- 1School of Pharmacy and Biomedical Sciences, Curtin Health Innovation Research Institute, Curtin University, Perth, WA, Australia
- 2CSIRO Land and Water, Floreat, WA, Australia
- 3Center for Bioinformatics and Genome Biology, Science for Life Foundation, Santiago, Chile
- 4Sodexo Australia, Perth, WA, Australia
- 5Centro de Genómica y Bioinformática, Facultad de Ciencias, Universidad Mayor, Santiago, Chile
There are few naturally occurring environments where both acid and salinity stress exist together, consequently, there has been little evolutionary pressure for microorganisms to develop systems that enable them to deal with both stresses simultaneously. Members of the genus Acidihalobacter are iron- and sulfur-oxidizing, halotolerant acidophiles that have developed the ability to tolerate acid and saline stress and, therefore, have the potential to bioleach ores with brackish or saline process waters under acidic conditions. The genus consists of four members, A. prosperus DSM 5130T, A. prosperus DSM 14174, A. prosperus F5 and “A. ferrooxidans” DSM 14175. An in depth genome comparison was undertaken in order to provide a more comprehensive description of the mechanisms of halotolerance used by the different members of this genus. Pangenome analysis identified 29, 3 and 9 protein families related to halotolerance in the core, dispensable and unique genomes, respectively. The genes for halotolerance showed Ka/Ks ratios between 0 and 0.2, confirming that they are conserved and stabilized. All the Acidihalobacter genomes contained similar genes for the synthesis and transport of ectoine, which was recently found to be the dominant osmoprotectant in A. prosperus DSM 14174 and A. prosperus DSM 5130T. Similarities also existed in genes encoding low affinity potassium pumps, however, A. prosperus DSM 14174 was also found to contain genes encoding high affinity potassium pumps. Furthermore, only A. prosperus DSM 5130T and “A. ferrooxidans” DSM 14175 contained genes allowing the uptake of taurine as an osmoprotectant. Variations were also seen in genes encoding proteins involved in the synthesis and/or transport of periplasmic glucans, sucrose, proline, and glycine betaine. This suggests that versatility exists in the Acidihalobacter genus in terms of the mechanisms they can use for halotolerance. This information is useful for developing hypotheses for the search for life on exoplanets and moons.
Introduction
The most extensively studied acidophiles are those that oxidize iron and/or sulfur for the catalytic dissolution of minerals in low pH environments (Johnson and Schippers, 2017). These microorganisms can be used in biomining, i.e., for the economic extraction of metals from low grade ores, which are otherwise too expensive to process through traditional mining processes such as smelting or roasting (Rohwerder et al., 2003).
In regions like Western Australia and Chile, groundwater is often limited, and seawater may be used for bioleaching operations. Moreover, with decreasing ore grades, mining companies are moving toward the use of low-grade, more complex ores, which may contain impurities, such as chloride. High concentration of chloride in process waters inhibits the growth of acidophiles, decreasing the bioleaching rates and yields. Desalination can be used to remove chloride ion but this is prohibitively expensive (Petry et al., 2007; Watling, 2016; Zammit and Watkin, 2016). While anions such as sulfate and cations such as sodium ions limit the growth of acidophiles due to their ability to cause osmotic stress, the biggest challenge to biomining operations using seawater is caused by chloride ions. These ions can cross the cell membrane and cause acidification of the cytoplasm by disrupting the reverse transmembrane potential and thereby inhibiting cell growth and ultimately causing cell death (Blight and Ralph, 2004; Shiers et al., 2005; Davis-Belmar et al., 2008; Rea et al., 2015; Boxall et al., 2016). Therefore, the discovery and characterization of halophilic acidophiles that can tolerate chloride ion concentrations greater than the 19 g/L present in seawater is important to the mining industry as they offer a means of leaching base metals with saline water and from high-salt ores (Zammit et al., 2009; Watling, 2016).
Most studies on the halotolerance of microorganisms at low pH have been conducted on pathogens that only have brief exposure to acid stress (Zammit and Watkin, 2016). Likewise, most of the studies on extreme halophiles have been undertaken under neutral or alkaline conditions (Empadinhas and da Costa, 2008). The combination of high salinity and low pH drastically reduces the number of organisms which can survive in this ecological niche. Despite the ongoing search for halotolerant acidophiles over the past 20 years, only a few microorganisms that are capable of oxidizing iron and sulfur in the presence of salt and acid stress simultaneously have been identified (Watling, 2016; Zammit and Watkin, 2016). This is because there are only a few geographical locations where both low pH and high salt environments exist, such as acidic saline lakes and drains and volcanoes near seawater (Zammit and Watkin, 2016). Members of the acidophilic and halotolerant species of the Acidihalobacter genus represent a group of Gram-negative, halophilic, iron- and sulfur-oxidizing, mesophilic, chemolithoautotrophic, extreme acidophiles that have been isolated from these unique environments (Huber and Stetter, 1989; Simmons and Norris, 2002; Zammit et al., 2009). To date, only four members of the Acidihalobacter genus have been characterized. The first member of the genus to be identified was A. prosperus DSM 5130T, isolated from a geothermal heated seafloor at Porto di Levante, Vulcano, Italy and shown to have a chloride ion tolerance of 35 g/L (Huber and Stetter, 1989). More recently, A. prosperus DSM 14174 and “A. ferrooxidans” DSM 14175 were isolated from hydrothermal pools at the Aeolian Islands, Vulcano, Italy (Simmons and Norris, 2002). Another isolate, A. prosperus F5 was the first of this species to be isolated in Australia from a mixed environmental culture obtained from an acidic saline drain (Zammit et al., 2009). All three of these isolates were found to tolerate up to 45 g/L chloride ion and to leach base metals from pyrite at up to 30 g/L chloride ion (Khaleque et al., 2017a,b). Furthermore, A. prosperus DSM 14174 was able to leach copper from a copper containing ore at 30 g/L chloride ion in the presence of “A. ferrooxidans” DSM 14175 and other salt-tolerant acidophiles (Davis-Belmar et al., 2008). Additionally, a pure culture of A. prosperus F5 could leach chalcopyrite at 18 g/L chloride ion and pentlandite at 45 g/L chloride ion (Khaleque et al., 2017a). The ability of these microorganisms to release metals from insoluble ores in the presence of acid and salt stress make them worthy candidates for elucidation of the mechanisms of salt stress tolerance in acidophiles.
Genome sequencing can be an important first step in characterizing a new organism as it provides critical genetic information required to elucidate biochemical pathways underpinning its metabolic capabilities and survival mechanisms. Several acidophiles have been sequenced and comparative genomics has shed light on their metabolic processes (Tyson et al., 2004; Levicán et al., 2008; Valdés et al., 2008, 2010; Zhou et al., 2008; Cárdenas et al., 2010, 2012; Zhang et al., 2016a,b; Tran et al., 2017). The sequencing of the genomes of the members of the Acidihalobacter genus (Ossandon et al., 2014; Khaleque et al., 2017a,b,c) has provided an opportunity to better study the mechanisms of survival used by these acidophilic, halotolerant acidophiles.
In this study, comparative genomic analysis of all members of the Acidihalobacter genus was used to enhance the understanding of the mechanisms these acidophiles employ to tolerate salt stress.
Materials and Methods
Acidihalobacter Genome Sequencing, Annotation and Comparisons
Genome sequencing and assembly were performed as previously described (Ossandon et al., 2014; Khaleque et al., 2017a,b,c). Genome sequences have previously been deposited at DDBJ/ENA/GenBank with the following accession numbers: A. prosperus DSM 5130T (JQSG00000000.2), A. prosperus F5 (CP017415.1), A. prosperus DSM 14174 (CP017448.1) and “A. ferrooxidans” DSM 14175 (CP019434.1).
The genome sequences were annotated using Rapid Annotation using Subsystem Technology (RAST) server1 using the ClassicRAST annotation scheme (Aziz et al., 2008; Overbeek et al., 2014). Metabolic pathways were predicted by Kyoto Encyclopedia of Genes and Genomes (KEGG)2. Whole genomes were aligned and compared by the construction of a circular genomic map for each genome using the BLAST Ring Image Generator (BRIG, version 0.95) as described by Alikhan et al. (2011). The complete genome sequence of A. prosperus F5 was used as the reference sequence for the Acidihalobacter genus. The genome maps were drawn on a local BLAST basis using an E-value of 1e-5 and upper and lower identity threshold percentages of 70% and 50%, respectively.
Predicted protein sequences corresponding to all Acidihalobacter proteomes were sorted using an all-vs-all BLASTP script based on Best Bidirectional BLAST Hit (BBBH; Altschul et al., 1997) with an E-value cut off of 1e-5. Protein families were constructed based on 50% of identity and coverage of alignments, assigning each protein to one protein family (Snipen and Ussery, 2010). The protein families were classified in core-, dispensable- and unique-genome.
Identification of Genes Involved in Halotolerance in Acidihalobacter
Potential mechanisms of halotolerance were identified through an extensive literature search for mechanisms related to halotolerance that have previously been identified in other microorganisms. These genes were then searched in Acidihalobacter species genomes through manual curation and by BLAST comparisons (minimal E-value of 1e-5) using Geneious v.8.1.8 bioinformatics software (Kearse et al., 2012; Overbeek et al., 2014). Synteny blocks between Acidihalobacter genomes and conservation of gene neighbors were determined by MAUVE (Darling et al., 2010). Genomic contexts were visualized using Artemis (Rutherford et al., 2000), the RAST server (Aziz et al., 2008) and Geneious v. 8.1.8 software (Kearse et al., 2012; Overbeek et al., 2014).
Amino acid alignments of families related to halotolerance were aligned using MUSCLE (Edgar, 2004). The alignments were used as input for PAL2NAL (Suyama et al., 2006) in conjunction with their nucleotide sequences to obtain their codon alignments. The ratio of non-synonymous (Ka) to synonymous (Ks) nucleotide substitution rates (Ka/Ks ratios) were calculated using SeqinR package of R project (Charif and Lobry, 2007). Mean Ka/Ks ratios were assigned for halotolerant resistance families, with ratios of > 1 indicating beneficial mutations and ratios of < 1 indicating purifying selection (Li et al., 1985).
Results and Discussion
In general, acidophiles maintain a near neutral intracellular pH despite a proton gradient of up to 10,000 fold across the cytoplasmic membrane (Baker-Austin and Dopson, 2007). This is achieved through a variety of mechanisms including: the maintenance of a positive membrane potential to reduce proton influx by electrostatic repulsion (through the accumulation of K+); using active proton pumps to export protons; altering their cytoplasmic membrane structures; the use of enzymes such as carboxylases to consume protons; and through various cytoplasmic buffering systems (Baker-Austin and Dopson, 2007). Furthermore, the Omp40 protein in Acidithiobacillus ferrooxidans has also previously been shown to be a small, slightly anionic pore hypothesized to prevent the movement of protons across its outer membrane (Guiliani and Jerez, 2000). Sodium chloride has a deleterious effect on acidophiles due to its ability to cause osmotic stress. Chloride, however, is far more harmful to the cells due to the ability of chloride ions to permeate the cell membrane leading protons to be electrostatically attracted to the increasing negative charge in the cell (Alexander et al., 1987; Grogan, 1989; Suzuki et al., 1999; Zammit et al., 2009, 2012). This results in acidification of the cytoplasm and a collapse of the reverse transmembrane potential, which ultimately results in cell death. While the effect of both osmotic stress and chloride on acidophilic microorganisms is well understood, mechanisms of halotolerance in acidophiles that can withstand high levels of salt, such as the members of the Acidihalobacter genus, are not well studied as only a few halotolerant, acidophilic iron and sulfur oxidizing microorganisms have been discovered. Therefore, analysis of the genomes of the members of this genus was undertaken to identify potential mechanisms of osmotic stress and chloride stress tolerance at low pH.
The genus Acidihalobacter is represented by four acidophilic, iron and sulfur oxidizing mesophiles that demonstrate a chemolithoautotrophic lifestyle and are able to bioleach metals from insoluble ores in the presence of higher salt stress than have previously been reported for other acidophilic biomining microorganisms (Huber and Stetter, 1989; Simmons and Norris, 2002; Norris and Simmons, 2004; Davis-Belmar et al., 2008; Nicolle et al., 2009; Ossandon et al., 2014; Cárdenas et al., 2015; Khaleque et al., 2017a,b,c, 2018a). The genomes of the isolates of the Acidihalobacter genus have all recently been sequenced (Ossandon et al., 2014; Khaleque et al., 2017a,b,c). The genomic features of the members of the Acidihalobacter genus are compared in Table 1. All genomes ranged in size between 3.36 and 3.57 Mbp and a GC content of 59.9–64.5%. Based on 99% sequence identity of the 16s rRNA sequence of A. prosperus DSM 14174 and A. prosperus F5 to the type strain, A. prosperus DSM 5130 (as given by BLAST), the former two strains are classified as the A. prosperus species. However, “A. ferrooxidans” has only 97% 16s rRNA sequence identity to A. prosperus DSM 5130 and A. prosperus DSM 14174 and 96% identity to A. prosperus F5, and is therefore believed to be a new species of the Acidihalobacter genus (unpublished data). A. prosperus DSM 14174 was the only isolate found to contain a plasmid. A. prosperus F5 was the only genome for which a complete sequence, with no gaps, was obtained and was, therefore, used as the reference sequence for comparison and visualization of circular genomic maps using BRIG (Alikhan et al., 2011). The resulting genomic maps (Figure 1) highlighted the similarity in the genomes of the members of the Acidihalobacter genus, suggesting conservation of genes amongst the species.
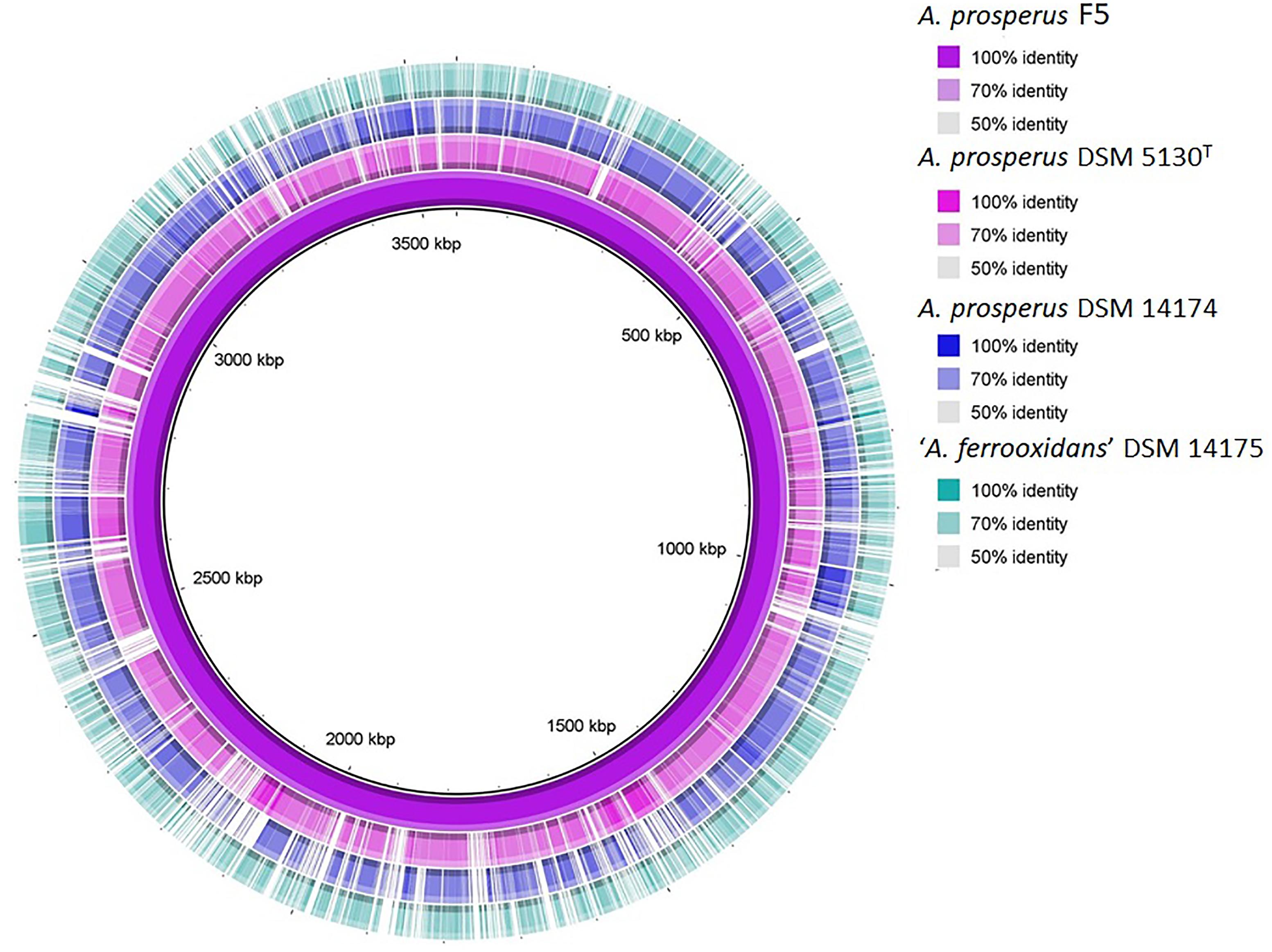
Figure 1. Whole genome comparisons of the members of the Acidihalobacter genus using the complete genome of A. prosperus F5 as the reference sequence. The BLAST match identity is represented by the color intensity in each ring.
Pangenome models are extremely useful in the characterization of the gene repertoire of microbes and as a tool to investigate the biology and lifestyle of individual microorganisms as well as the definition of species (Tettelin et al., 2008). Therefore, to investigate the differences between the genomes and to identify protein families that may play a role in the halotolerance of the members of the Acidihalobacter genus, pangenome analysis was performed. The complete gene pool of all Acidihalobacter species were found to consist a total of 6,243 protein families forming the pangenome. The pangenome was subsequently classified into the core-genome and the accessory genome (dispensable and unique genome). The core genome consists of 1422 protein families shared by all strains whereas the dispensable genome consists of 1496 protein families assigned to less than four but more than one strain. The unique genome consists of 3325 protein families assigned to only one strain. A total of 41 genes with roles in halotolerance were selected from the pangenome for further analysis. Of these, 29 were found in the core genome, 3 in the dispensable genome and 9 in the unique genome. Selected genes with potential roles in halotolerance in the members of the Acidihalobacter genus are shown in Table 2. Differences in the presence of genes for halotolerance between the species/strains are shown in Figure 2. Halotolerance mechanisms shared by the members of the Acidihalobacter genus are shown in Figure 3.
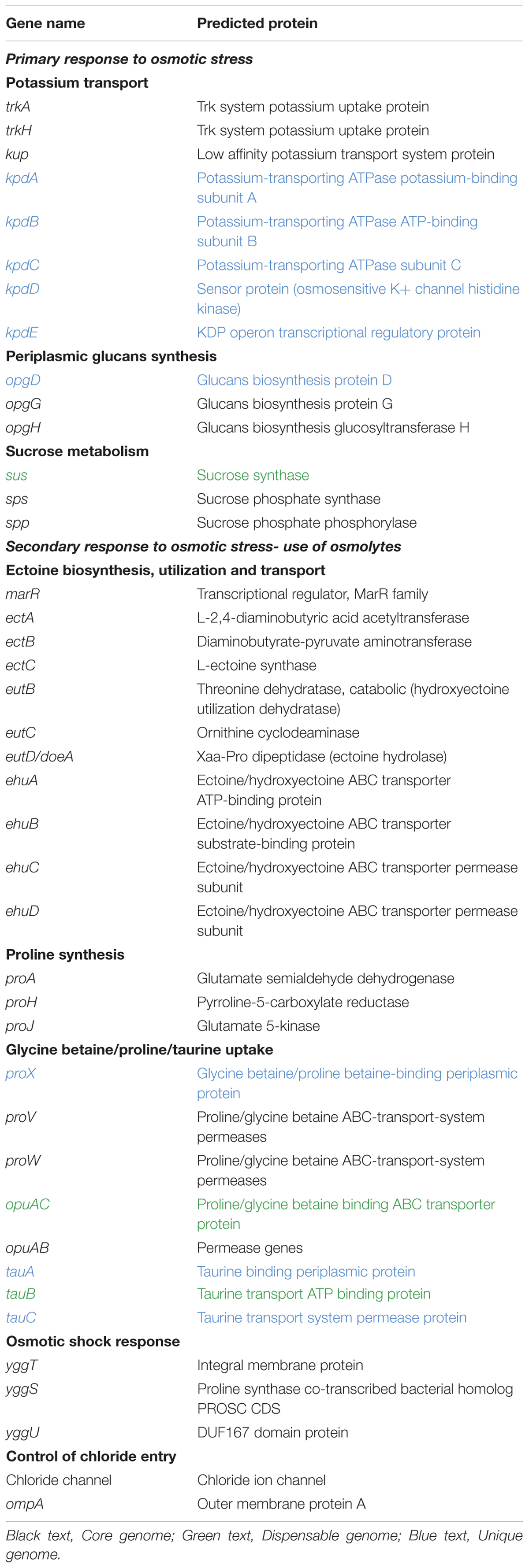
Table 2. Genes and their encoded proteins with potential roles in halotolerance in members of the Acidihalobacter genus.
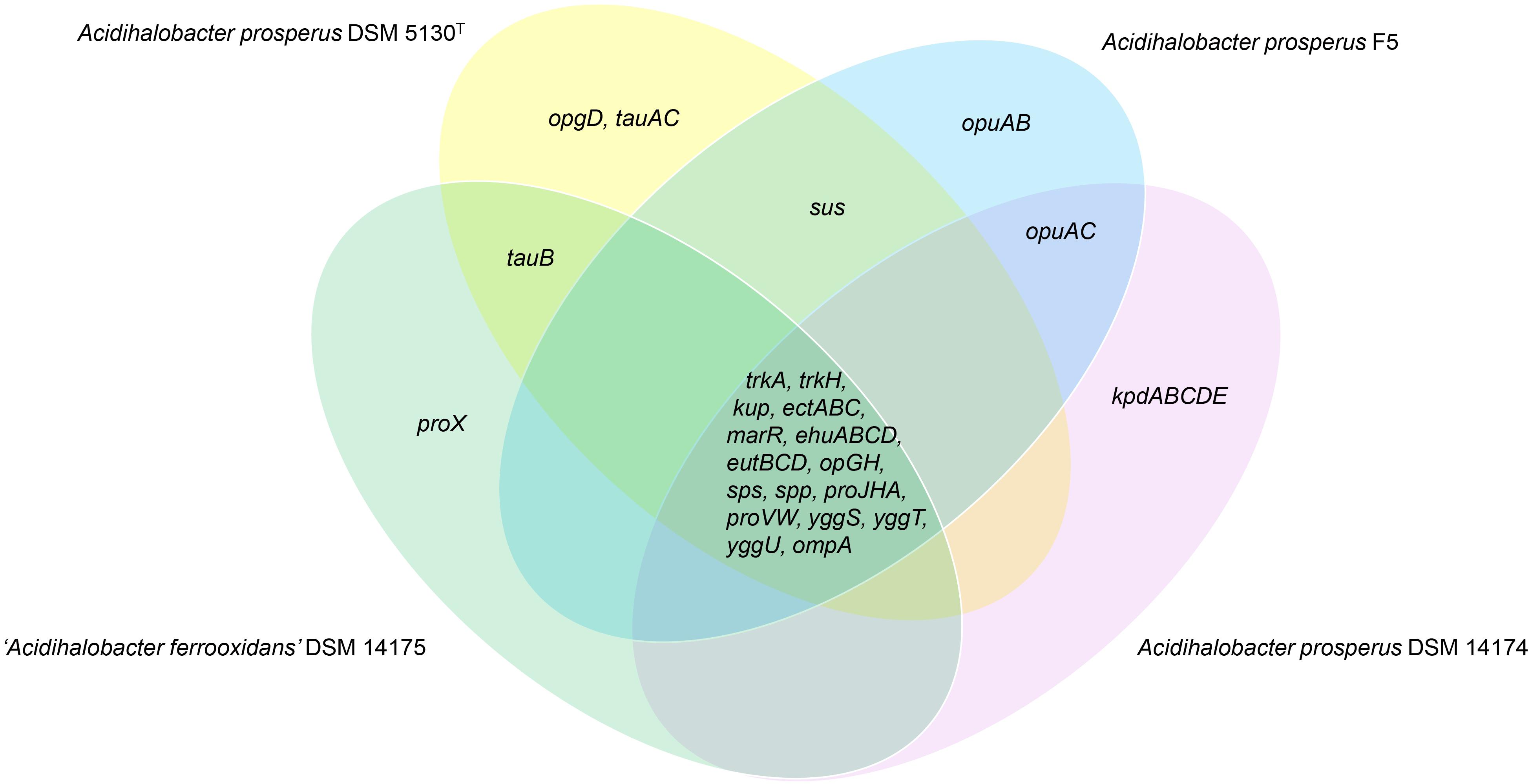
Figure 2. Venn diagram showing the shared and unique genes related to halotolerance in members of the Acidihalobacter genus.
It has previously been suggested that the core genome consists of protein families shared by all strains that encode functions related to the basic biology and phenotypes of a species (Tettelin et al., 2008). The protein families in the core genome of the members of the Acidihalobacter genus have important roles for pathways related to energy acquisition, carbohydrate metabolism and adaptation to extreme environments. Likewise, the majority of genes related to halotolerance in Acidihalobacter species were found in the core-genomes. This is not surprising, as it is expected that Acidihalobacter species are able to survive their extreme environments through well-conserved mechanisms. In order to confirm this, calculation of Ka/Ks ratios was performed in order to determine the magnitude and direction of natural selection on protein coding genes. For a given period, Ka/Ks ratios calculate the number of non-synonymous substitutions per non-synonymous sites (Ka) to the number of synonymous substitutions per synonymous site (Ks) in order to determine the net balance between beneficial and non-beneficial mutations. Ratios of significantly more than 1 indicate that mutations that have occurred are beneficial whereas values of less than 1 indicate purifying selection (tendency to be stabilized and against change) (Li et al., 1985). For the core genome of the members of the Acidihalobacter genus, Ka/Ks ratios were found to be between 0 and 0.2, suggesting that the protein families forming the core genome are well conserved. Similarly, the genes involved in halotolerance also showed ratios between 0 and 0.2 (Figure 4). This confirmed that there is pressure to conserve the sequences of housekeeping genes in order to maintain stable sequences that allow Acidihalobacter species to survive salt stress conditions.
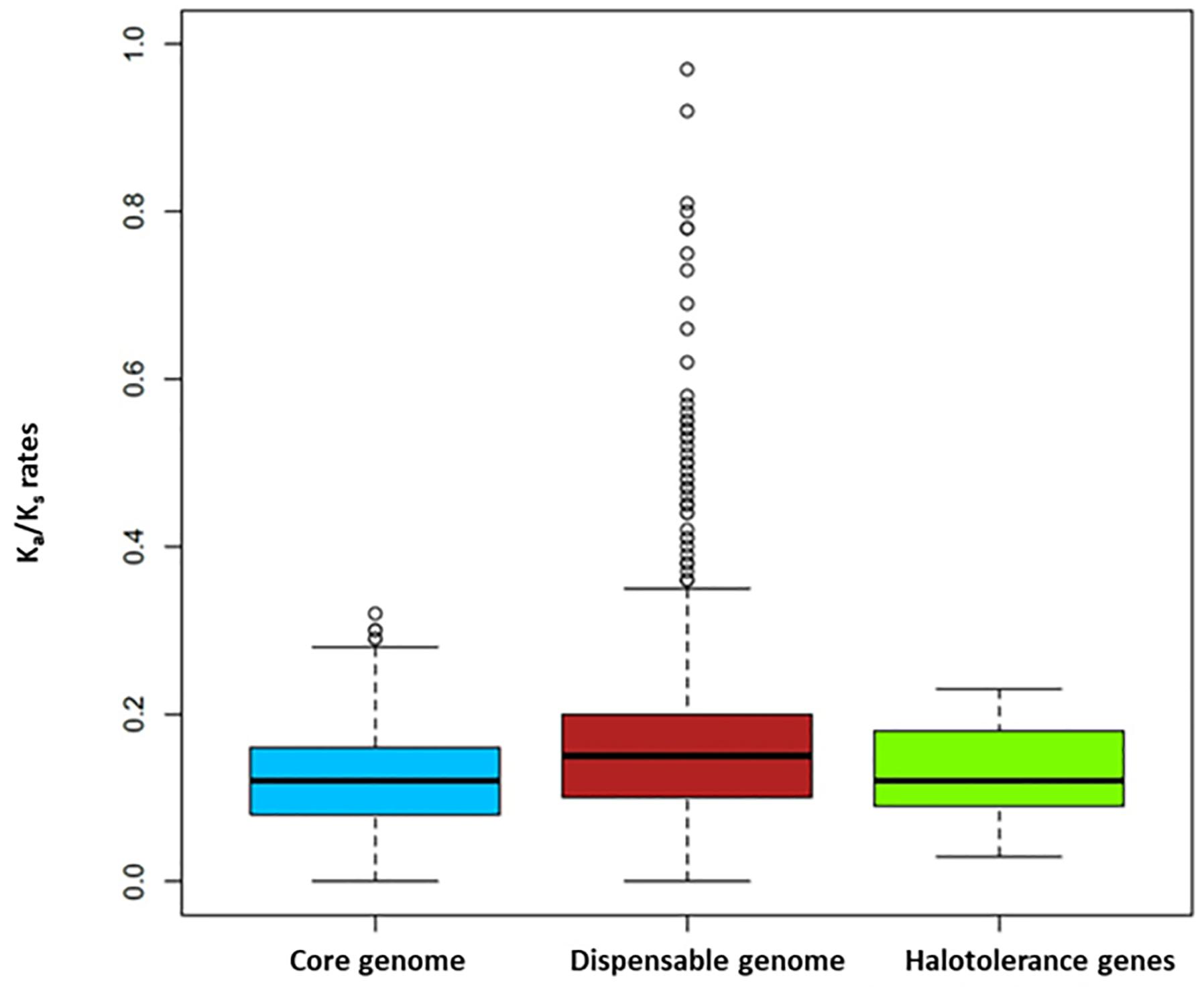
Figure 4. Boxplot of Ka/Ks ratios of core-genome (blue), dispensable-genome (red) and halotolerance genes (green).
Closer inspection of the mechanisms of halotolerance used by the members of the Acidihalobacter genus was undertaken by investigation of the possible roles of the genes involved in the primary and secondary responses to osmotic stress as well as those with roles in chloride tolerance, as described below.
Primary Responses of Acidihalobacter spp. to Osmotic Stress
The genomes of all described species of the Acidihalobacter genus harbor 2–3 copies of genes encoding energy dependent K+-transporters (trkH, trkA, kup) that may control the flux of potassium ions into the cells. As mentioned previously, potassium is important in pH homeostasis of acidophiles due to its role in generating a positive membrane potential and reducing the influx of protons when they are under pH stress. The accumulation of potassium ions along with its counter ion, glutamate, have also previously been described to form part of the primary response to osmotic stress in microorganisms (Sleator and Hill, 2002; Epstein, 2003). In extremely halotolerant bacteria, such as Halorubum saccharovorum and H. trapanicum, potassium has been shown to almost exclusively contribute to osmotic adjustment while in moderately tolerant species such as H. mediterranei and H. gibbonsii, it contributes to over 70% of osmotic adjustment (Shabala and Shabala, 2011). Therefore, it is hypothesized that these low affinity K+ uptake systems have an important role in salt stress tolerance through osmotic adjustment by members of the Acidihalobacter genus.
The genome of A. prosperus DSM 14174 was the only species in the genus to contain an operon consisting of genes for high affinity potassium transporting ATPase chains A, B and C (kdpABC), an osmosensitive K+ channel histidine kinase (kdpD) and the two- component system response regulator (kdpE). In addition to the low affinity K+ transport systems, it has previously been shown that osmotic stress and K+ limitation are responsible for strongly inducing the transcription of a kdp operon. The Kdp system was shown to play a role in increasing the osmotolerance of Escherichia coli, Staphylococcus aureus and Sinorhizobium meliloti by allowing scavenging of K+ when concentrations are low (Laimins et al., 1981; Gowrishankar, 1985; Domínguez-Ferreras et al., 2009; Price-Whelan et al., 2013). The genes kdpA, kdpB, and kdpC were found to be upregulated by 27-, 15-, and 8-fold, respectively, in Cronobacter sakazakii under osmotic stress (Lang et al., 2017). It is possible that the presence of this system in A. prosperus DSM 14174 renders this strain more resilient to salt stress than the other members of the genus when inhabiting K+ limited environments.
The synthesis of osmoregulated periplasmic glucans, which have been reviewed for Proteobacteria by Bohin (2000) may provide another well-known mechanism of osmotic adaptation in Acidihalobacter members (Kindzierski et al., 2017). In E. coli, Halomonas elongata and other Gram-negative bacteria, osmoregulated periplasmic glucans are synthesized at low osmolarity, suggesting that they play a role in the initial adaptation against osmotic stress (Kennedy, 1982; Miller et al., 1986; Kindzierski et al., 2017). We suggest a similar role for osmoregulated periplasmic glucans in Acidihalobacter members. Genes opgH and opgG coding for glucosyltransferase and glucan biosynthesis precursors, respectively, that are involved in the synthesis of these glucans were found on all Acidihalobacter genomes. A. prosperus DSM 5130T also had genes for opgD which is another glucan biosynthesis precursor. It has previously been seen that the opgD and opgG genes can be either present or absent on certain genomes and that the interactions between opgH and opgG/opgD have evolved differently in different species (Kindzierski et al., 2017).
Sucrose has previously been identified as a non-accumulated disaccharide that provides osmoprotection in S. meliloti (Gouffi et al., 1999). In this species, sucrose does not accumulate as an osmolyte or a precursor of osmolytes but, rather, indirectly enhances the levels of glutamate and N-acetylglutaminylglutamine in order to help increase growth at high salt (Sleator and Hill, 2002). The gene for sucrose synthase (sus) was found in all members of the A. prosperus species. However, genes for sucrose phosphate synthase (sps) and sucrose phosphorylase (spp) were found on all the genomes, suggesting that both sucrose synthesis and metabolism occurs in all the members of the Acidihalobacter genus and that this may play a role in the initial response to osmotic stress, as has previously been described for S. meliloti (Gouffi et al., 1999).
Osmoprotectants in Acidihalobacter spp.
The primary response to osmotic stress mentioned above is a temporary response, as potassium glutamate increases intracellular osmolarity, inducing a negative effect on cellular metabolism when salt stress is prolonged. Therefore, the effect of potassium glutamate is to act as a signal of osmotic stress and to stimulate the secondary response, i.e., the removal of potassium glutamate and the synthesis or uptake of osmoprotective compounds, known as osmoprotectants, osmolytes or compatible solutes (Sleator and Hill, 2002). Osmoprotectants are low molecular weight organic molecules that can accumulate to high concentrations in cells without affecting cellular processes due to their high solubility and their ability to not interact with proteins as a result of being uncharged at physiological pH. When cells are under stress, osmoprotectants help by stabilizing proteins and by balancing internal solute levels to match the immediate environment (Roeßler and Müller, 2001).
In a recent study, trehalose was identified as one of, or the sole, osmoprotectant/compatible solute in members of the salt sensitive, acidophilic, iron/sulfur oxidizing Leptospirillum and Acidithiobacillus spp., with the exception of A. thiooxidans (Galleguillos et al., 2018). Members of the Acidihalobacter spp. appear to be unique among the iron and sulfur oxidizing acidophiles in their absence of genes for the synthesis of trehalose. Potential osmoprotectants that can be used instead by the members of the Acidihalobacter genus are discussed below.
Ectoine is one of the most abundant osmolytes in nature and was first described in the extremely halophilic sulfobacterium Ectothiorhodospira halochloris (Galinski et al., 1985). It is a zwitterionic molecule that has been found to improve protein folding. It also has been found to protect biomolecules from extremes environments, including solute, temperature and chemical stresses (Barth et al., 2000). Genes coding for the enzymes required for ectoine biosynthesis were found in each of the Acidihalobacter spp. genomes. The detection of these genes in the Acidihalobacter genomes suggested that ectoine contributed to the osmotolerance of the species in this genus (Ossandon et al., 2014; Khaleque et al., 2017b,c). This was confirmed in recent proteomic studies that showed increased abundance of ectoine transporters (50 fold increase) and ectoine synthase (422 fold increase) in DSM 5130T and A. prosperus DSM 14174, respectively, when they were grown at high salt stress (Dopson et al., 2017; Khaleque et al., 2018b), thereby confirming that ectoine is an important osmoprotectant in these two strains. Ectoine biosynthesis in Acidihalobacter can proceed through a pathway involving the products of genes for diaminobutyrate-pyruvate aminotransferase (ectA), L-2,4-diaminobutyric acid acetyltransferase (ectB) and L-ectoine synthase (ectC) (Louis and Galinski, 1997; Figure 5). Genomes of members of the Acidihalobacter genus were found to contain genes for a marR-like regulatory protein associated with the ectoine operon that has previously been implicated in the transcriptional regulation of the ectoine operon in the halotolerant obligate methanotroph Methylomicrobium alcaliphilum strain 20Z in response to an increased salinity (Mustakhimov et al., 2010). Furthermore, transporters for ectoine encoded by genes ehuABCD were also identified on all of the genomes as were the genes eutBCD, which have previously been indicated to have roles in ectoine catabolism as part of the eutABCD operon (Jebbar et al., 2005). Leptospirillum spp. are the only other acidophilic species whose genomes contain genes for the biosynthesis and transport of this osmoprotectant (Mosier et al., 2013). Leptospirillum group II bacteria also contain the ectoine dioxygenase/hydroxylase (ectD) gene for the conversion of ectoine to hydroxyectoine, which has also been implicated as an osmoprotectant in this group of bacteria (Mosier et al., 2013; Figure 5). However, as the Acidihalobacter genomes do not contain the ectD gene it is not known if hydroxyectoine is synthesized by members of this genus as an osmoprotectant.
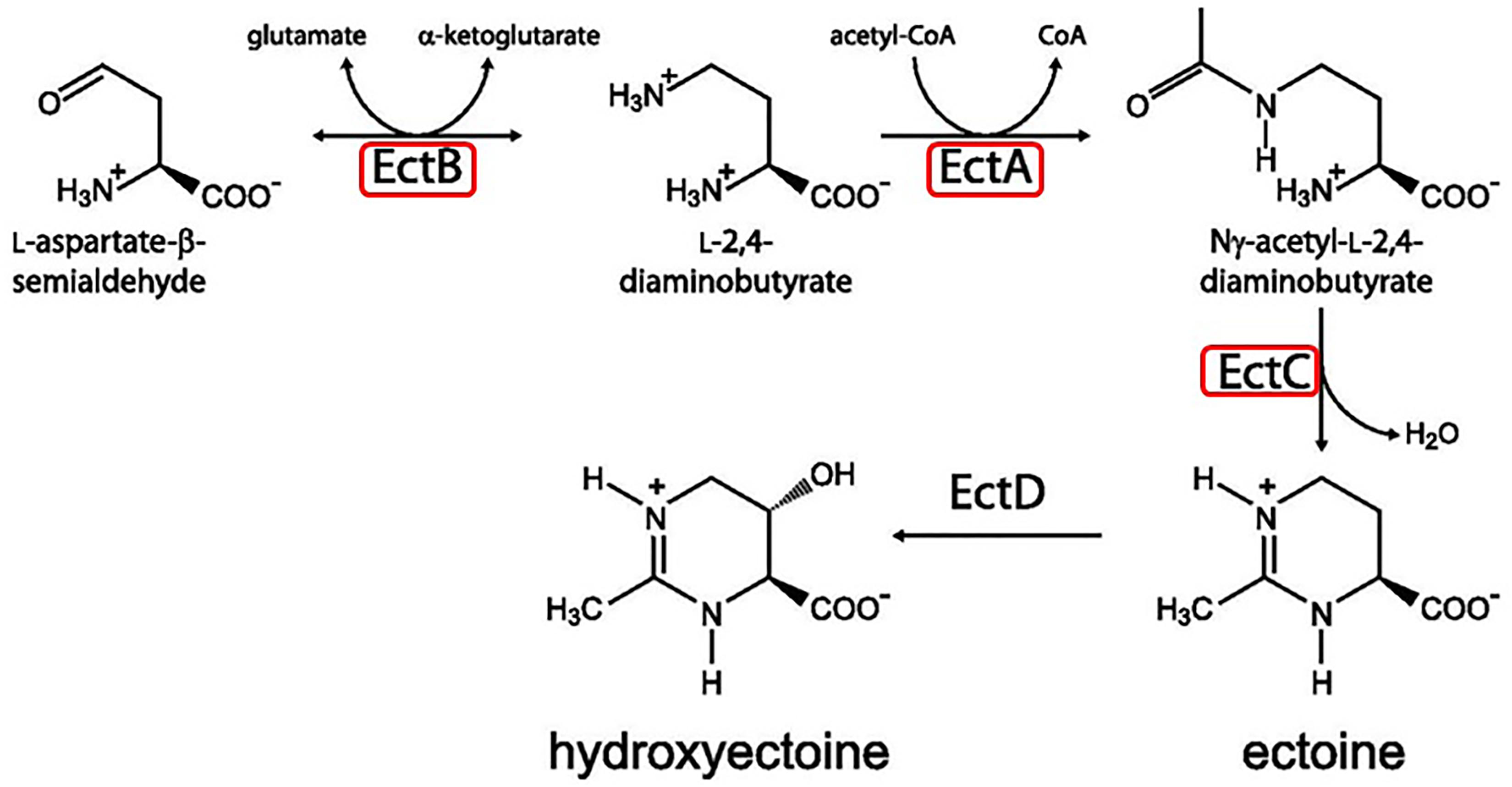
Figure 5. The ectoine biosynthesis pathway. Acidihalobacter genomes contain the genes ectABC (highlighted in red boxes) but not ectD and therefore are likely to synthesize ectoine but not hydroxyectoine through the pathway shown. Reproduced from Ofer et al. (2012) with permission from American Society for Microbiology.
Previously proline was found to be the predominant compatible solute used by the moderate halophile Halobacillus halophilus, when faced with increasing osmotic stress (Saum and Müller, 2007). In the acidophilic sulfur oxidizing A. caldus SM-1, enzymes involved in the proline synthesis were only observed in cells grown at 0.5 M sodium chloride (Guo et al., 2014). Proline biosynthesis from glutamate proceeds through three enzymes in this microorganism: pyrroline-5-carboxylate reductase (proH), glutamate 5-kinase (proJ) and a glutamate semialdehyde dehydrogenase (proA) (Saum and Müller, 2008). The inspection of the genomes in this study revealed the presence of these three genes in all Acidihalobacter genomes. Furthermore, in A. prosperus DSM 5130T, A. prosperus DSM 14174 and “A. ferrooxidans” DSM 14175, the proH gene was part of an operon consisting of a proline synthase homolog (yggS), proH, an integral membrane protein involved in osmotic shock response (yggT) and a DUF167 domain protein (yggU). In “A. ferrooxidans” DSM 14175, the yggS gene was not found as part of the same operon but was present elsewhere on the genome.
While the synthesis of compatible solutes is of importance in microorganisms faced with salt stress, the transport of these compounds can also provide osmoprotection. For example, although the Acidihalobacter genomes did not contain genes for the synthesis of glycine betaine, it is still possible that they can use it as an osmoprotectant because the transport and uptake of proline and glycine betaine can occur through the same transporters (Waditee et al., 2002). All four Acidihalobacter genomes showed the presence of genes for the proline/glycine betaine ABC-transport-system permeases, proV and proW. However, only A. prosperus species carried glycine betaine binding protein genes opuAC. The opuC protein has previously also been implicated in the transport and accumulation of ectoine in Bacillus subtilis under osmotic stress (Jebbar et al., 1997). A. prosperus F5 additionally carried the glycine betaine ABC transport permease genes, opuAB. “A. ferrooxidans” DSM 14175 lacked both opuAC and opuAB but carried a gene for an alternative proline/glycine betaine binding ABC transporter protein proX.
Taurine appears to be another osmoprotectant that can be accumulated in certain members of the Acidihalobacter genus. Taurine has previously been found to be used as an osmoprotectant in microbial communities from biofilms at the Richmond mine, Iron Mountain, though none of the bacteria or archaea in the study were able to synthesize it (Mosier et al., 2013). The study found that taurine uptake proteins were produced by Sulfobacillus spp. and these were predicted to provide osmoprotection by providing a means of uptake and accumulation of this amino acid (Mosier et al., 2013). Similarly, the genes tauABC encoding proteins involved in taurine uptake have also been found on the genome of A. prosperus DSM 5130T, whereas “A. ferrooxidans” DSM 14175 only contained tauB and A. prosperus DSM 14174 and A. prosperus F5 genomes did not contain any tau genes. The genomes of both DSM 5130T and “A. ferrooxidans” DSM 14175 lacked genes for the utilization of taurine (tauD), suggesting that in these species, taurine may accumulate as an osmoprotectant rather than for use as a metabolite.
Mechanisms of Dealing With Chloride Ion Stress
As mentioned previously, chloride ion stress is a limiting factor for growth of acidophilic bioleaching microorganisms. Therefore, the genomes of the Acidihalobacter spp. were searched for genes that may have a role in chloride ion tolerance. Multiple genes encoding chloride ion channel proteins were present on all the genomes of the Acidihalobacter species. Of special interest were the chloride ion channels found directly downstream of the previously mentioned yggS-proH-yggT-yggU operon in A. prosperus DSM 5130T, A. prosperus DSM 14174 and A. prosperus F5. The “A. ferrooxidans” DSM 14175 genome was missing the yggS gene in the operon but contained two separate genes encoding chloride ion channels. The genomes of the salt sensitive A. ferrooxidans ATCC 23270 and L. ferriphilum DSM 14167 were searched to identify whether this operon was present on their genomes. While the genome of A. ferrooxidans ATCC 23270 was found to contain an yggS-proH-yggT-yggU operon, chloride channels did not form part of this operon. Similarly, in L. ferriphilum DSM 14167, only yggS-proH-yggT were present as part of the operon but the other genes were absent. It is hypothesized that the proteins of the yggS-proH-yggT-yggU operon in Acidihalobacter spp. may be co-expressed as a result of chloride ion entry to the cells and therefore may provide a mechanism of dealing with chloride stress in Acidihalobacter species. A comparison of the operons in the different microorganisms is shown in Figure 6.
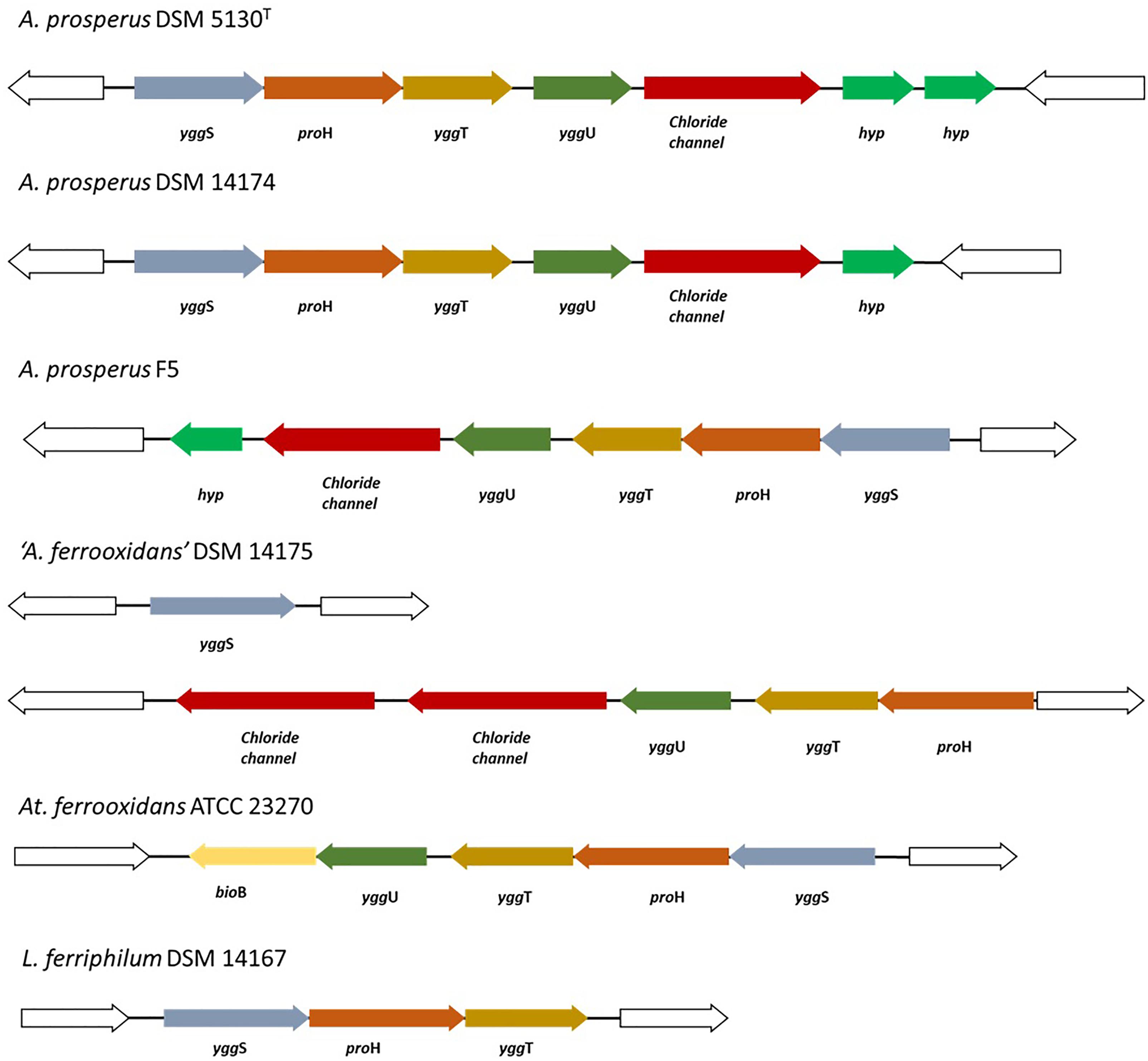
Figure 6. Comparison of the yggS-proH-yggT-yggU operon in members of the Acidihalobacter genus against that in salt sensitive, acidophilic A. ferrooxidans ATCC 23270 and the moderately salt tolerant L. ferriphilum DSM 14167. The members of the Acidihalobacter genus all encoded chloride ion channel proteins in the operon.
Another protein that has recently been found to decrease in abundance by 2 fold in A. prosperus DSM 5130T and 2.6 fold in A. prosperus V6 at high salt is the outer membrane protein A, encoded by ompA (Dopson et al., 2017; Khaleque et al., 2018b). Previous studies have suggested that a decrease in these proteins results in a reduction of pores in the outer membrane that may inhibit chloride from entering the cells (Csonka and Hanson, 1991; Dopson et al., 2017; Khaleque et al., 2018b). Genes encoding ompA precursors were found on the genomes of all Acidihalobacter species, and we hypothesize they have similar roles in A. prosperus F5 and “A. ferrooxidans” DSM 14175. Furthermore, outer membrane protein A has been shown to have a role in maintaining the stability and integrity of the bacterial membrane and previous studies have suggested that the periplasmic domain of this protein has a role in both acid and osmotic stress tolerance in E. coli (Koebnik, 1995; Wang et al., 2016). This suggests that ompA may play an important role in the tolerance of the Acidihalobacter spp. to chloride, osmotic stress and acid stress.
Astrobiological Significance
Extreme halophiles and the terrestrial environments they inhabit are being used as analogs for providing useful insights into the potential for life elsewhere in the universe (DasSarma, 2006; Cabrol, 2018). For example, it is widely accepted that Mars once had a liquid ocean that could potentially have supported life but it subsequently dried out leaving the arid surface of Mars today (Citron et al., 2018). However, as Mars dried out acidic, saline liquid waters were intermittently available and salts were precipitated from Martian brines (Tosca et al., 2008; Tosca et al., 2011). Analog hypersaline environments on Earth such as the Salares of Northern Chile, the Dead Sea, the Basque Lakes of British Colombia and many others are being exploited to determine what organisms are present in such environments and how they tolerate high concentrations of salts (Bodaker et al., 2010; Pontefract et al., 2017; Fox-Powell and Cockell, 2018). Within the next few years, missions will set out to search for biosignatures on Mars (Vago et al., 2017) and it is imperative to develop ideas and models of the sort of life, or evidence of past life, we should be looking for and in which Martian environments.
Conclusion
Comparative genomics is the first step in identifying how microorganisms differ on a molecular level and helps to identify genes conserved among species as well as genes that may give an organism its unique characteristics. The genomic comparison of the members of the Acidihalobacter genus has helped to extend the knowledge of the differences in their mechanisms of tolerance to salt stress thereby determining their usefulness for saline water bioleaching processes. The basic features for osmotolerance for the members of the Acidihalobacter genus appear to be the ability to accumulate potassium and synthesize osmoregulated periplasmic glucans and sucrose as the primary response to salt stress and then to replace these with osmoprotectants as salt stress increases. Differences could be seen in the genes coding for proteins involved in halotolerance suggesting that the different members of the Acidihalobacter genus may use a different mechanisms for surviving high salt stress. Further proteomic work is required to confirm the preferential mechanisms of halotolerance used by the extremely acidophilic members of this genus.
Supporting Information
The full list of gene annotations showing encoded proteins can be found at the following link: https://drive.google.com/open?id=0BwTiq6bJgkC_T1NHMGRuLUFjTEE.
Author Contributions
HK, EW, CG, and DH conceived and designed the experiments. HK, CG, and RS performed the experiments and analyzed the data. EW and AK contributed to the materials and analysis tools. HK and EW wrote the manuscript. All authors read and approved the final manuscript.
Funding
This work was supported in part by the Programa de Apoyo a Centros con Financiamiento Basal AFB 17004 to Fundación Ciencia & Vida and by Fondecyt 1181717. HK was the recipient of a Curtin Strategic International Research Scholarship and CSIRO Postgraduate Scholarship.
Conflict of Interest Statement
RS was employed by company Sodexo Australia.
The remaining authors declare that the research was conducted in the absence of any commercial or financial relationships that could be construed as a potential conflict of interest.
Footnotes
References
Alexander, B., Leach, S., and Ingledew, W. J. (1987). The relationship between chemiosmotic parameters and sensitivity to anions and organic acids in the acidophile Thiobacillus ferrooxidans. Microbiology 133, 1171–1179. doi: 10.1099/00221287-133-5-1171
Alikhan, N.-F., Petty, N. K., Zakour, N. L. B., and Beatson, S. A. (2011). BLAST Ring Image Generator (BRIG): simple prokaryote genome comparisons. BMC Genomics 12:1. doi: 10.1186/1471-2164-12-402
Altschul, S. F., Madden, T. L., Schäffer, A. A., Zhang, J., Zhang, Z., Miller, W., et al. (1997). Gapped BLAST and PSI-BLAST a new generation of protein database search programs. Nucleic Acids Res. 25, 3389–3402.
Aziz, R. K., Bartels, D., Best, A. A., DeJongh, M., Disz, T., Edwards, R. A., et al. (2008). The RAST Server: rapid annotations using subsystems technology. BMC Genomics 9:75. doi: 10.1186/1471-2164-9-75
Baker-Austin, C., and Dopson, M. (2007). Life in acid: pH homeostasis in acidophiles. Trends Microbiol. 15, 165–171. doi: 10.1016/j.tim.2007.02.005
Barth, S., Huhn, M., Matthey, B., Klimka, A., Galinski, E., and Engert, A. (2000). Compatible-solute-supported periplasmic expression of functional recombinant proteins under stress conditions. Appl. Environ. Microbiol. 66, 1572–1579. doi: 10.1128/AEM.66.4.1572-1579.2000
Blight, K. R., and Ralph, D. E. (2004). Effect of ionic strength on iron oxidation with batch cultures of chemolithotrophic bacteria. Hydrometallurgy 73, 325–334. doi: 10.1016/j.hydromet.2003.12.006
Bodaker, I., Sharon, I., Suzuki, M. T., Feingersch, R., Shmoish, M., Andreishcheva, E., et al. (2010). Comparative community genomics in the Dead Sea: an increasingly extreme environment. ISME J. 4:399. doi: 10.1038/ismej.2009.141
Bohin, J.-P. (2000). Osmoregulated periplasmic glucans in Proteobacteria. FEMS Microbiol. Lett. 186, 11–19. doi: 10.1111/j.1574-6968.2000.tb09075.x
Boxall, N., Rea, S., Li, J., Morris, C., and Kaksonen, A. (2016). Effect of high sulfate concentrations on chalcopyrite bioleaching and molecular characterisation of the bioleaching microbial community. Hydrometallurgy 168, 32–39. doi: 10.1016/j.hydromet.2016.07.006
Cabrol, N. A. (2018). The Coevolution of Life and Environment on Mars: An Ecosystem Perspective on the Robotic Exploration of Biosignatures. New Rochelle, NY: Mary Ann Liebert, Inc.
Cárdenas, J. P., Moya, F., Covarrubias, P., Shmaryahu, A., Levicán, G., Holmes, D. S., et al. (2012). Comparative genomics of the oxidative stress response in bioleaching microorganisms. Hydrometallurgy 127, 162–167. doi: 10.1016/j.hydromet.2012.07.014
Cárdenas, J. P., Ortiz, R., Norris, P. R., Watkin, E., and Holmes, D. S. (2015). Reclassification of ‘Thiobacillus prosperus’ Huber and Stetter, 1989 as Acidihalobacter prosperus gen. nov., sp. nov., a member of the family Ectothiorhodospiraceae. Int. J. Syst. Evol. Microbiol. 65, 3641–3644. doi: 10.1099/ijsem.0.000468
Cárdenas, J. P., Valdés, J., Quatrini, R., Duarte, F., and Holmes, D. S. (2010). Lessons from the genomes of extremely acidophilic bacteria and archaea with special emphasis on bioleaching microorganisms. Appl. Microbiol. Biotechnol. 88, 605–620. doi: 10.1007/s00253-010-2795-9
Charif, D., and Lobry, J. R. (2007). “SeqinR 1.0-2: a contributed package to the R project for statistical computing devoted to biological sequences retrieval and analysis,” in Structural Approaches to Sequence Evolution, eds U. Bastolla, M. Porto, E. Roman, and M. Vendruscolo (Berlin: Springer), 207–232. doi: 10.1007/978-3-540-35306-5_10
Citron, R. I., Manga, M., and Hemingway, D. J. (2018). Timing of oceans on Mars from shoreline deformation. Nature 555:643. doi: 10.1038/nature26144
Csonka, L. N., and Hanson, A. D. (1991). Prokaryotic osmoregulation: genetics and physiology. Ann. Rev. Microbiol. 45, 569–606. doi: 10.1146/annurev.mi.45.100191.003033
Darling, A. E., Mau, B., and Perna, N. T. (2010). progressiveMauve: multiple genome alignment with gene gain, loss and rearrangement. PLoS One 5:e11147. doi: 10.1371/journal.pone.0011147
DasSarma, S. (2006). Extreme halophiles are models for astrobiology. Microbe Am. Soc. Microbiol. 1:120. doi: 10.1128/microbe.1.120.1
Davis-Belmar, C. S., Nicolle, J. L. C., and Norris, P. R. (2008). Ferrous iron oxidation and leaching of copper ore with halotolerant bacteria in ore columns. Hydrometallurgy 94, 144–147. doi: 10.1016/j.hydromet.2008.05.030
Domínguez-Ferreras, A., Munoz, S., Olivares, J., Soto, M. J., and Sanjuán, J. (2009). Role of potassium uptake systems in Sinorhizobium meliloti osmoadaptation and symbiotic performance. J. Bacteriol. 191, 2133–2143. doi: 10.1128/JB.01567-08
Dopson, M., Holmes, D. S., Lazcano, M., McCredden, T. J., Bryan, C. G., Mulroney, K. T., et al. (2017). Multiple osmotic stress responses in Acidihalobacter prosperus result in tolerance to chloride ions. Front. Microbiol. 7:2132. doi: 10.3389/fmicb.2016.02132
Edgar, R. C. (2004). MUSCLE: multiple sequence alignment with high accuracy and high throughput. Nucleic Acids Res. 32, 1792–1797. doi: 10.1093/nar/gkh340
Empadinhas, N., and da Costa, M. (2008). Osmoadaptation mechanisms in prokaryotes: distribution of compatible solutes. Int. Microbiol. 11, 151–161.
Epstein, W. (2003). The roles and regulation of potassium in bacteria. Prog. Nucleic Acid Res. Mol. Biol. 75, 293–320. doi: 10.1016/s0079-6603(03)75008-75009
Fox-Powell, M. G., and Cockell, C. S. (2018). Building a geochemical view of microbial salt tolerance: halophilic adaptation of Marinococcus in a natural magnesium sulfate brine. Front. Microbiol. 9:739. doi: 10.3389/fmicb.2018.00739
Galinski, E. A., Pfeiffer, H. P., and Trueper, H. G. (1985). 1, 4, 5, 6-Tetrahydro-2-methyl-4-pyrimidinecarboxylic acid. FEBS J. 149, 135–139.
Galleguillos, P. A., Grail, B. M., Hallberg, K. B., Demergasso, C. S., and Johnson, D. B. (2018). Identification of trehalose as a compatible solute in different species of acidophilic bacteria. J. Microbiol. 56, 727–733. doi: 10.1007/s12275-018-8176-2
Gouffi, K., Pica, N., Pichereau, V., and Blanco, C. (1999). Disaccharides as a new class of nonaccumulated osmoprotectants for Sinorhizobium meliloti. Appl. Environ. Microbiol. 65, 1491–1500.
Gowrishankar, J. (1985). Identification of osmoresponsive genes in Escherichia coli: evidence for participation of potassium and proline transport systems in osmoregulation. J. Bacteriol. 164, 434–445.
Grogan, D. W. (1989). Phenotypic characterization of the archaebacterial genus Sulfolobus: comparison of five wild-type strains. J. Bacteriol. 171, 6710–6719. doi: 10.1128/jb.171.12.6710-6719.1989
Guiliani, N., and Jerez, C. A. (2000). Molecular cloning, sequencing, and expression of omp-40, the gene coding for the major outer membrane protein from the acidophilic bacterium Thiobacillus ferrooxidans. Appl. Environ. Microbiol. 66, 2318–2324. doi: 10.1128/AEM.66.6.2318-2324.2000
Guo, X., Jiang, C., Luo, Y., Zhang, M., Poetsch, A., and Liu, S. (2014). Proteomic and molecular investigations revealed that Acidithiobacillus caldus adopts multiple strategies for adaptation to NaCl stress. Chin. Sci. Bull. 59, 301–309. doi: 10.1007/s11434-013-0039-y
Huber, H., and Stetter, K. (1989). Thiobacillus prosperus sp. nov., represents a new group of halotolerant metal-mobilizing bacteria isolated from a marine geothermal field. Arch. Microbiol. 151, 479–485. doi: 10.1007/BF00454862
Jebbar, M., Sohn-Bösser, L., Bremer, E., Bernard, T., and Blanco, C. (2005). Ectoine-induced proteins in Sinorhizobium meliloti include an ectoine ABC-type transporter involved in osmoprotection and ectoine catabolism. J. Bacteriol. 187, 1293–1304. doi: 10.1128/JB.187.4.1293-1304.2005
Jebbar, M., von Blohn, C., and Bremer, E. (1997). Ectoine functions as an osmoprotectant in Bacillus subtilis and is accumulated via the ABC-transport system OpuC. FEMS Microbiol. Lett. 154, 325–330. doi: 10.1111/j.1574-6968.1997.tb12663.x
Johnson, D. B., and Schippers, A. (2017). Recent advances in acidophile microbiology: fundamentals and applications. Front. Microbiol. 8:428. doi: 10.3389/978-2-88945-163-0
Kearse, M., Moir, R., Wilson, A., Stones-Havas, S., Cheung, M., Sturrock, S., et al. (2012). Geneious Basic: an integrated and extendable desktop software platform for the organization and analysis of sequence data. Bioinformatics 28, 1647–1649. doi: 10.1093/bioinformatics/bts199
Kennedy, E. P. (1982). Osmotic regulation and the biosynthesis of membrane-derived oligosaccharides in Escherichia coli. Proc. Natl. Acad. Sci. 79, 1092–1095. doi: 10.1073/pnas.79.4.1092
Khaleque, H. N., Corbett, M. K., Ramsay, J. P., Kaksonen, A. H., Boxall, N. J., and Watkin, E. L. J. (2017a). Complete genome sequence of Acidihalobacter prosperus strain F5, an extremely acidophilic, iron- and sulfur-oxidizing halophile with potential industrial applicability in saline water bioleaching of chalcopyrite. J. Biotechnol. 262, 56–59. doi: 10.1016/j.jbiotec.2017.10.001
Khaleque, H. N., Ramsay, J. P., Murphy, R. J., Kaksonen, A. H., Boxall, N. J., and Watkin, E. L. (2017b). Draft genome sequence of the acidophilic, halotolerant, and iron/sulfur-oxidizing Acidihalobacter prosperus DSM 14174 (Strain V6). Genome Announc. 5:e1469-1416. doi: 10.1128/genomeA.01469-1416
Khaleque, H. N., Ramsay, J. P., Murphy, R. J. T., Kaksonen, A. H., Boxall, N. J., and Watkin, E. L. J. (2017c). Draft genome sequence of Acidihalobacter ferrooxidans DSM 14175 (Strain V8), a new iron- and sulfur-oxidizing, halotolerant, acidophilic species. Genome Announc. 5:e00413-417. doi: 10.1128/genomeA.00413-417
Khaleque, H. N., Kaksonen, A. H., Boxall, N. J., and Watkin, E. L. J. (2018a). Chloride ion tolerance and pyrite bioleaching capabilities of pure and mixed halotolerant, acidophilic iron- and sulfur-oxidizing cultures. Min. Eng. 120, 87–93. doi: 10.1016/j.mineng.2018.02.025
Khaleque, H. N., Shafique, R., Kaksonen, A. H., Boxall, N. J., and Watkin, E. L. (2018b). Quantitative proteomics using SWATH-MS identifies mechanisms of chloride tolerance in the halophilic acidophile Acidihalobacter prosperus DSM 14174. Res. Microbiol. 169, 638–648. doi: 10.1016/j.resmic.2018.07.002
Kindzierski, V., Raschke, S., Knabe, N., Siedler, F., Scheffer, B., Pflüger-Grau, K., et al. (2017). Osmoregulation in the halophilic bacterium Halomonas elongata: a case study for integrative systems biology. PLoS One 12:e0168818. doi: 10.1371/journal.pone.0168818
Koebnik, R. (1995). Proposal for a peptidoglycan-associating alpha-helical motif in the C-terminal regions of some bacterial cell-surface proteins. Mol. Microbiol. 16, 1269–1270. doi: 10.1111/j.1365-2958.1995.tb02348.x
Laimins, L., Rhoads, D., and Epstein, W. (1981). Osmotic control of kdp operon expression in Escherichia coli. Proc. Natl. Acad. Sci. 78, 464–468. doi: 10.1073/pnas.78.1.464
Lang, E., Guyot, S., Alvarez-Martin, P., Perrier-Cornet, J.-M., and Gervais, P. (2017). Caco-2 invasion by Cronobacter sakazakii and Salmonella enterica exposed to drying and heat treatments in dried state in milk powder. Front. Microbiol. 8:1893. doi: 10.3389/fmicb.2017.01893
Levicán, G., Ugalde, J. A., Ehrenfeld, N., Maass, A., and Parada, P. (2008). Comparative genomic analysis of carbon and nitrogen assimilation mechanisms in three indigenous bioleaching bacteria: predictions and validations. BMC Genomics 9:581. doi: 10.1186/1471-2164-9-581
Li, W.-H., Wu, C.-I., and Luo, C.-C. (1985). A new method for estimating synonymous and nonsynonymous rates of nucleotide substitution considering the relative likelihood of nucleotide and codon changes. Mol. Biol. Evol. 2, 150–174.
Louis, P., and Galinski, E. A. (1997). Characterization of genes for the biosynthesis of the compatible solute ectoine from Marinococcus halophilus and osmoregulated expression in Escherichia coli. Microbiology 143, 1141–1149. doi: 10.1099/00221287-143-4-1141
Miller, K. J., Kennedy, E. P., and Reinhold, V. N. (1986). Osmotic adaptation by gram-negative bacteria: possible role for periplasmic oligosaccharides. Science 231, 48–51. doi: 10.1126/science.3941890
Mosier, A. C., Justice, N. B., Bowen, B. P., Baran, R., Thomas, B. C., Northen, T. R., et al. (2013). Metabolites associated with adaptation of microorganisms to an acidophilic, metal-rich environment identified by stable-isotope-enabled metabolomics. mBio 4:e484-12. doi: 10.1128/mBio.00484-12
Mustakhimov, I. I., Reshetnikov, A. S., Glukhov, A. S., Khmelenina, V. N., Kalyuzhnaya, M. G., and Trotsenko, Y. A. (2010). Identification and characterization of EctR1, a new transcriptional regulator of the ectoine biosynthesis genes in the halotolerant methanotroph Methylomicrobium alcaliphilum 20Z. J. bacteriol. 192, 410–417. doi: 10.1128/JB.00553-09
Nicolle, J. L. C., Simmons, S., Bathe, S., and Norris, P. R. (2009). Ferrous iron oxidation and rusticyanin in halotolerant, acidophilic ‘Thiobacillus prosperus’. Microbiology 155, 1302–1309. doi: 10.1099/mic.0.023192-23190
Norris, P., and Simmons, S. (2004). Pyrite oxidation by halotolerant, acidophilic bacteria. Biohydrometallurgy: a sustainable technology in evolution, part II. Athens: National Technical University of Athens.
Ofer, N., Wishkautzan, M., Meijler, M., Wang, Y., Speer, A., Niederweis, M., et al. (2012). Ectoine biosynthesis in Mycobacterium smegmatis. Appl. Environ. Microbiol. 78, 7483–7486. doi: 10.1128/AEM.01318-12
Ossandon, F. J., Cárdenas, J. P., Corbett, M., Quatrini, R., Holmes, D. S., and Watkin, E. (2014). Draft genome sequence of the iron-oxidizing, acidophilic, and halotolerant “Thiobacillus prosperus” type strain DSM 5130. Genome Announc. 2:e1042-14. doi: 10.1128/genomeA.01042-14
Overbeek, R., Olson, R., Pusch, G. D., Olsen, G. J., Davis, J. J., Disz, T., et al. (2014). The SEED and the rapid annotation of microbial genomes using subsystems technology (RAST). Nucleic Acids Res. 42, D206–D214. doi: 10.1093/nar/gkt1226
Petry, M., Sanz, M. A., Langlais, C., Bonnelye, V., Durand, J.-P., Guevara, D., et al. (2007). The El Coloso (Chile) reverse osmosis plant. Desalination 203, 141–152. doi: 10.1016/j.desal.2006.05.007
Pontefract, A., Zhu, T. F., Walker, V. K., Hepburn, H., Lui, C., Zuber, M. T., et al. (2017). Microbial diversity in a hypersaline sulfate lake: a terrestrial analog of ancient Mars. Front. Microbiol. 8:1819. doi: 10.3389/fmicb.2017.01819
Price-Whelan, A., Poon, C. K., Benson, M. A., Eidem, T. T., Roux, C. M., Boyd, J. M., et al. (2013). Transcriptional profiling of Staphylococcus aureus during growth in 2 M NaCl leads to clarification of physiological roles for Kdp and Ktr K+ uptake systems. mBio 4:e00407-13. doi: 10.1128/mBio.00407-13
Rea, S., McSweeney, N., Degens, B., Morris, C., Siebert, H., and Kaksonen, A. (2015). Salt-tolerant microorganisms potentially useful for bioleaching operations where fresh water is scarce. Min. Eng. 75, 126–132. doi: 10.1016/j.mineng.2014.09.011
Roeßler, M., and Müller, V. (2001). Osmoadaptation in bacteria and archaea: common principles and differences. Environ. Microbiol. 3, 743–754. doi: 10.1046/j.1462-2920.2001.00252.x
Rohwerder, T., Gehrke, T., Kinzler, K., and Sand, W. (2003). Bioleaching review part A: progress in bioleaching: fundamentals and mechanisms of bacterial metal sulfide oxidation. Appl. Microbiol. Biotechnol. 63, 239–248. doi: 10.1007/s00253-003-1448-7
Rutherford, K., Parkhill, J., Crook, J., Horsnell, T., Rice, P., Rajandream, M. A., et al. (2000). Artemis: sequence visualization and annotation. Bioinformatics 16, 944–945. doi: 10.1093/bioinformatics/16.10.944
Saum, S. H., and Müller, V. (2007). Salinity-dependent switching of osmolyte strategies in a moderately halophilic bacterium: glutamate induces proline biosynthesis in Halobacillus halophilus. J. Bacteriol. 189, 6968–6975. doi: 10.1128/jb.00775-777
Saum, S. H., and Müller, V. (2008). Regulation of osmoadaptation in the moderate halophile Halobacillus halophilus: chloride, glutamate and switching osmolyte strategies. Saline Syst. 4:4. doi: 10.1186/1746-1448-4-4
Shabala, S., and Shabala, L. (2011). Ion transport and osmotic adjustment in plants and bacteria. Biomol. Concepts 2, 407–419. doi: 10.1515/BMC.2011.032
Shiers, D. W., Blight, K. R., and Ralph, D. E. (2005). Sodium sulphate and sodium chloride effects on batch culture of iron oxidising bacteria. Hydrometallurgy 80, 75–82. doi: 10.1016/j.hydromet.2005.07.001
Simmons, S., and Norris, P. (2002). Acidophiles of saline water at thermal vents of Vulcano, Italy. Extremophiles 6, 201–207. doi: 10.1007/s007920100242
Sleator, R. D., and Hill, C. (2002). Bacterial osmoadaptation: the role of osmolytes in bacterial stress and virulence. FEMS Microbiol. Rev. 26, 49–71. doi: 10.1111/j.1574-6976.2002.tb00598.x
Snipen, L., and Ussery, D. W. (2010). Standard operating procedure for computing pangenome trees. Stand. Genomic Sci. 2:135. doi: 10.4056/sigs.38923
Suyama, M., Torrents, D., and Bork, P. (2006). PAL2NAL: robust conversion of protein sequence alignments into the corresponding codon alignments. Nucleic Acids Res. 34, W609–W612. doi: 10.1093/nar/gkl315
Suzuki, I., Lee, D., Mackay, B., Harahuc, L., and Oh, J. K. (1999). Effect of various ions, ph, and osmotic pressure on oxidation of elemental sulfur by Thiobacillus thiooxidans. Appl. Environ. Microbiol. 65, 5163–5168.
Tettelin, H., Riley, D., Cattuto, C., and Medini, D. (2008). Comparative genomics: the bacterial pan-genome. Curr. Opin. Microbiol. 11, 472–477. doi: 10.1016/j.mib.2008.09.006
Tosca, N. J., Knoll, A. H., and McLennan, S. M. (2008). Water activity and the challenge for life on early Mars. Science 320, 1204–1207. doi: 10.1126/science.1155432
Tosca, N. J., McLennan, S. M., Lamb, M. P., and Grotzinger, J. P. (2011). Physicochemical properties of concentrated martian surface waters. J. Geophys. Res. 116:E05004. doi: 10.1029/2010JE003700
Tran, T. T. T., Mangenot, S., Magdelenat, G., Payen, E., Rouy, Z., Belahbib, H., et al. (2017). Comparative genome analysis provides insights into both the lifestyle of Acidithiobacillus ferrivorans strain CF27 and the chimeric nature of the iron-oxidizing Acidithiobacilli genomes. Front. Microbiol. 8:1009. doi: 10.3389/fmicb.2017.01009
Tyson, G. W., Chapman, J., Hugenholtz, P., Allen, E. E., Ram, R. J., Richardson, P. M., et al. (2004). Community structure and metabolism through reconstruction of microbial genomes from the environment. Nature 428, 37–43. doi: 10.1038/nature02340
Vago, J. L., Westall, F., Coates, A. J., Jaumann, R., Korablev, O., Ciarletti, V., et al. (2017). Habitability on early Mars and the search for biosignatures with the ExoMars Rover. Astrobiology 17, 471–510. doi: 10.1089/ast.2016.1533
Valdés, J., Cárdenas, J. P., Quatrini, R., Esparza, M., Osorio, H., Duarte, F., et al. (2010). Comparative genomics begins to unravel the ecophysiology of bioleaching. Hydrometallurgy 104, 471–476. doi: 10.1016/j.hydromet.2010.03.028
Valdés, J., Pedroso, I., Quatrini, R., and Holmes, D. S. (2008). Comparative genome analysis of Acidithiobacillus ferrooxidans, A. thiooxidans and A. caldus: Insights into their metabolism and ecophysiology. Hydrometallurgy 94, 180–184. doi: 10.1016/j.hydromet.2008.05.039
Waditee, R., Hibino, T., Tanaka, Y., Nakamura, T., Incharoensakdi, A., Hayakawa, S., et al. (2002). Functional characterization of betaine/proline transporters in betaine-accumulating mangrove. J. Biol. Chem. 277, 18373–18382. doi: 10.1074/jbc.M112012200
Wang, H., Li, Q., Fang, Y., Yu, S., Tang, B., Na, L., et al. (2016). Biochemical and functional characterization of the periplasmic domain of the outer membrane protein a from enterohemorrhagic Escherichia coli. Microbiol. Res. 182, 109–115. doi: 10.1016/j.micres.2015.10.004
Watling, H. (2016). Microbiological advances in biohydrometallurgy. Minerals 6:49. doi: 10.3390/min6020049
Zammit, C. M., Mangold, S., Jonna, V., Mutch, L. A., Watling, H. R., Dopson, M., et al. (2012). Bioleaching in brackish waters—effect of chloride ions on the acidophile population and proteomes of model species. Appl. Microbiol. Biotechnol. 93, 319–329. doi: 10.1007/s00253-011-3731-3733
Zammit, C. M., Mutch, L. A., Watling, H. R., and Watkin, E. L. J. (2009). The characterization of salt tolerance in biomining microorganisms and the search for novel salt tolerant strains. Adv. Mater. Res. 71-73, 283–286. doi: 10.4028/www.scientific.net/AMR.71-73.283
Zammit, C. M., and Watkin, E. L. J. (2016). “Adaptation to extreme acidity and osmotic stress,” in Acidophiles: Life in Extremely Acidic Environments, eds R. Quatrini and D. B. Johnson (Norfolk: Caister Academic Press), 49–62. doi: 10.21775/9781910190333.03
Zhang, X., Feng, X., Tao, J., Ma, L., Xiao, Y., Liang, Y., et al. (2016a). Comparative genomics of the extreme acidophile Acidithiobacillus thiooxidans reveals intraspecific divergence and niche adaptation. Int. J. Mol. Sci. 17:1355. doi: 10.3390/ijms17081355
Zhang, X., Liu, X., Liang, Y., Fan, F., Zhang, X., and Yin, H. (2016b). Metabolic diversity and adaptive mechanisms of iron-and/or sulfur-oxidizing autotrophic acidophiles in extremely acidic environments. Environ. Microbiol. Rep. 8, 738–751. doi: 10.1111/1758-2229.12435
Zhou, H.-B., Zhang, R.-B., Hu, P.-L., Zeng, W.-M., Xie, Y.-J., Wu, C.-B., et al. (2008). Isolation and characterization of Ferroplasma thermophilum sp. nov., a novel extremely acidophilic, moderately thermophilic archaeon and its role in bioleaching of chalcopyrite. J. Appl. Microbiol. 105, 591–601. doi: 10.1111/j.1365-2672.2008.03807.x
Keywords: acidophile, halophile, Acidihalobacter, osmoprotectant, ectoine, astrobiology
Citation: Khaleque HN, González C, Shafique R, Kaksonen AH, Holmes DS and Watkin ELJ (2019) Uncovering the Mechanisms of Halotolerance in the Extremely Acidophilic Members of the Acidihalobacter Genus Through Comparative Genome Analysis. Front. Microbiol. 10:155. doi: 10.3389/fmicb.2019.00155
Received: 16 November 2018; Accepted: 22 January 2019;
Published: 08 February 2019.
Edited by:
Claudia P. Saavedra, Universidad Andrés Bello, ChileReviewed by:
Mario A. Vera, Pontificia Universidad Católica de Chile, ChileNicolas Guiliani, Universidad de Chile, Chile
Copyright © 2019 Khaleque, González, Shafique, Kaksonen, Holmes and Watkin. This is an open-access article distributed under the terms of the Creative Commons Attribution License (CC BY). The use, distribution or reproduction in other forums is permitted, provided the original author(s) and the copyright owner(s) are credited and that the original publication in this journal is cited, in accordance with accepted academic practice. No use, distribution or reproduction is permitted which does not comply with these terms.
*Correspondence: Elizabeth L. J. Watkin, RS5XYXRraW5AY3VydGluLmVkdS5hdQ==