kim, H. J., Hyun, S. H., et al. (2005). Outer membrane protein 38 of Acinetobacter baumannii localizes to the mitochondria and induces apoptosis of epithelial cells. Cell Microbiol. 7, 1127–1138. doi: 10.1111/j.1462-5822.2005.00538.x
Choury, D., Aubert, G., Szajnert, M. F., Azibi, K., Delpech, M., and Paul, G. (1999). Characterization and nucleotide sequence of CARB-6, a new carbenicillin-hydrolyzing beta-lactamase from Vibrio cholerae. Antimicrob. Agents Chemother. 43, 297–301. doi: 10.1128/AAC.43.2.297
Choury, D., Szajnert, M. F., Joly-Guillou, M. L., Azibi, K., Delpech, M., and Paul, G. (2000). Nucleotide sequence of the bla(RTG-2) (CARB-5) gene and phylogeny of a new group of carbenicillinases. Antimicrob. Agents Chemother. 44, 1070–1074. doi: 10.1128/AAC.44.4.1070-1074.2000
Chusri, S., Chongsuvivatwong, V., Rivera, J. I., Silpapojakul, K., Singkhamanan, K., McNeil, E., et al. (2014). Clinical outcomes of hospital-acquired infection with Acinetobacter nosocomialis and Acinetobacter pittii. Antimicrob. Agents Chemother. 58, 4172–4179. doi: 10.1128/AAC.02992-14
Constança, P -F, and Manuela, C. (2003). A novel sequence framework (bla(TEM-1G)) encoding the parental TEM-1 beta-lactamase. FEMS Microbiol. Lett. 220, 177–180. doi: 10.1016/S0378-1097(03)00123-X
Coyne, S., Courvalin, P., and Perichon, B. (2011). Efflux-mediated antibiotic resistance in Acinetobacter spp. Antimicrob. Agents Chemother. 55, 947–953. doi: 10.1128/AAC.01388-10
Coyne, S., Rosenfeld, N., Lambert, T., Courvalin, P., and Perichon, B. (2010). Overexpression of resistance-nodulation-cell division pump AdeFGH confers multidrug resistance in Acinetobacter baumannii. Antimicrob. Agents Chemother. 54, 4389–4393. doi: 10.1128/AAC.00155-10
Decre, D., Arlet, G., Bergogne-Berezin, E., and Philippon, A. (1995). Identification of a carbenicillin-hydrolyzing beta-lactamase in Alcaligenes denitrificans subsp. xylosoxydans. Antimicrob. Agents Chemother. 39, 771–774. doi: 10.1128/AAC.39.3.771
Dramowski, A., Cotton, M. F., Rabie, H., and Whitelaw, A. (2015). Trends in paediatric bloodstream infections at a South African referral hospital. BMC Pediatr. 15:33. doi: 10.1186/s12887-015-0354-3
Drlica, K., and Zhao, X. (1997). DNA gyrase, topoisomerase IV, and the 4-quinolones. Microbiol. Mol. Biol. Rev. 61, 377–392.
D'Souza, R., Pinto, N. A., Higgins, P. G., Hwang, I., Yong, D., Choi, J., et al. (2017). First report of the carbapenemase gene blaOXA-499 in Acinetobacter pittii. Antimicrob. Agents Chemother. 61, e02676–16. doi: 10.1128/AAC.02676-16
Dsouza, R., Pinto, N. A., Hwang, I., Cho, Y., Yong, D., Choi, J., et al. (2017). Panel strain of Klebsiella pneumoniae for beta-lactam antibiotic evaluation: their phenotypic and genotypic characterization. PeerJ. 5:e2896. doi: 10.7717/peerj.2896
Edelstein, M., Suvorov, M., Edelstein, I., and Kozlov, R. (2000). “Identification of the naturally occurring variant genes blaTEM-1d and blaTEM-70 encoding broad-spectrum TEM-Type b-lactamases,” in 10th European Congress of Clinical Microbiology and Infectious Diseases (Stockholm).
Evans, B. A., and Amyes, S. G. (2014). OXA beta-lactamases. Clin. Microbiol. Rev. 27, 241–263. doi: 10.1128/CMR.00117-13
Evans, B. A., Hamouda, A., and Amyes, S. G. (2013). The rise of carbapenem-resistant Acinetobacter baumannii. Curr. Pharm. Des. 19, 223–238. doi: 10.2174/138161213804070285
Evans, B. A., Hamouda, A., Towner, K. J., and Amyes, S. G. (2008). OXA-51-like beta-lactamases and their association with particular epidemic lineages of Acinetobacter baumannii. Clin. Microbiol. Infect. 14, 268–275. doi: 10.1111/j.1469-0691.2007.01919.x
Fernandez-Cuenca, F., Martinez-Martinez, L., Conejo, M. C., Ayala, J. A., Perea, E. J., and Pascual, A. (2003). Relationship between beta-lactamase production, outer membrane protein and penicillin-binding protein profiles on the activity of carbapenems against clinical isolates of Acinetobacter baumannii. J. Antimicrob. Chemother. 51, 565–574. doi: 10.1093/jac/dkg097
Fishbain, J., and Peleg, A. Y. (2010). Treatment of Acinetobacter infections. Clin. Infect. Dis. 51, 79–84. doi: 10.1086/653120
François, J., Monique, C., Michèle, W, and Alain, G. (2004). From Antibiogram to Prescription. France: bioMérieux.
Gaddy, J. A., Arivett, B. A., McConnell, M. J., Lopez-Rojas, R., Pachon, J., and Actis, L. A. (2012). Role of acinetobactin-mediated iron acquisition functions in the interaction of Acinetobacter baumannii strain ATCC 19606T with human lung epithelial cells, Galleria mellonella caterpillars, and mice. Infect. Immun. 80, 1015–1024. doi: 10.1128/IAI.06279-11
Gaddy, J. A., Tomaras, A. P., and Actis, L. A. (2009). The Acinetobacter baumannii 19606 OmpA protein plays a role in biofilm formation on abiotic surfaces and in the interaction of this pathogen with eukaryotic cells. Infect. Immun. 77, 3150–3160. doi: 10.1128/IAI.00096-09
Garnacho-Montero, J., and Amaya-Villar, R. (2010). Multiresistant Acinetobacter baumannii infections: epidemiology and management. Curr. Opin. Infect. Dis. 23, 332–339. doi: 10.1097/QCO.0b013e32833ae38b
Hernandez-Alles, S., Alberti, S., Alvarez, D., Domenech-Sanchez, A., Martinez-Martinez, L., Gil, J., et al. (1999). Porin expression in clinical isolates of Klebsiella pneumoniae. Microbiology 145 (Pt 3), 673–679. doi: 10.1099/13500872-145-3-673
Higgins, P. G., Poirel, L., Lehmann, M., Nordmann, P., and Seifert, H. (2009). OXA-143, a novel carbapenem-hydrolyzing class D beta-lactamase in Acinetobacter baumannii. Antimicrob. Agents Chemother. 53, 5035–5038. doi: 10.1128/AAC.00856-09
Ho, P. L., Wong, R. C., Lo, S. W., Chow, K. H., Wong, S. S., and Que, T. L. (2010). Genetic identity of aminoglycoside-resistance genes in Escherichia coli isolates from human and animal sources. J. Med. Microbiol. 59(Pt 6), 702–707. doi: 10.1099/jmm.0.015032-0
Hornsey, M., Ellington, M. J., Doumith, M., Thomas, C. P., Gordon, N. C., Wareham, D. W., et al. (2010). AdeABC-mediated efflux and tigecycline MICs for epidemic clones of Acinetobacter baumannii. J. Antimicrob. Chemother. 65, 1589–1593. doi: 10.1093/jac/dkq218
Hu, W. S., Yao, S. M., Fung, C. P., Hsieh, Y. P., Liu, C. P., and Lin, J. F. (2007). An OXA-66/OXA-51-like carbapenemase and possibly an efflux pump are associated with resistance to imipenem in Acinetobacter baumannii. Antimicrob. Agents Chemother. 51, 3844–3852. doi: 10.1128/AAC.01512-06
Jacobs, A. C., Hood, I., Boyd, K. L., Olson, P. D., Morrison, J. M., Carson, S., et al. (2010). Inactivation of phospholipase D diminishes Acinetobacter baumannii pathogenesis. Infect. Immun. 78, 1952–1962. doi: 10.1128/IAI.00889-09
Jacoby, G. A., and Munoz-Price, L. S. (2005). The new beta-lactamases. N. Engl. J. Med. 352, 380–391. doi: 10.1056/NEJMra041359
Jin, J. S., Kwon, S. O., Moon, D. C., Gurung, M., Lee, J. H., Kim, S. I., et al. (2011). Acinetobacter baumannii secretes cytotoxic outer membrane protein A via outer membrane vesicles. PLoS ONE 6:e17027. doi: 10.1371/journal.pone.0017027
Jolley, K. A., and Maiden, M. C. (2010). BIGSdb: scalable analysis of bacterial genome variation at the population level. BMC Bioinform. 11:595. doi: 10.1186/1471-2105-11-595
Kearse, M., Moir, R., Wilson, A., Stones-Havas, S., Cheung, M., Sturrock, S., et al. (2012). Geneious basic: an integrated and extendable desktop software platform for the organization and analysis of sequence data. Bioinformatics 28, 1647–1649. doi: 10.1093/bioinformatics/bts199
Kim, U. J., Kim, H. K., An, J. H., Cho, S. K., Park, K. H., and Jang, H. C. (2014). Update on the epidemiology, treatment, and outcomes of carbapenem-resistant Acinetobacter infections. Chonnam. Med. J. 50, 37–44. doi: 10.4068/cmj.2014.50.2.37
La Scola, B., Gundi, V. A., Khamis, A., and Raoult, D. (2006). Sequencing of the rpoB gene and flanking spacers for molecular identification of Acinetobacter species. J. Clin. Microbiol. 44, 827–832. doi: 10.1128/JCM.44.3.827-832.2006
Lee, K., Chong, Y., Shin, H. B., Kim, Y. A., Yong, D., and Yum, J. H. (2001). Modified Hodge and EDTA-disk synergy tests to screen metallo-beta-lactamase-producing strains of Pseudomonas and Acinetobacter species. Clin. Microbiol. Infect. 7, 88–91. doi: 10.1046/j.1469-0691.2001.00204.x
Lee, K., Kim, C. K., Yong, D., Jeong, S. H., Yum, J. H., Seo, Y. H., et al. (2010). Improved performance of the modified hodge test with macconkey agar for screening carbapenemase-producing Gram-negative bacilli. J. Microbiol. Methods 83, 149–152. doi: 10.1016/j.mimet.2010.08.010
Lee, K., Yum, J. H., Yong, D., Lee, H. M., Kim, H. D., Docquier, J. D., et al. (2005). Novel acquired metallo-beta-lactamase gene, bla(SIM-1), in a class 1 integron from Acinetobacter baumannii clinical isolates from Korea. Antimicrob. Agents Chemother. 49, 4485–4491. doi: 10.1128/AAC.49.11.4485-4491.2005
Leflon-Guibout, V., Heym, B., and Nicolas-Chanoine, M. (2000). Updated sequence information and proposed nomenclature for bla(TEM) genes and their promoters. Antimicrob. Agents Chemother. 44, 3232–3234. doi: 10.1128/AAC.44.11.3232-3234.2000
Li, H., Liu, F., Zhang, Y., Wang, X., Zhao, C., Chen, H., et al. (2015). Evolution of carbapenem-resistant Acinetobacter baumannii revealed through whole-genome sequencing and comparative genomic analysis. Antimicrob. Agents Chemother. 59, 1168–1176. doi: 10.1128/AAC.04609-14
Lin, M. F., Lin, Y. Y., Yeh, H. W., and Lan, C. Y. (2014). Role of the BaeSR two-component system in the regulation of Acinetobacter baumannii adeAB genes and its correlation with tigecycline susceptibility. BMC Microbiol. 14:119. doi: 10.1186/1471-2180-14-119
Lin, Y. C., Hsia, K. C., Chen, Y. C., Sheng, W. H., Chang, S. C., Liao, M. H., et al. (2010). Genetic basis of multidrug resistance in Acinetobacter clinical isolates in Taiwan. Antimicrob. Agents Chemother. 54, 2078–2084. doi: 10.1128/AAC.01398-09
Liou, M. L., Soo, P. C., Ling, S. R., Kuo, H. Y., Tang, C. Y., and Chang, K. C. (2014). The sensor kinase BfmS mediates virulence in Acinetobacter baumannii. J. Microbiol. Immunol. Infect. 47, 275–281. doi: 10.1016/j.jmii.2012.12.004
Livak, K. J., and Schmittgen, T. D. (2001). Analysis of relative gene expression data using real-time quantitative PCR and the 2(−ΔΔCt) Method. Methods 25, 402–408. doi: 10.1006/meth.2001.1262
Lopes, B. S., and Amyes, S. G. (2012). Role of ISAba1 and ISAba125 in governing the expression of blaADC in clinically relevant Acinetobacter baumannii strains resistant to cephalosporins. J. Med. Microbiol. 61(Pt 8), 1103–1108. doi: 10.1099/jmm.0.044156-0
Luke, N. R., Sauberan, S. L., Russo, T. A., Beanan, J. M., Olson, R., Loehfelm, T. W., et al. (2010). Identification and characterization of a glycosyltransferase involved in Acinetobacter baumannii lipopolysaccharide core biosynthesis. Infect. Immun. 78, 2017–2023. doi: 10.1128/IAI.00016-10
Magnet, S., Courvalin, P., and Lambert, T. (2001). Resistance-nodulation-cell division-type efflux pump involved in aminoglycoside resistance in Acinetobacter baumannii strain BM4454. Antimicrob. Agents Chemother. 45, 3375–3380. doi: 10.1128/AAC.45.12.3375-3380.2001
Maragakis, L. L., and Perl, T. M. (2008). Acinetobacter baumannii: epidemiology, antimicrobial resistance, and treatment options. Clin. Infect. Dis. 46, 1254–1263. doi: 10.1086/529198
Marti, S., Sanchez-Cespedes, J., Oliveira, E., Bellido, D., Giralt, E., and Vila, J. (2006). Proteomic analysis of a fraction enriched in cell envelope proteins of Acinetobacter baumannii. Proteomics 6(Suppl. 1), S82–87. doi: 10.1002/pmic.200500323
McConnell, M. J., Actis, L., and Pachon, J. (2013). Acinetobacter baumannii: human infections, factors contributing to pathogenesis and animal models. FEMS Microbiol. Rev. 37, 130–155. doi: 10.1111/j.1574-6976.2012.00344.x
Metan, G., Sariguzel, F., and Sumerkan, B. (2009). Factors influencing survival in patients with multi-drug-resistant Acinetobacter bacteraemia. Eur. J. Intern. Med. 20, 540–544. doi: 10.1016/j.ejim.2009.05.005
Mussi, M. A., Limansky, A. S., and Viale, A. M. (2005). Acquisition of resistance to carbapenems in multidrug-resistant clinical strains of Acinetobacter baumannii: natural insertional inactivation of a gene encoding a member of a novel family of beta-barrel outer membrane proteins. Antimicrob. Agents Chemother. 49, 1432–1440. doi: 10.1128/AAC.49.4.1432-1440.2005
Naas, T., Bogaerts, P., Bauraing, C., Degheldre, Y., Glupczynski, Y., and Nordmann, P. (2006). Emergence of PER and VEB extended-spectrum beta-lactamases in Acinetobacter baumannii in Belgium. J. Antimicrob. Chemother. 58, 178–182. doi: 10.1093/jac/dkl178
Nordmann, P. (2010). Gram-negative bacteriae with resistance to carbapenems. Med. Sci. 26, 950–959. doi: 10.1051/medsci/20102611950
Nordmann, P., Ronco, E., Naas, T., Duport, C., Michel-Briand, Y., and Labia, R. (1993). Characterization of a novel extended-spectrum beta-lactamase from Pseudomonas aeruginosa. Antimicrob. Agents Chemother. 37, 962–969. doi: 10.1128/AAC.37.5.962
Pagani, L., Mantengoli, E., Migliavacca, R., Nucleo, E., Pollini, S., Spalla, M., et al. (2004). Multifocal detection of multidrug-resistant Pseudomonas aeruginosa producing the PER-1 extended-spectrum beta-lactamase in Northern Italy. J. Clin. Microbiol. 42, 2523–2529. doi: 10.1128/JCM.42.6.2523-2529.2004
Park, S., Lee, K. M., Yoo, Y. S., Yoo, J. S., Yoo, J. I., Kim, H. S., et al. (2011). Alterations of gyrA, gyrB, and parC and activity of efflux pump in fluoroquinolone-resistant Acinetobacter baumannii. Osong. Pub. Health Res. Perspect. 2, 164–170. doi: 10.1016/j.phrp.2011.11.040
Peleg, A. Y., Seifert, H., and Paterson, D. L. (2008). Acinetobacter baumannii: emergence of a successful pathogen. Clin. Microbiol. Rev. 21, 538–582. doi: 10.1128/CMR.00058-07
Perez, F., Hujer, A. M., Hujer, K. M., Decker, B. K., Rather, P. N., and Bonomo, R. A. (2007). Global challenge of multidrug-resistant Acinetobacter baumannii. Antimicrob. Agents Chemother. 51, 3471–3484. doi: 10.1128/AAC.01464-06
Piddock, L. J. (2006). Clinically relevant chromosomally encoded multidrug resistance efflux pumps in bacteria. Clin. Microbiol. Rev. 19, 382–402. doi: 10.1128/CMR.19.2.382-402.2006
Pinto, N. A., D'Souza, R., Hwang, I. S., Choi, J., In, Y. H., Park, H. S., et al. (2017). Whole genome and transcriptome analysis reveal MALDI-TOF MS and SDS-PAGE have limited performance for the detection of the key outer membrane protein in carbapenem-resistant Klebsiella pneumoniae isolates. Oncotarget 8, 84818–84826. doi: 10.18632/oncotarget.19005
Poirel, L., Cabanne, L., Vahaboglu, H., and Nordmann, P. (2005). Genetic environment and expression of the extended-spectrum beta-lactamase blaPER-1 gene in gram-negative bacteria. Antimicrob. Agents Chemother. 49, 1708–1713. doi: 10.1128/AAC.49.5.1708-1713.2005
Poirel, L., Naas, T., and Nordmann, P. (2010). Diversity, epidemiology, and genetics of class D beta-lactamases. Antimicrob. Agents Chemother. 54, 24–38. doi: 10.1128/AAC.01512-08
Poirel, L., and Nordmann, P. (2006). Carbapenem resistance in Acinetobacter baumannii: mechanisms and epidemiology. Clin. Microbiol. Infect. 12, 826–836. doi: 10.1111/j.1469-0691.2006.01456.x
Quale, J., Bratu, S., Landman, D., and Heddurshetti, R. (2003). Molecular epidemiology and mechanisms of carbapenem resistance in Acinetobacter baumannii endemic in New York City. Clin. Infect. Dis. 37, 214–220. doi: 10.1086/375821
Rafei, R., Pailhories, H., Hamze, M., Eveillard, M., Mallat, H., Dabboussi, F., et al. (2015). Molecular epidemiology of Acinetobacter baumannii in different hospitals in Tripoli, Lebanon using bla(OXA-51-like) sequence based typing. BMC Microbiol. 15:103. doi: 10.1186/s12866-015-0441-5
Ranellou, K., Kadlec, K., Poulou, A., Voulgari, E., Vrioni, G., Schwarz, S., et al. (2012). Detection of Pseudomonas aeruginosa isolates of the international clonal complex 11 carrying the blaPER-1 extended-spectrum beta-lactamase gene in Greece. J. Antimicrob. Chemother. 67, 357–361. doi: 10.1093/jac/dkr471
Ridley, A., and Threlfall, E. J. (1998). Molecular epidemiology of antibiotic resistance genes in multiresistant epidemic Salmonella typhimurium DT 104. Microb. Drug Resist. 4, 113–118. doi: 10.1089/mdr.1998.4.113
Rosenfeld, N., Bouchier, C., Courvalin, P., and Perichon, B. (2012). Expression of the resistance-nodulation-cell division pump AdeIJK in Acinetobacter baumannii is regulated by AdeN, a TetR-type regulator. Antimicrob. Agents Chemother. 56, 2504–2510. doi: 10.1128/AAC.06422-11
Rumbo, C., Gato, E., Lopez, M., Ruiz de Alegria, C., Fernandez-Cuenca, F., Martinez-Martinez, L., et al. (2013). Contribution of efflux pumps, porins, and beta-lactamases to multidrug resistance in clinical isolates of Acinetobacter baumannii. Antimicrob. Agents Chemother. 57, 5247–5257. doi: 10.1128/AAC.00730-13
Ruzin, A., Keeney, D., and Bradford, P. A. (2007). AdeABC multidrug efflux pump is associated with decreased susceptibility to tigecycline in Acinetobacter calcoaceticus-Acinetobacter baumannii complex. J. Antimicrob. Chemother. 59, 1001–1004. doi: 10.1093/jac/dkm058
Sievers, F., Wilm, A., Dineen, D., Gibson, T. J., Karplus, K., Li, W., et al. (2011). Fast, scalable generation of high-quality protein multiple sequence alignments using clustal omega. Mol. Syst. Biol. 7:539. doi: 10.1038/msb.2011.75
Singh, H., Thangaraj, P., and Chakrabarti, A. (2013). Acinetobacter baumannii: a brief account of mechanisms of multidrug resistance and current and future therapeutic management. J. Clin. Diagn. Res. 7, 2602–2605. doi: 10.7860/JCDR/2013/6337.3626
Siroy, A., Molle, V., Lemaitre-Guillier, C., Vallenet, D., Pestel-Caron, M., Cozzone, A. J., et al. (2005). Channel formation by CarO, the carbapenem resistance-associated outer membrane protein of Acinetobacter baumannii. Antimicrob. Agents Chemother. 49, 4876–4883. doi: 10.1128/AAC.49.12.4876-4883.2005
Takahashi, H., Watanabe, H., Kuroki, T., Watanabe, Y., and Yamai, S. (2002). Identification of tet(B), encoding high-level tetracycline resistance, in Neisseria meningitidis. Antimicrob. Agents Chemother. 46, 4045–4046. doi: 10.1128/AAC.46.12.4045-4046.2002
Turton, J. F., Woodford, N., Glover, J., Yarde, S., Kaufmann, M. E., and Pitt, T. L. (2006). Identification of Acinetobacter baumannii by detection of the blaOXA-51-like carbapenemase gene intrinsic to this species. J. Clin. Microbiol. 44, 2974–2976. doi: 10.1128/JCM.01021-06
Untergasser, A., Cutcutache, I., Koressaar, T., Ye, J., Faircloth, B. C., Remm, M., et al. (2012). Primer3–new capabilities and interfaces. Nucleic Acids Res. 40:e115. doi: 10.1093/nar/gks596
Vila, J., Marti, S., and Sanchez-Cespedes, J. (2007). Porins, efflux pumps and multidrug resistance in Acinetobacter baumannii. J. Antimicrob. Chemother. 59, 1210–1215. doi: 10.1093/jac/dkl509
Vila, J., and Pachon, J. (2008). Therapeutic options for Acinetobacter baumannii infections. Expert Opin. Pharmacother. 9, 587–599. doi: 10.1517/14656566.9.4.587
Vila, J., Ruiz, J., Goni, P., Marcos, A., and Jimenez de Anta, T. (1995). Mutation in the gyrA gene of quinolone-resistant clinical isolates of Acinetobacter baumannii. Antimicrob. Agents Chemother. 39, 1201–1203. doi: 10.1128/AAC.39.5.1201
Visca, P., Seifert, H., and Towner, K. J. (2011). Acinetobacter infection–an emerging threat to human health. IUBMB Life 63, 1048–1054. doi: 10.1002/iub.534
Wisplinghoff, H., Paulus, T., Lugenheim, M., Stefanik, D., Higgins, P. G., Edmond, M. B., et al. (2012). Nosocomial bloodstream infections due to Acinetobacter baumannii, Acinetobacter pittii and Acinetobacter nosocomialis in the United States. J. Infect. 64, 282–290. doi: 10.1016/j.jinf.2011.12.008
Wisplinghoff, H., and Seifert, H. (2014). Epidemiology and clinical features of Acinetobacter baumannii infections in humans. Berl. Munch. Tierarztl. Wochenschr. 127, 447–457.
Yong, D., Shin, J. H., Kim, S., Lim, Y., Yum, J. H., Lee, K., et al. (2003). High prevalence of PER-1 extended-spectrum beta-lactamase-producing Acinetobacter spp. in Korea. Antimicrob. Agents Chemother. 47, 1749–1751. doi: 10.1128/AAC.47.5.1749-1751.2003
Yoon, E. J., Courvalin, P., and Grillot-Courvalin, C. (2013). RND-type efflux pumps in multidrug-resistant clinical isolates of Acinetobacter baumannii: major role for AdeABC overexpression and AdeRS mutations. Antimicrob. Agents Chemother. 57, 2989–2995. doi: 10.1128/AAC.02556-12
Zander, E., Chmielarczyk, A., Heczko, P., Seifert, H., and Higgins, P. G. (2013). Conversion of OXA-66 into OXA-82 in clinical Acinetobacter baumannii isolates and association with altered carbapenem susceptibility. J. Antimicrob. Chemother. 68, 308–311. doi: 10.1093/jac/dks382
Zankari, E., Hasman, H., Cosentino, S., Vestergaard, M., Rasmussen, S., Lund, O., et al. (2012). Identification of acquired antimicrobial resistance genes. J. Antimicrob. Chemother. 67, 2640–2644. doi: 10.1093/jac/dks261
Keywords: Acinetobacter, panel strains, antimicrobial resistance, whole-genome sequencing, phenotypic characterization
Citation: D'Souza R, Pinto NA, Phuong NL, Higgins PG, Vu TN, Byun J-H, Cho YL, Choi JR and Yong D (2019) Phenotypic and Genotypic Characterization of Acinetobacter spp. Panel Strains: A Cornerstone to Facilitate Antimicrobial Development. Front. Microbiol. 10:559. doi: 10.3389/fmicb.2019.00559
Received: 10 December 2018; Accepted: 05 March 2019;
Published: 26 March 2019.
Edited by:
Maria Soledad Ramirez, California State University, Fullerton, United StatesReviewed by:
Mariana Andrea Papalia, Universidad de Buenos Aires, ArgentinaTimothy Meredith, Pennsylvania State University, United States
Copyright © 2019 D'Souza, Pinto, Phuong, Higgins, Vu, Byun, Cho, Choi and Yong. This is an open-access article distributed under the terms of the Creative Commons Attribution License (CC BY). The use, distribution or reproduction in other forums is permitted, provided the original author(s) and the copyright owner(s) are credited and that the original publication in this journal is cited, in accordance with accepted academic practice. No use, distribution or reproduction is permitted which does not comply with these terms.
*Correspondence: Dongeun Yong, ZGV5b25nQHl1aHMuYWM=