- 1Department of Microbiology, School of Medicine, Chungnam National University, Daejeon, South Korea
- 2Infection Control Convergence Research Center, School of Medicine, Chungnam National University, Daejeon, South Korea
- 3Department of Medical Science, School of Medicine, Chungnam National University, Daejeon, South Korea
- 4Department of Infection Biology, School of Medicine, Chungnam National University, Daejeon, South Korea
AMP-activated protein kinase (AMPK), a key metabolic regulator, plays an essential role in the maintenance of energy balance in response to stress. Tuberculosis (TB), primarily caused by the pathogen Mycobacterium tuberculosis (Mtb), remains one of the most important infectious diseases worldwide, characterized by both high incidence and mortality. Development of new preventive and therapeutic strategies against TB requires a profound understanding of the various host-pathogen interactions that occur during infection. Emerging data suggest that AMPK plays an essential regulatory role in host autophagy, mitochondrial biogenesis, metabolic reprogramming, fatty acid β-oxidation, and the control of pathologic inflammation in macrophages during Mtb infection. As described in this review, recent studies have begun to define the functional properties of AMPK modulators capable of restricting intracellular bacteria and promoting host defenses. Several host defense factors in the context of AMPK activation also participate in autophagic and non-autophagic pathways in a coordinated manner to enhance antimicrobial responses against Mtb infection. A better understanding of these AMPK-targeted effector networks offers significant potential for the development of novel therapeutics for human TB and other infectious diseases.
Introduction
AMP-activated protein kinase (AMPK) is an essential metabolic sensor that responds to increases in the cellular adenosine monophosphate (AMP) to adenosine triphosphate (ATP) ratio (AMP:ATP) by activating catabolic pathways for energy production. In addition to metabolic stresses, the AMPK pathway is also activated in response to various stress-related signals including infection and inflammation. A wide range of infectious diseases have been shown to trigger AMPK signaling cascades, resulting in modulation of host responses that can either enhance pathogen survival or promote host defenses in a context-dependent manner (Grahame Hardie, 2016; Moreira et al., 2016; Prantner et al., 2017; Lee et al., 2018). Recent studies have revealed a diverse array of functions associated with the AMPK pathway, including regulation of host signaling, and participation in important defensive functions such as autophagy, mitochondrial biogenesis, metabolic reprogramming, and regulation of inflammation during infection (Prantner et al., 2017; Silwal et al., 2018). Each of these discoveries offers the potential of new therapeutic strategies capable of harnessing the AMPK pathway to combat diverse infectious diseases including human tuberculosis (TB).
Mycobacterium tuberculosis (Mtb) is the major causal pathogen of TB, which remains a global health problem, with a high prevalence of both multidrug-resistant and extensively drug-resistant TB (WHO, 2017). With approximately one third of the world’s population thought to be latently infected with Mtb, there remains an urgent need for new therapeutic developmental modalities. These advances, however, remain limited by an incomplete understanding of the host-pathogen interaction due in part to the complicated lifestyle of Mtb within host cells (Hmama et al., 2015; Dorhoi and Kaufmann, 2016). Mtb has a unique waxy coating on its cell wall comprised primarily of mycolic acids, a unique adaptation which enables survival within host cells (Daffe et al., 2014). In addition to its cell wall, Mtb has evolved multiple strategies to evade both innate and adaptive immune defenses, enabling both persistent infection and even active replication within the human host (Hmama et al., 2015), though the exact mechanisms underlying this survival remain poorly understood.
Upon Mtb infection, a variety of mycobacterial components including protein antigens and lipids trigger a series of innate inflammatory responses in host macrophages, though these pathogens can often resist these responses and escape from immune clearance (Dorhoi and Kaufmann, 2016). Despite this, excessive inflammatory responses by the host can often lead to unwanted pathological damage during infection (Cooper, 2009). Since Mtb can persist within the highly lipophilic replicative niche of macrophages for most of its life cycle, an intricate interconnection between bacterial and host cellular metabolism will ultimately determine the overall picture of host-pathogen interaction (Hmama et al., 2015). Autophagy, as a cell-autonomous quality control system, is a crucial process for maintaining homeostasis of the immune, inflammatory, and metabolic responses in host cells during infection (Deretic et al., 2015; Paik et al., 2018). Given the clear need for overcoming drug-resistant issues, many efforts are being made to develop host-targeted therapies to combat TB and other infections.
In this review, we summarize the current literature suggesting a role for AMPK as a central mediator regulating a diverse set of biological responses including autophagic, lysosomal, and metabolic pathways in the Mtb-infected host. In addition, we analyze the regulatory mechanisms underlying the beneficial antimicrobial effects mediated by AMPK signaling during Mtb infection. Finally, we discuss the advances and technical challenges surrounding the use of AMPK-targeting small molecules as novel therapeutic strategies for the treatment of TB.
Overview of AMPK
AMP-activated protein kinase is a member of the serine/threonine (Ser/Thr) kinase family and is ubiquitously expressed in eukaryotic cells. AMPK monitors and senses the AMP/ADP relative to ATP to maintain an adequate energy supply by promoting catabolic pathways and/or decreasing anabolic pathways in response to stress conditions (Moreira et al., 2016). Maintaining proper ATP concentrations within cells is critical for cell survival, as dysregulation of energy homeostasis can lead to a wide range of pathologies including metabolic diseases, cardiovascular diseases, and cancer (Hardie, 2011a,b; Carling, 2017).
AMP-activated protein kinase exists as a heterotrimeric complex composed of a catalytic α subunit and two regulatory β and γ subunits (Hardie, 2011b; Hardie et al., 2016; Moreira et al., 2016). Furthermore, there are several isoforms for each subunit of AMPK (two for α and β subunits; three for γ subunits), which combine to form different AMPK complexes. As the catalytic subunit, the α subunit of AMPK complex is a main functional component and essential for AMPK activation through its phosphorylation of Thr172, whereas the γ subunit functions as a sensor of ADP levels and interacts with ADP (Novikova et al., 2015; Hardie et al., 2016; Moreira et al., 2016).
AMP-activated protein kinase activation is mediated by several upstream signaling pathways, including the liver kinase B 1 (LKB1) tumor suppressor, as well as Ca2+/calmodulin-dependent kinase II (CaMKKII)-mediated phosphorylation of AMPK (Green et al., 2011; Marcelo et al., 2016). In addition, TGF-β-activated kinase-1 (TAK1) acts as an upstream kinase for AMPK (Xie et al., 2006; Inokuchi-Shimizu et al., 2014; Neumann, 2018; Silwal et al., 2018). Several lines of evidence showed a reciprocal regulation between AMPK and mTOR signaling pathways. AMPK phosphorylation leads to the inhibition of mammalian target of rapamycin (mTOR) through phosphorylation of tuberous sclerosis complex 2 (TSC2), which in turn affects both cell metabolism and growth (Corradetti et al., 2004; Inoki et al., 2012). Another mechanism by which AMPK inhibits mTOR activity is mediated through phosphorylation of RAPTOR (regulatory-associated protein of mTOR) (Gwinn et al., 2008). On the other hands, mTOR signaling suppresses the activation of AMPK through p70 S6 kinase 1 (S6K1)-mediated inhibition of TAK1 (Xu et al., 2017; Sun et al., 2018). Interestingly, AMPK and its upstream kinase LKB1, as well as mammalian target of rapamycin complex 1 (mTORC1), are primarily located at the surface of the lysosome where they coordinate various homeostatic signaling pathways such as autophagy (Korolchuk et al., 2011; Carroll and Dunlop, 2017). The elaborate mechanisms that delineate the reciprocal regulation between AMPK and mTOR pathways are shown in Figure 1.
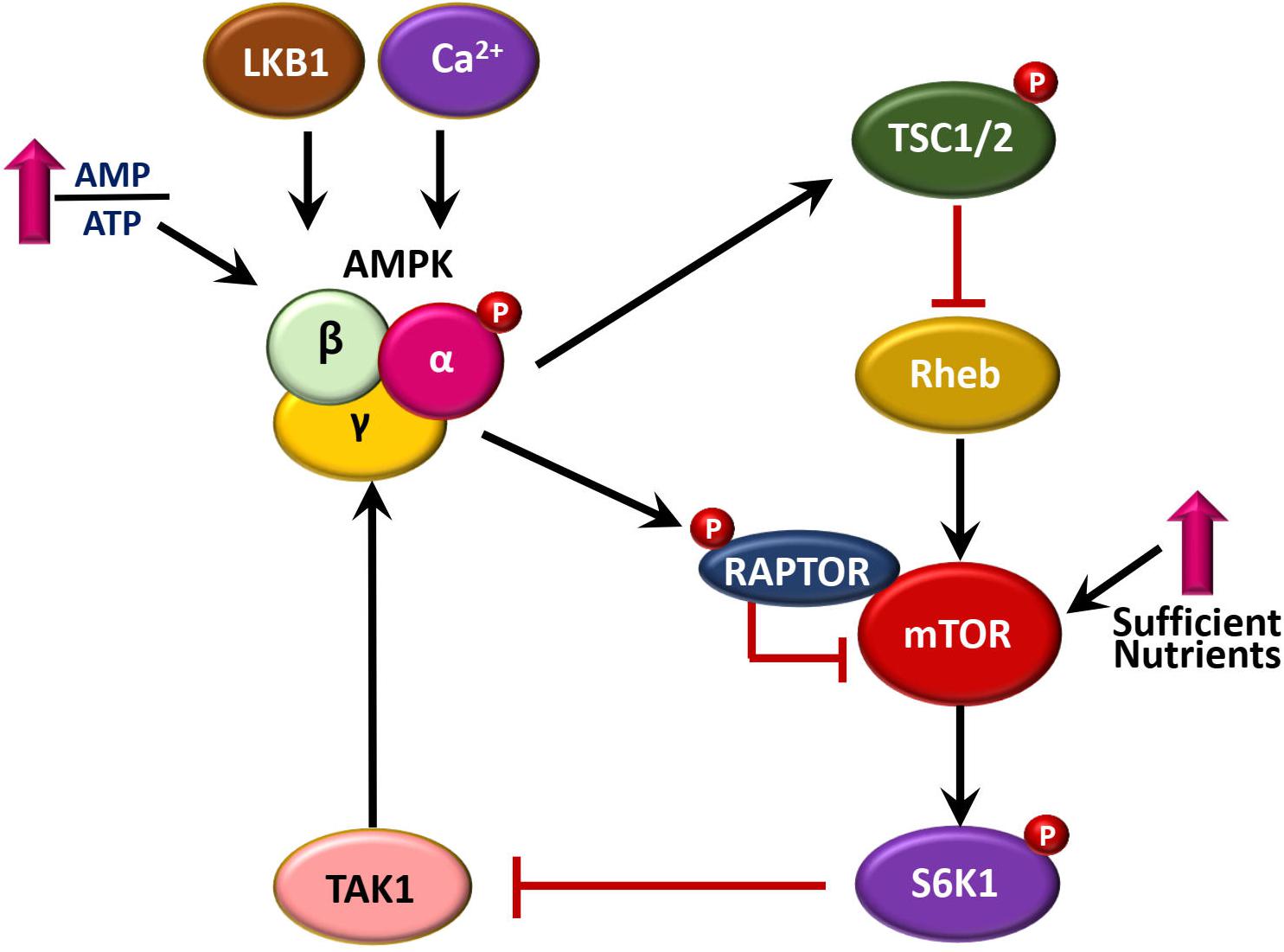
Figure 1. A brief summary of reciprocal regulation between AMPK and mTOR pathways. AMPK is activated by upstream signaling pathways, including LKB1, CaMKKII, and TAK1. AMPK pathway inhibits mTOR signaling, through at least two mechanisms, i.e., phosphorylation of TSC2 via Rheb, and direct phosphorylation of RAPTOR. In addition, mTOR-S6K1 signaling pathway inhibits AMPK activation through inactivation of TAK1.
Upon activation, AMPK functions as a signaling hub regulating a vast array of proteins, enzymes, metabolic pathways, and other signaling pathways (Hardie and Alessi, 2013; Moreira et al., 2016). Through its crosstalk with various signaling mediators, AMPK serves as a key modulator under diverse physiologic conditions, including cell growth, proliferation, autophagy, mitochondrial biogenesis, stress responses, and immune regulation (Hardie, 2011b; Shah et al., 2017). In addition, AMPK activation is critically required for controlling mitochondrial biogenesis and metabolic function to promote cell survival and keep homeostasis (Hardie, 2011b; Zhang et al., 2017). Under pathological condition, dysregulation of AMPK is associated with pathogenesis of various human diseases including inflammatory, metabolic, and neurodegenerative diseases (Ruderman et al., 2013; Peixoto et al., 2017; Szrejder and Piwkowska, 2019). Indeed, numerous pathogens can modulate AMPK activity to enhance or ameliorate host protective immune responses during infection (Moreira et al., 2016; Silwal et al., 2018). Moreover, AMPK activity is closely associated with immunometabolic regulation during a wide range of infections (Mahon and Hafner, 2015; Moreira et al., 2016). In addition to the roles described above, emerging evidence suggests that AMPK is involved in the activation of antimicrobial immunity and immunopathologic responses during mycobacterial infection through its interaction with other signaling pathways (Yang et al., 2014; Ong et al., 2015). These results, combined with other results which will be discussed in the later section of AMPK-targeting small molecules in this review, have shed the light on the development of potential therapeutics for host-directed therapy against TB and other infectious diseases. However, future studies are warranted to investigate the contribution of AMPK-targeted therapy in vivo and to delineate the mechanisms by which AMPK regulates innate immune responses during different stages of Mtb infection.
AMPK: Autophagy-Inducing Kinase in Mycobacterial Infection
Autophagy is an intracellular pathway that is crucial for maintenance of homeostasis by triggering the degradation of long-lived proteins and unnecessary intracellular components in response to a variety of stresses (Mizushima et al., 2008; Choi et al., 2013). Numerous studies have demonstrated a role for autophagy in a variety of biological responses including the regulation of innate and adaptive immunity, and antimicrobial responses (Levine and Deretic, 2007; Mizushima et al., 2008). A full overview of the roles and mechanisms by which autophagy activates antimicrobial effector pathways and controls inflammation during mycobacterial infection has been reviewed elsewhere (Deretic, 2008, 2011; Deretic et al., 2009; Ni Cheallaigh et al., 2011; Liu et al., 2017; Paik et al., 2018). As an autophagy-activating kinase, AMPK is involved in the regulation of innate host defense against intracellular pathogens, including Mtb (Yang et al., 2014; Singh and Subbian, 2018). Here, we briefly discuss autophagy and mycobacterial infection, and the role of AMPK activation in the antimicrobial response to Mtb infection.
AMPK Cross-Talk With Autophagy in Mycobacterial Infection
Initiation of macroautophagy is triggered by the UNC-51-like kinase 1/2 complex [ULK1 and ULK2, the mammalian orthologs of autophagy-related 1 (Atg1)] (Hara et al., 2008; Mercer et al., 2009) and serves as an important downstream effector. The resulting effector molecules are in turn regulated by the coordinated function of AMPK and mTORC1 (Figure 2) (Kim et al., 2011). Activated AMPK induces the phosphorylation of serine residues S317, S555, and S777 of ULK1, a mammalian autophagy-initiating kinase, which plays an important role in starvation-induced autophagy (Bach et al., 2011; Kim et al., 2011; Wong et al., 2013). Indeed, ULK1 has several phosphorylation sites relevant to this process, including mTORC1-mediated phosphorylation of ULK1, which disrupts the association between ULK1 and AMPK (Kim et al., 2011). Previous studies showed that Mtb infection of macrophages by the mTOR/70 kDa ribosomal S6 kinase 1 (S6K1) pathway serves as an inhibitor of host autophagy, as well as a driver of inflammatory responses (Yang et al., 2006, 2014). By contrast, induction of AMPK activation by AICAR resulted in phosphorylation of ULK1 at Ser317 and Ser777, thereby promoting host cell autophagy and antimicrobial responses in both in vitro macrophage assays and in vivo (Yang et al., 2014). These data suggest that Mtb infection drives activation of mTOR signaling, with the presumed benefits of nutrient support and immunologic shelter via lipid body formation in host macrophages. However, AMPK activation by autophagy-inducing agents may counteract the pathogen strategy and redirect intracellular defenses toward catabolism in the form of increased lysosomal activation. Given the well-established function of AMPK in autophagy activation and negative regulation of mTOR pathways, AMPK might represent a promising target of host-directed therapy against TB (Yao et al., 2016; Singh and Subbian, 2018).
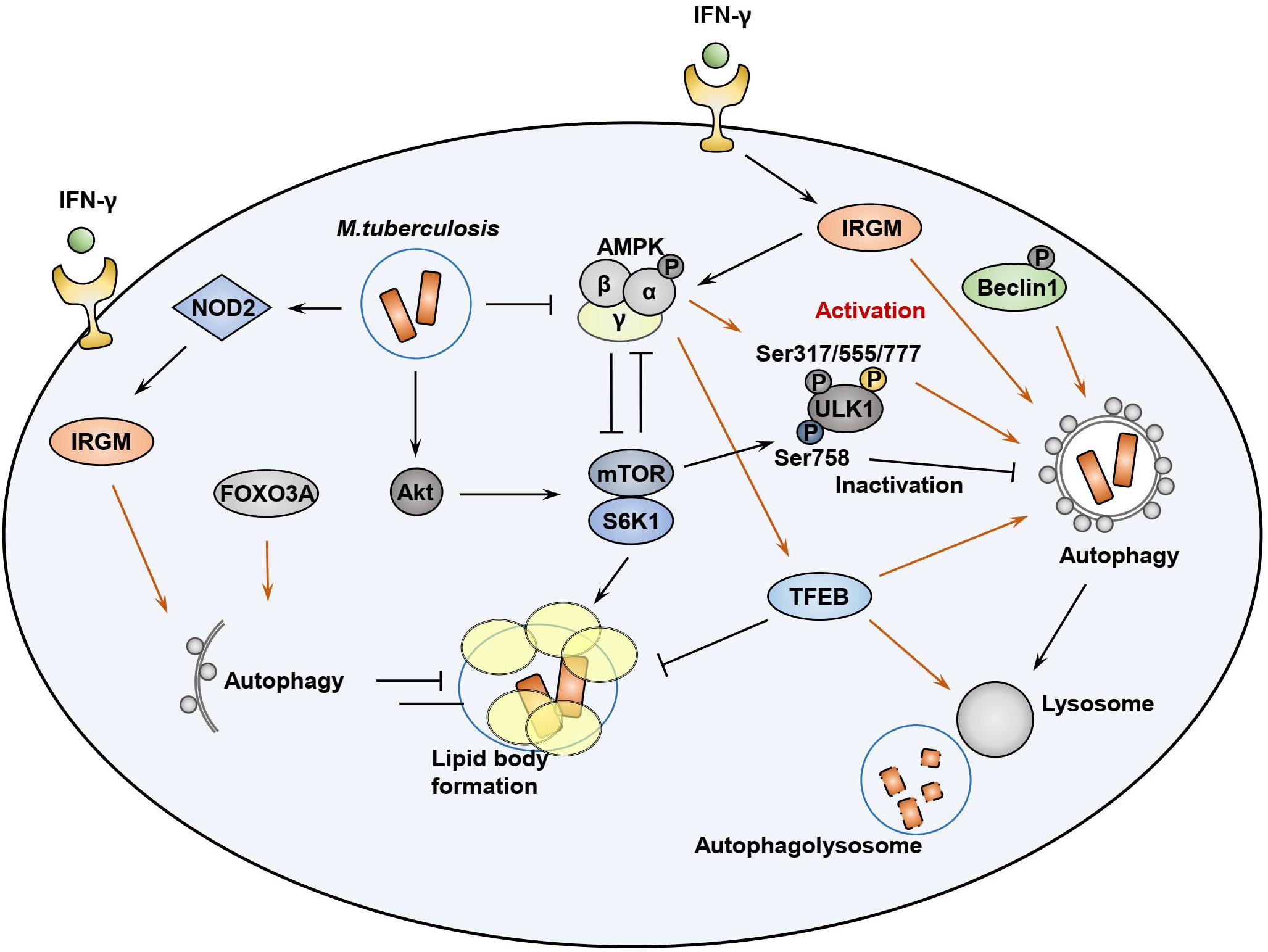
Figure 2. AMPK-mTOR signaling pathways during Mtb infection. During infection, Mtb can activate mTOR pathway to enhance lipid body formation and ULK1 inhibition. AMPK activation, which is induced by AMP/ATP ratio, LKB1, intracellular calcium influx, as well as IRGM, is required for autophagy activation through ULK1 phosphorylation at Ser317/555/777. Either adaptive (Th1 cytokine IFN-γ) or innate (NOD2) signaling can induce IRGM activation, which is required for autophagy activation. AMPK-mediated Beclin-1 phosphorylation also activates autophagy to enhance phagosomal maturation. AMPK and mTOR pathways reciprocally inhibit each other to regulate autophagy, metabolism, and inflammatory responses in host cells during Mtb infection. AMPK-mediated TFEB activation leads to lysosomal activation and fatty acid β-oxidation to suppress lipid body formation. Mtb-mediated Akt phosphorylation can inhibit Foxo3A activation, which is also required for autophagy activation in the host cells.
The IFN-γ-inducible GTPase IRGM is an autophagy protein associated with Crohn’s disease and TB (Bekpen et al., 2010). Activation of this protein is essential for phagosomal maturation and elimination of Mtb in murine and human macrophages (MacMicking et al., 2003; Gutierrez et al., 2004; Singh et al., 2006; Singh et al., 2010). Nevertheless, how the IRGM GTPase exerts its innate effector function during intracellular infection remains poorly understood. One pathway that has been implicated in innate immunity involves IRGM-mediated stabilization of intracellular AMPK (total and activated forms of AMPK), which in turn helps preserve levels of ULK1, ATG14, and ATG16L1, thereby enabling formation of the autophagic machinery (Chauhan et al., 2015). In addition, AMPK promotes autophagy via Beclin-1 phosphorylation at Thr388, which is required for Beclin-1 interaction with phosphatidylinositol 3-kinase catalytic subunit type 3 (PIK3C3) and autophagy-related 14 (ATG14) (Zhang et al., 2016). Given the previous findings of NOD2-mediated IRGM ubiquitination and activation (Chauhan et al., 2015), this IRGM-AMPK signaling pathway may serve as a bridge connecting innate immune signaling with functional activation of the autophagic machinery in response to mycobacterial infection.
AMPK and Lysosomal Function in Mycobacterial Infection
Transcription factor (TF) EB, a master gene for lysosomal biogenesis, is an essential upstream regulator of autophagy, serving as a transcriptional activator of numerous autophagy and lysosomal related genes (Settembre et al., 2011). Under basal conditions, TFEB is suppressed by the action of cathepsin B-mediated cleavage of the calcium channel MCOLN1 in lysosomes, thus controlling the number of autophagosomes and lysosomes (Man and Kanneganti, 2016). TFEB phosphorylation by the mTORC1 kinase retains TFEB in its inactivated state in the cytosol (Martina et al., 2012; Roczniak-Ferguson et al., 2012). However, recruitment of AMPK leads to the suppression of mTORC1 activity, thereby activating TFEB (Figure 2), which is then transported into the nucleus where it activates transcription of the autophagic and lysosomal machinery and can even activate ULK1 in cases of Francisella novicida infection (Qi et al., 2016). In addition, induction of phagocytosis via the Fc receptor can itself increase the expression of lysosomal proteins through nuclear translocation and activation of TFEB, serving as an essential step for lysosomal degradation of phagocytosed bacteria (Gray et al., 2016). A recent study showed that TRIM16, an interacting protein of galectin-3, played a protective role in controlling Mtb infection through cooperation with core autophagic machinery in autophagic responses, and simultaneously affected TFEB activation (Chauhan et al., 2016).
Previous studies showed a stronger activation of mTOR kinase phosphorylation, relative to AMPK, in macrophages following Mtb infection in a time-dependent manner (Yang et al., 2014). Data indicating that cytosolic Mtb co-localizes with the components of autophagic machinery, i.e., p62 and LC3, was evident in only 30% of total Mtb phagosomes (Watson et al., 2012), providing evidence for the idea that the majority of intracellular Mtb might stop the activation of xenophagy due to a downregulation of TFEB nuclear translocation by mTOR activation during infection. In addition, Mtb can induce diverse innate immune signaling responses which converge on the NF-κB pathway and manipulate the host protective immune responses (Schorey and Schlesinger, 2016; Stutz et al., 2018). Mtb infection enhances the levels of microRNA (miRNA)-33 and miR-33∗ via the NF-κB pathway to inhibit autophagy, lysosomal function, and fatty acid β-oxidation. In this setting, AMPK activation appears to be crucial for the induction of TFEB, which promoted lipid catabolism as well as xenophagy against Mtb (Ouimet et al., 2016).
As the role of AMPK as a key modulator of TFEB-mediated lysosomal activation becomes clearer, the mechanisms by which AMPK activates autolysosomal function in the context of host defense against mycobacterial infection have garnered greater attention. Our recent study highlighted the importance of TFEB activation in antimicrobial responses along with the dampening pathologic inflammation during Mtb infection (Kim Y.S. et al., 2017). Importantly, the activation of peroxisome proliferator-activated receptor (PPAR)-α, a nuclear receptor that interacts with AMPK during metabolism (Grabacka et al., 2016), was found to enhance lysosomal biogenesis and function via TFEB, as well as protective antimicrobial responses against Mtb (Kim Y.S. et al., 2017).
A recent study showed that lysosomal damage inhibits mTOR kinase activity by galectin-8 interaction with the mTOR apparatus (Jia et al., 2018). However, galectin-9 activates AMPK signaling in response to lysosomal injury, and the galectin-based signal-transduction system plays a critical role in the activation of autophagy and host defense against Mtb (Jia et al., 2018). Further investigations in the mechanisms by which the host integrates galectin-based signaling networks during persistent infection with Mtb will be important for the development of anti-mycobacterial therapies capable of providing protective immunity against infection.
The autophagosomal proteins soluble N-ethylmaleimide-sensitive factor attachment protein receptor (SNARE) and ATG14 are important in autophagosome–lysosome fusion (Itakura et al., 2012; Diao et al., 2015). Earlier studies showed that mannose-capped lipoarabinomannan of Mtb was an inhibitor of phagosomal acidification through a SNARE alteration, thus contributing to virulence during Mtb infection (Fratti et al., 2003). The autophagy protein Irgm1 has been shown to exert antimicrobial effects against mycobacterial infection through the recruitment and subsequent accumulation of PtdIns(3,4)P2 and PtdIns(3,4,5)P3 to phagosomal membranes. These proteins modify the ability of Irgm1 to enhance immunity to Mtb phagosomes via its interaction with SNARE effectors (Tiwari et al., 2009). Despite these observations, few studies have sought to examine the role of AMPK in the modulation of SNARE as part of the autophagic flux observed during mycobacterial infection. A future challenge will be to determine the distinct role of AMPK on the SNARE system and how it integrates complex signaling networks into lysosomal homeostasis during mycobacterial infection.
Roles of AMPK in Immunometabolism During Mycobacterial Infection
In response to inflammation, innate immune cells undergo an immunometabolic shift from enhanced aerobic glycolysis in early phases to high oxidative metabolism in later stages, which is often the result of AMPK activation (O’Neill and Hardie, 2013; Kelly and O’Neill, 2015). In addition to autophagy regulation, AMPK plays a crucial regulator of mitochondrial function and metabolism, through sensing the intracellular energy levels in innate immune cells (Williams and O’Neill, 2018). Here we briefly discuss the immunometabolism and its control by AMPK signaling pathways in innate immune cells in terms of mycobacterial infection.
Overview of Immunometabolism in Innate Immune Cells
Recent studies have highlighted on the metabolic reprogramming in various immune cells. In particular, macrophages and dendritic cells (DCs) are principal innate immune cells that participate in the initiation and resolution of inflammatory responses during infection (O’Neill and Hardie, 2013; Kelly and O’Neill, 2015; Williams and O’Neill, 2018). In M1 macrophages and DCs, the source of energy is mainly provided by upregulation of aerobic glycolysis, although less efficient in the ATP production. Utilizing glycolysis, M1 macrophages and DCs can respond to more rapidly than oxidative phosphorylation (OXPHOS), to meet the energy request for the generation of inflammatory responses and biosynthetic precursor molecules in the initial phase of innate immune responses (Galvan-Pena and O’Neill, 2014; Williams and O’Neill, 2018). M1 macrophages show the metabolic characteristics of broken Krebs cycle and decreased fatty acid oxidation (FAO) and OXPHOS (Galvan-Pena and O’Neill, 2014; Kelly and O’Neill, 2015; O’Neill and Pearce, 2016; Williams and O’Neill, 2018). In this process, the increase of certain metabolites such as succinate and citrate, byproducts of fragmented Krebs cycle, act as signaling molecules that modulate inflammatory pathways in M1 macrophages (Galvan-Pena and O’Neill, 2014; Kelly and O’Neill, 2015; O’Neill and Pearce, 2016; Williams and O’Neill, 2018). Recent studies also revealed that specific metabolic pathways and metabolites such as itaconate are crucial for anti-mycobacterial effects (Michelucci et al., 2013; Williams and O’Neill, 2018).
In DCs, TLR stimulation facilitates a metabolic shift to aerobic glycolysis that is linked to DC maturation and functional activation (Krawczyk et al., 2010; Cortese et al., 2014; Everts et al., 2014; O’Neill and Pearce, 2016). Importantly, the metabolic shift in DCs leads to the upregulation of fatty acid synthesis through pathways of recharging NADPH and utilization of citrate and isocitrate to efficiently accommodate cellular demand for protein synthesis during inflammation (Krawczyk et al., 2010; Cortese et al., 2014; Everts et al., 2014). Glycolysis is strongly upregulated in another inflammatory cells, Th17 cells, and functioned in the metabolic checkpoint regulation in T cell lineage differentiation (Shi et al., 2011; O’Neill et al., 2016). In murine M2 macrophages the Krebs cycle and OXPHOS are intact and functioning, and generate metabolic intermediates for protein glycosylation (Galvan-Pena and O’Neill, 2014; Jha et al., 2015; O’Neill and Pearce, 2016; Williams and O’Neill, 2018). It is now being recognized that immunoregulatory cells including M2 macrophages and regulatory T cells have a reliance on OXPHOS-dependent metabolism for their function (Williams and O’Neill, 2018). Taken together, Figure 3 summarizes a brief overview of immunometabolism in M1 and M2 macrophages, and DCs.
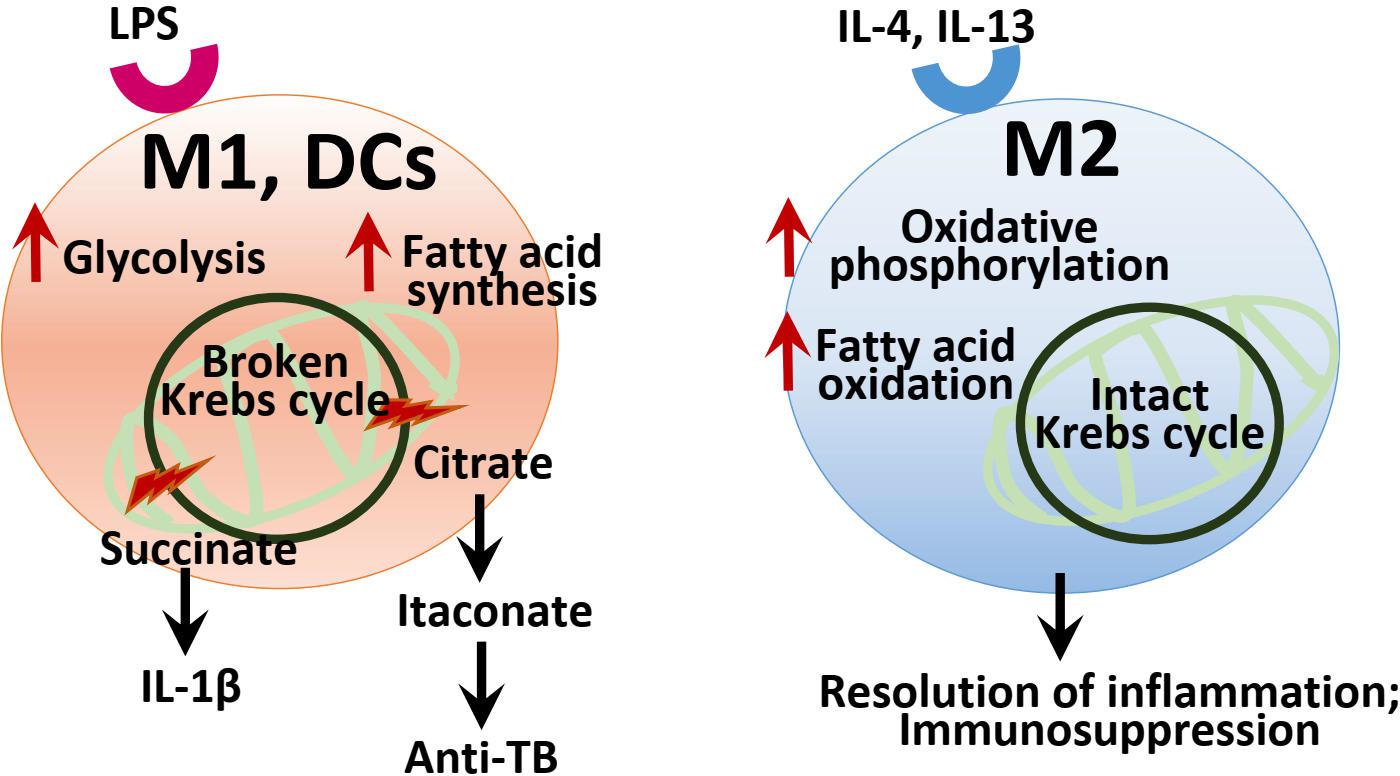
Figure 3. Summary of the immunometabolism in macrophages and DCs. In M1 and DCs, LPS stimulation leads to the upregulation of aerobic glycolysis, and altered TCA cycle with aberrant increase of several metabolic intermediates including succinate, citrate, and itaconate, which act as signaling and effector molecules in inflammatory responses and infection. In DCs, metabolic reprogramming results in the increased fatty acid synthesis through recharging NADPH and utilization of citrate. In M2 macrophages, the Krebs cycle and OXPHOS are intact to drive immunosuppression and the resolution of inflammation.
In innate immune cells, the pro-inflammatory signals inhibit AMPK activation in the initial stage of inflammation and negatively regulates the NF-κB signaling (Sag et al., 2008). Since AMPK senses the energy status in the cells undergoing inflammatory responses, its activation drives catabolic pathways such as FAO and OXPHOS, and simultaneously inhibits mTOR signaling and inflammatory pathways (O’Neill and Hardie, 2013; Kelly and O’Neill, 2015; Almeida et al., 2016; Williams and O’Neill, 2018). AMPK signaling, balanced with mTOR, appears to be a key immunometabolic regulator capable of influencing immune cell differentiation and is reinforced by autophagic function (Almeida et al., 2016; Riffelmacher et al., 2018). This review will focus on the role of AMPK in innate immune cell function during Mtb infection.
Warburg Effect Mediated by HIF-1α During Mycobacterial Infection
Upon Mtb infection, the host immune responses are often confronted with the Warburg effect, a process through which immune cells efficiently meet cellular biosynthetic capacity to activate inflammatory responses and antimicrobial molecules (Shi et al., 2016; Riffelmacher et al., 2018). In this process, Mtb pathogenicity may be attributable to its perturbation of the Warburg effect, enabling escape from M1 macrophage polarization and Th1 protective immunity, to replicate and persist inside host cells (Figure 4) (Shi et al., 2016).
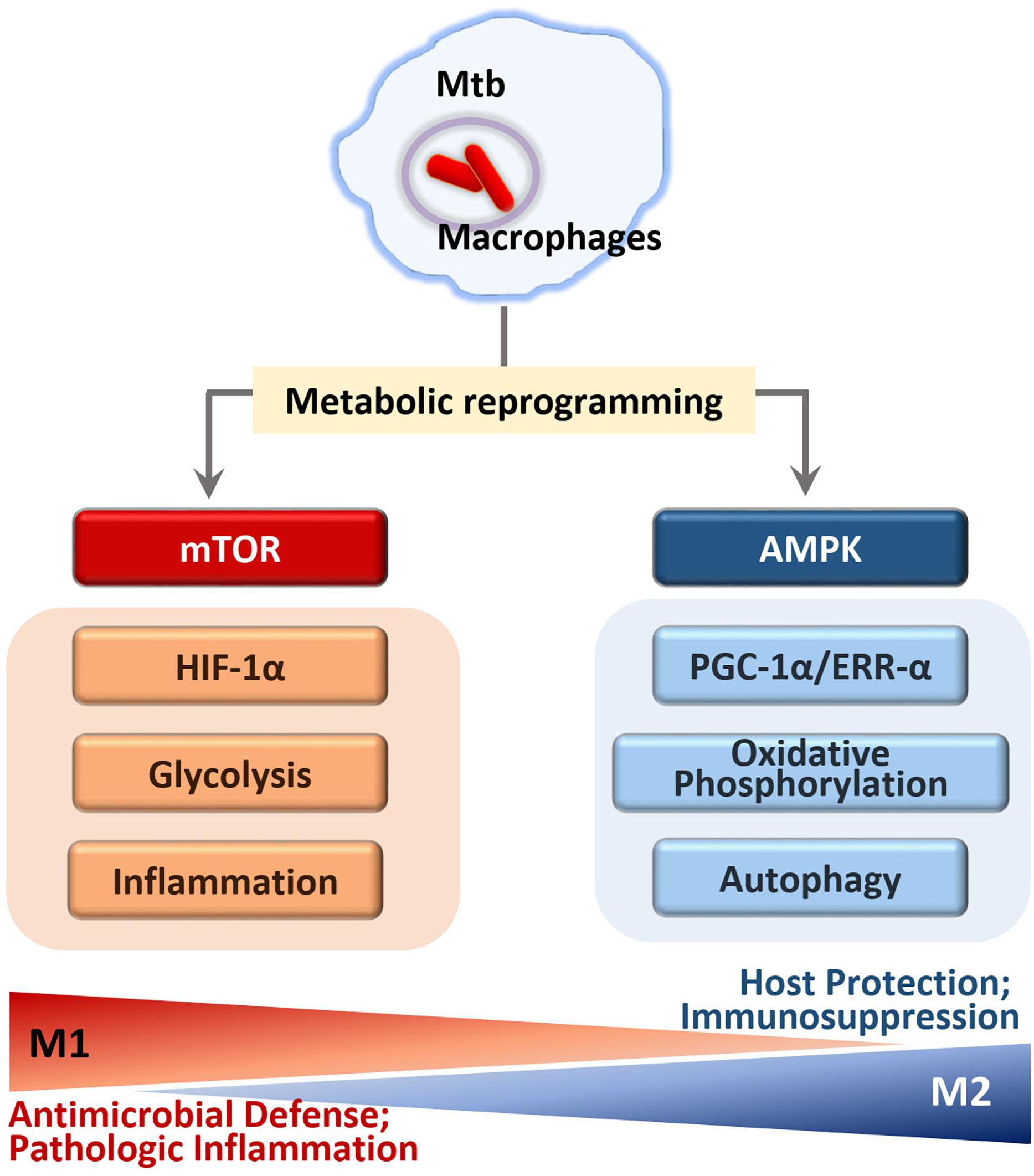
Figure 4. Regulation of immunometabolism in macrophages infected with Mtb. In early phase of Mtb infection, mTOR kinase activation leads to the immunometabolic shift to M1 macrophages that generate pro-inflammatory cytokine by activation of NF-κB and HIF1α signaling. M1-like macrophages increase glycolysis and inflammation through HIF1α and NF-κB signaling pathways. In later phase of infection, M2-like macrophages increase mitochondrial oxidative phosphorylation through AMPK activation. AMPK pathway enhances PGC1α and ERRα activity that is required for autophagy activation through transcriptional and translational regulation. Although the mTOR-HIF1α pathway is essential for initial control of Mtb growth, excessive induction of inflammation seems to be harmful to the host. Similarly, the prolonged activation of AMPK signaling to drive M2-like macrophages may result in the immunosuppression that is detrimental to eradicate intracellular mycobacteria.
Mtb infection of macrophages or peripheral blood mononuclear cells induces strong activation of the mTOR/S6K1 and Akt signaling pathways to modulate host autophagy and metabolic processes (Yang et al., 2006, 2014; Lachmandas et al., 2016). The signaling pathways involving mTOR and Akt play a crucial role in the glycolytic shift in macrophages during infection (Matta and Kumar, 2016). As part of the bioenergetics shift to the Warburg effect, hypoxia-inducible factor (HIF)-1α, a downstream target of mTORC1 (Riffelmacher et al., 2018), promotes transcription of inflammatory genes as well as transcripts involved in aerobic glycolysis and de novo lipid synthesis in immune cells (Tannahill et al., 2013; Kelly and O’Neill, 2015; Riffelmacher et al., 2018). A recent study using an experimental TB model suggested a dual role of HIF-1α; i.e., a protective role during early stages of the disease, but detrimental in later phage of TB (Baay-Guzman et al., 2018). HIF-1α-mediated Warburg effect likely contributes to protective immune responses at the site of infection early in disease but may lead to injurious necrosis and pathology during a prolonged infection (Figure 4).
AMPK-Mediated M1/M2 Regulation and Iron Homeostasis During Mtb Infection
Given the crucial function of AMPK in metabolic regulation, AMPK is often regarded as a master regulator of immunometabolism in immune cells, through orchestrating mitochondrial biogenesis, OXPHOS, and M2 macrophage differentiation (Figure 4) (O’Neill and Pearce, 2016; Griffiths et al., 2017). In M2 macrophages, the generation of proinflammatory cytokines decreased in response to Mtb infection, whereas the anti-inflammatory cytokines and growth factors are unaffected, maintaining normal function and tissue repair (Griffiths et al., 2017). Previous studies have largely found that M2 macrophages exhibit broad anti-inflammatory responses during Mtb infection, enabling a more permissive environment for intracellular mycobacteria growth (Sahu et al., 2017; Lugo-Villarino et al., 2018). By contrast, this type of immune response may also be advantageous to the host, protecting against potentially harmful immunopathology including extensive tissue damage (Pineros et al., 2017). Thus, AMPK may play as a checkpoint regulator of immunometabolism to tilt the balance to favor the increased host defense during Mtb infection.
A recent study has shown that ferritin heavy chain, a major factor regulating the preservation of iron in the host, is a critical factor in protective immunity, serving to ameliorate potentially pathologic inflammation caused by excessive mitochondrial activity and oxidative phosphorylation in host cells (Reddy et al., 2018). Given previous findings that AMPK is an important upstream regulator of ferritin heavy chain transcription (Iwasaki et al., 2013), further research into the role of AMPK in iron homeostasis and its effects on inflammation and immune responses against Mtb infection are warranted.
AMPK-Induced Downstream Effectors That Coordinate Mitochondrial Function and Host Defense
In addition to these studies, previous work from our laboratory reported that AMPK activation resulted in increased mitochondrial biogenesis through peroxisome proliferator-activated receptor-γ coactivator (PGC)-1α, a downstream molecule of AMPK signaling (Yang et al., 2014). Importantly, PGC1α is critically involved in the antimicrobial immune defense and phagosomal maturation against Mtb (Yang et al., 2014). A more recent study revealed that estrogen-related receptor (ERR)-α, which is closely associated with PGC1α, was required for autophagy activation and host defense to Mtb infection (Kim et al., 2018). ERR-α works as an essential autophagy regulator by transcriptional and post-translational regulation of autophagy genes (Kim et al., 2018). Although ERR-α is an orphan nuclear receptor, for which the physiological ligands have not been known, ERR-α expression was upregulated by either AMPK or sirtuin 1 (SIRT1) activator (Kim et al., 2018). There is a mutual regulation between ERR-α and SIRT1 to enhance innate immune responses and ameliorate inflammation (Yuk et al., 2015; Kim et al., 2018). Treatment of Mtb-infected cells with SIRT1 activator resveratrol increased co-localization of Mtb phagosomes with lysosomes, suggesting an improved phagosomal maturation in macrophages (Kim et al., 2018). In addition, a recent study showed that SIRT1 is required for dampening immunopathogenesis of TB, suggesting a beneficial role for SIRT1 activation in host protection (Cheng et al., 2017). Moreover, AMPK activation is linked to mitochondrial biogenesis through activation of PGC1α and SIRT1 (Zhu et al., 2013; Marcinko and Steinberg, 2014; Wu et al., 2014; Tang, 2016). Nevertheless, more work is necessary to determine how PGC1α/ERRα/SIRT1 mediates anti-TB immunity, and whether AMPK-induced mitochondrial biogenesis itself serves to limit intracellular mycobacterial survival.
Small Molecules That Modulate AMPK to Regulate Host Defense against Mtb Infection
Given the opposing roles of AMPK and mTOR, significant attention has been given to small molecules and other compounds capable of activating AMPK or inhibiting mTOR as a potential therapeutic strategy for the host-directed therapy against TB (Floto et al., 2007; Schiebler et al., 2015; Singh and Subbian, 2018). Earlier studies suggest that pharmacologic inhibition of mTOR by rapamycin promotes phagosomal colocalization with autophagosomes and restriction of intracellular mycobacterial growth in macrophages (Gutierrez et al., 2004). Rapamycin is well-known for immunosuppressive activity to be useful for prevention of organ transplantation rejection (Pascual et al., 2016). Several mTOR inhibitors including everolimus are being used in the treatment of various types of cancers due to the inhibitory function in cell proliferation (Pohanka, 2006; Koh et al., 2013). Although there might be a possibility of mTOR inhibitors as candidates of host-directed therapy against multi-drug-resistance (MDR)- or extensively drug-resistance (XDR)-TB, the impact for mTOR inhibitor-based adjunctive therapy in the context of TB remains to be determined, because of serious side effects such as hyperglycemia and immunosuppression (Singh and Subbian, 2018).
Targeting AMPK in mycobacterial infection appears to promote anti-mycobacterial effects and inhibit much of the exaggerated inflammatory responses associated with disease pathology. Indeed, numerous autophagy-activating agents appear to work by triggering the AMPK pathway, making this protein a useful target for modulating the antimicrobial response to Mtb (Hoyer-Hansen and Jaattela, 2007; Fabri et al., 2011). In human monocytes/macrophages, vitamin D induces antimicrobial effects through autophagy activation via cathelicidin expression (Yuk et al., 2009). Four-phenylbutyrate and vitamin D-induced autophagy was dependent on the endogenous antimicrobial protein LL-37, of which LL-37-mediated autophagy required activation of the AMPK pathway (Rekha et al., 2015). In addition, activation of functional vitamin D receptor signaling by the mycobacterial antigen LpqH was shown to be dependent on the AMPK-mediated p38 MAPK pathway to produce cathelicidin LL-37 for antimicrobial responses against Mtb infection (Shin et al., 2010). Furthermore, AMPK activator AICAR showed beneficial effects through the enhancement of bacterial colocalization with autophagosomes to enhance phagosomal maturation and antimicrobial responses against Mtb infection (Yang et al., 2014). AICAR treatment of macrophages led to an induction of DRAM2, an autophagy protein targeted by miR144∗ in human monocytes/macrophages, to enhance host defense to Mtb (Kim J.K. et al., 2017). A recent study also showed that ohmyungsamycins, a class of newly identified cyclic peptides, activated autophagy and antimicrobial effects, and attenuated inflammatory responses against Mtb through AMPK pathway activation (Kim T.S. et al., 2017).
Notably, metformin, the well-known antidiabetic drug and AMPK activator, was shown to be beneficial for host protection, even in cases of drug-resistant Mtb (Singhal et al., 2014). As a treatment for Mtb, AMPK activation is required for metformin-mediated reduction of Mtb growth in macrophages. In addition, metformin treatment was shown to ameliorate chronic inflammation and lung pathology and improved bacterial control in a mouse model of infection. In TB patients, metformin therapy was associated with improvements in several clinical parameters, including disease severity (Singhal et al., 2014). The dual role for metformin in diabetes and Mtb infection is important, as diabetes itself is an independent risk factor for active TB, and is associated with TB severity (Jeon and Murray, 2008); TB may lead to glucose intolerance, with TB patients often experiencing difficulty in glycemic control (Yorke et al., 2017). While still unproven, targeting AMPK represents an exciting new treatment option for TB combined with diabetes, with strong efficacy in animal models of TB driven by its support of healthy mitochondria, reduced inflammation, and regulation of autophagy and apoptosis (Madhavi et al., 2018). Studies involving the functions of AMPK in mycobacterial infection is summarized in Table 1. More comprehensive studies are warranted to explore the prospect of targeting AMPK in patients with both diabetes and TB.
Finally, it should be noted that AMPK has been shown to drive immunopathology through the production of neutrophil MMP-8 (Ong et al., 2015). In addition, there is evidence of potential side effects associated with AMPK modulators (Choi et al., 2008; Wang and Hoyte, 2018). Studies employing the role of AMPK in mycobacterial infection are summarized in Table 1. Future development of new therapeutics targeting AMPK should consider the crosstalk of this kinase with other essential signaling pathways to achieve acceptable efficacy and safety while minimizing unwanted side effects during TB treatment.
Conclusion
Since its discovery as a pivotal regulator of intracellular bioenergetics, AMPK has emerged as a central coordinator of a variety of biological responses, including autophagy, inflammation, and metabolic reprogramming during mycobacterial infection (Shi et al., 2016; Riffelmacher et al., 2018). The functions of AMPK are diametrically opposed by those of mTOR, and the balance of the activation of both kinases is reflected in the signaling pathways that converge on host defense in host cells against Mtb infection (Yang et al., 2014). AMPK promotes the induction and maturation of autophagy in response to infectious stresses and activates the transcriptional factor TFEB, which targets specific signaling modules for lysosomal function and fatty acid β-oxidation (Ouimet et al., 2016). AMPK-mediated autophagy activation provides a cell-autonomous defense framework, which is required for enhancement of phagosomal acidification and restriction of intracellular parasites. In addition, AMPK signaling is tightly coupled to the attenuation of pathologic inflammation, which can be harmful to the host during prolonged infection with Mtb.
Understanding how AMPK-mTOR signaling functions in response to infectious threats, and how these pathways orchestrate immunometabolic responses is essential for proper control of infectious diseases. Although AMPK activation appears to be involved in the host protection to Mtb infection through downstream regulators including PGC-1α, ERRα, SIRT1, and PPARα, little is known about the full picture of AMPK-induced effector pathways. Many questions remain unanswered, including the mechanisms by which host defenses and pathology are controlled over the course of infection, and the nature of key players that participate in the control of metabolic shifts during TB. While AMPK is well established as a central regulator of innate effector networks, a better understanding of how AMPK orchestrates this complicated host-pathogen response will be necessary for the development of new therapeutic approaches for TB. Thus, a challenge for the future studies involves coordinating a dedicated mechanism of AMPK-targeted effector pathways that integrate the antibacterial autophagy, controlling pathologic inflammation, mitochondrial biogenesis, and immunometabolism during infection.
Author Contributions
E-KJ and J-MY contributed to conception and design of the study. E-KJ wrote the first draft of the manuscript. E-KJ, PS, and J-MY wrote sections of the manuscript. All authors contributed to manuscript revision, read and approved the submitted version.
Funding
This work was supported by the National Research Foundation of Korea (NRF) grant funded by the Korean Government (MSIP) (No. 2017R1A5A2015385), and supported under the framework of international cooperation program managed by NRF of Korea (Grant No. 2015K2A2A6002008).
Conflict of Interest Statement
The authors declare that the research was conducted in the absence of any commercial or financial relationships that could be construed as a potential conflict of interest.
Acknowledgments
We thank J.-K. Kim and H.-W. Suh for kind modification of the figures and critical reading of the paper. We are indebted to current and past members of our laboratory for discussions and investigations who contributed to this article. We apologize to colleagues whose work and publications could not be referenced owing to space constraints.
References
Almeida, L., Lochner, M., Berod, L., and Sparwasser, T. (2016). Metabolic pathways in T cell activation and lineage differentiation. Semin. Immunol. 28, 514–524. doi: 10.1016/j.smim.2016.10.009
Baay-Guzman, G. J., Duran-Padilla, M. A., Rangel-Santiago, J., Tirado-Rodriguez, B., Antonio-Andres, G., Barrios-Payan, J., et al. (2018). Dual role of hypoxia-inducible factor 1 alpha in experimental pulmonary tuberculosis: its implication as a new therapeutic target. Future Microbiol. 13, 785–798. doi: 10.2217/fmb-2017-0168
Bach, M., Larance, M., James, D. E., and Ramm, G. (2011). The serine/threonine kinase ULK1 is a target of multiple phosphorylation events. Biochem. J. 440, 283–291. doi: 10.1042/BJ20101894
Bekpen, C., Xavier, R. J., and Eichler, E. E. (2010). Human IRGM gene “to be or not to be”. Semin. Immunopathol. 32, 437–444. doi: 10.1007/s00281-010-0224-x
Carling, D. (2017). AMPK signalling in health and disease. Curr. Opin. Cell Biol. 45, 31–37. doi: 10.1016/j.ceb.2017.01.005
Carroll, B., and Dunlop, E. A. (2017). The lysosome: a crucial hub for AMPK and mTORC1 signalling. Biochem. J. 474, 1453–1466. doi: 10.1042/BCJ20160780
Chauhan, S., Kumar, S., Jain, A., Ponpuak, M., Mudd, M. H., Kimura, T., et al. (2016). TRIMs and galectins globally cooperate and TRIM16 and galectin-3 co-direct autophagy in endomembrane damage homeostasis. Dev. Cell 39, 13–27. doi: 10.1016/j.devcel.2016.08.003
Chauhan, S., Mandell, M. A., and Deretic, V. (2015). IRGM governs the core autophagy machinery to conduct antimicrobial defense. Mol. Cell 58, 507–521. doi: 10.1016/j.molcel.2015.03.020
Cheng, C. Y., Gutierrez, N. M., Marzuki, M. B., Lu, X., Foreman, T. W., Paleja, B., et al. (2017). Host sirtuin 1 regulates mycobacterial immunopathogenesis and represents a therapeutic target against tuberculosis. Sci. Immunol. 2:eaaj1789. doi: 10.1126/sciimmunol.aaj1789
Choi, A. M., Ryter, S. W., and Levine, B. (2013). Autophagy in human health and disease. N. Engl. J. Med. 368, 1845–1846. doi: 10.1056/NEJMc1303158
Choi, K., Mollapour, E., Choi, J. H., and Shears, S. B. (2008). Cellular energetic status supervises the synthesis of bis-diphosphoinositol tetrakisphosphate independently of AMP-activated protein kinase. Mol. Pharmacol. 74, 527–536. doi: 10.1124/mol.107.044628
Cooper, A. M. (2009). Cell-mediated immune responses in tuberculosis. Annu. Rev. Immunol. 27, 393–422. doi: 10.1146/annurev.immunol.021908.132703
Corradetti, M. N., Inoki, K., Bardeesy, N., DePinho, R. A., and Guan, K. L. (2004). Regulation of the TSC pathway by LKB1: evidence of a molecular link between tuberous sclerosis complex and Peutz-jeghers syndrome. Genes Dev. 18, 1533–1538. doi: 10.1101/gad.1199104
Cortese, M., Sinclair, C., and Pulendran, B. (2014). Translating glycolytic metabolism to innate immunity in dendritic cells. Cell Metab. 19, 737–739. doi: 10.1016/j.cmet.2014.04.012
Daffe, M., Crick, D. C., and Jackson, M. (2014). Genetics of capsular polysaccharides and cell envelope (glyco)lipids. Microbiol. Spectr. 2:14. doi: 10.1128/microbiolspec.MGM2-0021-2013
Deretic, V. (2008). Autophagy, an immunologic magic bullet: Mycobacterium tuberculosis phagosome maturation block and how to bypass it. Future Microbiol. 3, 517–524. doi: 10.2217/17460913.3.5.517
Deretic, V. (2011). Autophagy in immunity and cell-autonomous defense against intracellular microbes. Immunol. Rev. 240, 92–104. doi: 10.1111/j.1600-065X.2010.00995.x
Deretic, V., Delgado, M., Vergne, I., Master, S., De Haro, S., Ponpuak, M., et al. (2009). Autophagy in immunity against Mycobacterium tuberculosis: a model system to dissect immunological roles of autophagy. Curr. Top. Microbiol. Immunol. 335, 169–188. doi: 10.1007/978-3-642-00302-8-8
Deretic, V., Kimura, T., Timmins, G., Moseley, P., Chauhan, S., and Mandell, M. (2015). Immunologic manifestations of autophagy. J. Clin. Invest. 125, 75–84. doi: 10.1172/JCI73945
Diao, J., Liu, R., Rong, Y., Zhao, M., Zhang, J., Lai, Y., et al. (2015). ATG14 promotes membrane tethering and fusion of autophagosomes to endolysosomes. Nature 520, 563–566. doi: 10.1038/nature14147
Dorhoi, A., and Kaufmann, S. H. (2016). Pathology and immune reactivity: understanding multidimensionality in pulmonary tuberculosis. Semin. Immunopathol. 38, 153–166. doi: 10.1007/s00281-015-0531-3
Everts, B., Amiel, E., Huang, S. C., Smith, A. M., Chang, C. H., Lam, W. Y., et al. (2014). TLR-driven early glycolytic reprogramming via the kinases TBK1-IKKvarepsilon supports the anabolic demands of dendritic cell activation. Nat. Immunol. 15, 323–332. doi: 10.1038/ni.2833
Fabri, M., Realegeno, S. E., Jo, E. K., and Modlin, R. L. (2011). Role of autophagy in the host response to microbial infection and potential for therapy. Curr. Opin. Immunol. 23, 65–70. doi: 10.1016/j.coi.2010.10.010
Floto, R. A., Sarkar, S., Perlstein, E. O., Kampmann, B., Schreiber, S. L., and Rubinsztein, D. C. (2007). Small molecule enhancers of rapamycin-induced TOR inhibition promote autophagy, reduce toxicity in huntington’s disease models and enhance killing of mycobacteria by macrophages. Autophagy 3, 620–622. doi: 10.4161/auto.4898
Fratti, R. A., Chua, J., Vergne, I., and Deretic, V. (2003). Mycobacterium tuberculosis glycosylated phosphatidylinositol causes phagosome maturation arrest. Proc. Natl. Acad. Sci. U.S.A. 100, 5437–5442. doi: 10.1073/pnas.0737613100
Galvan-Pena, S., and O’Neill, L. A. (2014). Metabolic reprograming in macrophage polarization. Front. Immunol. 5:420. doi: 10.3389/fimmu.2014.00420
Grabacka, M., Pierzchalska, M., Dean, M., and Reiss, K. (2016). Regulation of ketone body metabolism and the role of PPARalpha. Int. J. Mol. Sci. 17:E2093. doi: 10.3390/ijms17122093
Grahame Hardie, D. (2016). Regulation of AMP-activated protein kinase by natural and synthetic activators. Acta Pharm. Sin. B 6, 1–19. doi: 10.1016/j.apsb.2015.06.002
Gray, M. A., Choy, C. H., Dayam, R. M., Ospina-Escobar, E., Somerville, A., Xiao, X., et al. (2016). Phagocytosis enhances lysosomal and bactericidal properties by activating the transcription factor TFEB. Curr. Biol. 26, 1955–1964. doi: 10.1016/j.cub.2016.05.070
Green, A. S., Chapuis, N., Lacombe, C., Mayeux, P., Bouscary, D., and Tamburini, J. (2011). LKB1/AMPK/mTOR signaling pathway in hematological malignancies: from metabolism to cancer cell biology. Cell Cycle 10, 2115–2120. doi: 10.4161/cc.10.13.16244
Griffiths, H. R., Gao, D., and Pararasa, C. (2017). Redox regulation in metabolic programming and inflammation. Redox Biol. 12, 50–57. doi: 10.1016/j.redox.2017.01.023
Gutierrez, M. G., Master, S. S., Singh, S. B., Taylor, G. A., Colombo, M. I., and Deretic, V. (2004). Autophagy is a defense mechanism inhibiting BCG and Mycobacterium tuberculosis survival in infected macrophages. Cell 119, 753–766. doi: 10.1016/j.cell.2004.11.038
Gwinn, D. M., Shackelford, D. B., Egan, D. F., Mihaylova, M. M., Mery, A., Vasquez, D. S., et al. (2008). AMPK phosphorylation of raptor mediates a metabolic checkpoint. Mol. Cell 30, 214–226. doi: 10.1016/j.molcel.2008.03.003
Hara, T., Takamura, A., Kishi, C., Iemura, S., Natsume, T., Guan, J. L., et al. (2008). FIP200, a ULK-interacting protein, is required for autophagosome formation in mammalian cells. J. Cell Biol. 181, 497–510. doi: 10.1083/jcb.200712064
Hardie, D. G. (2011a). AMP-activated protein kinase: a cellular energy sensor with a key role in metabolic disorders and in cancer. Biochem. Soc. Trans. 39, 1–13. doi: 10.1042/BST0390001
Hardie, D. G. (2011b). AMP-activated protein kinase: an energy sensor that regulates all aspects of cell function. Genes Dev. 25, 1895–1908. doi: 10.1101/gad.17420111
Hardie, D. G., and Alessi, D. R. (2013). LKB1 and AMPK and the cancer-metabolism link - ten years after. BMC Biol. 11:36. doi: 10.1186/1741-7007-11-36
Hardie, D. G., Schaffer, B. E., and Brunet, A. (2016). AMPK: an energy-sensing pathway with multiple inputs and outputs. Trends Cell Biol. 26, 190–201. doi: 10.1016/j.tcb.2015.10.013
Hmama, Z., Pena-Diaz, S., Joseph, S., and Av-Gay, Y. (2015). Immunoevasion and immunosuppression of the macrophage by Mycobacterium tuberculosis. Immunol. Rev. 264, 220–232. doi: 10.1111/imr.12268
Hoyer-Hansen, M., and Jaattela, M. (2007). AMP-activated protein kinase: a universal regulator of autophagy? Autophagy 3, 381–383.
Inoki, K., Kim, J., and Guan, K. L. (2012). AMPK and mTOR in cellular energy homeostasis and drug targets. Annu. Rev. Pharmacol. Toxicol. 52, 381–400. doi: 10.1146/annurev-pharmtox-010611-134537
Inokuchi-Shimizu, S., Park, E. J., Roh, Y. S., Yang, L., Zhang, B., Song, J., et al. (2014). TAK1-mediated autophagy and fatty acid oxidation prevent hepatosteatosis and tumorigenesis. J. Clin. Invest. 124, 3566–3578. doi: 10.1172/JCI74068
Itakura, E., Kishi-Itakura, C., and Mizushima, N. (2012). The hairpin-type tail-anchored SNARE syntaxin 17 targets to autophagosomes for fusion with endosomes/lysosomes. Cell 151, 1256–1269. doi: 10.1016/j.cell.2012.11.001
Iwasaki, K., Ray, P. D., Huang, B. W., Sakamoto, K., Kobayashi, T., and Tsuji, Y. (2013). Role of AMP-activated protein kinase in ferritin H gene expression by resveratrol in human T cells. Biochemistry 52, 5075–5083. doi: 10.1021/bi400399f
Jeon, C. Y., and Murray, M. B. (2008). Diabetes mellitus increases the risk of active tuberculosis: a systematic review of 13 observational studies. PLoS Med. 5:e152. doi: 10.1371/journal.pmed.0050152
Jha, A. K., Huang, S. C., Sergushichev, A., Lampropoulou, V., Ivanova, Y., Loginicheva, E., et al. (2015). Network integration of parallel metabolic and transcriptional data reveals metabolic modules that regulate macrophage polarization. Immunity 42, 419–430. doi: 10.1016/j.immuni.2015.02.005
Jia, J., Abudu, Y. P., Claude-Taupin, A., Gu, Y., Kumar, S., Choi, S. W., et al. (2018). Galectins control mTOR in response to endomembrane damage. Mol. Cell 70, 120.e8–135.e8. doi: 10.1016/j.molcel.2018.03.009
Kelly, B., and O’Neill, L. A. (2015). Metabolic reprogramming in macrophages and dendritic cells in innate immunity. Cell Res. 25, 771–784. doi: 10.1038/cr.2015.68
Kim, J., Kundu, M., Viollet, B., and Guan, K. L. (2011). AMPK and mTOR regulate autophagy through direct phosphorylation of Ulk1. Nat. Cell Biol. 13, 132–141. doi: 10.1038/ncb2152
Kim, J. K., Lee, H. M., Park, K. S., Shin, D. M., Kim, T. S., Kim, Y. S., et al. (2017). MIR144∗ inhibits antimicrobial responses against Mycobacterium tuberculosis in human monocytes and macrophages by targeting the autophagy protein DRAM2. Autophagy 13, 423–441. doi: 10.1080/15548627.2016.1241922
Kim, T. S., Shin, Y. H., Lee, H. M., Kim, J. K., Choe, J. H., Jang, J. C., et al. (2017). Ohmyungsamycins promote antimicrobial responses through autophagy activation via AMP-activated protein kinase pathway. Sci. Rep. 7:3431. doi: 10.1038/s41598-017-03477-3
Kim, Y. S., Lee, H. M., Kim, J. K., Yang, C. S., Kim, T. S., Jung, M., et al. (2017). PPAR-alpha activation mediates innate host defense through induction of tfeb and lipid catabolism. J. Immunol. 198, 3283–3295. doi: 10.4049/jimmunol.1601920
Kim, S. Y., Yang, C. S., Lee, H. M., Kim, J. K., Kim, Y. S., Kim, Y. R., et al. (2018). ESRRA (estrogen-related receptor alpha) is a key coordinator of transcriptional and post-translational activation of autophagy to promote innate host defense. Autophagy 14, 152–168. doi: 10.1080/15548627.2017.1339001
Koh, Y., Lim, H. Y., Ahn, J. H., Lee, J. L., Rha, S. Y., Kim, Y. J., et al. (2013). Phase II trial of everolimus for the treatment of nonclear-cell renal cell carcinoma. Ann. Oncol. 24, 1026–1031. doi: 10.1093/annonc/mds582
Korolchuk, V. I., Saiki, S., Lichtenberg, M., Siddiqi, F. H., Roberts, E. A., Imarisio, S., et al. (2011). Lysosomal positioning coordinates cellular nutrient responses. Nat. Cell. Biol. 13, 453–460. doi: 10.1038/ncb2204
Krawczyk, C. M., Holowka, T., Sun, J., Blagih, J., Amiel, E., DeBerardinis, R. J., et al. (2010). Toll-like receptor-induced changes in glycolytic metabolism regulate dendritic cell activation. Blood 115, 4742–4749. doi: 10.1182/blood-2009-10-249540
Lachmandas, E., Beigier-Bompadre, M., Cheng, S. C., Kumar, V., van Laarhoven, A., Wang, X., et al. (2016). Rewiring cellular metabolism via the AKT/mTOR pathway contributes to host defence against Mycobacterium tuberculosis in human and murine cells. Eur. J. Immunol. 46, 2574–2586. doi: 10.1002/eji.201546259
Lee, K. A., Cho, K. C., Kim, B., Jang, I. H., Nam, K., Kwon, Y. E., et al. (2018). Inflammation-modulated metabolic reprogramming is required for duox-dependent gut immunity in drosophila. Cell Host Microbe 23, 338.e5–352.e5. doi: 10.1016/j.chom.2018.01.011
Levine, B., and Deretic, V. (2007). Unveiling the roles of autophagy in innate and adaptive immunity. Nat. Rev. Immunol. 7, 767–777. doi: 10.1038/nri2161
Liu, C. H., Liu, H., and Ge, B. (2017). Innate immunity in tuberculosis: host defense vs pathogen evasion. Cell Mol. Immunol. 14, 963–975. doi: 10.1038/cmi.2017.88
Lugo-Villarino, G., Troegeler, A., Balboa, L., Lastrucci, C., Duval, C., Mercier, I., et al. (2018). The C-type lectin receptor DC-SIGN has an anti-inflammatory role in human M(IL-4) macrophages in response to Mycobacterium tuberculosis. Front. Immunol. 9:1123. doi: 10.3389/fimmu.2018.01123
MacMicking, J. D., Taylor, G. A., and McKinney, J. D. (2003). Immune control of tuberculosis by IFN-gamma-inducible LRG-47. Science 302, 654–659. doi: 10.1126/science.1088063
Madhavi, Y. V., Gaikwad, N., Yerra, V. G., Kalvala, A. K., Nanduri, S., and Kumar, A. (2018). Targeting AMPK in diabetes and diabetic complications: energy homeostasis, autophagy and mitochondrial health. Curr. Med. Chem. doi: 10.2174/0929867325666180406120051 [Epub ahead of print].
Mahon, R. N., and Hafner, R. (2015). Immune cell regulatory pathways unexplored as host-directed therapeutic targets for Mycobacterium tuberculosis: an opportunity to apply precision medicine innovations to infectious diseases. Clin. Infect. Dis. 61(Suppl. 3), S200–S216. doi: 10.1093/cid/civ621
Man, S. M., and Kanneganti, T. D. (2016). Regulation of lysosomal dynamics and autophagy by CTSB/cathepsin B. Autophagy 12, 2504–2505. doi: 10.1080/15548627.2016.1239679
Marcelo, K. L., Means, A. R., and York, B. (2016). The Ca(2+)/Calmodulin/CaMKK2 axis: nature’s metabolic CaMshaft. Trends Endocrinol. Metab. 27, 706–718. doi: 10.1016/j.tem.2016.06.001
Marcinko, K., and Steinberg, G. R. (2014). The role of AMPK in controlling metabolism and mitochondrial biogenesis during exercise. Exp. Physiol. 99, 1581–1585. doi: 10.1113/expphysiol.2014.082255
Martina, J. A., Chen, Y., Gucek, M., and Puertollano, R. (2012). MTORC1 functions as a transcriptional regulator of autophagy by preventing nuclear transport of TFEB. Autophagy 8, 903–914. doi: 10.4161/auto.19653
Matta, S. K., and Kumar, D. (2016). Hypoxia and classical activation limits Mycobacterium tuberculosis survival by Akt-dependent glycolytic shift in macrophages. Cell Death Discov. 2:16022. doi: 10.1038/cddiscovery.2016.22
Mercer, C. A., Kaliappan, A., and Dennis, P. B. (2009). A novel, human Atg13 binding protein, Atg101, interacts with ULK1 and is essential for macroautophagy. Autophagy 5, 649–662. doi: 10.4161/auto.5.5.8249
Michelucci, A., Cordes, T., Ghelfi, J., Pailot, A., Reiling, N., Goldmann, O., et al. (2013). Immune-responsive gene 1 protein links metabolism to immunity by catalyzing itaconic acid production. Proc. Natl. Acad. Sci. U.S.A. 110, 7820–7825. doi: 10.1073/pnas.1218599110
Mizushima, N., Levine, B., Cuervo, A. M., and Klionsky, D. J. (2008). Autophagy fights disease through cellular self-digestion. Nature 451, 1069–1075. doi: 10.1038/nature06639
Moreira, D., Silvestre, R., Cordeiro-da-Silva, A., Estaquier, J., Foretz, M., and Viollet, B. (2016). AMP-activated protein kinase as a target for pathogens: friends or foes? Curr. Drug Targets 17, 942–953. doi: 10.2174/1389450116666150416120559
Neumann, D. (2018). Is TAK1 a direct upstream kinase of AMPK? Int. J. Mol. Sci. 19:E2412. doi: 10.3390/ijms19082412
Ni Cheallaigh, C., Keane, J., Lavelle, E. C., Hope, J. C., and Harris, J. (2011). Autophagy in the immune response to tuberculosis: clinical perspectives. Clin. Exp. Immunol. 164, 291–300. doi: 10.1111/j.1365-2249.2011.04381.x
Novikova, D. S., Garabadzhiu, A. V., Melino, G., Barlev, N. A., and Tribulovich, V. G. (2015). AMP-activated protein kinase: structure, function, and role in pathological processes. Biochemistry 80, 127–144. doi: 10.1134/S0006297915020017
O’Neill, L. A., and Hardie, D. G. (2013). Metabolism of inflammation limited by AMPK and pseudo-starvation. Nature 493, 346–355. doi: 10.1038/nature11862
O’Neill, L. A., Kishton, R. J., and Rathmell, J. (2016). A guide to immunometabolism for immunologists. Nat. Rev. Immunol. 16, 553–565. doi: 10.1038/nri.2016.70
O’Neill, L. A., and Pearce, E. J. (2016). Immunometabolism governs dendritic cell and macrophage function. J. Exp. Med. 213, 15–23. doi: 10.1084/jem.20151570
Ong, C. W., Elkington, P. T., Brilha, S., Ugarte-Gil, C., Tome-Esteban, M. T., Tezera, L. B., et al. (2015). Neutrophil-derived MMP-8 drives AMPK-dependent matrix destruction in human pulmonary tuberculosis. PLoS Pathog. 11:e1004917. doi: 10.1371/journal.ppat.1004917
Ouimet, M., Koster, S., Sakowski, E., Ramkhelawon, B., van Solingen, C., Oldebeken, S., et al. (2016). Mycobacterium tuberculosis induces the miR-33 locus to reprogram autophagy and host lipid metabolism. Nat. Immunol. 17, 677–686. doi: 10.1038/ni.3434
Paik, S., Kim, J. K., Chung, C., and Jo, E. K. (2018). Autophagy: a new strategy for host-directed therapy of tuberculosis. Virulence doi: 10.1080/21505594.2018.1536598 [Epub ahead of print].
Pascual, J., Royuela, A., Fernandez, A. M., Herrero, I., Delgado, J. F., Sole, A., et al. (2016). Role of mTOR inhibitors for the control of viral infection in solid organ transplant recipients. Transpl. Infect. Dis. 18, 819–831. doi: 10.1111/tid.12601
Peixoto, C. A., Oliveira, W. H., Araujo, S., and Nunes, A. K. S. (2017). AMPK activation: role in the signaling pathways of neuroinflammation and neurodegeneration. Exp. Neurol. 298(Pt A), 31–41. doi: 10.1016/j.expneurol.2017.08.013
Pineros, A. R., Campos, L. W., Fonseca, D. M., Bertolini, T. B., Gembre, A. F., Prado, R. Q., et al. (2017). M2 macrophages or IL-33 treatment attenuate ongoing Mycobacterium tuberculosis infection. Sci. Rep. 7:41240. doi: 10.1038/srep41240
Pohanka, E. (2006). Conversion to everolimus in maintenance patients–current clinical strategies. Nephrol. Dial. Transplant. 21(Suppl. 3), iii24–iii29. doi: 10.1093/ndt/gfl301
Prantner, D., Perkins, D. J., and Vogel, S. N. (2017). AMP-activated kinase (AMPK) promotes innate immunity and antiviral defense through modulation of stimulator of interferon genes (STING) signaling. J. Biol. Chem. 292, 292–304. doi: 10.1074/jbc.M116.763268
Qi, X., Man, S. M., Malireddi, R. K., Karki, R., Lupfer, C., Gurung, P., et al. (2016). Cathepsin B modulates lysosomal biogenesis and host defense against Francisella novicida infection. J. Exp. Med. 213, 2081–2097. doi: 10.1084/jem.20151938
Reddy, V. P., Chinta, K. C., Saini, V., Glasgow, J. N., Hull, T. D., Traylor, A., et al. (2018). Ferritin H deficiency in myeloid compartments dysregulates host energy metabolism and increases susceptibility to Mycobacterium tuberculosis infection. Front. Immunol. 9:860. doi: 10.3389/fimmu.2018.00860
Rekha, R. S., Rao Muvva, S. S., Wan, M., Raqib, R., Bergman, P., Brighenti, S., et al. (2015). Phenylbutyrate induces LL-37-dependent autophagy and intracellular killing of Mycobacterium tuberculosis in human macrophages. Autophagy 11, 1688–1699. doi: 10.1080/15548627.2015.1075110
Riffelmacher, T., Richter, F. C., and Simon, A. K. (2018). Autophagy dictates metabolism and differentiation of inflammatory immune cells. Autophagy 14, 199–206. doi: 10.1080/15548627.2017.1362525
Roczniak-Ferguson, A., Petit, C. S., Froehlich, F., Qian, S., Ky, J., Angarola, B., et al. (2012). The transcription factor TFEB links mTORC1 signaling to transcriptional control of lysosome homeostasis. Sci. Signal. 5:ra42. doi: 10.1126/scisignal.2002790
Ruderman, N. B., Carling, D., Prentki, M., and Cacicedo, J. M. (2013). AMPK, insulin resistance, and the metabolic syndrome. J. Clin. Invest. 123, 2764–2772. doi: 10.1172/JCI67227
Sag, D., Carling, D., Stout, R. D., and Suttles, J. (2008). Adenosine 5’-monophosphate-activated protein kinase promotes macrophage polarization to an anti-inflammatory functional phenotype. J. Immunol. 181, 8633–8641. doi: 10.4049/jimmunol.181.12.8633
Sahu, S. K., Kumar, M., Chakraborty, S., Banerjee, S. K., Kumar, R., Gupta, P., et al. (2017). MicroRNA 26a (miR-26a)/KLF4 and CREB-C/EBPbeta regulate innate immune signaling, the polarization of macrophages and the trafficking of Mycobacterium tuberculosis to lysosomes during infection. PLoS Pathog. 13:e1006410. doi: 10.1371/journal.ppat.1006410
Schiebler, M., Brown, K., Hegyi, K., Newton, S. M., Renna, M., Hepburn, L., et al. (2015). Functional drug screening reveals anticonvulsants as enhancers of mTOR-independent autophagic killing of Mycobacterium tuberculosis through inositol depletion. EMBO Mol. Med. 7, 127–139. doi: 10.15252/emmm.201404137
Schorey, J. S., and Schlesinger, L. S. (2016). Innate immune responses to tuberculosis. Microbiol Spectr 4, 3–31. doi: 10.1128/microbiolspec.TBTB2-0010-2016
Settembre, C., Di Malta, C., Polito, V. A., Garcia Arencibia, M., Vetrini, F., Erdin, S., et al. (2011). TFEB links autophagy to lysosomal biogenesis. Science 332, 1429–1433. doi: 10.1126/science.1204592
Shah, S. Z. A., Zhao, D., Hussain, T., and Yang, L. (2017). Role of the AMPK pathway in promoting autophagic flux via modulating mitochondrial dynamics in neurodegenerative diseases: Insight into prion diseases. Ageing Res. Rev. 40, 51–63. doi: 10.1016/j.arr.2017.09.004
Shi, L., Eugenin, E. A., and Subbian, S. (2016). Immunometabolism in tuberculosis. Front. Immunol. 7:150. doi: 10.3389/fimmu.2016.00150
Shi, L. Z., Wang, R., Huang, G., Vogel, P., Neale, G., Green, D. R., et al. (2011). HIF1alpha-dependent glycolytic pathway orchestrates a metabolic checkpoint for the differentiation of TH17 and Treg cells. J. Exp. Med. 208, 1367–1376. doi: 10.1084/jem.20110278
Shin, D. M., Yuk, J. M., Lee, H. M., Lee, S. H., Son, J. W., Harding, C. V., et al. (2010). Mycobacterial lipoprotein activates autophagy via TLR2/1/CD14 and a functional vitamin D receptor signalling. Cell Microbiol. 12, 1648–1665. doi: 10.1111/j.1462-5822.2010.01497.x
Silwal, P., Kim, J. K., Yuk, J. M., and Jo, E. K. (2018). AMP-Activated protein kinase and host defense against infection. Int. J. Mol. Sci. 19:E3495. doi: 10.3390/ijms19113495
Singh, P., and Subbian, S. (2018). Harnessing the mTOR pathway for tuberculosis treatment. Front. Microbiol. 9:70. doi: 10.3389/fmicb.2018.00070
Singh, S. B., Davis, A. S., Taylor, G. A., and Deretic, V. (2006). Human IRGM induces autophagy to eliminate intracellular mycobacteria. Science 313, 1438–1441. doi: 10.1126/science.1129577
Singh, S. B., Ornatowski, W., Vergne, I., Naylor, J., Delgado, M., Roberts, E., et al. (2010). Human IRGM regulates autophagy and cell-autonomous immunity functions through mitochondria. Nat. Cell Biol. 12, 1154–1165. doi: 10.1038/ncb2119
Singhal, A., Jie, L., Kumar, P., Hong, G. S., Leow, M. K., Paleja, B., et al. (2014). Metformin as adjunct antituberculosis therapy. Sci. Transl. Med. 6:263ra159. doi: 10.1126/scitranslmed.3009885
Stutz, M. D., Clark, M. P., Doerflinger, M., and Pellegrini, M. (2018). Mycobacterium tuberculosis: rewiring host cell signaling to promote infection. J. Leukoc. Biol. 103, 259–268. doi: 10.1002/JLB.4MR0717-277R
Sun, J., Mu, Y., Jiang, Y., Song, R., Yi, J., Zhou, J., et al. (2018). Inhibition of p70 S6 kinase activity by A77 1726 induces autophagy and enhances the degradation of superoxide dismutase 1 (SOD1) protein aggregates. Cell Death Dis. 9:407. doi: 10.1038/s41419-018-0441-0
Szrejder, M., and Piwkowska, A. (2019). AMPK signaling: implications for podocyte biology in diabetic nephropathy. Biol. Cell doi: 10.1111/boc.201800077 [Epub ahead of print].
Tang, B. L. (2016). Sirt1 and the mitochondria. Mol. Cells 39, 87–95. doi: 10.14348/molcells.2016.2318
Tannahill, G. M., Curtis, A. M., Adamik, J., Palsson-McDermott, E. M., McGettrick, A. F., Goel, G., et al. (2013). Succinate is an inflammatory signal that induces IL-1beta through HIF-1alpha. Nature 496, 238–242. doi: 10.1038/nature11986
Tiwari, S., Choi, H. P., Matsuzawa, T., Pypaert, M., and MacMicking, J. D. (2009). Targeting of the GTPase Irgm1 to the phagosomal membrane via PtdIns(3,4)P(2) and PtdIns(3,4,5)P(3) promotes immunity to mycobacteria. Nat. Immunol. 10, 907–917. doi: 10.1038/ni.1759
Wang, G. S., and Hoyte, C. (2018). Review of biguanide (metformin) toxicity. J. Inten. Care Med. doi: 10.1177/0885066618793385 [Epub ahead of print].
Watson, R. O., Manzanillo, P. S., and Cox, J. S. (2012). Extracellular M. tuberculosis DNA targets bacteria for autophagy by activating the host DNA-sensing pathway. Cell 150, 803–815. doi: 10.1016/j.cell.2012.06.040
Williams, N. C., and O’Neill, L. A. J. (2018). A role for the krebs cycle intermediate citrate in metabolic reprogramming in innate immunity and inflammation. Front. Immunol. 9:141. doi: 10.3389/fimmu.2018.00141
Wong, P. M., Puente, C., Ganley, I. G., and Jiang, X. (2013). The ULK1 complex: sensing nutrient signals for autophagy activation. Autophagy 9, 124–137. doi: 10.4161/auto.23323
Wu, S. B., Wu, Y. T., Wu, T. P., and Wei, Y. H. (2014). Role of AMPK-mediated adaptive responses in human cells with mitochondrial dysfunction to oxidative stress. Biochim. Biophys. Acta 1840, 1331–1344. doi: 10.1016/j.bbagen.2013.10.034
Xie, M., Zhang, D., Dyck, J. R., Li, Y., Zhang, H., Morishima, M., et al. (2006). A pivotal role for endogenous TGF-beta-activated kinase-1 in the LKB1/AMP-activated protein kinase energy-sensor pathway. Proc. Natl. Acad. Sci. U.S.A. 103, 17378–17383. doi: 10.1073/pnas.0604708103
Xu, X., Sun, J., Song, R., Doscas, M. E., Williamson, A. J., Zhou, J., et al. (2017). Inhibition of p70 S6 kinase (S6K1) activity by A77 1726, the active metabolite of leflunomide, induces autophagy through TAK1-mediated AMPK and JNK activation. Oncotarget 8, 30438–30454. doi: 10.18632/oncotarget.16737
Yang, C. S., Kim, J. J., Lee, H. M., Jin, H. S., Lee, S. H., Park, J. H., et al. (2014). The AMPK-PPARGC1A pathway is required for antimicrobial host defense through activation of autophagy. Autophagy 10, 785–802. doi: 10.4161/auto.28072
Yang, C. S., Song, C. H., Lee, J. S., Jung, S. B., Oh, J. H., Park, J., et al. (2006). Intracellular network of phosphatidylinositol 3-kinase, mammalian target of the rapamycin/70 kDa ribosomal S6 kinase 1, and mitogen-activated protein kinases pathways for regulating mycobacteria-induced IL-23 expression in human macrophages. Cell Microbiol. 8, 1158–1171. doi: 10.1111/j.1462-5822.2006.00699.x
Yao, F., Zhang, M., and Chen, L. (2016). 5’-Monophosphate-activated protein kinase (AMPK) improves autophagic activity in diabetes and diabetic complications. Acta Pharm. Sin. B 6, 20–25. doi: 10.1016/j.apsb.2015.07.009
Yorke, E., Atiase, Y., Akpalu, J., Sarfo-Kantanka, O., Boima, V., and Dey, I. D. (2017). The bidirectional relationship between tuberculosis and diabetes. Tuberc. Res. Treat. 2017:1702578. doi: 10.1155/2017/1702578
Yuk, J. M., Kim, T. S., Kim, S. Y., Lee, H. M., Han, J., Dufour, C. R., et al. (2015). Orphan nuclear receptor ERRalpha controls macrophage metabolic signaling and A20 expression to negatively regulate tlr-induced inflammation. Immunity 43, 80–91. doi: 10.1016/j.immuni.2015.07.003
Yuk, J. M., Shin, D. M., Lee, H. M., Yang, C. S., Jin, H. S., Kim, K. K., et al. (2009). Vitamin D3 induces autophagy in human monocytes/macrophages via cathelicidin. Cell Host Microbe 6, 231–243. doi: 10.1016/j.chom.2009.08.004
Zhang, D., Wang, W., Sun, X., Xu, D., Wang, C., Zhang, Q., et al. (2016). AMPK regulates autophagy by phosphorylating BECN1 at threonine 388. Autophagy 12, 1447–1459. doi: 10.1080/15548627.2016.1185576
Zhang, J., Wang, Y., Liu, X., Dagda, R. K., and Zhang, Y. (2017). How AMPK and PKA interplay to regulate mitochondrial function and survival in models of ischemia and diabetes. Oxid. Med. Cell Longev. 2017:4353510. doi: 10.1155/2017/4353510
Keywords: AMPK, mycobacteria, macrophage, autophagy, mitochondria, immunometabolism
Citation: Jo E-K, Silwal P and Yuk J-M (2019) AMPK-Targeted Effector Networks in Mycobacterial Infection. Front. Microbiol. 10:520. doi: 10.3389/fmicb.2019.00520
Received: 14 December 2018; Accepted: 28 February 2019;
Published: 15 March 2019.
Edited by:
Maria Laura Gennaro, Rutgers, The State University of New Jersey, United StatesReviewed by:
Vikram Saini, All India Institute of Medical Sciences, IndiaShashank Gupta, Brown University, United States
Copyright © 2019 Jo, Silwal and Yuk. This is an open-access article distributed under the terms of the Creative Commons Attribution License (CC BY). The use, distribution or reproduction in other forums is permitted, provided the original author(s) and the copyright owner(s) are credited and that the original publication in this journal is cited, in accordance with accepted academic practice. No use, distribution or reproduction is permitted which does not comply with these terms.
*Correspondence: Eun-Kyeong Jo, aGF5b3VuZ2pAY251LmFjLmty