- 1Kochi Institute for Core Sample Research, Japan Agency for Marine-Earth Science and Technology, Kochi, Japan
- 2Research and Development Center for Submarine Resources, Japan Agency for Marine-Earth Science and Technology, Yokosuka, Japan
- 3National Energy Technology Laboratory, United States Department of Energy, Albany, OR, United States
- 4College of Earth, Ocean, and Atmospheric Sciences, Oregon State University, Corvallis, OR, United States
- 5Marine Works Japan, Yokosuka, Japan
- 6Research and Development Center for Ocean Drilling Science, Japan Agency for Marine-Earth Science and Technology, Yokohama, Japan
Hydraulic fracturing is a prominent method of natural gas production that uses injected, high-pressure fluids to fracture low permeability, hydrocarbon rich strata such as shale. Upon completion of a well, the fluid returns to the surface (produced water) and contains natural gas, subsurface constituents, and microorganisms (Barbot et al., 2013; Daly et al., 2016). While the microbial community of the produced fluids has been studied in multiple gas wells, the activity of these microorganisms and their relation to biogeochemical activity is not well understood. In this experiment, we supplemented produced fluid with 13C-labeled carbon sources (glucose, acetate, bicarbonate, methanol, or methane), and 15N-labeled ammonium chloride in order to isotopically trace microbial activity over multiple day in anoxic incubations. Nanoscale secondary ion mass spectrometry (NanoSIMS) was used to generate isotopic images of 13C and 15N incorporation in individual cells, while isotope ratio monitoring–gas chromatography–mass spectrometry (IRM–GC–MS) was used to measure 13CO2, and 13CH4 as metabolic byproducts. Glucose, acetate, and methanol were all assimilated by microorganisms under anoxic conditions. 13CO2 production was only observed with glucose as a substrate indicating that catabolic activity was limited to this condition. The microbial communities observed at 0, 19, and 32 days of incubation did not vary between different carbon sources, were low in diversity, and composed primarily of the class Clostridia. The primary genera detected in the incubations, Halanaerobium and Fusibacter, are known to be adapted to harsh physical and chemical conditions consistent with those that occur in the hydrofracturing environment. This study provides evidence that microorganisms in produced fluid are revivable in laboratory incubations and retained the ability to metabolize added carbon and nitrogen substrates.
Introduction
Hydraulic fracturing (hydrofracturing, HF), injection of pressurized fluid into the subsurface to create new fractures and to extend natural fractures, is used to extract natural gas and oil from hydrocarbon-rich geological strata. These fracture networks greatly increase the amount of hydrocarbons that can be extracted (Arthur et al., 2008; Jenkins and Li, 2008; Kargbo et al., 2010). The injected fluids are composed primarily of water and a propping agent such as sand that holds the fractures open (Nicot and Scanlon, 2012). Chemical additives increase the efficacy of the process and include viscosity modifiers, chemical stabilizers, corrosion inhibitors, and biocides (Gregory et al., 2011; Nicot and Scanlon, 2012). After a well is completed, depressurization causes 10–70% of the hydrofracturing fluid to rapidly return to the surface while the remaining fluid stays in place or flows to the surface later in production (Olmstead et al., 2013). This expelled fluid from a hydrofractured well is called “produced fluids.”
Produced fluids from hydrofractured wells are a concern because large volumes of the fluid are produced at the surface and in addition to the additives, they often contain high concentrations of salt, metals, organic compounds, radionuclides, and microorganisms due to the fluid-rock interactions at depth (Nicot and Scanlon, 2012; Barbot et al., 2013). Changes in chemical composition of produced fluids from the fluid originally injected for hydrofracturing occur due to shale dissolution, brine migration, and water-rock reactions (Blauch et al., 2009; Dresel and Rose, 2010; Warner et al., 2012). Biogeochemical activity may also change the chemical composition of produced fluids (Strong et al., 2013; Akob et al., 2015). Produced fluids must be treated for disposal or for recycling as they are important source of water for subsequent hydrofracturing fluid (Boschee, 2015); however, methods are often limited by resource availability, cost, and energy requirements (Gregory et al., 2011; Lutz et al., 2013; Rahm et al., 2013). Mitigating the compounds present in the produced fluids requires an accounting of their potential sources as well as an understanding of how they might be transformed in a hydrofractured well.
Microorganisms in the produced fluids can originate from the fractured geological formation or from anything at the surface that contacts the fluids used (e.g., water or sand used to produce hydrofracturing fluid, drilling equipment, drilling muds, well casing, gas separator) (Struchtemeyer et al., 2011; Mohan et al., 2013a,b). During hydrofracturing, the addition of chemicals such as biocides and the exposure to extreme conditions of the subsurface (high temperature, change in pH, high concentrations of salt and metals) leads to a microbial community that exhibits low diversity (Mohan et al., 2013a; Booker et al., 2017). Minor components of the community become dominant members of the produced fluids community (Mohan et al., 2013a; Cluff et al., 2014). Cluff et al. (2014) identified “indicator” genera in late stage produced fluids as being dominated by the bacterial Halanaerobium, unclassified Halanaerobiaceae, Selenihalanaerobacter, Flexistipes, and the archaeal Methanohalophilus and Methanosarcinaceae. These taxa are anaerobic halophiles that would appear to be adapted to hydrofracturing well conditions and may alter subsurface geochemistry and the legacy of the well (Cluff et al., 2014).
In order to know what long-term effects microorganisms could have on the produced fluid chemistry or in a hydrofractured well, it is important to know the fraction of these organisms that are alive in the fluids. Sulfate reducing, acid producing, and fermenting microorganisms have all been cultured from produced fluids which demonstrates their viability (Davis et al., 2012; Struchtemeyer et al., 2012; Liang et al., 2016; Booker et al., 2017); however, the ability to grow in culture may not indicate the physiological status of these cells (Rappé and Giovannoni, 2003; Puspita et al., 2012). In one metagenomic study, genes related to carbohydrate metabolism and stress response increased during the first 9 days of hydrofracturing suggesting that the microbial community responds to changes associated with hydrofracturing (Mohan et al., 2014). These investigations have clarified some aspects of the microbial ecology of the produced fluids but additional studies are needed to assess the degree to which microbes are alive in the produced fluids.
The aim of this research was to determine whether microbial communities in the produced fluids from hydrofractured systems retain the ability to assimilate carbon and nitrogen, the conditions under which such assimilation occurs, and the types of microbial communities associated with assimilation. We incubated a produced fluid for 60 days with 13C-labeled glucose, acetate, methanol, bicarbonate, or methane, and 15N-labeled ammonium chloride, all compounds that could be derived either from the subsurface or hydraulic fracturing fluid (Orberger et al., 2005; Horsfield et al., 2006; Environmental Protection Agency [EPA], 2012; Stringfellow et al., 2014). A nano-scale secondary ion mass spectrometry (NanoSIMS), a ultra-high spatial resolution isotope imaging instrument, was used to detect incorporation of stable isotopes in individual cells (Morono et al., 2011). Isotope ratio monitoring-gas chromatography-mass spectrometry, and high temperature catalytic oxidation were used to measure 13C-labeled metabolic products. Community analysis was conducted to determine the microbial communities that developed due to the availability of different substrates.
Materials and Methods
Sample Collection
Produced fluid samples were collected from the gas/liquid separator of a hydraulically fractured, horizontal well in Greene Co., Pennsylvania in May 2014. The well was drilled into the Marcellus shale which has a temperature that ranges from 35 to 51°C and an approximate pressure of 40 MPa at this location (USGS; Kargbo et al., 2010). The sample was collected in a sterile 4 L polypropylene bottle filled to capacity without headspace and shipped overnight to Oregon State University on ice.
Experiment Setup
Forty milliliter aliquots of the produced fluid were incubated in 50 mL serum bottles that had been acid washed and combusted at 400°C for 4 h. Killed controls were autoclaved prior to allocation for 2 h at 121°C. Anoxic samples were sparged with nitrogen gas in an anaerobic chamber and capped with butyl rubber septa (Chemglass, CLS-4209-14). The absence of oxygen was confirmed by measuring oxygen saturation with a Micro TX3 – AOT Microsensor oxygen meter (PreSens, Regensburg, Germany). The supplemental carbon substrates were 13C-labeled-glucose (13C6, 99%), -acetate (1,2-13C2, 99%), bicarbonate (13C, 99%), methanol (13C, 99%), and methane (13C, 99%) and the nitrogen substrate was 15N-labeled ammonium chloride (15N, 99%) (Cambridge Isotope Laboratories), and added to final concentrations of 1 and 0.1 mM, respectively, excluding methane. For methane samples, 5 mL of gas was added with a syringe and tightly sealed with an aluminum cap afterward. No other supplemental nutrient was added. In total, 25 bottles (five substrates, four time points, one killed control) were prepared and shipped via express courier (48 h transit time) to the Kochi Institute for Core Sample Research, JAMSTEC in Kochi, Japan for downstream isotopic and genomic analysis. Once at Kochi, all samples were incubated at 25°C. Individual incubations with each supplemental carbon source were destructively sampled at 0, 19, 32, or 60 days for analyses.
Cell Counts
To prepare samples for cell counts, serum bottles were sonicated for 30 s and briefly vortexed to remove precipitates and cells from the sides of the bottles. Twenty milliliters were transferred to a Falcon tube and centrifuged. The sediment pellets were fixed with 4% paraformaldehyde in 1 × phosphate buffered saline (PBS) for 3 h at 4°C. The pellets were washed twice with 1 × PBS and then stored in a 1:1 solution of 1 × PBS: 100% ethanol at -20°C. Samples were prepared as described in Morono et al. (2013). Briefly, fixed sediment slurry was resuspended in 2.5% NaCl and treated with a detergent mix composed of 12.5 mM EDTA, 12.5 mM Na3PO4, 0.1% v/v Tween-80, 0.1% MeOH, final concentration. Cells were detached from the produced fluid precipitates by shaking at 500 rpm for 1 h (Shake Master, Bio Medical Science) and sonicating at 200 watts in 30 s intervals for 10 cycles (Bioruptor UCD-250, Cosmo Bio). Carbonate minerals were removed with hydrofluoric acid (1.1% v/v, final concentration) for 20 min and then samples were neutralized with 1.5 M Tris-base. The final solution was added to 2.5% NaCl and filtered through 0.22 μm black polycarbonate membranes (Isopore Membrane Filter GTBP, Merck). Cells were stained with a 1:40 dilution of SYBR-green I (Thermo): 1 × TE buffer. Five hundred microliters of 1:100 diluted microspheres were added as a reference for microscopic focusing and the resulting membrane was mounted with 3–10 μL of mounting solution (1:2 mixture of TE buffer and Vectashield [Vector Laboratories]). The cells were counted with an automated fluorescence microscope (Morono and Inagaki, 2010).
DNA Extraction and 16S rDNA Sequencing
To collect microbial community DNA, 10 mL aliquots of the produced fluids were collected after sonication and vortexing. The aliquots were centrifuged and the remaining pelleted solid was stored at -80°C. Genomic DNA was extracted using the hot alkaline method (Morono et al., 2014). In brief, pellets were warmed to 70°C in 12.5 mM EDTA for 10 min and cells were lysed at 70°C with lysis solution (1% SDS, and 1 N NaOH, final concentration) for 20 min. Supernatant was transferred to a tube with neutralization buffer (1 M N HCl, 0.3 M Tris-HCl). The remaining pellet was washed with prewarmed distilled water, centrifuged, and supernatant was transferred to the same sample tube. The extract was treated with equal volumes of phenol–chloroform–isoamyl alcohol (25:24:1) and chloroform–isoamyl alcohol (24:1) and then precipitated by adding a 1/10 volume of 3 M sodium acetate, and a 1:500 volume of polyacrylamide. The V4 region of the 16S rRNA gene was sequenced using Illumina MiSeq. The Illumina sequences were aligned to the SILVA database (release 123, Quast et al., 2013) using the program Mothur (version 1.37.0, Schloss et al., 2009). Chao richness, abundance-based coverage estimator (ACE), Jackknife, Shannon, and Simpson diversity estimators were calculated using Mothur.
The genera detected mainly constituted less than 1% of the community and were considered to be minor community. Genera that were abundant in PCR blanks or DNA negative controls and not abundant in the experimental samples (Supplementary Table 1) were deemed to be contaminants and were manually removed from the dataset. Some genera were also removed because they are often detected as contaminants during sequencing studies or because they are known to be associated with human contamination (Supplementary Table 1; Salter et al., 2014). Using this approach, approximately 5% of the sequences from all of the experimental samples were removed. However, the percent of sequences removed also varied from sample to sample (Supplementary Table 2). Two samples had notably high percentages of putative contaminants and fewer sequences as well.
Activity Detection Using NanoSIMS
Cells were separated from sediments using a multi-layer density gradient separation protocol (Morono et al., 2013). Samples were layered over a Nycodenz and sodium polytungstate multi-layer density solution. After centrifugation, the supernatant was removed and filtered through an Anodisc membrane (GE Healthcare) followed by staining with 1:40 dilution of SYBR Green I in 1 × TE buffer. Cells were resuspended by sonicating the membrane and the resulting cell suspension was subjected to fluorescence-activated cell sorting by using Moflo Cell Sorter (Beckman Coulter). Sorted cells were captured onto an indium-tin-oxide (ITO) coated black polycarbonate membrane.
Isotopic imaging analysis was conducted with a NanoSIMS 50L ion microprobe (AMETEK Co., Ltd., CAMECA BU) at the Kochi Institute for Core Sample Research, JAMSTEC. Samples on the ITO coated polycarbonate membrane were pre-sputtered at high beam currents (30–40 pA) for a few minutes to remove surface contamination and to obtain steady state of the secondary ion intensities before measurement. The secondary ions of 12C-, 13C-, 16O-, 12C14N-, and 12C15N- and 32S- were collected and measured in parallel at a mass resolution of ∼9,000 that is sufficient to separate the 13C- from the 12C1H- and 12C15N- from the 13C14N-. Detailed isotopic images of the cells were obtained by rastering a 0.8–1.3 pA 16 keV Cs+ primary ion beam (∼100 nmφ) over an area of 25 × 25 μm field of view. Each image consisted of 256 × 256 pixels with a dwell time of 2 ms per pixel, and the final image was created by amalgamating 20 images for same analysis area. Recorded images and data were processed using a CAMECA WinImage software and OpenMIMS plugin (Gormanns et al., 2012) in ImageJ (Schneider et al., 2012) distribution of Fiji (Schindelin et al., 2012). The different scans of each image were aligned to correct image drift during acquisition. Final images were generated by adding the secondary ion counts of each recorded secondary ion for each pixel over all scans. Intracellular carbon and nitrogen uptake from stable isotope-labeled substrates was calculated by drawing regions-of-interest on 12C14N- and/or 32S- images and calculating 13C/12C and 15N/14N ratio (inferred from the 12C15N/12C14N ratio) while having the data from blank filter area for standardizing multiple analysis data.
Metabolic Byproducts
The metabolic byproducts of the supplemented 13C-labeled carbon sources, 13CO2, dissolved inorganic 13C, and 13CH4, were all measured with IRM–GC–MS using a Thermo/Finnigan Delta Plus XP IRMS instrument (Thermo Electron Corp., San Jose, CA, United States). Total CO2, dissolved inorganic carbon (DIC), and CH4 were also measured.
Nucleotide Sequence Accession Numbers
The nucleotide sequences reported in this study have been deposited in the DDBJ/EMBL/GenBank database under accession number DRA007788.
Results
Sample Collection and Cell Counts
Upon arrival at Oregon State University, the produced fluid sample was black in color. The solids at the bottom of each serum bottle (Figure 1) served as a relative indicator for the presence of oxygen wherein the anoxic incubations remained black. A sample exposed to oxygen turned orange suggesting oxidation of iron.
Cells were enumerated with automated fluorescence microscopy at 0, 19, 32, and 60 days of incubation. At the beginning of the incubation period (0 day), the cell numbers in the samples varied between 7.9 (±1.5) × 107 cells/mL of the produced fluid. During the incubation period from 0 to 19 days, cell numbers declined to between 1.6 × 107 and 3.5 × 107 cells/mL and then, between 19 and 60 days, a gradual decline in cell numbers was observed yielding between 1.9 × 106 and 3.6 × 106 cells/mL by the time the experiment ended.
Metabolic Byproducts
We measured DIC in the incubation samples at 60 days in order to determine the degree to which different substrates were respired under anoxic conditions. Samples containing 13C-labeled acetate, methanol, methane, and no substrate addition control contained DIC concentrations of 2.8, 2.7, 2.9, and 2.7 mM, respectively, and carbon isotopic composition (atomic % of 13C-DIC) of 1.19, 1.19, 1.12, and 1.10%, respectively. The killed controls all had a lower concentration of DIC with lower 13C (13C-labeled acetate: 0.9 mM, 1.12%; 13C-labeled methanol: 0.9 mM, 1.11%; 13C-labeled methane: 1.0 mM, 1.13%, we did not prepare a killed control for no substrate addition control). Although DIC in the killed control for 13C-labeled glucose was not measured, the sample containing 13C-labeled glucose had the highest concentrations of DIC and atomic % of 13C of 4.8 mM and 1.40%, respectively.
Total CH4 and carbon isotopic composition of CH4 (atomic % of 13C) in the incubation samples at 32 days and 60 days were measured to determine if any of the substrates were used for methanogenesis. The total CH4 at 32 days was between 0.8 and 1.6 μM for all samples. At 60 days the total methane concentrations were more varied with samples containing acetate, methanol, bicarbonate, and glucose showing evidence of 7.5, 0.9, 3.4, and 2.1 μM CH4, respectively. The killed controls had no detectable methane. Atomic % of 13C-CH4 values were highest in the incubation containing methanol at both 32 and 60 days with 34.54 and 36.13%, respectively. Atomic % of 13C-CH4 in the incubation with 13C-bicarbonate at 32 and 60 days were 3.36 and 2.08%, respectively. Atomic % of 13C-CH4 in the incubations with 13C-acetate or 13C-glucose didn’t show high values (0.997–1.002%).
NanoSIMS Analysis
NanoSIMS analysis revealed that microorganisms in the produced fluid actively assimilated carbon and nitrogen provided in the incubations. For carbon, this was indicated by an atomic % 13C value of ≥1 in individual cells (see Table 1 for summary of 13C assimilation in different treatments) and NanoSIMS images that were taken for different carbon sources under anoxic conditions. 13C assimilation was observed in all of the substrates other than methane (Figure 2 and Supplementary Figure 1). The relative abundance of 13C in individual cells is illustrated in Figure 2 (middle row) by color gradients with warmer colors indicating a higher percentage of 13C incorporated during the incubation and cooler colors indicating a lower abundance of 13C incorporated during the incubation. Glucose and methanol were assimilated favorably in the incubations. The incubation containing bicarbonate and acetate showed very slight 13C assimilation after 60 days. Anoxic methane samples showed no assimilation of 13C (Table 1).
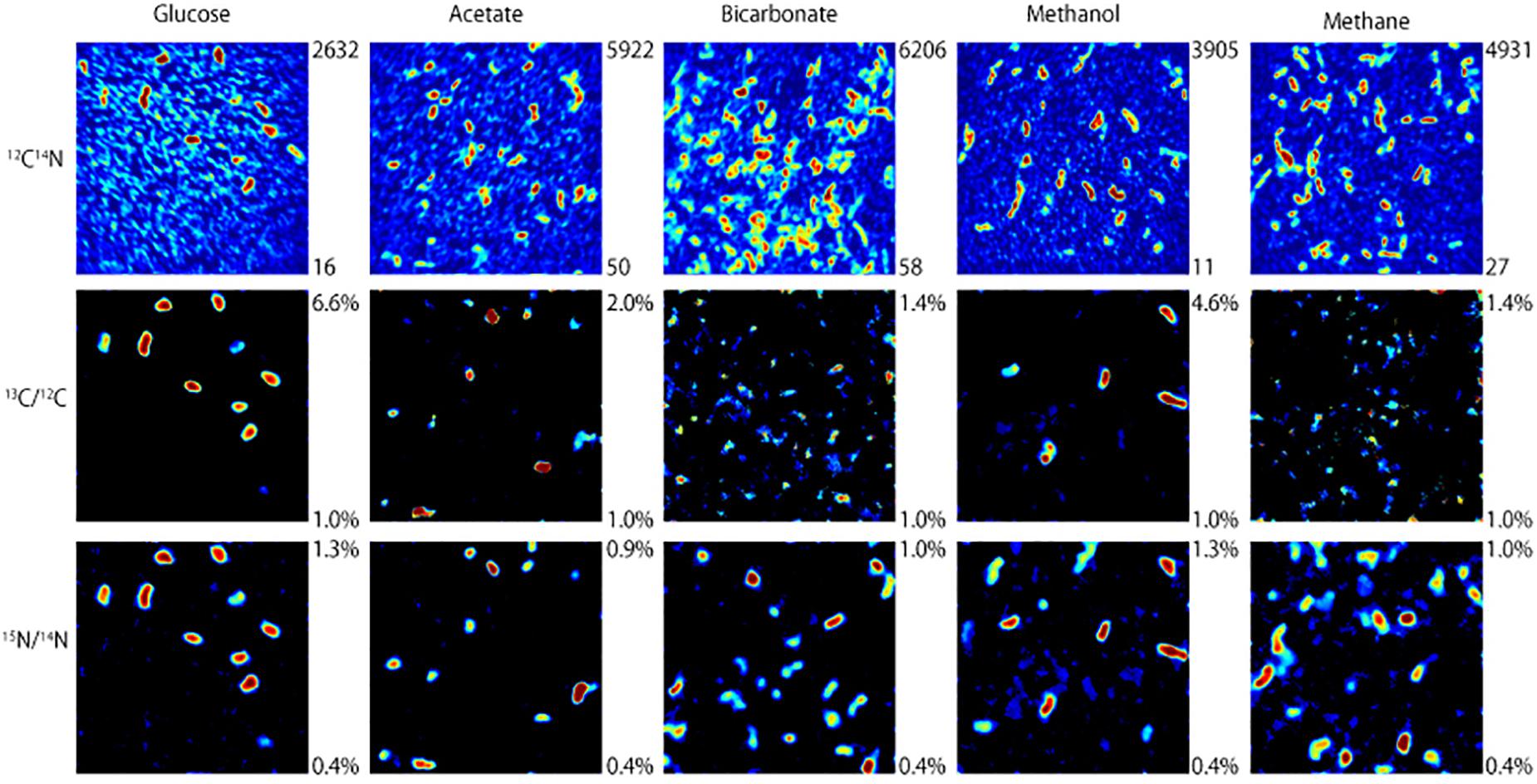
Figure 2. NanoSIMS images of microbial cells incubated with 13C-labeled carbon sources and 15NH4Cl for 32 days under anoxic conditions. The top row illustrates 12C14N ion counts that identify microbial cells. The middle and bottom rows show which of those microbial cells assimilated 13C and 15N, respectively, with warmer colors indicating a higher degree of assimilation.
To determine the relative preference that substrate-assimilating cells had for carbon or nitrogen, the assimilation ratios of 13C and 15N were plotted (Figure 3). In general, cells that were able to assimilate nutrients assimilated more 13C than 15N. Carbon from glucose was preferentially assimilated over nitrogen between 19 and 60 days. Carbon from acetate was preferentially assimilated over nitrogen at 32 and 60 days. Methanol assimilation was only observed at 32 days, but was again preferred over nitrogen. Few of the observed cells assimilated a significant amount of bicarbonate by 60 days.
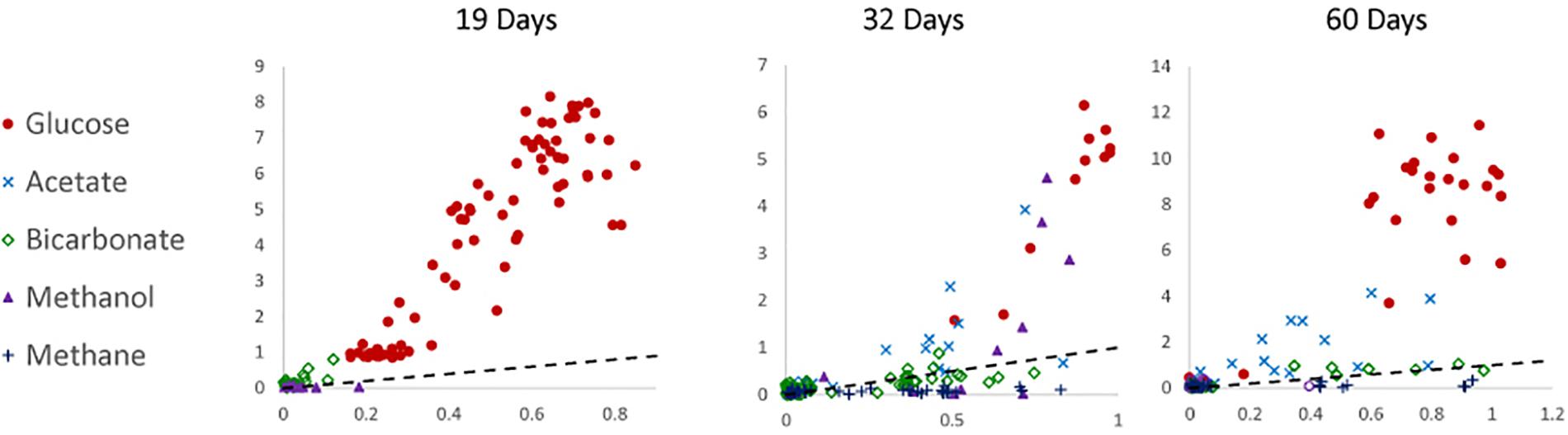
Figure 3. Carbon (atomic % 13C; y-axis) to nitrogen (atomic % 15N; x-axis) assimilation ratios of 19-, 32-, and 60-day incubations under anoxic conditions. The dotted line represents a 1:1 ratio. Data were not available for 0-day incubations.
Microbial Community Characterization
All samples at 19 and 32 days were dominated by the Clostridia such that cells from this class of Bacteria composed over 95% of the community (Figure 4). Within the Clostridia, Halanaerobium and Fusibacter were the dominant genera (Figure 5). While Halanaerobium composed 80% or more of the community in most incubations, Fusibacter became a larger portion of the community by 32 days, with the exception of samples containing glucose (Figure 5). The diversity of the microbial communities varied over time and between carbon substrates (Table 2). For example, Chao richness as an indicator of community diversity decreased between 19 and 32 days in incubations that contained acetate or methane, but increased during this same interval in incubations that contained glucose or methanol. Rarefaction curves indicated that the sequencing effort captured part of the diversity in the incubated samples of produced fluid (Supplementary Figure 2).
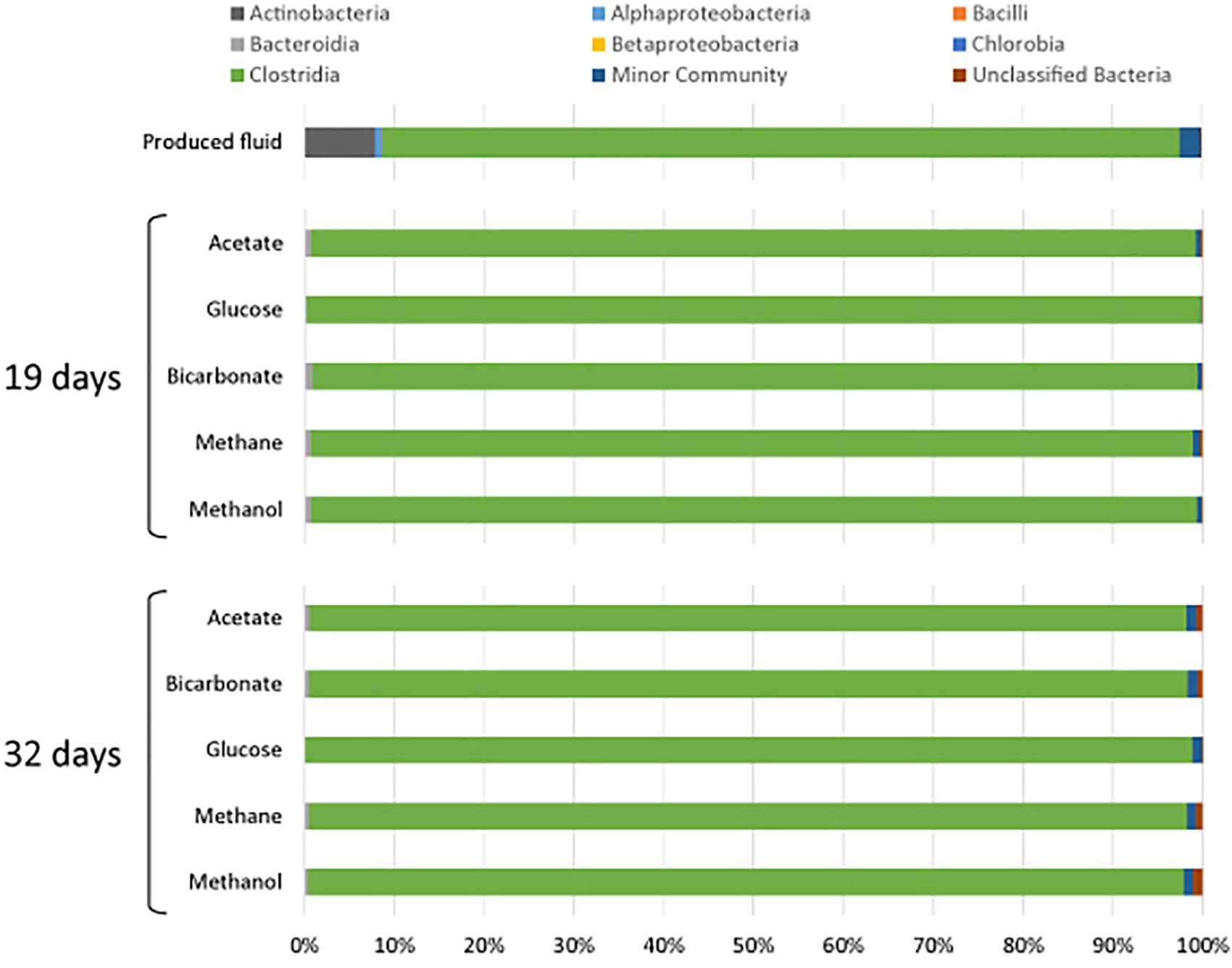
Figure 4. Microbial community composition of produced water after 19 and 32 days of incubation with different carbon substrates added.
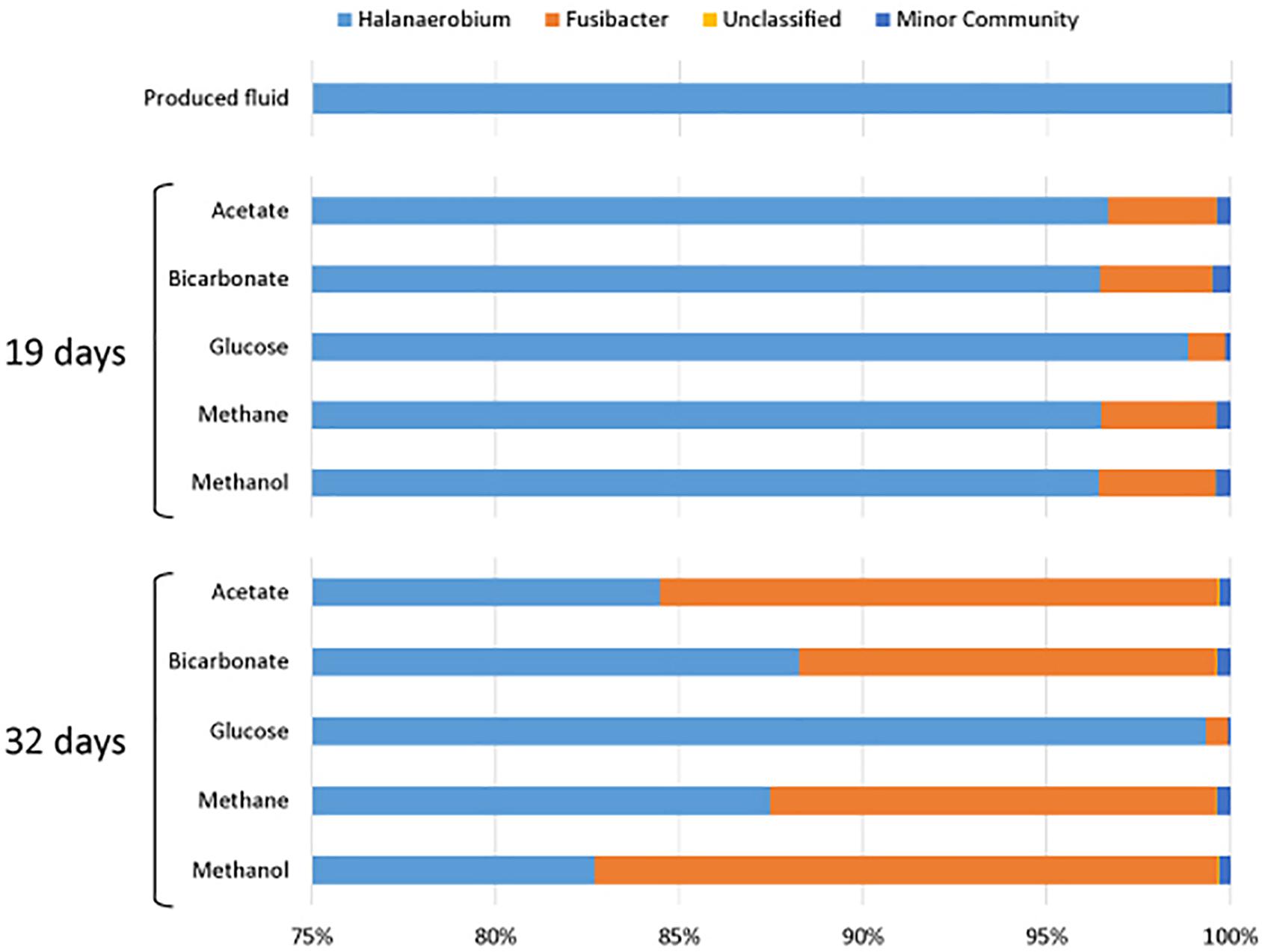
Figure 5. Genera of the class Clostridia that were present in produced water after 19 and 32 days of incubations with different carbon substrates added.
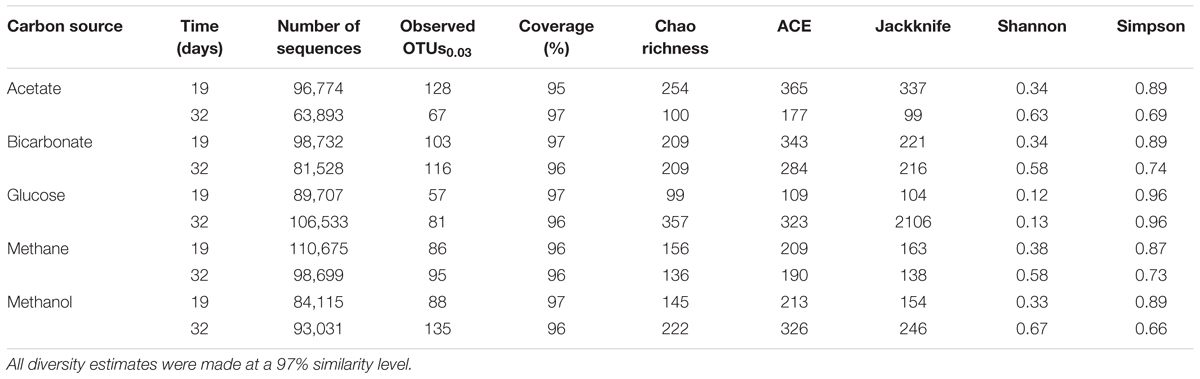
Table 2. Sequencing and diversity estimates of the produced fluids for 19 or 32 days under anoxic conditions.
Discussion
Our research on the metabolic activity of cells present in produced fluids is consistent with reports from past investigations that establish the role of microbes in corrosion and gas souring during hydrocarbon recovery (Enning and Garrelfs, 2014), and those specific to hydrofracturing that have characterized the microbial ecology of the produced fluids (Struchtemeyer et al., 2011; Struchtemeyer and Elshahed, 2012; Mohan et al., 2013b, 2014; Cluff et al., 2014; Akob et al., 2015; Daly et al., 2016). In our research, isotopically labeled nutrients were used to determine the survival and revivability of microorganisms derived from a fluid produced from hydrofractured shales. The carbon compounds used were those that could be available in hydrofractured wells including carbohydrates, short-chain fatty acids, C1 compounds, and inorganic carbon. Glucose, acetate, and methanol are all compounds that may be derived from chemical additives. Methanol is added as a gel stabilizer, acid corrosion inhibitor or winterizing agent (Stringfellow et al., 2014; Daly et al., 2016; FracFocus Chemical Disclosure Registry, 2016). Some hydrofracturing additives can break down into simpler carbon compounds such as glucose or acetate (Stringfellow et al., 2014). Acetate can be an important carbon source in the subsurface if formed by thermal cracking or biodegradation of complex organic compounds found in the subsurface (Horsfield et al., 2006). Methane and bicarbonate are naturally present in shale and dissolve into the produced fluids. The nitrogen source, ammonium, can be present naturally in shale as a substitute for potassium ions in illite, and present as ammonium chloride which may be added to hydrofracturing fluid as a scale inhibitor (Orberger et al., 2005; Environmental Protection Agency [EPA], 2012). We found that the produced fluid microorganisms, dominated by two genera of halophiles, were alive, revivable, and able to metabolize added compounds anabolically and catabolically under anoxic conditions.
Metabolism of Carbon Sources Under Anoxic Conditions
To determine which carbon compounds were metabolized under anoxic conditions, the concentration and 13C-atomic % of DIC and CH4 were analyzed. DIC concentrations were higher in the no substrate added incubation (2.7 mM) than all killed control conditions (0.9∼1.0 mM) showing that the organic carbon originally present in produced fluid was metabolized by the microbial community. As discussed above, carbohydrates, short-chain fatty acids, C1 compounds, and inorganic carbon as well as nitrogen compounds such as ammonium salts and amines are all compounds that may be present in produced fluid (Stringfellow et al., 2014; FracFocus Chemical Disclosure Registry, 2016). Also, degradation of guar gum, a viscosifier used in injected fluids, by microorganism of Halanaerobium sp. can produce acetate (Liang et al., 2016).
The atomic % of 13C-DIC in the sample containing 13C-labeled glucose (1.40%) demonstrates the ability of microbes in the produced fluid to metabolize glucose. Fermentative glucose consumption was observed in Halanaerobium isolate (Booker et al., 2017). It is possible that anaerobic respiration in hydrofractured gas wells is similar to that observed in oil wells where ferrous iron or sulfate are often plentiful and commonly used as electron acceptors (Nazina et al., 1995; Röling et al., 2003; Struchtemeyer and Elshahed, 2012). Sulfate reducers are particularly troublesome in hydrofracturing wells because they produce hydrogen sulfide, which corrodes well casings and equipment (Bottero et al., 2010; Cord-Ruwisch et al., 2013; Booker et al., 2017; Lipus et al., 2017). On the other hand, fermentation has been used to enhance oil recovery through the release of metabolic byproducts such as acids, solvents, or gases which can increase access to oil and increase its mobility in the formation (Desai and Banat, 1997). We observed a 2.1 mM increase in DIC concentration (to 4.8 mM) over the no substrate addition control (2.7 mM); however, the increase of atomic % of 13C-DIC to 1.40% could not solely be explained by the metabolism of glucose. This suggests that, when added, glucose is metabolized as a carbon source but also may stimulate the activity of microorganisms to metabolize other organic compounds present in the produced fluid during incubation.
Incubations containing 13C-labeled acetate showed a significant increase of DIC concentrations and atomic % of 13C-DIC, which indicate anaerobic metabolism of both added acetate and other organic compounds in the produced fluid. High atomic % of 13C-CH4 was not observed in the incubation. Because acetoclastic methanogens are sensitive to high salinity environments (Waldron et al., 2007), they may be less active in hydrofractured systems than cells that possess other metabolic strategies.
There was a slight increase in atomic % of 13C-DIC in incubations containing 13C-labeled methanol. This is consistent with methanol incorporation into microbial cells, as carried out via methylotrophic metabolisms. Methylotrophs can use methane, methanol and other methylated compounds as their sole carbon and energy source (Chistoserdova, 2011). Incubations containing methanol yielded high levels of atomic % of 13C-CH4 by the end of the 60-day incubation period compared to incubations with all other substrates, indicating high methanogenesis activity using methyl moiety.
The high atomic % of 13C-CH4 values in incubations containing 13C-labeled bicarbonate suggest the occurrence of hydrogenotrophic methanogenesis.
Assimilation of Carbon and Nitrogen Under Anoxic Conditions
NanoSIMS was used to view carbon and nitrogen assimilation in individual cells and to compare the utilization of different carbon sources by the microorganisms in the produced fluids under anoxic incubations. Anaerobic activity is expected as the produced fluid is derived from a highly reduced, anoxic subsurface environment. The produced fluid used in this sample had low oxygen saturation when the experiments were started. Aerobic microbial communities have been identified in hydrofracturing fluid prior to injection into the subsurface; however, facultative and strict anaerobes dominate in the fluids when they return from the subsurface (Mohan et al., 2011, 2013a; Cluff et al., 2014). Glucose was the most readily assimilated carbon source under anoxic conditions. Between 73 and 79% of the microbial cells in the incubated samples showed assimilation of glucose and the highest assimilation of 13C (∼11.4%) was observed. A wide variety of anaerobic microorganisms, particularly fermenters possess phosphoenolpyruvate:carbohydrate transferase systems that can transport glucose into the cell and phosphorylate it, making it directly available for catabolic or anabolic reactions (Roseman, 1969; Romano et al., 1970, 1979). This finding demonstrates the ability of microorganisms in the produced fluids to utilize carbohydrates as their carbon source and is consistent with published evidence of microbes that possess genes related to carbohydrate metabolism or microbes capable of glucose use in produced fluids (Mohan et al., 2014; Daly et al., 2016; Booker et al., 2017). Polymer gels are added as proppant carriers and viscosity modifiers; however, high temperatures, extreme chemical conditions, or biological activity can degrade them to simple carbohydrates (Kahrilas et al., 2015). For example, guar gum is added in high concentrations to hydrofracturing fluid to adjust viscosity, and its degraded byproducts promote microbial activity downhole (Lester et al., 2014).
Acetate was the next most readily assimilated carbon source (Table 1 and Figure 3) and is a common byproduct of fermentation in the subsurface (Lovley and Chapelle, 1995). Acetic acid, which is added to hydrofracturing fluid as a buffer, could also be a source of carbon (Stringfellow et al., 2014). Fermentative production of acetate was observed in a microcosm experiment dominated by Halanaerobium (Borton et al., 2018). Acetate can be assimilated by the glyoxylate cycle, alternate glyoxylate pathway, and glyoxylate regeneration cycle (Ensign, 2006) and is used by iron, sulfate, and nitrate reducing bacteria, all of which have been identified in the produced fluids from gas wells (Struchtemeyer and Elshahed, 2012; Mohan et al., 2013a,b). Acetoclastic methanogens can also assimilate acetate, but their presence may be unlikely in this setting because of their sensitivity to high salinity and to changes in salt concentration (Patel and Roth, 1977; Waldron et al., 2007; Winkel et al., 2014).
A particularly interesting result of our study was that microorganisms in the produced fluid assimilated carbon from methanol, a compound sometimes added to hydrofracturing fluid as a winterizer or chemical stabilizer. This result was observed after 32 days of incubation and not again at 60 days; however, such cells may have been present but below the limit of detection using NanoSIMS. This may suggest the presence of methylotrophs, which could also include methanotrophs and methanogenic archaea. We did not observe either anabolic or catabolic metabolism of methane during our study. This may have resulted because 13CH4 was added as a gas without pressurization and was therefore less accessible by microorganisms. Methanotrophy should not be ruled out; however, rates of anaerobic oxidation of methane can be quite low (c.f., Orcutt et al., 2013) and may not have been detected during our relatively short incubations. Methane in the subsurface is dissolved into hydraulic fracturing fluid under pressure, increasing its solubility and making it readily available.
Carbon fixation was not significantly observed in cells incubated with bicarbonate. The maximum percentage of cells that were observed to assimilate 13C-bicarbonate was only 1% at 60 days, but cells that assimilated bicarbonate also assimilated small amounts of nitrogen. This may suggest that autotrophy is not an energy efficient metabolism for microorganisms in hydrofracturing wells, at least under the conditions of our experiment. Ample carbonate (as calcium carbonate) often exists as natural fractures in shale, and can dissolve into solution upon the addition of hydrofracturing fluid (Gale and Holder, 2010). Sodium or potassium carbonate may be added to hydrofracturing fluid (Stringfellow et al., 2014) such that concentrations reach tens to hundreds of mg/L (Haluszczak et al., 2013; Lester et al., 2015). Produced fluids from the Marcellus shale contains an average of 165 mg/L of calcium carbonate (Barbot et al., 2013).
The ratios of 13C and 15N assimilation into microorganisms in the produced fluid showed that cells assimilating supplied nutrients incorporated more carbon than nitrogen although DIC measurements clearly showed metabolism of other non-labeled sources of carbon or nitrogen present in the produced fluid. This apparent preference for carbon was particularly noticeable in glucose incubations. This may be because nitrogen is more limiting than carbon in such environments, or alternatively that these microorganisms were incorporating high C/N ratio organic matter rather than ammonium. In our study, it seems unlikely that the produced fluid is carbon limited, considering the high organic content of shale. The C/N ratio of Devonian (Marcellus shale) age organic carbon is between 174.80 and 193.28 atomic C/N, and the C/N ratio of kerogen is even higher, making nitrogen the limiting nutrient (Orberger et al., 2005). Produced fluids from Marcellus shale were reported to have total organic carbon concentrations of between 1.2 and 1530 mg/L (Barbot et al., 2013). Also considering the length of these incubations, the incorporation of 13C may have not come from the original carbon source but rather from cell debris that had previously incorporated the 13C, which may have served as an additional nitrogen source. In this interpretation, there may have been some turnover of the originally incorporated labeled substrates. Heterotrophic bacteria can assimilate amino acids over ammonium as this allows them to conserve energy required to build amino acids (Kirchman et al., 1989; Morono et al., 2011).
Interpretation of microbial activity, including carbon and nitrogen assimilation, can be confounded by environmental conditions such as high salinity, high temperature, high pressure, or non-neutral pH that are common in subsurface environments (Postma and Jakobsen, 1996; Colwell et al., 1997; Fredrickson et al., 1997). Hydrofracturing produces a dynamic environment where these conditions vary depending on the stage of hydrofracturing and the residence time of the fluid downhole. Microbial cells may not be assimilating carbon and nitrogen for growth and replication, but may instead assimilate these elements mainly for cell maintenance. Extreme conditions increase the amount of energy that microorganisms need to repair or replace cellular components even if they are well adapted to the environment (Hoehler, 2007; Morozkina et al., 2010). Furthermore, slow growth could be advantageous for cells in hydrofractured wells where biocides are used because slow growth and nutrient limitation can reduce microbial sensitivity to antimicrobials, which are commonly used in hydrofracturing (Mah and O’Toole, 2001).
Microbial Communities in Produced Fluid Incubations
Microbial community diversity determinations were conducted on the produced fluid samples incubated under anoxic conditions for 0, 19, and 32 days. The 0 day glucose sample had 50% of the sequences removed and this was also apparent in the microbial community, but less so than the 0 day no substrate control. It is suspected that these samples had poorer DNA recovery and PCR amplification than other samples.
Microbial diversity estimates indicated that the diversity in these samples was lower than what has been previously found in produced fluids and impoundment waters (Struchtemeyer et al., 2012; Mohan et al., 2013a,b). This may have resulted from conditions when the samples were collected, sample handling, or from the placement of these microbial communities into microcosms thereby eliminating the input and output of nutrients and metabolic waste products. Most of the microbial communities in this experiment were very similar, indicating that the addition of substrate did not affect the microbial community. The composition of the microbial community was similar to what has been observed in late stage produced fluids (Mohan et al., 2013a; Cluff et al., 2014; Liang et al., 2016; Booker et al., 2017).
All of the samples were dominated by Clostridia. This is in agreement with past observations wherein late stage produced fluids were dominated by Clostridia, specifically Halanaerobium (Mohan et al., 2013a; Cluff et al., 2014). Cluff et al. (2014) also reported other indicator genera that we detected including Flexistipes, and Methanohalophilus. Halanaerobium accounted for 80% or more of the Clostridia sequences in all of the incubations. Halanaerobium are anaerobic, halophilic, alkalophilic, and thermophilic bacteria and thus likely to be adapted to oil and gas wells environments. For example, H. locusroseus can grow in 5–30% NaCl, and at 20–50°C (Cayol et al., 1995). H. congolense in particular has been isolated from a number of oil wells and implicated in well corrosion due to hydrogen sulfide production by thiosulfate reduction (Magot et al., 2000; Ravot et al., 2006). Halanaerobium can use carbohydrates as carbon sources and therefore may have been responsible for the assimilation of glucose under anoxic conditions (Oren, 2002). As a fermenter, Halanaerobium produces acetate and CO2, compounds that may sustain other microorganisms. Halanaerobium spp. have previously been detected in hydrofracturing fluids from separators, storage tanks and impoundments (Davis et al., 2012; Mohan et al., 2013a,b; Akob et al., 2015; Liang et al., 2016; Booker et al., 2017; Lipus et al., 2017).
Fusibacter was a prominent member of the microbial communities throughout this experiment. Fusibacter are also fermenters and can produce acetate by fermenting glucose (Ravot et al., 1999). This genus has been isolated from many hydrocarbon-rich environments and can produce hydrogen sulfide by reducing thiosulfate or elemental sulfur (Ravot et al., 1999; Agrawal et al., 2010; Ben Hania et al., 2012; Smii et al., 2015). Its role in carbon assimilation is likely similar to that of Halanaerobium in that it may use glucose as a carbon or energy source. Interestingly, Fusibacter began to increase in percentage of the community between 0 and 32 days, as did the number of sequences. Possibly, Fusibacter was less sensitive to conditions (e.g., product inhibition) in the microcosms than Halanaerobium. For example, the growth of H. saccharolyticum can be inhibited by an excess of acetate (Kivistö et al., 2012). Because Halanaerobium is an alkaliphile, the production of hydrogen during fermentation may have caused a reduction in pH, making the produced fluids environment in a closed system less habitable. However, some species of Fusibacter are also prone to product inhibition by hydrogen, unless thiosulfate is present (Ravot et al., 1999). The common finding of both Fusibacter and Halanaerobium in oil and gas reservoirs suggests that additional research should be conducted on their presence and potential interactions in these environments.
Halanaerobium and Fusibacter were the most abundant microorganisms, but their presence does not explain metabolic processes observed with carbon sources other than glucose. Minor members of the community may have played a larger part in metabolizing other carbon sources. Appearing in much lower abundance were the genera Anaerophaga, Geotoga, Flexistipes, Asticcacaulis, Carboxydocella, Desulfotomaculum, Methanohalophilus, and a number of unclassified microorganisms. Most of these organisms are halophilic, thermophilic, or both, and should be well adapted to gas well conditions. Representatives of Anaerophaga and Geotoga can ferment glucose and may have also contributed to respiration or assimilation of glucose (Davey et al., 1993; Denger et al., 2002). Flexistipes, a thermophile which requires at least 3% NaCl to grow, can utilize acetate as an energy source (Fiala et al., 1990; Nakano and Zuber, 2004). The presence of Carboxydocella is intriguing as it is known for its use of carbon monoxide (Sokolova et al., 2002; Slepova et al., 2006) which may be present in shales and may be removed by the produced fluid along with methane to the surface. Desulfotomaculum spp. are sulfate reducers often found in oil wells, and one species, D. kuznetsovii, is methylotrophic (Nazina et al., 1988; Nilsen et al., 1996). Recent reconstruction of the genome of Methanohalophilus from produced shale fluids indicated methanogenesis using methanol via methanol:MtaC co-methyltransferase (MtaB) (Daly et al., 2016). Methanohalophilus was detected in all of our incubations; however, the highest atomic % of 13C-CH4 was observed in the methanol-amended incubation (34.54 and 36.13% at 32 and 60 days), strongly indicating methanogenesis by Methanohalophilus in our incubations. These findings are consistent with evidence presented by others showing a prominent role for microbes of this genus in produced fluids (Daly et al., 2016; Borton et al., 2018) and for their ability to use methylated compounds (Mathrani et al., 1988).
Conclusion
We found that microorganisms in incubations of produced fluid from a hydrofractured shale were revivable in laboratory incubations and retained the ability to metabolize added substrates catabolically and anabolically. When incubated anoxically, microbial communities were active and responsive to diverse carbon substrates. Following anoxic incubations, microbial communities were not diverse and many of the genera observed were related to microbes that have previously been detected in hydraulically fractured shale fluids or similar environments and that possess qualities such as resistance to high salinity, heat, and the presence of metals. An ideal sample set to examine microbial properties of shales would involve collecting and analyzing subsurface cores with attention to sampling technique to prevent microbial contamination. Such an effort would verify whether microbes that we studied and that others have detected exist in and are active in these formations. The microbes that we examined in the produced fluid were at least fractionally alive and may have the metabolic potential to affect subsurface geochemistry and to consume organics found in the subsurface or delivered during the hydraulic fracturing process.
Author Contributions
JW, MT, CV, and FC designed the project. JW collected the samples. JW, YM, MI, AI, TH, and TT conducted the experiments, analyzed the samples, and interpreted the data. JW, YM, AI, FI, and FC wrote the manuscript.
Funding
This research was supported by grants from the National Energy Technology Laboratory Oak Ridge Institute for Science and Education (ORISE) fellowship, the National Science Foundation East Asia and Pacific Summer Institutes (EAPSI) fellowship, and the Japan Society for the Promotion of Science Summer Program.
Disclaimer
This report was prepared as an account of work sponsored by an agency of the United States Government. Neither the United States Government nor any agency thereof, nor any of their employees, makes any warranty, express or implied, or assumes any legal liability or responsibility for the accuracy, completeness, or usefulness of any information, apparatus, product, or process disclosed, or represents that its use would not infringe privately owned rights. Reference therein to any specific commercial product, process, or service by trade name, trademark, manufacturer, or otherwise does not necessarily constitute or imply its endorsement, recommendation, or favoring by the United States Government or any agency thereof. The views and opinions of authors expressed therein do not necessarily state or reflect those of the United States Government or any agency thereof.
Conflict of Interest Statement
The authors declare that the research was conducted in the absence of any commercial or financial relationships that could be construed as a potential conflict of interest.
Acknowledgments
We thank the staff at the Japan Agency for Marine-Earth Science and Technology (JAMSTEC) for their help with isotopic and microbiological analyses. We also thank Harry Edenborn (National Energy Technology Laboratory) for providing the produced fluid samples and Sae Fukunaga (JAMSTEC) for conducting the cell counts.
Supplementary Material
The Supplementary Material for this article can be found online at: https://www.frontiersin.org/articles/10.3389/fmicb.2019.00376/full#supplementary-material
References
Agrawal, A., Vanbroekhoven, K., and Lal, B. (2010). Diversity of culturable sulfidogenic bacteria in two oil-water separation tanks in the north-eastern oil fields of India. Anaerobe 16, 12–18. doi: 10.1016/j.anaerobe.2009.04.005
Akob, D. M., Cozzarelli, I. M., Dunlap, D. S., Rowan, E. L., and Lorah, M. M. (2015). Organic and inorganic composition and microbiology of produced waters from pennsylvania shale gas wells. Appl. Geochem. 60, 116–125. doi: 10.1016/j.apgeochem.2015.04.011
Arthur, J. D., Bohm, B., Coughlin, B., and Layne, M. (2008). Hydraulic Fracturing Considerations For Natural Gas Well of the Fayetteville Shale. Available at: http://www.aogc2.state.ar.us/OnlineData/reports/ALL FayettevilleFrac FINAL.pdf (Accessed March 10, 2016).
Barbot, E., Vidic, N. S., Gregory, K. B., and Vidic, R. D. (2013). Spatial and temporal correlation of water quality parameters of produced waters from Devonian-age shale following hydraulic fracturing. Environ. Sci. Technol. 47, 2562–2569. doi: 10.1021/es304638h
Ben Hania, W., Fraj, B., Postec, A., Fadhlaoui, K., Hamdi, M., Ollivier, B., et al. (2012). Fusibacter tunisiensis sp. nov., isolated from an anaerobic reactor used to treat olive-mill wastewater. Int. J. Syst. Evol. Microbiol. 62, 1365–1368. doi: 10.1099/ijs.0.034603-0
Blauch, M. E., Myers, R. R., Moore, T., Lipinski, B. A., and Houston, N. A. (2009). Marcellus Shale Post-Frac Flowback Waters - Where Is All The Salt Coming From and What are the Implications? in SPE Eastern Regional Meeting. Charleston: Society of Petroleum Engineers. doi: 10.2118/125740-MS
Booker, A. E., Borton, M. A., Daly, R. A., Welch, S. A., Nicora, C. D., Hoyt, D. W., et al. (2017). Sulfide generation by dominant Halanaerobium microorganisms in hydraulically fractured shales. mSphere 2, e00257–e00317. doi: 10.1128/mSphereDirect.00257-17
Borton, M. A., Hoyt, D. W., Roux, S., Daly, R. A., Welch, S. A., Nicora, C. D., et al. (2018). Coupled laboratory and field investigations resolve microbial interactions that underpin persistence in hydraulically fractured shales. Proc. Natl. Acad. Sci. U S A. 115, E6585–E6594. doi: 10.1073/pnas.1800155115
Boschee, P. (2015). Produced and flowback water recycling and reuse: economics, limitations, and technology. Oil Gas Facil. 3, 16–21. doi: 10.2118/0214-0016-OGF
Bottero, S., Picioreanu, C., Delft, T. U., Enzien, M., Control, D. M., Loosdrecht, M. V., et al. (2010). “Formation damage and impact on gas flow caused by biofilms growing within proppant packing used in hydraulic fracturing,” in Proceedings of the SPE International Symposium on Formation Damage Control, SPE-128066, (Lafayette: Society of Petroleum Engineers). doi: 10.2118/128066-MS
Cayol, J. L., Ollivier, B., Patel, B. K., Ageron, E., Grimont, P. A., Prensier, G., et al. (1995). Haloanaerobium lacusroseus sp. nov., an extremely halophilic fermentative bacterium from the sediments of a hypersaline lake. Int. J. Syst. Bacteriol. 45, 790–797. doi: 10.1099/00207713-45-4-790
Chistoserdova, L. (2011). Modularity of methylotrophy, revisited. Environ. Microbiol. 13, 2603–2622. doi: 10.1111/j.1462-2920.2011.02464.x
Cluff, M. A., Hartsock, A., MacRae, J. D., Carter, K., and Mouser, P. J. (2014). Temporal changes in microbial ecology and geochemistry in produced water from hydraulically fractured marcellus shale gas wells. Environ. Sci. Technol. 48, 6508–6517. doi: 10.1021/es501173p
Colwell, F. S., Onstott, T. C., Delwiche, M. E., Chandler, D., Fredrickson, J. K., Yao, Q.-J., et al. (1997). Microorganisms from deep, high temperature sandstones: constraints on microbial colonization. FEMS Microbiol. Rev. 20, 425–435. doi: 10.1111/j.1574-6976.1997.tb00327.x
Cord-Ruwisch, R., Kleinitz, W., and Widdel, F. (2013). Sulfate-reducing bacteria and their activities in oil production. J. Pet. Technol. 39, 97–106. doi: 10.2118/13554-PA
Daly, R. A., Borton, M. A., Wilkins, M. J., Hoyt, D. W., Kountz, D. J., Wolfe, R. A., et al. (2016). Microbial metabolisms in a 2.5-km-deep ecosystem created by hydraulic fracturing in shales. Nat. Microbiol. 1:16146. doi: 10.1038/nmicrobiol.2016.146
Davey, M. E., Wood, W. A., Key, R., Nakamura, K., and Stahl, D. A. (1993). Isolation of three species of geotoga and petrotoga: two new genera, representing a new lineage in the bacterial line of descent distantly related to the “thermotogales.”. Syst. Appl. Microbiol. 16, 191–200. doi: 10.1016/S0723-2020(11)80467-4
Davis, J. P., Struchtemeyer, C. G., and Elshahed, M. S. (2012). Bacterial communities associated with production facilities of two newly drilled thermogenic natural gas wells in the barnett shale (texas, USA). Microb. Ecol. 64, 942–954. doi: 10.1007/s00248-012-0073-3
Denger, K., Warthmann, R., Ludwig, W., and Schink, B. (2002). Anaerophaga thermohalophila gen. nov., sp. nov., a moderately thermohalophilic, strictly anaerobic fermentative bacterium. Int. J. Syst. Evol. Microbiol. 52, 173–178. doi: 10.1099/00207713-52-1-173
Desai, J., and Banat, I. (1997). Microbial production of surfactants and their commercial potential. Microbiol. Mol. Biol. Rev. 61, 47–64.
Dresel, E., and Rose, A. W. (2010). Chemistry and Origin of Oil and Gas Well Brines in Western PENNSYLVANIA. Pennsylvania Geological Survey, 4th series Open-File Report OFOG 10-01.0. State College, PA, Penn State University.
Enning, D., and Garrelfs, J. (2014). Corrosion of iron by sulfate-reducing bacteria: new views of an old problem. Appl. Environ. Microbiol. 80, 1226–1236. doi: 10.1128/AEM.02848-13
Ensign, S. A. (2006). Revisiting the glyoxylate cycle: alternate pathways for microbial acetate assimilation. Mol. Microbiol. 61, 274–276. doi: 10.1111/j.1365-2958.2006.05247.x
Environmental Protection Agency [EPA]. (2012). Study of the Potential Impacts of Hydraulic Fracturing On Drinking Water Resources. Progress Report. (EPA/601/R-12/011). Washington, DC: U.S.Environmental Protection Agency, Office of Research and Development.
Fiala, G., Woese, C. R., Langworthy, T. A., and Stetter, K. O. (1990). Flexistipes sinusarabici, a novel genus and species of eubacteria occurring in the atlantis II deep brines of the red sea. Arch. Microbiol. 154, 120–126. doi: 10.1007/BF00423320
FracFocus Chemical Disclosure Registry. (2016). FracFocus 3.0. Available at: http://fracfocus.org/ (Accessed January 14, 2016).
Fredrickson, J. K., McKinley, J. P., Bjornstad, B. N., Long, P. E., Ringelberg, D. B., White, D. C., et al. (1997). Pore-size constraints on the activity and survival of subsurface bacteria in a late cretaceous shale-sandstone sequence. northwestern new mexico. Geomicrobiol. J. 14, 183–202. doi: 10.1080/01490459709378043
Gale, J. F. W., and Holder, J. (2010). Natural fractures in some US shales and their importance for gas production. Petrol. Geol. Conf. Proc. 7, 1131–1140. doi: 10.1144/0071131
Gormanns, P., Reckow, S., Poczatek, J. C., Turck, C. W., and Lechene, C. (2012). Segmentation of Multi-Isotope Imaging Mass Spectrometry Data for Semi-Automatic Detection of Regions of Interest. PLoS One 7:e30576. doi: 10.1371/journal.pone.0030576
Gregory, K. B., Vidic, R. D., and Dzombak, D. (2011). Water management challenges associated with the production of shale gas by hydraulic fracturing. Elements 7, 181–186. doi: 10.2113/gselements.7.3.181
Haluszczak, L. O., Rose, A. W., and Kump, L. R. (2013). Geochemical evaluation of flowback brine from marcellus gas wells in pennsylvania. U S A. Appl. Geochem. 28, 55–61. doi: 10.1016/j.apgeochem.2012.10.002
Hoehler, T. M. (2007). An energy balance concept for habitability. Astrobiology 7, 824–838. doi: 10.1089/ast.2006.0095
Horsfield, B., Schenk, H., Zink, K., Ondrak, R., Dieckmann, V., Kallmeyer, J., et al. (2006). Living microbial ecosystems within the active zone of catagenesis: implications for feeding the deep biosphere. Earth Planet. Sci. Lett. 246, 55–69. doi: 10.1016/j.epsl.2006.03.040
Jenkins, C. D., and Li, C. M. B. (2008). Coalbed- and shale-gas reservoirs. J. Petrol. Technol. 60, 92–99. doi: 10.2118/103514-JPT
Kahrilas, G., Blotevogel, J., Stewart, P. S., and Borch, T. (2015). Biocides in hydraulic fracturing fluids: a critical review of their usage, mobility, degradation, and toxicity. Environ. Sci. Technol. 49, 16–32. doi: 10.1021/es503724k
Kargbo, D. M., Wilhelm, R. G., and Campbell, D. J. (2010). Natural gas plays in the marcellus shale: challenges and potential opportunities. Environ. Sci. Technol. 44, 5679–5684. doi: 10.1021/es903811p
Kirchman, D. L., Keil, R. G., and Wheeler, P. A. (1989). The effect of amino acids on ammonium utilization and regeneration by heterotrophic bacteria in the subarctic pacific. Deep. Res. 36, 1763–1776. doi: 10.1016/0198-0149(89)90071-X
Kivistö, A., Santala, V., and Karp, M. (2012). 1,3-propanediol production and tolerance of a halophilic fermentative bacterium, Halanaerobium saccharolyticum subsp. saccharolyticum. J. Biotechnol. 158, 242–247. doi: 10.1016/j.jbiotec.2011.10.013
Lester, Y., Ferrer, I., Thurman, E. M., Sitterley, K., Korak, J., Aiken, G., et al. (2015). Characterization of hydraulic fracturing flowback water in colorado: implications for water treatment. Sci. Total Environ. 51, 637–644. doi: 10.1016/j.scitotenv.2015.01.043
Lester, Y., Yacob, T., Morrissey, I., and Linden, K. G. (2014). Can we treat hydraulic fracturing flowback with a conventional biological process? The case of guar gum. Environ. Sci. Technol. Lett. 1, 133–136. doi: 10.1021/ez4000115
Liang, R., Davidova, I. A., Marks, C. R., Stamps, B. W., Harriman, B. H., Stevenson, B. S., et al. (2016). Metabolic capability of a predominant Halanaerobium sp. in hydraulically fractured gas wells and its implication in pipeline corrosion. Front. Microbiol. 7:988. doi: 10.3389/fmicb.2016.00988
Lipus, D., Vikram, A., Ross, D., Bain, D., Gulliver, D., Hammack, R., et al. (2017). Predominance and metabolic potential of Halanaerobium spp. in produced water from hydraulically fractured marcellus shale wells. Appl. Environ. Microbiol. 83, e2659–e2716. doi: 10.1128/AEM.02659-16
Lovley, D. R., and Chapelle, F. H. (1995). Deep subsurface microbial processes. Rev. Geophys. 33, 365–381. doi: 10.1029/95RG01305
Lutz, B. D., Lewis, A. N., and Doyle, M. W. (2013). Generation, transport, and disposal of wastewater associated with marcellus shale gas development. Water Resour. Res. 49, 647–656. doi: 10.1002/wrcr.20096
Magot, M., Ollivier, B., and Patel, B. K. C. (2000). Microbiology of petroleum reservoirs. Antonie Van Leeuwenhoek 77, 103–116. doi: 10.1023/A:1002434330514
Mah, T.-F. C., and O’Toole, G. A. (2001). Mechanisms of biofilm resistance to antimicrobial agents. Trends Microbiol. 9, 34–39. doi: 10.1016/S0966-842X(00)01913-2
Mathrani, I. M., Boone, D. R., Mah, R. A., Fox, G. E., and Lau, P. P. (1988). Methanohalophilus zhilinae sp. nov., an alkaliphilic, halophilic, methylotrophic methanogen. Int. J. Syst. Bacteriol. 38, 139–142. doi: 10.1099/00207713-38-2-139
Mohan, A. M., Bibby, K. J., Lipus, D., Hammack, R. W., and Gregory, K. B. (2014). The functional potential of microbial communities in hydraulic fracturing source water and produced water from natural gas extraction characterized by metagenomic sequencing. PLoS One 9:e107682. doi: 10.1371/journal.pone.0107682
Mohan, A. M., Gregory, K., Vidic, R., Miller, P., and Hammack, R. (2011). “Characterization of microbial diversity in treated and untreated flowback water impoundments from gas fracturing operations,” in Proceedings of the SPE Annual Technical Conference and Exhibition. SPE-147414-MS, (Denver: Society of Petroleum Engineers).
Mohan, A. M., Hartsock, A., Bibby, K. J., Hammack, R. W., Vidic, R. D., and Gregory, K. B. (2013a). Microbial community changes in hydraulic fracturing fluids and produced water from shale gas extraction. Environ. Sci. Technol. 47, 13141–13150. doi: 10.1021/es402928b
Mohan, A. M., Hartsock, A., Hammack, R. W., Vidic, R. D., and Gregory, K. B. (2013b). Microbial communities in flowback water impoundments from hydraulic fracturing for recovery of shale gas. FEMS Microbiol. Ecol. 86, 567–580. doi: 10.1111/1574-6941.12183
Morono, Y., and Inagaki, F. (2010). Automatic slide-loader fluorescence microscope for discriminative enumeration of subseafloor life. Sci. Drill. 9, 32–36. doi: 10.5194/sd-9-32-2010
Morono, Y., Terada, T., Hoshino, T., and Inagaki, F. (2014). Hot-alkaline DNA extraction method for deep-subseafloor archaeal communities. Appl. Environ. Microbiol. 80, 1985–1994. doi: 10.1128/AEM.04150-13
Morono, Y., Terada, T., Kallmeyer, J., and Inagaki, F. (2013). An improved cell separation technique for marine subsurface sediments: applications for high-throughput analysis using flow cytometry and cell sorting. Environ. Microbiol. 15, 2841–2849. doi: 10.1111/1462-2920.12153
Morono, Y., Terada, T., Nishizawa, M., Ito, M., Hillion, F., Takahata, N., et al. (2011). Carbon and nitrogen assimilation in deep subseafloor microbial cells. Proc. Natl. Acad. Sci. 108, 18295–18300. doi: 10.1073/pnas.1107763108
Morozkina, E. V., Slutskaia, E. S., Fedorova, T. V., Tugaǐ, T. I., Golubeva, L. I., and Koroleva, O. V. (2010). Extremophilic microorganisms: biochemical adaptation and biotechnological application (review). Prikl. Biokhim. Mikrobiol. 46, 5–20. doi: 10.1134/S0003683810010011
Nakano, M. M., and Zuber, P. (2004). Strict and Facultative Anaerobes: Medical and Environmental Aspects. Wymondham: CRC Press. doi: 10.1201/9781482292503
Nazina, T., Ivanova, A., Kanchaveli, L., and Rozanova, E. (1988). Desulfotomaculum kuznetsovii sp. nov., a new spore-forming thermophilic methylotrophic sulfate-reducing bacterium. Mikrobiologiya 57, 823–827.
Nazina, T. N., Ivanova, A. E., Golubeva, O. V., Ibatullin, R. R., Belyaev, S. S., and Ivanov, M. V. (1995). Occurrence of sulfate- and iron-reducing bacteria in stratal waters of the romashkinskoe oil field. Microbiology 64, 245–251.
Nicot, J.-P., and Scanlon, B. R. (2012). Water use for shale gas production in texas. U.S. Environ. Sci. Technol. 46, 3580–3586. doi: 10.1021/es204602t
Nilsen, R. K., Torsvik, T., and Lien, T. (1996). Desulfotomaculum thermocisternum sp. nov., a sulfate reducer isolated from a hot north sea oil reservoir. Int. J. Syst. Bacteriol. 46, 397–402. doi: 10.1099/00207713-46-2-397
Olmstead, S. M., Muehlenbachs, L., Shih, J., Chu, Z., and Krupnick, A. J. (2013). Shale gas development impacts on surface water quality in pennsylvania. Proc. Natl. Acad. Sci. U SA. 110, 4962–4967. doi: 10.1073/pnas.1213871110
Orberger, B., Gallien, J.-P., Pinti, D. L., Fialin, M., Daudin, L., Gröcke, D. R., et al. (2005). Nitrogen and carbon partitioning in diagenetic and hydrothermal minerals from paleozoic black shales, (selwyn basin, yukon territories, canada). Chem. Geol. 218, 249–264. doi: 10.1016/j.chemgeo.2005.01.012
Orcutt, B. N., LaRowe, D. E., Biddle, J. F., Colwell, F. S., Glazer, B. T., Reese, B. K., et al. (2013). Microbial activity in the marine deep biosphere: progress and prospects. Front. Microbiol. 4:189. doi: 10.3389/fmicb.2013.00189
Oren, A. (2002). Halophilic Microorganisms and their Environments. Dordrecht: Kluwer Scientific Publishers. doi: 10.1007/0-306-48053-0
Patel, G. B., and Roth, L. A. (1977). Effect of sodium chloride on growth and methane production of methanogens. Can. J. Microbiol. 23, 893–897. doi: 10.1139/m77-131
Postma, D., and Jakobsen, R. (1996). Redox zonation: equilibrium constraints on the Fe(III)/SO4-reduction interface. Geochim. Cosmochim. Acta 60, 3169–3175. doi: 10.1016/0016-7037(96)00156-1
Puspita, I. D., Kamagata, Y., Tanaka, M., Asano, K., and Nakatsu, C. H. (2012). Are uncultivated bacteria really uncultivable? Microb. Environ. 27, 356–366. doi: 10.1264/jsme2.ME12092
Quast, C., Pruesse, E., Yilmaz, P., Gerken, J., Schweer, T., Yarza, P., et al. (2013). The SILVA ribosomal RNA gene database project: improved data processing and web-based tools. Nucl. Acids Res. 41, D590–D596. doi: 10.1093/nar/gks1219
Rahm, B. G., Bates, J. T., Bertoia, L. R., Galford, A. E., Yoxtheimer, D. A., and Riha, S. J. (2013). Wastewater management and marcellus shale gas development: Trends. drivers, and planning implications. J. Environ. Manag. 120, 105–113. doi: 10.1016/j.jenvman.2013.02.029
Rappé, M. S., and Giovannoni, S. J. (2003). The uncultured microbial majority. Annu. Rev. Microbiol. 57, 369–394. doi: 10.1146/annurev.micro.57.030502.090759
Ravot, G., Magot, M., Fardeau, M.-L., Patel, B. K. C., Thomas, P., Garcia, J.-L., et al. (1999). Fusibacter paucivorans gen. nov., sp. nov., an anerobic, thiosulfate-reducing bacterium from an oil-producing well. J. Syst. Bacteriol. 49, 1141–1147. doi: 10.1099/00207713-49-3-1141
Ravot, G., Magot, M., Ollivier, B., Patel, B. K., Ageron, E., Grimont, P. A., et al. (2006). Haloanaerobium congolense sp. nov., an anaerobic, moderately halophilic, thiosulfate- and sulfur-reducing bacterium from an African oil field. FEMS Microbiol. Lett. 147, 81–88. doi: 10.1111/j.1574-6968.1997.tb10224.x
Röling, W. F. M., Head, I. M., and Larter, S. R. (2003). The microbiology of hydrocarbon degradation in subsurface petroleum reservoirs: perspectives and prospects. Res. Microbiol. 154, 321–328. doi: 10.1016/S0923-2508(03)00086-X
Romano, A. H., Eberhard, S. J., Dingle, S. L., and McDowell, T. D. (1970). Distribution of the phosphoenolpyruvate:glucose phosphotransferase system in bacteria. J. Bacteriol. 104, 808–813.
Romano, A. H., Trifone, J. D., and Brustolon, M. (1979). Distribution of the phosphoenolpyruvate:glucose phosphotransferase system in fermentative bacteria. J. Bacteriol. 139, 93–97.
Roseman, S. (1969). The transport of carbohydrates by a bacterial phosphotransferase system. J. Gen. Physiol. 54, 138–184. doi: 10.1085/jgp.54.1.138
Salter, S. J., Cox, M. J., Turek, E. M., Calus, S. T., Cookson, W. O., Moffatt, M. F., et al. (2014). Reagent and laboratory contamination can critically impact sequence-based microbiome analyses. BMC Biol. 12:87. doi: 10.1186/s12915-014-0087-z
Schindelin, J., Arganda-Carreras, I., Frise, E., Kaynig, V., Longair, M., Pietzsch, T., et al. (2012). Fiji: an open-source platform for biological-image analysis. Nat. Methods 9, 676–682. doi: 10.1038/nmeth.2019
Schneider, C. A., Rasband, W. S., and Eliceiri, K. W. (2012). NIH Image to ImageJ: 25 years of image analysis. Nat. Methods 9, 671–675. doi: 10.1038/nmeth.2089
Schloss, P. D., Westcott, S. L., Ryabin, T., Hall, J. R., Hartmann, M., Hollister, E. B., et al. (2009). Introducing mothur: open-source, platform-independent, community-supported software for describing and comparing microbial communities. Appl. Environ. Microbiol. 75, 7537–7541. doi: 10.1128/AEM.01541-09
Slepova, T. V., Sokolova, T. G., Lysenko, A. M., Tourova, T. P., Kolganova, T. V., Kamzolkina, O. V., et al. (2006). Carboxydocella sporoproducens sp. nov., a novel anaerobic CO-utilizing/H2-producing thermophilic bacterium from a kamchatka hot spring. Int. J. Syst. Evol. Microbiol. 56, 797–800. doi: 10.1099/ijs.0.63961-0
Smii, L., Ben Hania, W., Cayol, J.-L., Joseph, M., Hamdi, M., Ollivier, B., et al. (2015). Fusibacter bizertensis sp. nov., isolated from a corroded kerosene storage tank. Int. J. Syst. Evol. Microbiol. 65, 117–121. doi: 10.1099/ijs.0.066183-0
Sokolova, T. G., Kostrikina, N. A., Chernyh, N. A., Tourova, T. P., Kolganova, T. V., and Bonch-Osmolovskaya, E. A. (2002). Carboxydocella thermautotrophica gen. nov., sp. nov., a novel anaerobic, CO-utilizing thermophile from a kamchatkan hot spring. Int. J. Syst. Evol. Microbiol. 52, 1961–1967. doi: 10.1099/00207713-52-6-1961
Stringfellow, W. T., Domen, J. K., Camarillo, M. K., Sandelin, W. L., and Borglin, S. (2014). Physical, chemical, and biological characteristics of compounds used in hydraulic fracturing. J. Hazard. Mater. 275, 37–54. doi: 10.1016/j.jhazmat.2014.04.040
Strong, L. C., Gould, T., Kasinkas, L., Sadowsky, M. J., Aksan, A., and Wackett, L. P. (2013). Biodegradation in waters from hydraulic fracturing: chemistry, microbiology, and engineering. J. Environ. Eng. 140:B4013001 doi: 10.1061/(ASCE)EE.1943-7870.0000792
Struchtemeyer, C. G., Davis, J. P., and Elshahed, M. S. (2011). Influence of the drilling mud formulation process on the bacterial communities in thermogenic natural gas wells of the barnett shale. Appl. Environ. Microbiol. 77, 4744–4753. doi: 10.1128/AEM.00233-11
Struchtemeyer, C. G., and Elshahed, M. S. (2012). Bacterial communities associated with hydraulic fracturing fluids in thermogenic natural gas wells in north central texas. U S A. FEMS Microbiol. Ecol. 81, 13–25. doi: 10.1111/j.1574-6941.2011.01196.x
Struchtemeyer, C. G., Morrison, M. D., and Elshahed, M. S. (2012). A critical assessment of the efficacy of biocides used during the hydraulic fracturing process in shale natural gas wells. Int. Biodeterior. Biodegr. 71, 15–21. doi: 10.1016/j.ibiod.2012.01.013
Waldron, P. J., Petsch, S. T., Martini, A. M., Nüsslein, K., and Nüslein, K. (2007). Salinity constraints on subsurface archaeal diversity and methanogenesis in sedimentary rock rich in organic matter. Appl. Environ. Microbiol. 73, 4171–4179. doi: 10.1128/AEM.02810-06
Warner, N. R., Jackson, R. B., Darrah, T. H., Osborn, S. G., Down, A., Zhao, K., et al. (2012). Geochemical evidence for possible natural migration of marcellus Formation brine to shallow aquifers in pennsylvania. Proc. Natl. Acad. Sci. U. S. A. 109, 11961–11966. doi: 10.1073/pnas.1121181109
Keywords: NanoSIMS, hydraulic fracturing, microbial metabolism, microbial community, N and C assimilation
Citation: Morono Y, Wishart JR, Ito M, Ijiri A, Hoshino T, Torres M, Verba C, Terada T, Inagaki F and Colwell FS (2019) Microbial Metabolism and Community Dynamics in Hydraulic Fracturing Fluids Recovered From Deep Hydrocarbon-Rich Shale. Front. Microbiol. 10:376. doi: 10.3389/fmicb.2019.00376
Received: 26 June 2018; Accepted: 13 February 2019;
Published: 12 March 2019.
Edited by:
Yiran Dong, China University of Geosciences Wuhan, ChinaReviewed by:
Theodore M. Flynn, Argonne National Laboratory (DOE), United StatesMichael J. Wilkins, The Ohio State University, United States
Copyright © 2019 Morono, Wishart, Ito, Ijiri, Hoshino, Torres, Verba, Terada, Inagaki and Colwell. This is an open-access article distributed under the terms of the Creative Commons Attribution License (CC BY). The use, distribution or reproduction in other forums is permitted, provided the original author(s) and the copyright owner(s)are credited and that the original publication in this journal is cited, in accordance with accepted academic practice. No use, distribution or reproduction is permitted which does not comply with these terms.
*Correspondence: Frederick S. Colwell, cmNvbHdlbGxAY29hcy5vcmVnb25zdGF0ZS5lZHU=