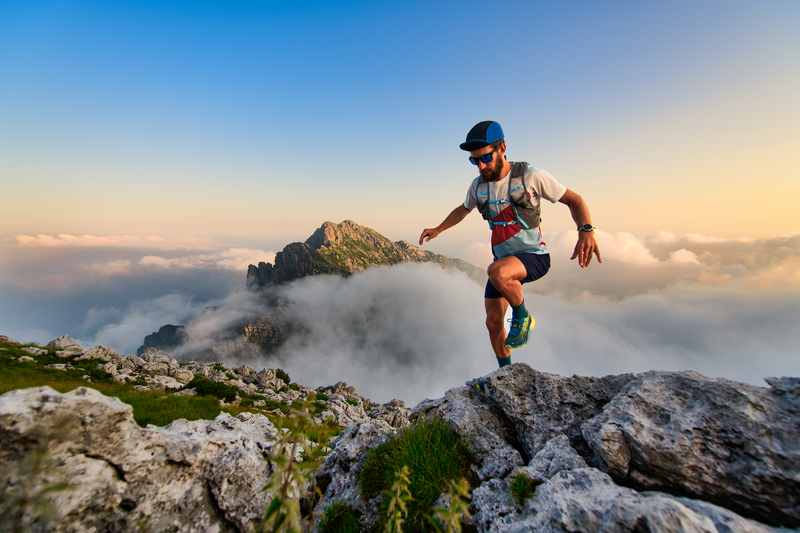
94% of researchers rate our articles as excellent or good
Learn more about the work of our research integrity team to safeguard the quality of each article we publish.
Find out more
ORIGINAL RESEARCH article
Front. Microbiol. , 07 March 2019
Sec. Extreme Microbiology
Volume 10 - 2019 | https://doi.org/10.3389/fmicb.2019.00381
This article is part of the Research Topic Microbial Life Under Stress: Biochemical, Genomic, Transcriptomic, Proteomic, Bioinformatics, Evolutionary Aspects and Biotechnological Applications of Poly-Extremophilic Bacteria View all 27 articles
Cyclic and linear nucleotides are key elements of the signal transduction networks linking perception of the environment to specific cellular behavior of prokaryotes. These molecular mechanisms are particularly important in bacteria exposed to different, and frequently simultaneous, types of extreme conditions. This is the case in acidithiobacilli, a group of extremophilic bacteria thriving in highly acidic biotopes, that must also cope with significant variations in temperature, osmotic potentials and concentrations of various transition metals and metalloids. Environmental cues sensed by bacteria are transduced into differential levels of nucleotides acting as intracellular second messengers, promoting the activation or inhibition of target components and eliciting different output phenotypes. Cyclic (c) di-GMP, one of the most common bacterial second messengers, plays a key role in lifestyle changes in many bacteria, including acidithiobacilli. The presence of functional c-di-GMP-dependent signal transduction pathways in representative strains of the best-known linages of this species complex has been reported. However, a comprehensive panorama of the c-di-GMP modulated networks, the cognate input signals and output responses, are still missing for this group of extremophiles. Moreover, little fundamental understanding has been gathered for other nucleotides acting as second messengers. Taking advantage of the increasing number of sequenced genomes of the taxon, here we address the challenge of disentangling the nucleotide-driven signal transduction pathways in this group of polyextremophiles using comparative genomic tools and strategies. Results indicate that the acidithiobacilli possess all the genetic elements required to establish functional transduction pathways based in three different nucleotide-second messengers: (p)ppGpp, cyclic AMP (cAMP), and c-di-GMP. The elements related with the metabolism and transduction of (p)ppGpp and cAMP appear highly conserved, integrating signals related with nutrient starvation and polyphosphate metabolism, respectively. In contrast, c-di-GMP networks appear diverse and complex, differing both at the species and strain levels. Molecular elements of c-di-GMP metabolism and transduction were mostly found scattered along the flexible genome of the acidithiobacilli, allowing the identification of probable control modules that could be critical for substrate colonization, biofilm development and intercellular interactions. These may ultimately convey increased endurance to environmental stress and increased potential for gene sharing and adaptation to changing conditions.
Acidithiobacillus are chemolithoautotrophic Gram-negative bacteria that thrive in environments with few nutrients, high concentrations of heavy metals and extreme acidity. They obtain energy from the oxidation of reduced inorganic sulfur compounds (RISCs), including many metal sulfides, one of the most abundant mineral classes on earth (Rohwerder and Sand, 2007). Bio-oxidation of metal sulfides allows the release of metal cations from minerals into solution and the production of sulfuric acid as a by-product. Therefore, the acidithiobacilli not only tolerate a wide range of metal ions and low pH (Dopson et al., 2003), but also are key players in promoting these extreme conditions.
Acidithiobacillus species have been the focus of research in areas as diverse as astrobiology (González-Toril et al., 2005) and nanotechnology (Ulloa et al., 2018). However, it is in the context of biomining applications and associated acid mine drainage phenomena that they have been more thoroughly studied. Microbial oxidation of metal sulfides has been used by the mining industry as a biotechnological process for metal extraction, allowing the recovery of economically relevant elements such as cobalt, copper, nickel, uranium, and zinc (Harrison, 2016). On the other hand, microbial leaching activity promotes the formation of highly contaminating metal loaded acidic waters that permeate into waterways generating acid rock or acid mine drainages (ARD/AMD) both in mining sites and in naturally exposed ore deposits, acidic rivers, geothermal sites and caves, impacting enormously human life and wildlife (Johnson and Hallberg, 2005).
Despite common characteristics, the genus Acidithiobacillus is a heterogeneous group of bacteria, currently consisting of seven species: A. thiooxidans (Waksman and Joffe, 1922), A. ferrooxidans (Temple and Colmer, 1951), A. albertensis (Bryant et al., 1983), A. caldus (Hallberg and Lindström, 1994), A. ferrivorans (Hallberg et al., 2010), A. ferridurans (Hedrich and Johnson, 2013), and A. ferriphilus (Falagán and Johnson, 2016). The most notable differences among these include the capability to oxidize ferrous to ferric iron (A. ferrooxidans, A. ferrivorans, A. ferridurans, and A. ferriphilus); the presence/absence of flagella-based motility (Valdés et al., 2008; Castro et al., 2017); and the growth temperature ranges. While most of acidithiobacilli are mesophilic (∼30°C), the psychrotolerant A. ferrivorans and the moderate thermophlic A. caldus expand the temperature niche of this taxon, the former by being able to grow at 4°C, and the latter withstanding 45°C. These differences influence substrate colonization and dominance by the diverse acidithiobacilli in different micro-niches in natural environments as well as in bioleaching operations. Since bio-oxidation activity depends largely on the physiological state of the cell, the understanding of molecular mechanisms that allow the bacteria to sense and respond to their challenging environment becomes relevant.
Different bacteria use linear and cyclic nucleotides as second messengers to regulate diverse cellular processes in response to environmental cues. Among the most important ones are cyclic adenosine 3′,5′-monophosphate (cAMP) (McDonough and Rodriguez, 2011), cyclic guanosine 3′,5′-monophosphate (cGMP) (Marden et al., 2011), cyclic dimeric guanosine 3′,5′-monophosphate (c-di-GMP) (Römling et al., 2013), cyclic dimeric adenosine 3′,5′-monophosphate (c-di-AMP) (Corrigan and Gründling, 2013), guanosine 3′,5′-bispyrophosphate (ppGpp) and guanosine 3′-diphosphate, 5′-triphosphate (pppGpp) (Hauryliuk et al., 2015). Despite the structural diversity of these nucleotides, their signaling pathways work in analogous ways. In response to environmental signals (first messenger), different enzymatic activities catalyze the synthesis or the degradation of specific nucleotide second messengers, amplifying the signal. Nucleotide levels are mainly transduced by effector proteins, which directly bind the nucleotide and interact with target components promoting diverse responses. Synthesis and degradation enzymes together with effectors and target components that work in concerted action to produce a particular output phenotype, through a common pool of the nucleotide molecules, constitute a second-messenger control module (Hengge, 2009). These control modules show important differences in terms of the nature of detected signal and the range of the output response.
Cyclic AMP (cAMP) and (p)ppGpp have been studied for decades in the context of nutrient deprivation in E. coli. In this neutrophilic bacterium, amino acid deficit promotes the “stringent response,” a series of physiological changes driven by an increase of intracellular levels of (p)ppGpp. Acting at transcriptional and post-traductional levels, (p)ppGpp regulates biosynthesis of amino acid and ribosomal proteins (Potrykus and Cashel, 2008) and represses nucleotide metabolism (Gallant et al., 1971; Hochstadt-Ozer and Cashel, 1972). Furthermore, in response to low energy charge (low levels of ATP), E. coli increases the intracellular cAMP concentration, promoting catabolism and inhibiting anabolism through gene regulation (Narang, 2009). More recently, work on the α-proteobacterium Rhodospirillum centenum demonstrated the role of cGMP in cyst development, metabolically dormant cells able to survive environmental stresses such as desiccation and nutrient starvation (Marden et al., 2011). Cyclic-di-AMP has been implicated in diverse essential cellular processes in bacteria (mainly in Gram-positive), including cell wall and membrane homeostasis, regulation of potassium ion channels, DNA damage repair, and sporulation (Commichau et al., 2018), although the triggering environmental stimuli are less well-characterized. Cyclic-di-GMP is the most common bacterial second messenger, being widespread in most bacterial phyla, in which it controls a variety of processes related with lifestyle and the switch from the individual motile state to the multicellular biofilm state (Jenal et al., 2017).
These signal transduction pathways are particularly important in bacteria exposed to different, and frequently simultaneous, types of extreme conditions. This is the case of the acidithiobacilli, which in addition to enduring extreme acidic conditions must cope with significant variations in temperature, osmotic potentials and concentrations of various transition metals and metalloids. Despite this fact, little is known about the scope of regulation through second messenger-driven mechanisms in this group of polyextemophiles. Recently, some components of the c-di-GMP pathway of type strains of A. ferrooxidans (Ruiz et al., 2012), A. thiooxidans (Díaz et al., 2013, 2018), and A. caldus (Castro et al., 2015) have been characterized. In these acidophiles, c-di-GMP signaling is related to biofilm development, a key lifestyle for substrate colonization and energy acquisition. However, as it occurs in most bacteria, the multiplicity of c-di-GMP related genes in the same microorganism hinders the determination of specific c-di-GMP control modules. Moreover, the presence and expression of multiple c-di-GMP related genes under the same conditions (Castro et al., 2015; Díaz et al., 2018) suggest the need for a spatial post-translational regulation.
Taking advantage of the increasing number of sequenced genomes of the taxon, here we address the challenge of disentangling the nucleotide-driven signal transduction pathways in this group of polyextremophiles by using a comparative genomics strategy. First, the presence, occurrence, and conservation of nucleotide second messenger related gene products in available bacterial genomes of Acidithiobacillus spp. were assessed. Second, to circumscribe specific c-di-GMP control modules, the distribution of metabolism-effector-target genes on discrete genetic units, such as secondary replicons and mobile genetic elements (MGEs), was analyzed. Finally, to evaluate the interconnection of predicted control/effector/target gene modules, the sub-cellular localization of c-di-GMP related proteins and the concurrence of signal-sensing associated domains were examined.
Table 1. Occurrence and abundance of proteins predicted to be involved in synthesis and/or degradation of nucleotide second messengers in Acidithiobacillia.
Complete and draft genomes sequences (chromosomes and plasmids) for Acidithiobacillus spp. and Thermithiobacillus tepidarius DSM 3134 (outgroup) were downloaded from the NCBI repository as of June 2018 and filtered based on quality and completion criteria (Figure 1). All 35 chromosomal replicons retained in the analysis encode at least one 16S rRNA gene and are >90% complete; a set of plasmids (16 in total) was also included in the filtered data repository (Supplementary Table S1). Completeness was assessed as in Raes et al. (2007). Multiple sequence alignments of the protein families of interest to be used as queries in the identification of conserved domains in candidate protein sequences were downloaded from the following domain models/profiles databases: Pfam (Finn et al., 2016), COG (Tatusov et al., 2000), TIGRFAMs (Haft et al., 2013), SMART (Letunic and Bork, 2018) and clusterized to remove redundancy (see below). The full list of models/profiles used in this study can be found in Supplementary Table S2.
Figure 1. In silico analysis workflow. Overview of the datasets and general strategy used in the study. (A) Data collection and formatting. (B) Candidate protein identification. (C) Analysis and visualization of results. Symbols are shown in the bottom menu.
ORF prediction was done using GeneMarkS+ software with MetageneMark_v1mod model (Zhu et al., 2010) and functional assignment and curation was done using the workflow presented in Figure 1. Briefly, orthologs of the domain models/profiles of interest (see above) were identified in each genome using PSI-BLAST (with five iterations, e-value 0.01; Altschul et al., 1997) and candidate target sequence assignments were validated against the Conserved Domain Database v.3.16 (CDD, Marchler-Bauer et al., 2017) using CD-search (Marchler-Bauer and Bryant, 2004) and hhsearch (Fidler et al., 2016) with defaults parameters (e-value < 0.00001 for CD-search and hhsearch and probability > 90% for hhsearch). Identification of specific domains in selected proteins was done with RPS-BLAST v2.2.26 versus CDD database. Elimination of overlapping motifs and redundant results were done with rpsbproc v0.11.
Selected proteins were clustered by identity (threshold of 50% and defaults parameters) with Usearch v1.2.22 (Edgar, 2010). Protein alignments were constructed with the MAFFT v7.123 program (Katoh and Standley, 2013). Selected clusters and proteins were re-annotated and curated manually.
The FastTree program v2.1.7 (Price et al., 2010) was used for phylogenetic analysis (WAG evolutionary model for amino acid sequences analyses; GTR evolutionary model for nucleotide sequences analyses) with bootstrap resampling (1,000 replicates). FigTree v1.4.3 (Rambaut, 2009) was used for tree visualization and manipulation.
All candidate proteins were analyzed with additional resources, including SignalP v4.0 (Petersen et al., 2011) and Phobius v1.0 (Kall et al., 2004) for signal peptide prediction; TMhmm v2.0c (Krogh et al., 2001) for transmembrane helices predictions; Psortb v3.0.5 (Gardy et al., 2003) for protein subcellular localization prediction; LipoP v1.0 for lipoproteins prediction and discrimination of lipoprotein signal peptides (Juncker et al., 2003); Tatp v1.0 for Twin-arginine signal peptide cleavage sites identification (Bendtsen et al., 2005). All software was downloaded and run locally using default parameters for gram-negative bacteria. The theoretical pI (isoelectric point) of candidate proteins was computed through the Expasy server1.
Complete genomes (NZ_CP005986, NC_015850, NZ_CP026328, NC_015942, NZ_LT841305, NC_011761, NC_011206) were used for identification of putative MGEs using the following programs: AlienHunter (Vernikos and Parkhill, 2006), PAI-DA (Tu and Ding, 2003), IslandPath (Hsiao et al., 2005) for genomic island identification; PhiSpy (Akhter et al., 2012) and Phage Finder (Fouts, 2006) for prophage identification; TnpPred (Riadi et al., 2012) for prediction of prokaryotic transposases; CONJscan (Guglielmini et al., 2014) for Type IV secretion systems prediction; tRNAscan (Lowe and Eddy, 1997) and Aragorn (Laslett and Canback, 2004) for search tRNA and tmRNA. All predictions were analyzed and curated manually.
Protein and genome comparisons were performed with Blastp and Blastn respectively and visualized with Artemis Comparison Tool (ACT) v1.0 (Carver et al., 2005). Figures of gene contexts and neighborhood were performed with genoPlotR (Guy et al., 2010). Genome circular visualization was performed using circos (Krzywinski et al., 2009).
To reconstruct nucleotide-driven signal transduction pathways in polyextremophilic acidithiobacilli, we analyzed 51 replicons [35 chromosomes (7 closed and 28 at more than 90% completeness) and 16 plasmids] belonging to Acidithiobacillus species (Supplementary Table S1), comprising extreme (pH ≤ 3) to moderate (3 ≤ pH ≤ 5) acidophiles and psychrotolerant (growth at 4°C) to mesothermophiles (growth at 45°). The genomes were searched for hallmark sequences containing the signature protein domains related with the synthesis and degradation of nucleotide second messengers, as well as downstream effector proteins (Supplementary Table S2). Workflow details are summarized in Figure 1.
Synthesis functions were identified in all 35 chromosomes for every signaling nucleotide targeted, except c-di-AMP (Table 1). All genomes analyzed encode (p)ppGpp synthetases and nucleotidyl cyclases (NCs) with readily identifiable domains conferring to these enzymes specific biosynthetic activities, such as adenylyl cyclases (ACs) or diguanylate cyclases (DGCs) for the synthesis of cAMP and c-di-GMP, respectively (Table 1 and Supplementary Table S3). Consistently, hydrolases for degradation of the three nucleotides are also encoded in every Acidithiobacillus genome analyzed. Surprisingly, hydrolases for the degradation of c-di-AMP dinucleotide were also identified in all genomes, even if the synthesizing diadenylate cyclases (DACs) appeared to be absent. Globally, these results suggest that signaling nucleotides are relevant as second messenger molecules for bacteria thriving in acidic econiches, as they are in other environments. Each of the pathways uncovered is analyzed in further detail in the sections below and, when possible, the implications in acidithiobacilli ecophysiology are inferred.
We first assessed the presence, occurrence, and conservation of guanosine tetra and/or pentaphosphate synthesis and degradation functions in Acidithiobacillus spp. Without exception, every genome analyzed was found to encode a single copy of the large (p)ppGpp synthetases belonging to the RSH protein superfamily (RelA/SpoT Homolog), named after the discovery of RelA and SpoT proteins in Escherichia coli (Stent and Brenner, 1961; Cashel and Gallant, 1969). The acidithiobacilli’s long RSHs will be collectively referred to as SpoTAci onwards (see below).
Most γ- and β-proteobacteria bare two long RSH gene orthologs per genome (relA and spoT), arisen through a gene duplication event of an ancestral bifunctional “Rel” protein (Atkinson et al., 2011). In contrast, evaluated members of Acidithiobacillus genus only possess one long RSH (spoT) per genome. This is consistent with the recent re-classification of this acidophilic taxon as a new class (Acidithiobacillia), ancestral to both the γ- and β-proteobacteria (Williams and Kelly, 2013). Long RSHs are conformed by the synthesis [SYN (RelA_SpoT: pfam04607)] and hydrolysis [HYD (HD: pfam13328)] domains, generally escorted by accessory domains that regulate their activities.
The SYN domain is known to catalyze the synthesis of ppGpp and pppGpp by the addition of a pyrophosphate (Pi) group from ATP to the 3′ position of GDP and GTP, respectively. SpoTAci conserve the RXKD motif at the substrate-binding pocket of the predicted SYN domain (Supplementary Figure S1A) instead of the EXDD motif present in relA orthologs. This aspect suggests that the acidithiobacilli SpoTAci would prefer GTP over GDP as substrate and therefore it is predicted to mainly synthesize pppGpp over ppGpp (Sajish et al., 2009). Despite this, Acidithiobacillus species could still produce ppGpp linear messengers through enzymatic removal of one Pi from pppGpp. All Acidithiobacillus species analyzed encode the ppx gene (exopolyphosphatase), an ortholog of the gppA gene encoding for the pppGpp phosphohydrolase (Keasling et al., 1993). This is relevant since pppGpp and ppGpp bind to different target effector molecules and produce different output responses (Wang et al., 2007; Mechold et al., 2013). All SpoTAci also conserve the HDXXED motif at HYD domain needed for (p)ppGpp hydrolysis (Aravind and Koonin, 1998), and are therefore predicted as bifunctional.
In well-studied microbial models, synthesis of (p)ppGpp is activated by different environmental stresses, including temperature changes (e.g., English et al., 2011) and limitation of several nutrients such as: carbon sources (e.g., Xiao et al., 1991), phosphate (e.g., Spira et al., 1995), iron (e.g., Vinella et al., 2005), fatty acids (e.g., Battesti and Bouveret, 2006), and amino acids (e.g., Haseltine and Block, 1973). Synthesis and hydrolysis of long RSHs are controlled by additional domains that integrate these diverse signals. Identified SpoTAci accessory domains are TGS (ThrRS, GTPase, and SpoT), ZFD (zinc-finger domain), and RRM (RNA recognition motif) (HYD-SYN-TGS-ZFD-RRM configuration), which are widely conserved in the protein family. The presence and conservation of the TGS domain in SpoTAci suggests that its synthetase activity may be tiriggered by amino acid and/or fatty acid starvation (Battesti and Bouveret, 2006, 2009; Winther et al., 2018). Congruently, (p)ppGpp binds to many targets including amino acid decarboxylases (LdcI/CadA, LdcC, SpeF, SpeC, SlyA, PigR, PNPase), lipid metabolism protein (PlsB, PgsA, AccA, AccD, FabA, FabZ, GdhA, GlgC, Ppc) and nucleotide metabolism proteins (DnaG, MazG, GuaB, APT, Hpt, HisG) (Kanjee et al., 2012), most of which occur in the Acidithiobacillia genomes screened (data not shown).
Acidithiobacillia long RSH protein encoding genes occur in a highly conserved gene cluster, which resembles the spoT operon of E. coli both in structure and sequence (Figure 2). Conservation of gene context provides further clues of its functionality and expected physiological outputs. Genes immediately upstream of spoTAci are gmk and rpoZ. One of the best known (p)ppGpp mediated effects is its global control of gene expression, through direct binding to the RNA polymerase omega subunit (RpoZ). In this way (p)ppGpp simultaneously inhibits rRNA synthesis and the expression of ribosomal proteins (Paul et al., 2004), and activates transcription of amino acid biosynthesis genes (Paul et al., 2005). In the Acidithiobacillia, the RpoZ predicted proteins are well-conserved and possess the conserved MAR motif in its N-terminal for (p)ppGpp binding (Hauryliuk et al., 2015) (Supplementary Figure S1B). In turn, gmk, which codes for a guanylate (GMP) kinase, is a key player in de novo pathway for GTP synthesis catalyzing the formation of GDP from GMP (plus ATP) (Srivatsan and Wang, 2008). In other bacteria (e.g., in Firmicutes) this kinase is inhibited by (p)ppGpp promoting simultaneously the decrease of GTP levels and an accumulation of GMP, affecting the transcription of genes under GTP-dependent promotors (Kriel et al., 2012; Liu et al., 2015). The gmk gene occurs in just one copy per genome and its predicted protein product bares a highly conserved essential motif (K..S..RxxxR..Y..Y..E..Y..E..I..E) required for (p)ppGpp binding (Liu et al., 2015) (Supplementary Figure S1C). The co-occurrence of gmk and spoTAci in the same operon and amino acidic sequence level conservation of GMK (data not shown) suggests that in Acidithiobacillia GMK is also inhibited by (p)ppGpp. The decrease of intracellular GTP levels may have an impact on the synthesis of others nucleotide messengers based in GTP such as c-di-GMP.
Figure 2. Organization and conservation of the SpoTAci gene clusters in Acidithiobacillia reference genomes. YicC, uncharacterized stress-induced protein (COG1561); GMK, guanylate kinase (COG0194); RpoZ, RNA polymerase, omega subunit (COG1758); SpoT, bifunctional (p)ppGpp synthetase/guanosine-3′-5′-bis(diphosphate) 3′pyrophosphohydrolase (COG0317); RidA, enamine deaminase (COG0251); hyp (orf6), hypothetical protein with a hybrid cluster protein-associated redox disulfide domain (TIGR03980); RecG, ATP-dependent DNA helicase (pfam13749); YkuD, murein L,D-transpeptidase catalytic domain containing protein (pfam03734) in TTP, T. tepidarius; ACA, A. caldus; AFE, A. ferrooxidans; AFD, A. ferridurans; AFV, A. ferrivorans; AFP, A. ferriphilus; AAL, A. albertensis; ATH, A. thiooxidans. Percentage of amino acid similarity is depicted.
In some strains of A. thiooxidans (6 out of 10) and A. ferrivorans (2 out of 7) one copy of Small Alarmone Hydrolases (SAHs) containing just the HYD domain (Atkinson et al., 2011) were identified (Supplementary Figure S1A). Presently, there is no evidence of their functionality; yet as the SAHs of other microorganisms, it is likely that they play a role in fine-tuning sensitivity and speed of (p)ppGpp degradation (Atkinson et al., 2011).
A second highly conserved signal transduction pathway in Acidithiobacillia corresponds to the one driven by cyclic AMP (cAMP). cAMP is synthesized from ATP by cognate nucleotidyl cyclases (Supplementary Table S2). ACs are very diverse and have been grouped in six classes (I–VI), which share no sequence similarities (Bârzu and Danchin, 1994). Every Acidithiobacillus genome queried in this work only encodes a single putative AC belonging to class IV. No other type of ACs (classes I–III, V–VI) were detected in any of the species of the genus.
To date, only a few class IV ACs have been biochemically characterized. One of such proteins is CyaB from mesophilic bacteria Aeromonas (Ae.) hydrophila and Yersinia pestis (YpAC-IV), which are formed by a single CYTH domain (pfam01928) and exhibit thermophilic properties (Sismeiro et al., 1998; Smith et al., 2006). Residues linked to the active site of this reference protein (Iyer and Aravind, 2002; Gallagher et al., 2011) are well-conserved in the predicted CYTH domains from ACs found in the acidithiobacilli (ACAci) (Supplementary Figure S1D). In Ae. hydrophila biochemical conditions for maximal enzymatic activity of CyaB were found to be 65°C and pH 9.5, while a second AC CyaA (class I) performs optimally at 37–45°C and pH 8.5, suggesting that cAMP is synthetized under different in vivo situations by either one of the enzymes (Sismeiro et al., 1998). Despite its range of temperature (4–45°C) and pH (2.5–4.5) for growth, no other type of AC beside the class IV ACAci was detected in the Acidithiobacillia class, suggesting that the capacity to synthetize cAMP in response to different conditions depends specifically on a single AC protein.
Figure 3. cAMP-dependent signaling in the Acidithiobacillia class. (A) Organization of the ACAci operon and conservation of the protein coding genes. Percentage of amino-acid similarity is indicated. (B) Model of cAMP-dependent signaling in the acidithiobacilli. ATP serves as precursor in the synthesis of cAMP. Levels of ATP and PolyP are modulated by the activity of PPK and ACAci. A putative alternative reaction catalyzed by ACAci, the polyP degradation is indicated by a dashed arrow. cAMP synthetized by ACAci binds to cAMP receptor proteins (CRPs) and transduces the signal. Outer membrane (omp), periplasmic (mfp) and inner membrane (imp) subunits of the Resistance-Nodulation-Division (RND) transport; folD, methenyltetrahydrofolate cyclohydrolase FolD; ppk, polyphosphate kinase PPK1; parA, chromosome partitioning protein ParA.
Genomic evidence has shown that CYTH domains of many ACs co-occur in the same microbe, or in the same polypeptide, with few different domains (Iyer and Aravind, 2002). In the case of Acidithiobacillus ACAci, a “conserved histidine alpha-helical domain” (CHAD) is present at the proteins C-terminus (Figure 3A). CHAD domain is qualified as phosin given its capacity to bind polyphosphate (polyP) granules (Tumlirsch and Jendrossek, 2017). Therefore, the cyclase activity of ACAci is probably regulated by the presence of polyP. PolyP is a linear polymer of 1000s of orthophosphate residues linked by phosphoanhydride bonds, involved in diverse roles in both Prokaryotes and Eukaryotes. The main enzyme involved in the biosynthesis of polyP in Acidithiobacillus is the phosin PolyP kinase (PPK), catalyzing the reversible conversion of the terminal phosphate of ATP into polyP (Orell et al., 2012). The presence of ppk in the immediate vicinity of the ACAci encoding gene suggests a functional association between these two proteins. A direct connection could be established by the reverse activity of PPK providing the substrate ATP to ACAci for cAMP synthesis (Figure 3B). Besides, ACAci could represent an ancient partner of PPK in PolyP metabolism, since CYTH domain (named due to its presence in enzymes acting on triphosphorylated substrates, CyaB from Ae. hydrophila and the human Thiamine triphosphatase) has been proposed to work as phosphoesterase through a two-metal reaction mechanism, with the AC activity being a secondary reaction (Iyer and Aravind, 2002). The cognate enzyme that liberates inorganic phosphate (Pi) from polyP hydrolysis is a polyphosphatase (PPX) (Rao et al., 2009). In E. coli, both ppk and ppx genes are encoded in the same operon, while in Acidithiobacillus spp. ppx forms part of the pho operon (Navarro et al., 2013). In the acidithiobacillli, PolyP is a key source of Pi for metal detoxification, effecting and/or facilitating the formation of metal-phosphate and metal-sulfur complexes and their elimination (Alvarez and Jerez, 2004; Navarro et al., 2013; Ulloa et al., 2018). In this context, occurrence of a conserved RND family efflux pump in the gene neighborhood of ACAci suggests a possible implication of this pump in P-metal complexes elimination.
Another gene in the ACAci vicinity is folD (Figure 3A), which appears conserved in all Acidithiobacillus analyzed and several other gamma-proteobacteria (data not shown). This gene encodes a dual function enzyme (5,10-methylenetetrahydrofolate dehydrogenase and 5,10-methenyltetrahydrofolate cyclohydrolase) central to the folate (vitamin B9)-dependent C1 metabolism, highly conserved in all domains of life for its role in the biosynthesis of key biological building blocks (nucleic acids, amino acids, provitamines, etc.) (Shen et al., 1999). In this respect, the observed operon structure suggests that cAMP acts in the acidithiobacilli as a signal connecting the energetic status of the cell (ATP and polyP levels) with central metabolism and cell division functions to act coordinately.
The major known outcome of cAMP signaling is the regulation of gene expression by direct binding to the transcription regulator CRP (cAMP receptor protein) (Green et al., 2014). All Acidithiobacillus genomes analyzed encode for cAMP-receptor proteins (CRPs), with the typical domain architecture described for E. coli (Green et al., 2014), i.e., an N-terminal region harboring the cAMP-binding domain (cNMP_binding, pfam00027), and a C-terminal region with the DNA-binding domain (canonical helix-turn-helix motif). However, these proteins are diversified among Acidithiobacillia members, which encode from 1 to 9 crp orthologs per genome depending of the species [1 plasmid pTF53 (A. ferridurans ATCC 33020); 3 Acidithiobacilllus sp. SH; 5 T. tepidarius; 3-5 A. caldus; 4-6 A. ferrivorans, 4-7 A. thiooxidans; 2-7 A. ferrooxidans; 9 A. albertensis], being A. caldus and T. tepidarius CRPs the most divergent. Intriguingly, amino acidic sequence alignment of the Acidithiobacillia CRP orthologs showed that the residues involved in cAMP binding (Townsend et al., 2015) are not well-conserved [G71, E72, R82, S83, T127, S128 (Supplementary Figure S1E)] and substitutions are not conservative. This could indicate absence of function, low affinity for cAMP or divergent evolution to acquire novel functions. It is known that some variations of CRP have evolved to respond at high or low cAMP intracellular concentrations (Green et al., 2014), however further studies are required for the assignment of physiological function to acidithobacilli’s CRPs.
We next assessed the occurrence, diversity, and abundance of proteins predicted to be involved in cyclic diguanylate (c-di-GMP) dependent signaling in the targeted Acidithiobacillia genomes. This cyclic nucleotide is best known for its role as second messenger controlling the transition from motile planktonic state to the attached multicellular state achieved in biofilms (Hengge, 2009). Within biofilms, bacteria benefit from physical and physiological interactions that enhance nutrient availability, potentiate toxic metabolites removal and provide protection against a variety of environmental stresses. This response is thus particularly important in extreme acidophiles, such as the acidithiobacilli.
Figure 4. c-di-GMP-dependent pathways in the Acidithiobacillia class. (A) Frequency of c-di-GMP metabolism elements encoded in the genome of sequenced Acidithiobacillus. (B) Scheme of the signal transduction pathways) inferred from genomic information. Signals perceived by different sensor domains (SD) are transduced into the cell as differential levels of c-di-GMP through regulation of DGC and PDE activities residing on GGDEF, and EAL and HDGYP domains, respectively. Different c-di-GMP pools affect several cellular responses through diverse receptor protein families (purple). Protein domains are colored as follows: GGDEF (green), EAL and HDGYP (red), Oligoribonuclease Orn (orange).
The Acidithiobacillus species analyzed encode in their genomes a vast diversity of genes linked to c-di-GMP metabolism (total 754), including opposing diguanylate cyclase (DGCs) and phosphodiesterase (PDEs) activities (Figure 4 and Table 2). The DGC activity resides in the GGDEF protein domain (pfam00990), while PDE activity resides in two unrelated domains: EAL (pfam00563) and HD-GYP (COG2206). Globally, Acidithiobacillia members show a higher proportion of synthesis versus degradation elements, which agrees with reported trends in other microorganisms (Seshasayee et al., 2010; Römling et al., 2013). As it occurs in other microorganisms, both synthesis and degradation functions in the acidithiobacilli take place in different configurations: GGDEF-only (206 in total), GGDEF-EAL combined (387 in total), EAL-only (122 in total), and HD-GYP-only (39 in total). Notably, HD-GYP proteins are present in all species except in the moderate thermophilic bacteria A. caldus (Figures 4A, 5C).
C-di-GMP metabolism proteins bare different subcellular localization signals that partition them differentially at inner or outer membrane, periplasmic space or even secreted out of the cell. The outer membrane localization signals are mostly represented in synthesis-related proteins (29% in GGDEF-EAL and 17% in GGDEF-only) rather than degradation related proteins (2% in EAL-only and 0% in HD-GYP) (Table 2). Twenty five percent of all Acidithiobacillus GGDEF-EAL proteins have predicted transmembrane regions. Besides, some species present GGDEF-EAL proteins with signals for translocation through internal membrane, which may reach the periplasm remaining able (or not) to be secreted through outer membrane by the type 2 secretion system (T2SS) protein complex (Cianciotto, 2005). Two subgroups can be distinguished, a GCS-GAF-GGDEF-EAL with tat signal present in A. caldus, and a few GGDEF-EAL proteins with sec signal present in A. albertensis, A. ferrivorans, and A. thiooxidans. The last two bacteria also present GGDEF-only proteins with localization signals.
Figure 5. Phylogenetic tree of DGCs and PDEs proteins identified in the acidithiobacilli. (A) GGDEF DGCs, (B) EAL, and (C) HDGYP PDEs. Sensor domains are represented graphically next to the corresponding tree branches. Proteins are colored by taxonomy as follows: TTP, Thermithiobacillus tepidarius (pink); ACA, Acidithiobacillus caldus (green); ATH, Acidithiobacillus thiooxidans (purple); AAL, Acidithiobacillus albertensis (yellow); AFV, Acidithiobacillus ferrivorans (blue); AFE, Acidithiobacillus ferrooxidans (light blue).
Most GGDEF-EAL proteins (65%) and nearly half of the GGDEF-only proteins (42%) contain partner domains (Table 2). Most common partner domains found to concur with c-di-GMP metabolism proteins in Acidithiobacillia are shown in Figures 4B, 5A–C including: PAS (Period circadian protein, Aryl hydrocarbon receptor nuclear translocator protein and Single-minded protein), GAF (cGMP specific phosphodiesterases, Adenylyl cyclases and FhlA), REC (response regulator receiver domain), Protoglobin [or globin coupled sensor (GCS)], and CZB (Chemoreceptor Zn-Binding domain). Some of these domains are very well-characterized and thus their role in signal transduction can be robustly inferred. For example, the REC domain is typically present in the receiver element (or response regulator) of two-component signal transduction systems (Zschiedrich et al., 2016) and undergoes distinctive conformational changes upon phosphorylation (e.g., Lee et al., 2001). Both A. ferrivorans SS3 and A. caldus ATCC 51756T encode in their genomes a REC-GGDEF-EAL type protein and this gene is preceded by a histidine kinase encoding gene, in a configuration that is characteristic of well-known two-component systems. In the case of A. caldus, this two-component system is genetically linked to other genes involved in the SOS response, suggesting that this nucleotide dependent signal transduction pathway may trigger a collective behavior such as biofilm formation in response to DNA damaging conditions, as recently demonstrated in Pseudomonas aeruginosa (Weitao, 2011). On the other hand, the roles of other sensor domains are not so easy to relate to particular input signals (and/or output responses). This is the case of the small molecule-sensing domains, PAS and GAF, were significant sequence variability has been reported to occur (Zoraghi et al., 2004; Gilles-González and González, 2005). Just a few c-di-GMP metabolism proteins containing GAF domain have been characterized so far. Lcd1 (GAF-GGDEF) protein from Leptospira interrogans increase its DGC activity upon cAMP binding to its GAF domain (da Costa Vasconcelos et al., 2017). Recently, it was demonstrated that a bifunctional enzyme (GAF-GGDEF-EAL) from Mycobacterium smegmatis binds GDP at GAF domain, increasing PDE activity and decreasing DGC activity, which causes a quick decrease of intracellular levels of c-di-GMP (Chen et al., 2018). Thus, the GAF domain may establish a functional link between small nucleotides and c-di-GMP signaling. PAS and GAF domains share a similar structural fold, but are distantly related (Aravind and Ponting, 1997; Ho et al., 2000). Using diverse prosthetic groups, such FAD, heme and biliverdin, PAS domains bind different signals such as redox potential (Qi et al., 2009), O2 (Chang et al., 2001; Tuckerman et al., 2009), and light (Tarutina et al., 2006). To elucidate which signals may be transduced by PAS domains found in Acidithiobacillia acidophiles, we compared the DGCs derived PAS domains with experimentally validated representatives from other bacterial species. Most Acidithiobacillus DGCs and PDEs PAS-domains grouped with molecular oxygen and redox potential sensing referenced domains (Supplementary Figure S1F). This seems relevant in the context of the oxygen, redox and energetic requirements of the acidithiobacilli (Nitschke and Bonnefoy, 2016).
Together, these numbers suggest that Acidithiobacillus spp. are able to transduce several signals, originated both extra-and intra-cellularly, in c-di-GMP synthesis, while its degradation may be regulated in a different way. Acidithiobacillus PDEs are less likely to be regulated by externals cues given the low diversity of accessory domains identified. Near 70% of the EAL proteins containing accessory domains correspond to HD-related output domain (HDOD; EAL-HDOD configuration) (Figure 5B), which has been involved in the indirect activation of the transcription of chemotaxis genes (Cha et al., 2019). On the other hand, 100% of HDGYP proteins containing accessory domains correspond to the receiver element of two-component signal transduction systems [REC-HDGYP configuration (59% of total HD-GYP proteins)]. In these cases, the output of the two-component system may be the degradation of c-di-GMP. The rest of EAL and HDGYP PDEs are probably regulated at transcriptional level.
The abundance and diversity of c-di-GMP metabolism enzymes is thought to reflect the extent and complexity of the c-di-GMP dependent network in any given microorganism. In this respect, we observed important differences between Acidithiobacillus species (Figure 4A and Supplementary Table S3), in both total and relative numbers of c-di-GMP metabolism elements (DGCs and/or PDEs): 34-29 for A. thiooxidans > 29 for A. albertensis and T. tepidarius > 22-16 for A. caldus > 28-10 for A. ferrivorans > 8-4 for A. ferrooxidans. As a whole, these numbers show no correlation with the genome size of each strain analyzed (data not shown). The highest net number of DGCs/PDEs observed occurred in A. thiooxidans GD1-3, with a total of 34 c-di-GMP metabolism elements predicted. In turn, the lowest number occurred in A. ferrooxidans strains. The type of strains of the A. ferrooxidans, ATCC 23270T and strain ATCC 53993, had only four predicted c-di-GMP metabolism proteins, the lowest number in the whole Acidithiobacillia class, potentially representing the minimal essential set of DGCs/PDEs for the taxon. Yet, only one of them is conserved through the Acidithiobacillia class members (AFE_1712, Protoglobin-GGDEF-EAL-CZB), and two other across A. ferrivorans strains (AFE_3057, GAF-PAS-GGDEF-EAL, and AFE_1725, PAS-GGDEF-EAL-CZB). The fourth gene (AFE_1707, GAF-PAS-PAS-GAF-GGDEF-EAL) is shared only with A. thiooxidans. This distribution suggests that DGCs/PDEs shared with specific groups may have been gained in informative ecological contexts (e.g., through acquisition of gene islands) that may provide clues on the specific effectors targeted by these and/or other specific control proteins.
The larger the number of transducing components, the larger the range of potential signals that may be integrated and the number of cellular functions that may be expected to be targeted. For instance, host-associated bacteria have few c-di-GMP metabolism proteins while free-living organisms as a general rule tend to have more (Seshasayee et al., 2010). In this respect it is interesting to understand why A. ferrooxidans, one of the more versatile representatives of the taxon [capable of aerobic growth using ferrous iron, several different RISCS and hydrogen as electron donor and facultative ferric iron reduction also in the absence of oxygen (Quatrini and Johnson, 2018)], has the fewest. This observation stands for both culture collection and recent isolates of the species. Alternative regulation pathways (that partially replace regulatory function of c-di-GMP) could be part of the explanation. It has been demonstrated that A. ferrooxidans possesses a functional Quorum Sensing type AI-1 system (Farah et al., 2005), which is absent in other Acidithiobacillus (Valdés et al., 2008; this study, data not shown). Yet, additional underlying reasons require further investigation of the nature of the control modules, the number and diversity of targeted effectors and the interactions established by the elements of this system, both of which are quite challenging tasks.
The c-di-GMP second messenger has been proven to bind a wide diversity of effector proteins triggering a plethora of different specific physiological and behavioral responses (Chou and Galperin, 2016). This diversity is also represented in c-di-GMP effectors predicted in the genomes of sequenced Acidithiobacillia representatives (Supplementary Table S3). These include PilZ domains, PelD and FleQ proteins, and other less characterized proteins such as CckA, MshE, CX_3703, BdcA, BldD, VpsT, BcsE, BCAM1349, Clp, BrlR, LtmA, VpsR, and PnpA (not shown).
Figure 6. Diversity of Acidithiobacillia PilZ proteins. (A) Phylogenetic tree showing the six principal groups of PilZ proteins found in the acidithiobacilli. (B) Conservation of signature motifs for c-di-GMP binding in PilZ protein groups.
Among these, the PilZ protein domain is considered to be the universal c-di-GMP-binding adaptor and experimental demonstrations of this fact abound in the literature (Ryjenkov et al., 2006; Boehm et al., 2010; Fang and Gomelsky, 2010; Paul et al., 2010; Wilksch et al., 2011). Every Acidithiobacillus genome analyzed encodes PilZ proteins. In agreement with the low number of c-di-GMP metabolism proteins, A. ferrooxidans strains showed the fewest amount of PilZ domains too. As occurs in other bacteria, the PilZ domain exists alone or in conjunction with other domains in the same polypeptide chain in the acidithiobacilli predicted proteins, suggesting that binding of c-di-GMP can trigger different responses in this acidophilic taxon as well. A phylogenetic tree of the PilZ family proteins identified in the acidithiobacilli shows six large protein groups clearly differentiated by their domain configuration (Figure 6A). Most of PilZ proteins of Acidithiobacillus (65.4%) are predicted to contain just the PilZ domain, however, they could be distributed at least in three different sub-groups (groups 1, 2, and 5), which differ in their ability to bind c-di-GMP due the presence/absence of two sequence motifs: RxxxR and [N/D]xSxxG (Figure 6B and Supplementary Figure S1G). These motifs are separated by ∼20 amino acid residues, and each one mediates the protein interaction with a guanine base of the cyclic di-nucleotide molecule (Benach et al., 2007; Ko et al., 2010; Habazettl et al., 2011). PilZ-only proteins are generally related with Type IV Pili (T4P) formation and twitching motility, which has been implicated in irreversible attachment to surfaces, microcolony grouping and structural development of biofilm (O’Toole and Kolter, 1998 Semmler et al., 1999). PilZ proteins of Group 3 consist in long proteins (∼1000 residues) that coupled PilZ domain with uncharacterized domain DUF390, and they are present only in A. caldus strains. Group 4 are conformed by proteins containing Cellulose_synt domain (pfam03552), which are homologs to bacterial cellulose synthase A subunit (BcsA), and therefore may synthetize cellulose or some other glucose-based exopolysacharrides needed for attachment and extracellular matrix construction. In Komagataeibacter xylinus, cellulose synthesis depends on the presence of the cellulose synthase complex (Saxena et al., 1994), which binds c-di-GMP directly (Weinhouse et al., 1997) and increases 200 times its basal activity (Ross et al., 1985). Finally, group 6 contain proteins with an YcgR domain, known to down regulate flagellar motility in response to c-di-GMP binding (Fang and Gomelsky, 2010; Paul et al., 2010).
Another class of c-di-GMP effector protein was widely found in Acidithiobacillia. FleQ is a protein composed of three domains (FleQ-AAA-HTH) involved in the transcriptional regulation of flagella and EPS production. In P. aeruginosa, the binding of c-di-GMP in the AAA domain of FleQ determines a slight decrease in the transcription of flagellar genes, but simultaneously causes a great increase in the transcription of the pelABCDEFG operon required for the synthesis of PEL exopolysaccharide and the formation of biofilms (Hickman and Harwood, 2008). Some of FleQ proteins of Acidithiobacillus do not possess the FleQ domain, however, they present most of the key amino acids for the binding of c-di-GMP in their AAA domains (Supplementary Figure S1H).
The pel-like operon was also found in Acidithiobacillus strains. While bacterial cellulose synthase machinery is present in both sulfur and sulfur/iron oxidizer species, a complete pel-like operon is only present in A. caldus, A. thiooxidans, and Acidithiobacillus sp. SH (Supplementary Table S3), corroborating its presence only in sulfur-oxidizing species (Castro et al., 2015; Díaz et al., 2018). This operon encodes the c-di-GMP receptor protein PelD, which contains the key amino acid for c-di-GMP binding (Supplementary Figure S1I). By comparing wild type and ΔpelD null-mutant strains, Díaz et al. (2018) have recently reported that PelD and c-di-GMP pathway are involved in biofilm formation and architecture in A. thioooxidans. This is likely also true for A. caldus, and Acidithiobacillus sp. SH. The absence of the pel-like operon in A. albertensis is intriguing due to its close phylogenetic relationship with A. thiooxidans (Castro et al., 2017).
In addition to the typical effector proteins, catalytically degenerate and inactive GGDEF and EAL domains have been shown to act as effector proteins by allosteric binding of c-di-GMP (Beyhan et al., 2008; Abel et al., 2011; Newell et al., 2011; Qi et al., 2011). Candidate effector proteins were also identified in the Acidithiobacillia class among the predicted DGCs and PDEs, but their function as such awaits experimental validation.
Figure 7. Acidithiobacillus mobile genetic elements (MGEs) containing c-di-GMP related proteins. (A) MGEs present in A. ferrooxidans ATCC 23270T. Genomes order: protein predictions in AFE ATCC 23270, AFE Wenelen, AFE DLC-5, AFE GGI-221, AFE 53993, AFE HEL-18, AFE YQH-1, MGE predictions. (B) MGEs present in A. caldus ATCC 51756T. Genomes order: protein predictions in ACA ATCC 51756, ACA SM-1, ACA MTH-04, ACA DX, ACA S1, ACA ZBY, ACA ZJ. MGE predictions. C-di-GMP related protein domain configurations are detailed.
Inside the cell, effector molecules interact with cognate target components to produce a variety of output phenotypes. However, given the multiplicity of c-di-GMP related genes and their scattered distribution within most bacterial genomes, the elements that belong to the same c-di-GMP control module are difficult to uncover. Furthermore, since many of the predicted protein products with GGDEF/EAL domains pertain to the flexible genome of a given species [e.g., 40% of the DGCs/PDEs of A. albertensisT are exclusive to this strain (Castro et al., 2017)], concurrencies between cyclases, esterases, effectors and targets are blurry. Previous work from our group and others has shown that the Acidithiobacillus spp. harbor different types of MGEs including prophages (Tapia et al., 2012; Covarrubias et al., 2018), plasmids (reviewed in Quatrini et al., 2016) and integrative conjugative elements (ICEs) (Bustamante et al., 2012; Acuña et al., 2013). Albeit a few reports on the occurrence of c-di-GMP pathway modules on MGEs exist (Bordeleau et al., 2010; Madsen et al., 2018), bioinformatic analyses of several Acidithiobacillus genomes point out the frequent occurrence of c-di-GMP related elements in this taxon’s mobilome.
Figure 8. Working model of relationship between (p)ppGpp, cAMP and c-di-GMP based second messenger signaling in Acidithiobacillus. SpoTAci, bifunctional (p)ppGpp synthetase/guanosine-3′-5′-bis(diphosphate) 3′pyrophos′hohydrolase; ACAci, adenylyl cyclase; Ndk, nucleoside diphosphate kinase; Gmk, GMP kinase; GGDEF, DGC domain; EAL and HDGYP, PDE domains; Orn, oligoribonuclease.
Considering that MGEs behave as cohesive heritable units and that genes present on a particular element or replicon (in the case of plasmids) are likely to have co-evolved to accomplish a common function, we decided to inspect this aspect in detail as a strategy to uncover potential c-di-GMP responsive control modules. Using genome information and comparative genomics tools, we mapped c-di-GMP related elements (DGC, PDE, c-di-GMP effector proteins) to the core and flexible/mobile genome of five completely sequenced Acidithiobacillia members: A. caldus ATCC 51756T, A. caldus SM-1, A ferrooxidans ATCC 23270T, A. ferrooxidans ATCC53993, and A. ferrivorans SS3. For every candidate gene identified, systematic analyses of the genomic context were performed in order to derive probable target components through functional association analysis. Results obtained are summarized in Table 3. All five microorganisms analyzed harbored at least one integrated MGE and/or a plasmid baring one or more signature proteins for c-di-GMP metabolism. In most of these elements, one or more potential targets could be pinpointed (Table 3). Concurrence of c-di-GMP synthesis/degradation genes, effectors genes and either biofilm- or conjugation-related genes was observed in four of out five genomes alluded.
In the case of A. ferrooxidans ATCC 23270 (Figure 7A), both previously identified ICE elements ICEAfe1 (Bustamante et al., 2012) and ICEAfe2 (Bustamante et al., 2014) code for DGCs and/or PDEs. One of these elements, ICEAfe2, which encodes the carboxysome protein complex and the RuBisCo biphosphate carboxylase enzyme, both relevant for CO2 fixation, is partially conserved in A. ferrooxidans ATCC 53993. This element also bares three GGDEF-EAL proteins with accessory domains of the GAF, PAS, and GCS type in the vicinity of a PilZ-type effector proteins and trb-type conjugative genes, suggesting a connection between these c-di-GMP dependent signal transduction pathways and conjugation. In A. caldus ATCC 51756T (Figure 7B) the ICEAcaTY.2, a 180 kb MGE (Acuña et al., 2013), comprises a predicted bifunctional DGC/PDE (Aca1879) and the c-di-GMP receptors/effector proteins PilZ (Aca1908), FleQ (Aca1947) and YcgR-like (un-annotated gene between Aca1939 and Aca1940). In the immediate context of pilZ, chemotaxis and flagellar motility related functions were identified. Also, in the vicinity of the flagellar biosynthesis genes, we identified the Aca1947 gene, whose genetic product share is 69% of similarity with FleQ, a well-characterized transcriptional activator of the flagellar apparatus gene operon in other microorganisms (Arora et al., 1997). The third predicted effector protein identified in the A. caldus ICEAcaTY.2, YcgR-like, shows 36% of similarity with flagellar brake protein YcgR. In enterobacteria the YcgR protein affects negatively both swimming and swarming in a c-di-GMP dependent manner (Ryjenkov et al., 2006; Christen et al., 2007). An equivalent or related function may be proposed for the ycgR-like gene present in ICEAcaTY.2, although demonstration of this function remains a challenge. Besides, a region rich in predicted insertion sequences (IS) was identified in the A. caldus ATCC 51756T chromosome (and conserved in a SM1), which contains five predicted c-di-GMP elements (3 GGDEF, 1 inactive GGDEF and 1 EAL). One of the GGDEF proteins encoded by genes present in this c-di-GMP “hot spot” (designated ISR7, for IS-rich region), Aca1319 (formerly Aca1413), has already been experimentally characterized (Castro et al., 2015). This protein showed to be a functional DGC, responsible for the synthesis of most c-di-GMP produced by A. caldus when grown under standard conditions with elemental sulfur as energy source. Moreover, its gene has been mutated (ΔAca1319), being one of the few Acidithiobacillus mutants obtained world-wide (Castro et al., 2015). In comparison with the wild type strain, this mutant presented delayed attachment to a solid substrate in early stages of mineral colonization, and also exacerbated swarming motility levels. Also, putative c-di-GMP control modules were identified in two out of three A. caldus ATCC 51756T plasmids, including a pilZ ortholog occurring next to the cellulose synthetase in the mpAca1 megaplasmid. These results point to an important contribution of the mobile genetic complement in nucleotide signal transduction in these acidophiles.
Several of the c-di-GMP metabolism proteins identified in this study are candidate integral membrane proteins, others are predicted to be targeted to the outer membrane and yet others are presumably secreted into extracellular media (Table 2). Most proteins located in the extracellular compartments were GGDEF (17%) and GGDEF-EAL combined proteins (29%) and these occurred invariably in all Acidithiobacillus species analyzed. Such a finding raises the possibility that c-di-GMP may act as an extracellular signal linking perception of specific environmental or populational cues, to specific alterations in cellular function. In other bacteria, extracellular c-di-GMP has been shown to inhibit adherence of Streptococcus mutans to tooth surfaces (Yan et al., 2010), while treatment of Staphylococcus aureus with external c-di-GMP inhibited cell adhesion in liquid medium, and adherence to human epithelial cells (Karaolis et al., 2005). Certainly, harsh conditions imposed by an acidic milieu could prevent extracellular communication through nucleotides. However, their stability at acidic condition may be robust enough for message delivery; c-di-GMP shows resistance to boiling and to extreme acid exposition (Karaolis et al., 2005). Cyclic AMP and c-di-AMP are good candidates for this kind of signaling, since they also are stable under low pH (and high temperature). The half-life of cAMP is close to 30 min at 100°C in 1N HCl (Revankar and Robins, 1982), while c-di-AMP shows slow degradation into different intermediaries at 90°C and pH below 3 (Ora et al., 2012).
Bioinformatic analysis performed in this study failed to identify proteins carrying the domains required for c-di-AMP synthesis (DisA_N, pfam02457; Witte et al., 2008; Bai et al., 2012; Corrigan and Gründling, 2013) in the Acidithiobacillia, but hallmark proteins responsible for c-di-AMP hydrolysis (DHH domains, pfam01368; Rao et al., 2010) and candidate effectors proteins (KtrA, KdpD) are present in most genomes analyzed (Supplementary Figure 1J). Interestingly, other acidophilic bacteria (e.g., Leptospirillum and Sulfobacillus among others) do possess all genes required to construct a functional c-di-AMP signaling pathway, including synthesis, degradation and effectors proteins (data not shown). Therefore, evidence gathered in this work suggests that extracellular signal transduction pathways relying on cyclic nucleotidic second messengers may indeed exist in acidic econiches and raises the intriguing possibility that these nucleotides may mediate both intra- and inter-species communication. The existence and role of these interaction merits further exploration.
This study is the first comprehensive genomic analysis of nucleotide second messengers-based signaling pathways in polyextremophilic bacteria belonging to the Acidithiobacillia class. Our results showed that these acidophiles conserve at least three signaling mechanisms based on (p)ppGpp, cAMP, and c-di-GMP. As in other bacteria, in Acidithiobacillus the (p)ppGpp synthesis through SpoTACI is predicted to respond to nutrient limitation, reprograming global gene expression through direct binding to RpoZ RNA polymerase subunit. Cyclic AMP nucleotide synthesis in the acidithiobacilli seems to be limited by ATP availability, yet functional association-based inference suggests it could also be regulated by the presence of polyP. To our knowledge, this link has not been pointed out nor explored previously in the acidithiobacilli. The most versatile and diverse signaling pathway uncovered in the Acidithiobacillia was the one relying on c-di-GMP. On the basis of diverse partner sensor, synthesis and degradation domains, this pathway is predicted to transduce environmental cues (such as redox potential and oxygen levels) as well as intracellular signals (such as phosphorylation cascades), into differential levels of intracellular c-di-GMP. These, in turn, are predicted to regulate flagellar based motility, substrate attachment, and subsequent biofilm development through binding to diverse effector proteins included PilZ domain, FleQ and PelD proteins encoded in the gene vicinity of the DGCs and/or PDEs or confined to specific MGEs. All the (p)ppGpp, cAMP, and c-di-GMP second messenger systems, may cross-talk with each other through different components of the respective pathways (Figure 8). According to this working model, (p)ppGpp is predicted to have a direct impact on GDP and GTP synthesis, through inhibition of GMK activity. In turn, GTP, GDP, and cAMP levels feed in the c-di-GMP pathway principally through DGCs, while PDEs recycle c-di-GMP to restore nucleotide pools. While GTP is the substrate for c-di-GMP synthesis, GDP and cAMP molecules could be sensed by GGDEF proteins containing GAF domains, regulating its DGC activity.
Protein alignments of Supplementary Figure S1 can be found in Figshare, https://figshare.com/s/63b7234a67db25ff7a8b; doi: 10.6084/m9.figshare.7553921.
MC and RQ conceived and supervised the study and metabolic reconstruction analysis. AM-B and CR-V carried out the sequence processing and bioinformatic analyses. NG and MD contributed in data analysis. MC, AM-B, and RQ analyzed and interpreted the data and wrote the manucript. All authors read and approved the final manuscript.
This work was supported by grants from the Comisión Nacional de Ciencia y Tecnología: FONDECYT 314003 to MC, FONDECYT 1181251 to RQ, FONDECYT 1160702 to NG, Programa de Apoyo a Centros con Financiamiento Basal AFB 170004 to RQ, CONICYT-PFCHA/Doctorado Nacional/21171049 to AM-B) and by Millennium Science Initiative, Ministry of Economy, Development and Tourism of Chile (under Grant “Millennium Nucleus in the Biology of the Intestinal Microbiota” to RQ, MC, AM-B, and CR-V).
The authors declare that the research was conducted in the absence of any commercial or financial relationships that could be construed as a potential conflict of interest.
The Supplementary Material for this article can be found online at: https://www.frontiersin.org/articles/10.3389/fmicb.2019.00381/full#supplementary-material
FIGURE S1 | Supporting alignments for protein domains in nucleotide-driven signal transduction pathways of the acidithiobacilli. (A) SpoT; (B) RpoZ; (C) GMK; (D) AC; (E) CRP; (F) PAS; (G) PliZ; (H) FleQ; (I) PelD; and (J) DHH. Figshare, https://figshare.com/s/63b7234a67db25ff7a8b; doi: 10.6084/m9.figshare.7553921.
TABLE S1 | Acidithiobacillia class genomes analyzed in this study.
TABLE S2 | Hallmark genes or profile list used in the analysis.
TABLE S3 | Abundance of proteins predicted to be involved in nucleotide second messenger metabolism in different replicons from Acidithiobacillus.
Abel, S., Chien, P., Wassmann, P., Schirmer, T., Kaever, V., and Laub, M. T. (2011). Regulatory cohesion of cell cycle and cell differentiation through interlinked phosphorylation and second messenger networks. Mol. Cell 43, 550–560. doi: 10.1016/j.molcel.2011.07.018
Acuña, L. G., Cárdenas, J. P., Covarrubias, P. C., Haristoy, J. J., Flores, R., Nuñez, H., et al. (2013). Architecture and gene repertoire of the flexible genome of the extreme acidophile Acidithiobacillus caldus. PLoS One 8:e78237. doi: 10.1371/journal.pone.0078237
Akhter, S., Ramy, K., and Edwards, R. (2012). PhiSpy: a novel algorithm for finding prophages in bacterial genomes that combines similarity and composition-based strategies. Nucleic Acids Res. 40:e126. doi: 10.1093/nar/gks406
Altschul, S. F., Madden, T. L., Schäffer, A. A., Zhang, J., Zhang, Z., Miller, W., et al. (1997). Gapped blast and PSI-BLAST: a new generation of protein database search programs. Nucleic Acids Res. 25, 3389–3402. doi: 10.1093/nar/25.17.3389
Alvarez, S., and Jerez, C. A. (2004). Copper ions stimulate polyphosphate degradation and phosphate efflux in Acidithiobacillus ferrooxidans. Appl. Environ. Microbiol. 70, 5177–5182. doi: 10.1128/AEM.70.9.5177-5182.2004
Aravind, L., and Koonin, E. V. (1998). The HD domain defines a new superfamily of metal-dependent phosphohydrolases. Trends Biochem. Sci. 1998, 469–472. doi: 10.1016/S0968-0004(98)01293-6
Aravind, L., and Ponting, C. P. (1997). The GAF domain: an evolutionary link between diverse phototransducing proteins. Trends Biochem. Sci. 22, 458–459. doi: 10.1016/S0968-0004(97)01148-1
Arora, S. K., Ritchings, B. W., Almira, E. C., Lory, S., and Ramphal, R. (1997). A transcriptional activator, FleQ, regulates mucin adhesion and flagellar gene expression in Pseudomonas aeruginosa in a cascade manner. J. Bacteriol. 179, 5574–5581. doi: 10.1128/jb.179.17.5574-5581.1997
Atkinson, G. C., Tenson, T., and Hauryliuk, V. (2011). The RelA/SpoT homolog (RSH) superfamily: distribution and functional evolution of ppGpp synthetases and hydrolases across the tree of life. PLoS One 6:e23479. doi: 10.1371/journal.pone.0023479
Bai, Y., Yang, J., Zhou, X., Ding, X., Eisele, L. E., and Bai, G. (2012). Mycobacterium tuberculosis Rv3586 (DacA) is a diadenylate cyclase that converts ATP or ADP into c-di-AMP. PLoS One 7:e35206. doi: 10.1371/journal.pone.0035206
Bârzu, O., and Danchin, A. (1994). Adenylyl cyclases: a heterogeneous class of ATP-utilizing enzymes. Prog. Nucleic Acid Res. Mol. Biol. 49, 241–283. doi: 10.1016/S0079-6603(08)60052-5
Battesti, A., and Bouveret, E. (2006). Acyl carrier protein/SpoT interaction, the switch linking SpoT-dependent stress response to fatty acid metabolism. Mol. Microbiol. 62, 1048–1063. doi: 10.1111/j.1365-2958.2006.05442.x
Battesti, A., and Bouveret, E. (2009). Bacteria possessing two RelA/SpoT-like proteins have evolved a specific stringent response involving the acyl carrier protein-SpoT interaction. J. Bacteriol. 191, 616–624. doi: 10.1128/JB.01195-08
Benach, J., Swaminathan, S. S., Tamayo, R., Handelman, S. K., Folta-Stogniew, E., Ramos, J. E., et al. (2007). The structural basis of cyclic diguanylate signal transduction by PilZ domains. EMBO J. 26, 5153–5166. doi: 10.1038/sj.emboj.7601918
Bendtsen, J. D., Nielsen, H., Widdick, D., Palmer, T., and Brunak, S. (2005). Prediction of twin-arginine signal peptides. BMC Bioinformatics 6:167. doi: 10.1186/1471-2105-6-167
Beyhan, S., Odell, L. S., and Yildiz, F. H. (2008). Identification and characterization of cyclic diguanylate signaling systems controlling rugosity in Vibrio cholerae. J Bacteriol. 190, 7392–7405. doi: 10.1128/JB.00564-08
Boehm, A., Kaiser, M., Li, H., Spangler, C., Kasper, C. A., Ackermann, M., et al. (2010). Second messenger-mediated adjustment of bacterial swimming velocity. Cell 141, 107–116. doi: 10.1016/j.cell.2010.01.018
Bordeleau, E., Brouillette, E., Robichaud, N., and Burrus, V. (2010). Beyond antibiotic resistance: integrating conjugative elements of the SXT/R391 family that encode novel diguanylate cyclases participate to c-di-GMP signaling in Vibrio cholerae. Environ. Microbiol. 12, 510–523. doi: 10.1111/j.1462-2920.2009.02094.x
Bryant, R. D., McGroarty, K. M., Costerton, J. W., and Laishley, E. J. (1983). Isolation and characterization of a new acidophilic Thiobacillus species (T. albertis). J. Microbiol. 29, 1159–1170. doi: 10.1139/m83-178
Bustamante, P., Covarrubias, P., Levican, G., Katz, A., Tapia, P., Bonnefoy, V., et al. (2012). ICEAfe1, an actively excising integrativeconjugative element from the biomining bacterium Acidithiobacillus ferrooxidans. J. Mol. Microbiol. Biotech. 22, 399–407. doi: 10.1159/000346669
Bustamante, P., Tello, M., and Orellana, O. (2014). Toxin-antitoxin systems in the mobile genome of Acidithiobacillus ferrooxidans. PLoS One 9:e112226. doi: 10.1371/journal.pone.0112226
Carver, T. J., Rutherford, K. M., Berriman, M., Rajandream, M. A., Barrell, B. G., and Parkhill, J. (2005). ACT: the artemis comparison tool. Bioinformatics 16, 3422–3423. doi: 10.1093/bioinformatics/bti553
Cashel, M., and Gallant, J. (1969). Two compounds implicated in the function of the RC gene of Escherichia coli. Nature 221, 838–841. doi: 10.1038/221838a0
Castro, M., Deane, S., Ruiz, L., Rawlings, D. E., and Guiliani, N. (2015). Diguanylate cyclase null mutant reveals that c-di-GMP pathway regulates the motility and adherence of the extremophile bacterium Acidithiobacillus caldus. PLoS One 10:e0116399. doi: 10.1371/journal.pone.0116399
Castro, M., Moya-Beltrán, A., Covarrubias, P. C., Gonzalez, M., Cardenas, J. P., Issotta, F., et al. (2017). Draft genome sequence of the type strain of the sulfur-oxidizing acidophile, Acidithiobacillus albertensis (DSM 14366). Stand. Genomic Sci. 12:77. doi: 10.1186/s40793-017-0282-y
Cha, G., Chen, Z., Mo, R., Lu, G., and Gao, B. (2019). The novel regulators CheP and CheQ control the core chemotaxis operon cheVAW in Campylobacter jejuni. Mol. Microbiol. 111, 145–158. doi: 10.1111/mmi.14144
Chang, A. L., Tuckerman, J. R., Gonzalez, G., Mayer, R., Weinhouse, H., Volman, G., et al. (2001). Phosphodiesterase A1, a regulator of cellulose synthesis in Acetobacter xylinum, is a heme-based sensor. Biochemistry 40, 3420–3426. doi: 10.1021/bi0100236
Chen, H. J., Li, N., Luo, Y., Jiang, Y. L., Zhou, C. Z., Chen, Y., et al. (2018). The GDP-switched GAF domain of DcpA modulates the concerted synthesis/hydrolysis of c-di-GMP in Mycobacterium smegmatis. Biochem. J. 475, 1295–1308. doi: 10.1042/BCJ20180079
Chou, S. H., and Galperin, M. Y. (2016). Diversity of cyclic Di-GMP-binding proteins and mechanisms. J. Bacteriol. 198, 32–46. doi: 10.1128/JB.00333-15
Christen, M., Christen, B., Allan, M. G., Folcher, M., Jeno, P., Grzesiek, S., et al. (2007). DgrA is a member of a new family of cyclic diguanosine monophosphate receptors and controls flagellar motor function in Caulobacter crescentus. Proc. Natl. Acad. Sci. U.S.A. 104, 4112–4117. doi: 10.1073/pnas.0607738104
Cianciotto, N. P. (2005). Type II secretion: a protein secretion system for all seasons. Trends Microbiol. 13, 581–588. doi: 10.1016/j.tim.2005.09.005
Commichau, F. M., Gibhardt, J., Halbedel, S., Gundlach, J., and Stulke, J. (2018). A delicate connection: c-di-AMP affects cell integrity by controlling osmolyte transport. Trends Microbiol. 26, 175–185. doi: 10.1016/j.tim.2017.09.003
Corrigan, R. M., and Gründling, A. (2013). Cyclic di-AMP: another second messenger enters the fray. Nat. Rev. Microbiol. 11, 513–524. doi: 10.1038/nrmicro3069
Covarrubias, P. C., Moya-Beltrán, A., Atavales, J., Moya-Flores, F., Tapia, P. S., Acuña, L. G., et al. (2018). Occurrence, integrity and functionality of AcaML1-like viruses infecting extreme acidophiles of the Acidithiobacillus species complex. Res. Microbiol. 169, 628–637. doi: 10.1016/j.resmic.2018.07.005
da Costa Vasconcelos, F. N., Maciel, N. K., Favaro, D. C., de Oliveira, L. C., Barbosa, A. S., Salinas, R. K., et al. (2017). Structural and enzymatic characterization of a cAMP-dependent diguanylate cyclase from pathogenic leptospira species. J. Mol. Biol. 429, 2337–2352. doi: 10.1016/j.jmb.2017.06.002
Díaz, M., Castro, M., Copaja, S., and Guiliani, N. (2018). Biofilm formation by the acidophile bacterium Acidithiobacillus thiooxidans involves c-di-GMP pathway and Pel exopolysaccharide. Genes 9:E113. doi: 10.3390/genes9020113
Díaz, M., Copaja, S., and Guiliani, N. (2013). Functional analysis of c-di-GMP pathway in biomining bacteria Acidithiobacillus thiooxidans. Adv. Mater. Res. 825, 133–136. doi: 10.4028/www.scientific.net/AMR.825.133
Dopson, M., Baker-Austin, C., Koppineedi, P. R., and Bond, P. L. (2003). Growth in sulfidic mineral environments: metal resistance mechanisms in acidophilic micro-organisms. Microbiology 149, 1959–1970. doi: 10.1099/mic.0.26296-0
Edgar, R. C. (2010). Search and clustering orders of magnitude faster than BLAST. Bioinformatics 26, 2460–2461. doi: 10.1093/bioinformatics/btq461
English, B. P., Hauryliuk, V., Sanamrad, A., Tankov, S., Dekker, N. H., and Elf, J. (2011). Single-molecule investigations of the stringent response machinery in living bacterial cells. Proc. Natl. Acad. Sci. U.S.A. 108, E365–E373. doi: 10.1073/pnas.1102255108
Falagán, C., and Johnson, D. (2016). Acidithiobacillus ferriphilus sp. nov., a facultatively anaerobic iron- and sulfur-metabolizing extreme acidophile. Int. J. Syst. Evol. Microbiol. 66, 206–211. doi: 10.1099/ijsem.0.000698
Fang, X., and Gomelsky, M. (2010). A post-translational, c-di-GMP-dependent mechanism regulating flagellar motility. Mol. Microbiol. 76, 1295–1305. doi: 10.1111/j.1365-2958.20
Farah, C., Vera, M., Morin, D., Haras, D., Jerez, C. A., and Guiliani, N. (2005). Evidence for a functional quorum-sensing type AI-1 system in the extremophilic bacterium Acidithiobacillus ferrooxidans. Appl. Environ. Microbiol. 71, 7033–7040. doi: 10.1128/AEM.71.11.7033-7040.2005
Fidler, D. R., Murphy, S. E., Courtis, K., Antonoudiou, P., El-Tohamy, R., Ient, J., et al. (2016). Using HHsearch to tackle proteins of unknown function: a pilot study with PH domains. Traffic 17, 1214–1226. doi: 10.1111/tra.12432
Finn, R. D., Coggill, P., Eberhardt, R. Y., Eddy, S. R., Mistry, J., Mitchell, A. L., et al. (2016). The Pfam protein families database: towards a more sustainable future. Nucleic Acids Res. Database Issue 44, D279–D285. doi: 10.1093/nar/gkv1344
Fouts, D. (2006). Phage_Finder: automated identification and classification of prophage regions in complete bacterial genome sequences. Nucleic Acids Res. 34, 5839–5851. doi: 10.1093/nar/gkl732
Gallagher, D. T., Kim, S. K., Robinson, H., and Reddy, P. T. (2011). Active-site structure of class IV adenylyl cyclase and transphyletic mechanism. J. Mol. Biol. 405, 787–803. doi: 10.1016/j.jmb.2010.11.026
Gallant, J., Irr, J., and Cashel, M. (1971). The mechanism of amino acid control of guanylate and adenylate biosynthesis. J. Biol. Chem. 246, 5812–5816.
Gardy, J. L., Spencer, C., Wang, K., Ester, M., Tusnády, G. E., Simon, I., et al. (2003). PSORTb: improving protein subcellular localization prediction for Gram-negative bacteria. Nucleic Acids Res. 31, 3613–3617. doi: 10.1093/nar/gkg602
Gilles-González, M. A., and González, G. (2005). Heme-based sensors: defining characteristics, recent developments, and regulatory hypotheses. J. Inorg. Biochem. 99, 1–22. doi: 10.1016/j.jinorgbio.2004.11.006
González-Toril, E., Martínez-Frías, J., Gómez, J. M., Rull, F., and Amils, R. (2005). Iron meteorites can support the growth of acidophilic chemolithoautotrophic microorganisms. Astrobiology 5, 406–414. doi: 10.1089/ast.2005.5.406
Green, J., Stapleton, M. R., Smith, L. J., Artymiuk, P. J., Kahramanoglou, C., Hunt, D. M., et al. (2014). Cyclic-AMP and bacterial cyclic-AMP receptor proteins revisited: adaptation for different ecological niches. Curr. Opin. Microbiol. 18, 1–7. doi: 10.1016/j.mib.2014.01.003
Guglielmini, J., Néron, B., Abby, S. S., Garcillán-Barcia, M. P., de la Cruz, F., and Rocha, E. P. (2014). Key components of the eight classes of type IV secretion systems involved in bacterial conjugation or protein secretion. Nucleic Acids Res. 42, 5715–5727. doi: 10.1093/nar/gku194
Guy, L., Kultima, J. R., and Andersson, S. G. (2010). Andersson: genoPlotR: comparative gene and genome visualization in R. Bioinformatics 26, 2334–2335. doi: 10.1093/bioinformatics/btq413
Habazettl, J., Allan, M. G., Jenal, U., and Grzesiek, S. (2011). Solution structure of the PilZ domain protein PA4608 complex with cyclic di-GMP identifies charge clustering as molecular readout. J. Biol. Chem. 286, 14304–14314. doi: 10.1074/jbc.M110.209007
Haft, D. H., Selengut, J. D., Richter, R. A., Harkins, D., Basu, M. K., and Beck, E. (2013). TIGRFAMs and genome properties in 2013. Nucleic Acids Res. 41, D387–D395. doi: 10.1093/nar/gks1234
Hallberg, K., González-Toril, E., and Johnson, D. (2010). Acidithiobacillus ferrivorans, sp. nov.; facultatively anaerobic, psychrotolerant iron-, and sulfur-oxidizing acidophiles isolated from metal mine-impacted environments. Extremophiles 14, 9–19. doi: 10.1007/s00792-009-0282-y
Hallberg, K., and Lindström, E. (1994). Characterization of Thiobacillus caldus sp. nov., a moderately thermophilic acidophile. Microbiology 140, 3451–3456. doi: 10.1099/13500872-140-12-3451
Harrison, S. T. L. (2016). “Biotechnologies that utilize acidophiles,” in Acidophiles: Life in Extremely Acidic Environments, eds R. Quatrini and D. B. Johnson (Poole: Caister Academic Press), 265–284. doi: 10.21775/9781910190333.16
Haseltine, W. A., and Block, R. (1973). Synthesis of guanosine tetra- and pentaphosphate requires the presence of a codon-specific, uncharged transfer ribonucleic acid in the acceptor site of ribosomes. Proc. Natl. Acad. Sci. U.S.A. 70, 1564–1568. doi: 10.1073/pnas.70.5.1564
Hauryliuk, V., Atkinson, G. C., Murakami, K. S., Tenson, T., and Gerdes, K. (2015). Recent functional insights into the role of (p)ppGpp in bacterial physiology. Nat. Rev. Microbiol. 13, 298–309. doi: 10.1038/nrmicro3448
Hedrich, S., and Johnson, D. (2013). Acidithiobacillus ferridurans sp. nov., an acidophilic iron-, sulfur- and hydrogen-metabolizing chemolithotrophic gammaproteobacterium. Int. J. Syst. Evol. Microbiol. 63, 4018–4025. doi: 10.1099/ijs.0.049759-0
Hengge, R. (2009). Principles of cyclic-di-GMP signaling. Nat. Rev. Microbiol. 7, 263–273. doi: 10.1038/nrmicro2109
Hickman, J. W., and Harwood, C. S. (2008). Identification of FleQ from Pseudomonas aeruginosa as a c-di-GMP-responsive transcription factor. Mol. Microbiol. 69, 376–389. doi: 10.1111/j.1365-2958.2008.06281.x
Ho, Y., Burden, L., and Hurley, J. (2000). Structure of the GAF domain, a ubiquitous signaling motif and a new class of cyclic GMP receptor. EMBO J. 19, 5288–5299. doi: 10.1093/emboj/19.20.5288
Hochstadt-Ozer, J., and Cashel, M. (1972). The regulation of purine utilization in bacteria. V. Inhibition of purine phosphoribosyltransferase activities and purine uptake in isolated membrane vesicles by guanosine tetraphosphate. J. Biol. Chem. 247, 7067–7072.
Hsiao, W. W., Ung, K., Aeschliman, D., Bryan, J., Finlay, B. B., and Brinkman, F. S. (2005). Evidence of a large novel gene pool associated with prokaryotic genomic islands. PLoS Genet. 1:e62. doi: 10.1371/journal.pgen.0010062
Iyer, L. M., and Aravind, L. (2002). The catalytic domains of thiamine triphosphatase and CyaB-like adenylyl cyclase define a novel superfamily of domains that bind organic phosphates. BMC Genomics 3:33. doi: 10.1186/1471-2164-3-33
Jenal, U., Reinders, A., and Lori, C. (2017). Cyclic di-GMP: second messenger extraordinaire. Nat. Rev. Microbiol. 15, 271–284. doi: 10.1038/nrmicro.2016.190
Johnson, D. B., and Hallberg, K. B. (2005). Acid mine drainage remediation options: a review. Sci. Total Environ. 338, 3–14. doi: 10.1016/j.scitotenv.2004.09.002
Juncker, A., Willenbrock, H., von Heijne, G., Brunak, S., Nielsen, H., and Krogh, A. (2003). Prediction of lipoprotein signal peptides in Gram-negative bacteria. Protein Sci. 12, 1652–1662. doi: 10.1110/ps.0303703
Kall, L., Krogh, A., and Sonnhammer, E. (2004). A combined transmembrane topology and signal peptide prediction method. J. Mol. Biol. 338, 1027–1036. doi: 10.1016/j.jmb.2004.03.016
Kanjee, U., Ogata, K., and Houry, W. A. (2012). Direct binding targets of the stringent response alarmone (p)ppGpp. Mol. Microbiol. 85, 1029–1043. doi: 10.1111/j.1365-2958.2012.08177.x
Karaolis, D. K., Rashid, M. H., Chythanya, R., Luo, W., Hyodo, M., and Hayakawa, Y. (2005). c-di-GMP (3’-5’-cyclic diguanylic acid) inhibits Staphylococcus aureus cell-cell interactions and biofilm formation. Antimicrob. Agents Chemother. 49, 1029–1038. doi: 10.1128/AAC.49.3.1029-1038.2005
Katoh, K., and Standley, D. M. (2013). MAFFT multiple sequence alignment software version 7: improvements in performance and usability. Mol. Biol. Evol. 30, 772–780. doi: 10.1093/molbev/mst010
Keasling, J. D., Bertsch, L., and Kornberg, A. (1993). Guanosine pentaphosphate phosphohydrolase of Escherichia coli is a long-chain exopolyphosphatase. Proc. Natl. Acad. Sci. U.S.A. 90, 7029–7033. doi: 10.1073/pnas.90.15.7029
Ko, J., Ryu, K. S., Kim, H., Shin, J. S., Lee, J. O., Cheong, C., et al. (2010). Structure of PP4397 reveals the molecular basis for different c-di-GMP binding modes by PilZ domain proteins. J. Mol. Biol. 398, 97–110. doi: 10.1016/j.jmb.2010.03.007
Kriel, A., Bittner, A. N., Kim, S. H., Liu, K., Tehranchi, A. K., Zou, W. Y., et al. (2012). Direct regulation of GTP homeostasis by (p)ppGpp: a critical component of viability and stress resistance. Mol. Cell 48, 231–241. doi: 10.1016/j.molcel.2012.08.009
Krogh, A., Larsson, B., von Heijne, G., and Sonnhammer, E. (2001). Predicting transmembrane protein topology with a hidden markov model: application to complete genomes. J. Mol. Biol. 305, 567–580. doi: 10.1006/jmbi.2000.4315
Krzywinski, M. I., Schein, J. E., Birol, I., Connors, J., Gascoyne, R., Horsman, D., et al. (2009). Circos: an information aesthetic for comparative genomics. Genome Res. 19, 1639–1645. doi: 10.1101/gr.092759.109
Laslett, D., and Canback, B. (2004). Aragorn, a program to detect tRNA genes and tmRNA genes in nucleotide sequences. Nucleic Acids Res. 32, 11–16. doi: 10.1093/nar/gkh152
Lee, S. Y., Cho, H. S., Pelton, J. G., Yan, D., Henderson, R. K., King, D. S., et al. (2001). Crystal structure of an activated response regulator bound to its target. Nat. Struct. Biol. 8, 52–56. doi: 10.1038/nsb0901-789
Letunic, I., and Bork, P. (2018). 20 years of the SMART protein domain annotation resource. Nucleic Acids Res. 46, D493–D496. doi: 10.1093/nar/gkx922
Liu, K., Bittner, A. N., and Wang, J. D. (2015). Diversity in (p)ppGpp metabolism and effectors. Curr. Opin. Microbiol. 24, 72–79. doi: 10.1016/j.mib.2015.01.012
Lowe, T. M., and Eddy, S. R. (1997). tRNAscan-SE: a program for improved detection of transfer RNA genes in genomic sequence. Nucleic Acids Res. 25, 955–964. doi: 10.1093/nar/25.5.955
Madsen, J. S., Hylling, O., Jacquiod, S., Pécastaings, S., Hansen, L. H., Riber, L., et al. (2018). An intriguing relationship between the cyclic diguanylate signaling system and horizontal gene transfer. ISME J. 12, 2330–2334. doi: 10.1038/s41396-018-0183-0
Marchler-Bauer, A., Bo, Y., Han, L., He, J., Lanczycki, C. J., Lu, S., et al. (2017). CDD/SPARCLE: functional classification of proteins via subfamily domain architectures. Nucleic Acids Res. 45, D200–D203. doi: 10.1093/nar/gkw1129
Marchler-Bauer, A., and Bryant, S. H. (2004). CD-Search: protein domain annotations on the fly. Nucleic Acids Res. 32, W327–W331. doi: 10.1093/nar/gkh454
Marden, J. N., Dong, Q., Roychowdhury, S., Berleman, J. E., and Bauer, C. E. (2011). Cyclic GMP controls Rhodospirillum centenum cyst development. Mol. Microbiol. 79, 600–615. doi: 10.1111/j.1365-2958.2010.07513.x
McDonough, K. A., and Rodriguez, A. (2011). The myriad roles of cyclic AMP in microbial pathogens: from signal to sword. Nat. Rev. Microbiol. 10, 27–38. doi: 10.1038/nrmicro2688
Mechold, U., Potrykus, K., Murphy, H., Murakami, K. S., and Cashel, M. (2013). Differential regulation by ppGpp versus pppGpp in Escherichia coli. Nucleic Acids Res. 41, 6175–6189. doi: 10.1093/nar/gkt302
Narang, A. (2009). Quantitative effect and regulatory function of cyclic adenosine 5’-phosphate in Escherichia coli. J. Biosci. 34, 445–463. doi: 10.1007/s12038-009-0051-1
Navarro, C. A., von Bernath, D., and Jerez, C. A. (2013). Heavy metal resistance strategies of acidophilic bacteria and their acquisition: importance for biomining and bioremediation. Biol. Res. 46, 363–371. doi: 10.4067/S0716-97602013000400008
Newell, P. D., Boyd, C. D., Sondermann, H., and O’Toole, G. A. (2011). A c-di-GMP effector system controls cell adhesion by inside-out signaling and surface protein cleavage. PLoS Biol. 9:e1000587. doi: 10.1371/journal.pbio.1000587
Nitschke, W., and Bonnefoy, V. (2016). “Energy acquisition in low pH environments,” in Life in Extremely Acidic Environments, eds R. Quatrini and D. B. Johnson (Poole: Caister Academic Press), 19–48. doi: 10.21775/9781910190333.02
Ora, M., Martikainen, K., and Lautkoski, K. (2012). Hydrolytic reactions of cyclic bis(3’-5’) diadenylic acid (c-di-AMP). J. Phys. Org. Chem. 26, 218–225. doi: 10.1002/poc.3070
Orell, A., Navarro, C. A., Rivero, M., Aguilar, J. S., and Jerez, C. A. (2012). Inorganic polyphosphates in extremophiles and their possible functions. Extremophiles 16, 573–583. doi: 10.1007/s00792-012-0457-9
O’Toole, G. A., and Kolter, R. (1998). Flagellar and twitching motility are necessary for Pseudomonas aeruginosa biofilm development. Mol. Microbiol. 30, 295–304. PMID: 9791175 doi: 10.1046/j.1365-2958.1998.01062.x
Paul, B. J., Barker, M. M., Ross, W., Schneider, D. A., Webb, C., Foster, J. W., et al. (2004). DksA: a critical component of the transcription initiation machinery that potentiates the regulation of rRNA promoters by ppGpp and the initiating NTP. Cell 118, 311–322. doi: 10.1016/j.cell.2004.07.009
Paul, B. J., Berkmen, M. B., and Gourse, R. L. (2005). DksA potentiates direct activation of amino acid promoters by ppGpp. Proc. Natl. Acad. Sci. U.S.A. 102, 7823–7828. doi: 10.1073/pnas.0501170102
Paul, K., Nieto, V., Carlquist, W. C., Blair, D. F., and Harshey, R. M. (2010). The c-di-GMP binding protein YcgR controls flagellar motor direction and speed to affect chemotaxis by a “backstop brake” mechanism. Mol. Cell 38, 128–139. doi: 10.1016/j.molcel.2010.03.001
Petersen, T., Brunak, S., von Heijne, G., and Nielsen, H. (2011). SignalP 4.0: discriminating signal peptides from transmembrane regions. Nat. Methods 8, 785–786. doi: 10.1038/nmeth.1701
Potrykus, K., and Cashel, M. (2008). (p)ppGpp: still magical? Annu. Rev. Microbiol. 62, 35–51. doi: 10.1146/annurev.micro.62.081307.162903
Price, M. N., Dehal, P. S., and Arkin, A. P. (2010). FastTree 2–approximately maximum-likelihood trees for large alignments. PLoS One 5:e9490. doi: 10.1371/journal.pone.0009490
Qi, Y., Chuah, M. L., Dong, X., Xie, K., Luo, Z., Tang, K., et al. (2011). Binding of c-di-GMP in the non-catalytic EAL domain of FimX induces a long-range conformational change. J. Biol. Chem. 286, 2910–2917. doi: 10.1074/jbc.M110.196220
Qi, Y., Rao, F., Luo, Z., and Liang, Z. X. (2009). A flavin cofactor-binding PAS domain regulates c-di-GMP synthesis in AxDGC2 from Acetobacter xylinum. Biochemistry 48, 10275–10285. doi: 10.1021/bi901121w
Quatrini, R., and Johnson, D. B. (2018). Acidithiobacillus ferrooxidans. Trends Microbiol. 27, 282–283. doi: 10.1016/j.tim.2018.11.009
Quatrini, R., Ossandon, F. J., and Rawlings, D. (2016). “The flexible genome of acidophilic prokaryotes,” in Acidophiles: Life in Extremely Acidic Environments, eds R. Quatrini and D. B. Johnson (Poole: Caister Academic Press), 199–220. doi: 10.21775/9781910190333.12
Raes, J., Korbel, J. O., Lercher, M. J., von Mering, C., and Bork, P. (2007). Prediction of effective genome size in metagenomic samples. Genome Biol. 8:R10. doi: 10.1186/gb-2007-8-1-r10
Rambaut, A. (2009). FigTree Version 1.3.1 [Computer Program]. Available at: http://tree.bio.ed.ac.uk
Rao, F., See, R. Y., Zhang, D., Toh, D. C., Ji, Q., and Liang, Z. X. (2010). YybT is a signaling protein that contains a cyclic dinucleotide phosphodiesterase domain and a GGDEF domain with ATPase activity. J. Biol. Chem. 285, 473–482. doi: 10.1074/jbc.M109.040238
Rao, N. N., Gómez-García, M. R., and Kornberg, A. (2009). Inorganic polyphosphate: essential for growth and survival. Annu. Rev. Biochem. 78, 605–647. doi: 10.1146/annurev.biochem.77.083007.093039
Revankar, G. R., and Robins, R. K. (1982). “Chemistry of cyclic nucleotides and cyclic nucleotide analogs,” in Handbook of Experimental Pharmacology, eds J. A. Nathanson and J. W. Kebabian (Berlin: Springer-Verlag), 17–124.
Riadi, G., Medina-Moenne, C., and Holmes, D. S. (2012). TnpPred: a web service for the robust prediction of prokaryotic transposases. Comp. Funct. Genomics 2012, 1–5. doi: 10.1155/2012/678761
Rohwerder, T., and Sand, W. (2007). Oxidation of inorganic sulfur compounds in acidophilic prokaryotes. Eng. Life Sci. 7, 301–309. doi: 10.1002/elsc.200720204
Römling, U., Galperin, M. Y., and Gomelsky, M. (2013). Cyclic di-GMP: the first 25 years of a universal bacterial second messenger. Microbiol. Mol. Biol. Rev. 77, 1–52. doi: 10.1128/MMBR.00043-12
Ross, P., Aloni, Y., Weinhouse, C., Michaeli, D., Weinberger-Ohana, P., Meyer, R., et al. (1985). An unusual guanyl oligonucleotide regulates cellulose synthesis in Acetobacter xylinum. FEBS Lett. 186, 191–196. doi: 10.1016/0014-5793(85)80706-7
Ruiz, L. M., Castro, M., Barriga, A., Jerez, C. A., and Guiliani, N. (2012). The extremophile Acidithiobacillus ferrooxidans possesses a c-di-GMP signalling pathway that could play a significant role during bioleaching of minerals. Lett. Appl. Microbiol. 54, 133–139. doi: 10.1111/j.1472-765X.2011.03180.x
Ryjenkov, D., Simm, R., Römling, U., and Gomelsky, M. (2006). The PilZ domain is a receptor for the second messenger c-di-GMP: the PilZ domain protein YcgR controls motility in enterobacteria. J. Biol. Chem. 281, 30310–30314. doi: 10.1074/jbc.C600179200
Sajish, M., Kalayil, S., Verma, S. K., Nandicoori, V. K., and Prakash, B. (2009). The significance of EXDD and RXKD motif conservation in Rel proteins. J. Biol. Chem. 284, 9115–9123. doi: 10.1074/jbc.M807187200
Saxena, I. M., Kudlicka, K., Okuda, K., and Brown, R. M. (1994). Characterization of genes in the cellulose-synthesizing operon (acs operon) of Acetobacter xylinum: implications for cellulose crystallization. J. Bacteriol. 176, 5735–5752. doi: 10.1128/jb.176.18.5735-5752.1994
Semmler, A. B., Whitchurch, C. B., and Mattick, J. S. (1999). A re-examination of twitching motility in Pseudomonas aeruginosa. Microbiology 145(Pt 10), 2863–2873. doi: 10.1099/00221287-145-10-2863
Seshasayee, A. S., Fraser, G. M., and Luscombe, N. M. (2010). Comparative genomics of cyclic-di-GMP signalling in bacteria: post-translational regulation and catalytic activity. Nucleic Acids Res. 38, 5970–5981. doi: 10.1093/nar/gkq382
Shen, B. W., Dyer, D. H., Huang, J. Y., D’Ari, L., Rabinowitz, J., and Stoddard, B. L. (1999). The crystal structure of a bacterial, bifunctional 5,10 methylene-tetrahydrofolate dehydrogenase/cyclohydrolase. Protein Sci. 8, 1342–1349. doi: 10.1110/ps.8.6.1342
Sismeiro, O., Trotot, P., Biville, F., Vivares, C., and Danchin, A. (1998). Aeromonas hydrophila adenylyl cyclase 2: a new class of adenylyl cyclases with thermophilic properties and sequence similarities to proteins from hyperthermophilic archaebacteria. J. Bacteriol. 180, 3339–3344.
Smith, N., Kim, S. K., Reddy, P. T., and Gallagher, D. T. (2006). Crystallization of the class IV adenylyl cyclase from Yersinia pestis. Acta Cryst. 62(Pt 3), 200–204. doi: 10.1107/S1744309106002855
Spira, B., Silberstein, N., and Yagil, E. (1995). Guanosine 3’,5’-bispyrophosphate (ppGpp) synthesis in cells of Escherichia coli starved for Pi. J. Bacteriol. 177, 4053–4058. doi: 10.1128/jb.177.14.4053-4058.1995
Srivatsan, A., and Wang, J. D. (2008). Control of bacterial transcription, translation and replication by (p)ppGpp. Curr. Opin. Microbiol. 11, 100–105. doi: 10.1016/j.mib.2008.02.001
Stent, G. S., and Brenner, S. (1961). A genetic locus for the regulation of ribonucleic acid synthesis. Proc. Natl. Acad. Sci. U.S.A. 47, 2005–2014. doi: 10.1073/pnas.47.12.2005
Tapia, P., Flores, F. M., Covarrubias, P. C., Acuña, L. G., Holmes, D. S., and Quatrini, R. (2012). Complete genome sequence of temperate bacteriophage AcaML1 from the extreme acidophile Acidithiobacillus caldus ATCC 51756. J. Virol. 86, 12452–12453. doi: 10.1128/JVI.02261-12
Tarutina, M., Ryjenkov, D. A., and Gomelsky, M. (2006). An unorthodox bacteriophytochrome from Rhodobacter sphaeroides involved in turnover of the second messenger c-di-GMP. J. Biol. Chem. 281, 34751–34758. doi: 10.1074/jbc.M604819200
Tatusov, R., Galperin, M., Natale, D., and Koonin, E. (2000). The COG database: a tool for genome-scale analysis of protein functions and evolution. Nucleic Acids Res. 28, 33–36. doi: 10.1093/nar/28.1.33
Temple, K., and Colmer, A. (1951). The autotrophic oxidation of iron by a new bacterium: Thiobacillus ferrooxidans. J. Bacteriol. 62, 605–611.
Townsend, P. D., Rodgers, T. L., Glover, L. C., Korhonen, H. J., Richards, S. A., Colwell, L. J., et al. (2015). The role of protein-ligand contacts in allosteric regulation of the Escherichia coli catabolite activator protein. J. Biol. Chem. 290, 22225–22235. doi: 10.1074/jbc.M115.669267
Tu, Q., and Ding, D. (2003). Detecting pathogenicity islands and anomalous gene clusters by iterative discriminant analysis. FEMS Microbiol. Lett. 221, 269–275. doi: 10.1016/S0378-1097(03)00204-0
Tuckerman, J. R., Gonzalez, G., Sousa, E. H., Wan, X., Saito, J. A., Alam, M., et al. (2009). An oxygen-sensing diguanylate cyclase and phosphodiesterase couple for c-di-GMP control. Biochemistry 48, 9764–9774. doi: 10.1021/bi901409g
Tumlirsch, T., and Jendrossek, D. (2017). Proteins with CHADs (Conserved Histidine α-Helical Domains) are attached to polyphosphate granules in vivo and constitute a novel family of polyphosphate-associated proteins (Phosins). Appl. Environ. Microbiol. 83, e3399–e3316. doi: 10.1128/AEM.03399-16
Ulloa, G., Quezada, C. P., Araneda, M., Escobar, B., Fuentes, E., Álvarez, S. A., et al. (2018). Phosphate favors the biosynthesis of CdS Quantum Dots in Acidithiobacillus thiooxidans ATCC 19703 by improving metal uptake and tolerance. Front. Microbiol. 9:234. doi: 10.3389/fmicb.2018.00234
Valdés, J., Pedroso, I., Quatrini, R., and Holmes, D. S. (2008). Comparative genome analysis of Acidithiobacillus ferrooxidans, A. thiooxidans and A. caldus: insights into their metabolism and ecophysiology. Hydrometallurgy 94, 180–184. doi: 10.1016/j.hydromet.2008.05.039
Vernikos, G. S., and Parkhill, J. (2006). Interpolated variable order motifs for identification of horizontally acquired DNA: revisiting the Salmonella pathogenicity islands. Bioinformatics 22, 2196–2203. doi: 10.1093/bioinformatics/btl369
Vinella, D., Albrecht, C., Cashel, M., and D’Ari, R. (2005). Iron limitation induces SpoT-dependent accumulation of ppGpp in Escherichia coli. Mol. Microbiol. 56, 958–970. doi: 10.1111/j.1365-2958.2005.04601.x
Waksman, S., and Joffe, J. S. (1922). Microörganisms concerned in the oxidation of sulfur in the soil. II. Thiobacillus Thiooxidans, a new sulfur-oxidizing organism isolated from the soil. J. Bacteriol. 7, 239–256.
Wang, J. D., Sanders, G. M., and Grossman, A. D. (2007). Nutritional control of elongation of DNA replication by (p)ppGpp. Cell 128, 865–875. doi: 10.1016/j.cell.2006.12.043
Weinhouse, H., Sapir, S., Amikam, D., Shilo, Y., Volman, G., Ohana, P., et al. (1997). c-di-GMP-binding protein, a new factor regulating cellulose synthesis in Acetobacter xylinum. FEBS Lett. 416, 207–211. doi: 10.1016/S0014-5793(97)01202-7
Weitao, T. (2011). “SOS-inducible biofilms, in microbiology book series volume # 3,” in Science Against Microbial Pathogens: Communicating Current Research and Technological Advances, ed. A. Mendez-Vilas (Spain: Formatex Research Center).
Wilksch, J. J., Yang, J., Clements, A., Gabbe, J. L., Short, K. R., Cao, H., et al. (2011). MrkH, a novel c-di-GMP-dependent transcriptional activator, controls Klebsiella pneumoniae biofilm formation by regulating type 3 fimbriae expression. PLoS Pathog. 7:e1002204. doi: 10.1371/journal.ppat.1002204
Williams, K. P., and Kelly, D. P. (2013). Proposal for a new class within the phylum Proteobacteria, Acidithiobacillia classis nov., with the type order Acidithiobacillales, and emended description of the class Gammaproteobacteria. Int. J. Syst. Evol. Microbiol. 63(Pt 8), 2901–2906. doi: 10.1099/ijs.0.049270-0
Winther, K. S., Roghanian, M., and Gerdes, K. (2018). Activation of the stringent response by loading of RelA-tRNA complexes at the ribosomal A-Site. Mol. Cell 70, 95–105.e1-e4. doi: 10.1016/j.molcel.2018.02.033
Witte, G., Hartung, S., Büttner, K., and Hopfner, K. P. (2008). Structural biochemistry of a bacterial checkpoint protein reveals diadenylate cyclase activity regulated by DNA recombination intermediates. Mol. Cell 30, 167–178. doi: 10.1016/j.molcel.2008.02.020
Xiao, H., Kalman, M., Ikehara, K., Zemel, S., Glaser, G., and Cashel, M. (1991). Residual guanosine 3’,5’-bispyrophosphate synthetic activity of relA null mutants can be eliminated by spoT null mutations. J. Biol. Chem. 266, 5980–5990.
Yan, W., Qu, T., Zhao, H., Su, L., Yu, Q., Gao, J., et al. (2010). The effect of c-di-GMP (3’-5’-cyclic diguanylic acid) on the biofilm formation and adherence of Streptococcus mutans. Microbiol. Res. 165, 87–96. doi: 10.1016/j.micres.2008.10.001
Zhu, W., Lomsadze, A., and Borodovsky, M. (2010). Ab initio gene identification in metagenomics sequences. Nucleic Acids Res. 38:e132. doi: 10.1093/nar/gkq275
Zoraghi, R., Corbin, J. D., and Francis, S. H. (2004). Properties and functions of GAF domains in cyclic nucleotide phosphodiesterases and other proteins. Mol. Pharmacol. 65, 267–278. doi: 10.1124/mol.65.2.267
Keywords: Acidithiobacillia, biofilm, c-di-GMP, extremophile, nucleotide second messenger, signal transduction, (p)ppGpp, cAMP
Citation: Moya-Beltrán A, Rojas-Villalobos C, Díaz M, Guiliani N, Quatrini R and Castro M (2019) Nucleotide Second Messenger-Based Signaling in Extreme Acidophiles of the Acidithiobacillus Species Complex: Partition Between the Core and Variable Gene Complements. Front. Microbiol. 10:381. doi: 10.3389/fmicb.2019.00381
Received: 08 January 2019; Accepted: 13 February 2019;
Published: 07 March 2019.
Edited by:
Davide Zannoni, University of Bologna, ItalyReviewed by:
Volkhard Kaever, Hannover Medical School, GermanyCopyright © 2019 Moya-Beltrán, Rojas-Villalobos, Díaz, Guiliani, Quatrini and Castro. This is an open-access article distributed under the terms of the Creative Commons Attribution License (CC BY). The use, distribution or reproduction in other forums is permitted, provided the original author(s) and the copyright owner(s) are credited and that the original publication in this journal is cited, in accordance with accepted academic practice. No use, distribution or reproduction is permitted which does not comply with these terms.
*Correspondence: Raquel Quatrini, cnF1YXRyaW5pQGNpZW5jaWF2aWRhLm9yZw== Matías Castro, bWNhc3Ryb0BjaWVuY2lhdmlkYS5vcmc=
Disclaimer: All claims expressed in this article are solely those of the authors and do not necessarily represent those of their affiliated organizations, or those of the publisher, the editors and the reviewers. Any product that may be evaluated in this article or claim that may be made by its manufacturer is not guaranteed or endorsed by the publisher.
Research integrity at Frontiers
Learn more about the work of our research integrity team to safeguard the quality of each article we publish.