- Genecology Research Centre, The University of the Sunshine Coast, Maroochydore, QLD, Australia
Chlamydia is a major bacterial pathogen that infects humans, as well as a wide range of animals, including marsupials, birds, cats, pigs, cattle, and sheep. Antibiotics are the only treatment currently available, however, with high rates of re-infection, there is mounting pressure to develop Chlamydia vaccines. In this review, we analyzed how Chlamydia vaccine trials have developed over the past 70 years and identified where future trials need to be focused. There has been a strong bias toward studies targeting C. muridarum and C. trachomatis within mice and a lack of studies matching chlamydial species to their end target host. Even though a large number of specific antigenic targets have been studied, the results from whole-cell vaccine targets show slightly more promising results overall. There has also been a strong bias toward systemic vaccine delivery systems, despite the finding that mucosal delivery systems have shown more promising outcomes. However, the only successful vaccines with matched chlamydial species/infecting host are based on systemic vaccine delivery methods. We highlight the extensive work done with mouse model trials and indicate that whole cell antigenic targets are capable of inducing an effective response, protecting from disease and reducing shedding rates. However, replication of these results using antigen preparations more conducive to commercial vaccine production has proven difficult. To date, the Major Outer Membrane Protein (MOMP) has emerged as the most suitable substitute for whole cell targets and its delivery as a combined systemic and mucosal vaccine is most effective. Finally, although mouse model trials are useful, differences between hosts and infecting chlamydial strains are preventing vaccine formulations from mouse models to be translated into larger animals or intended hosts.
Introduction
Chlamydiae are gram-negative, obligate intracellular pathogens that infect eukaryotic cells (Oehme et al., 1991). There are currently 16 classified and / or formally proposed species that comprise the Chlamydiaceae family and these species infect a wide range of hosts and anatomical sites (Table 1 and Figure 1) (Taylor-Brown and Polkinghorne, 2017). Vaccines are being developed to target some of these chlamydial species for a variety of reasons (Table 2). Vaccines targeting human pathogens are designed to protect human health, while vaccines targeting livestock and wildlife pathogens aim to prevent economic damage, protect endangered animals and prevent zoonotic disease transmission. Although these 16 species of Chlamydia infect a range of different hosts, the site of infection and disease pathology within hosts are highly similar, indicating commonalities between a seemingly diverse group of chlamydial organisms.
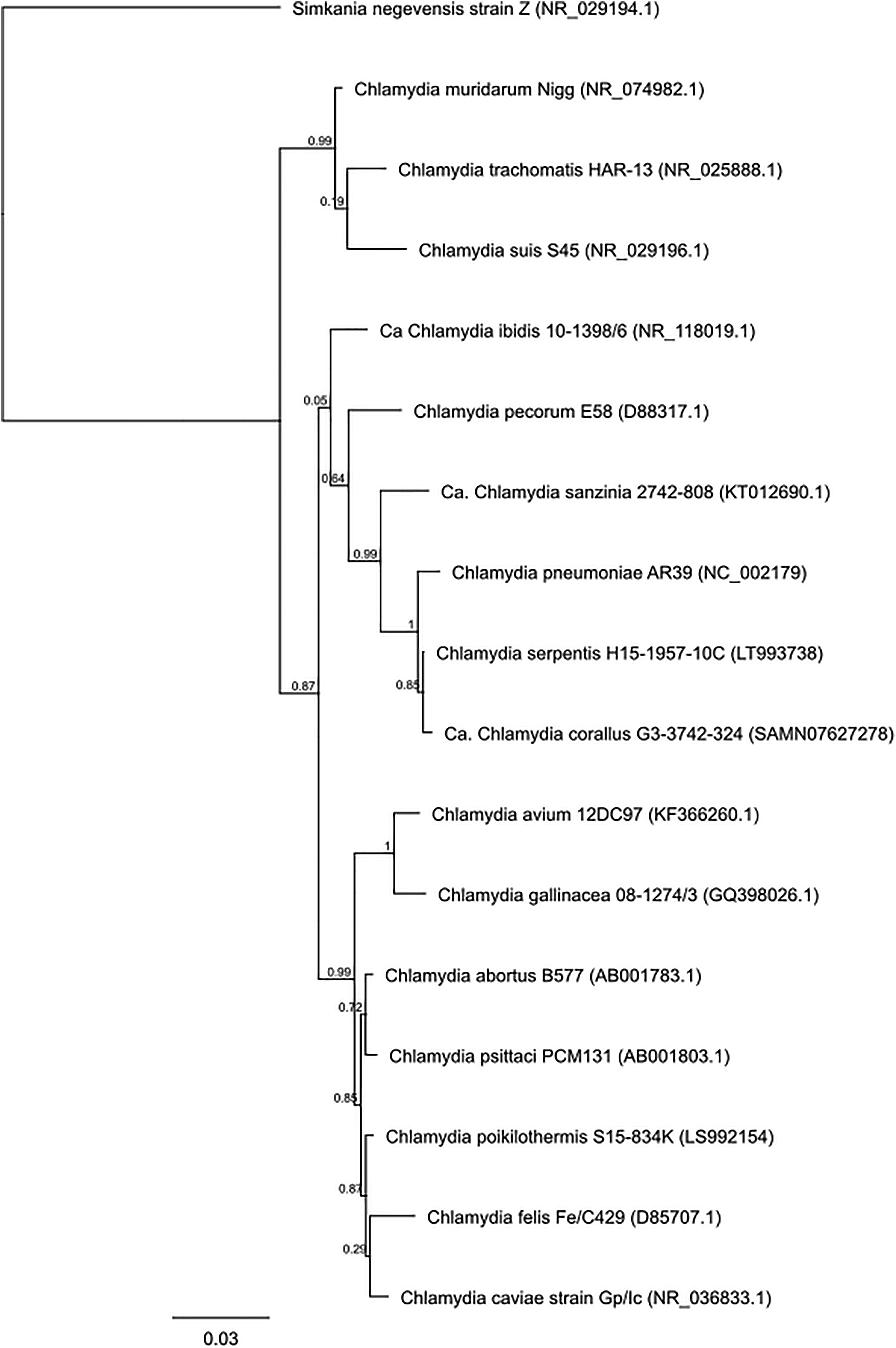
Figure 1. Phylogenetic tree analysis of the family Chlamydiaceae. Approximate likelihood phylogenetic tree analysis, MAFFT alignment of NCBI (Genbank) 16S sequences (1,587 bp) assembled using Geneious v 11.1.4.
Human Pathogenic Species
Chlamydia trachomatis has been dived into 13 different genotypes based on the major outer membrane protein (MOMP) (Stevens et al., 2010). Genotypes A, B, and C infect the conjunctiva of humans leading to active and scarring trachoma and eventually blindness (Garland et al., 1995). Genotypes D – K and L1 – L3 predominantly infect the urogenital tract, leading to inflammation, scarring and infertility. In women, these genotypes can also result in pelvic inflammatory disease, which increases the risk of ectopic pregnancy (Menon et al., 2015). It has been reported that up to 80% of C. trachomatis infections are asymptomatic (no signs of pathology), resulting in individuals who are unaware they are infected and leading to an extremely high rate of transmission (Korenromp et al., 2002; Farley et al., 2003; Ljubin-Sternak and Mestrovic, 2014; Menon et al., 2015).
Chlamydia pneumoniae predominantly infects the respiratory tract of humans leading to pneumonia (Shi et al., 2002; Kurz et al., 2009) as well as having some links to atherosclerosis, Alzheimer’s disease and asthma (Balin et al., 1998; Daba et al., 2002; Deniset et al., 2010; Iramain et al., 2016). In addition, C. pneumoniae has been reported in a range of animals such as mice, pigs, marsupials, birds, cats, and livestock, leading to respiratory disease (Borel et al., 2018).
Animal Pathogenic Species With Zoonotic Potential
Other species of Chlamydia infect a wide range of animals leading to disease and reported zoonotic potential (Li et al., 2017; Jelocnik et al., 2018; Pisanu et al., 2018; Torres-Mejía et al., 2018).
Chlamydia psittaci is a respiratory and reproductive pathogen of birds with zoonotic potential for humans. C. psittaci disease (psittacosis) outbreaks in humans date back to 1879 where humans were infected from pet parrots and finches. In the 1930s, human pandemic outbreaks were linked to racing pigeons imported from South America to Europe and North America. More recently, human psittacosis outbreaks throughout Europe have been linked to turkey and duck farming (Beeckman and Vanrompay, 2009). Broadly, a recent review and meta-analysis demonstrated that C. psittaci is the causative agent in 1% of worldwide community-acquired pneumonia (Hogerwerf et al., 2017).
Chlamydophila abortus predominantly infects the placenta of livestock resulting in fetal death and has the zoonotic potential to cause abortions in women if infected during pregnancy (Szeredi and Bacsadi, 2002; DeGraves et al., 2004; Meijer et al., 2004; Masala et al., 2007).
Chlamydophila felis infects the respiratory tract and conjunctiva of cats, leading to respiratory disease and conjunctivitis, respectively (Sykes, 2001; Cai et al., 2002; Rampazzo et al., 2003). C. felis has also been reported in as many as eight different zoonotic transmission events, however, these all occurred within immunocompromised humans (Browning, 2004). Other chlamydial species identified with zoonotic potential include C. caviae in guinea pigs, where three unrelated, zoonotic transmission events were reported in healthy adult humans, presenting with respiratory failure due to severe community-acquired pneumonia (Ramakers et al., 2017). Finally, C. suis has been detected from farm workers who have close contact to pigs, however no clinical symptoms of illness has yet been reported, so further research is needed to evaluate the potential risk to people (De Puysseleyr et al., 2017).
Other Animal Pathogenic Species
Chlamydia pecorum is one of the most diverse chlamydial species and can be separated into two genetically distinct clades based on host species. The first clade of C. pecorum infects the conjunctiva, limb joints and urogenital tract of livestock, leading to conjunctivitis, arthritis, cystitis and the development of reproductive cysts resulting in infertility (Reinhold et al., 2011; Jelocnik et al., 2014; Walker et al., 2016, 2018; Bommana et al., 2018). Furthermore, gastrointestinal infections have been shown to cause overall health deterioration, including increased body temperature and decreased body weights (Reinhold et al., 2008, 2011; Li et al., 2016). The second clade of C. pecorum infect the conjunctiva, and urogenital tract of predominantly koalas, leading to keratoconjunctivitis, cystitis and the development of reproductive cysts resulting in infertility (Burnard et al., 2017).
Chlamydia suis strains infect the conjunctiva, gastrointestinal tract and respiratory tract of pigs, resulting in a range of diseases including conjunctivitis (pink eye), intestinal lesions and respiratory disease (Rogers and Andersen, 2000; Sachse et al., 2004; Reinhold et al., 2005; Becker et al., 2007). C. suis has also been reported from pig oviducts and uteri, however links to disease development at these anatomical sites is yet to be established (Kauffold et al., 2006).
Chlamydia avium, C. ibidis and C. gallinacea are all predominantly infections of birds, infecting the respiratory tract and resulting in respiratory disease (Mitura et al., 2014; Guo et al., 2016; Chu et al., 2017).
Chlamydia serpentis, C. poikilothermis, C. corallus, and C. sanzinia are recently discovered species of Chlamydia which have been identified from cloacal and choanal samples of captive and wild snakes, with C. sanzinia also recently identified from cloacal and pharyngeal samples of turtles and tortoises. These newly identified species have very little known about their pathogenic potential (Taylor-Brown et al., 2016, 2017; Mitura et al., 2017; Staub et al., 2018).
Surrogate Models
Finally, C. muridarum in mice and C. caviae in guinea pigs have been used as models for chlamydial research. These two strains infect the urogenital tract and conjunctiva of their respective hosts, leading to hydrosalpinx and conjunctivitis, respectively (Andrew et al., 2011; Wali et al., 2014; De La Maza et al., 2017).
Mice and guinea pigs are used as surrogate models as their disease pathology mirrors diseases seen in humans, they have very similar biological process to humans and can be utilized in challenge trials, testing the effect of a vaccine candidate.
Immunological Response to Chlamydial Infections
For the successful clearance of chlamydial infections, cell-mediated and humoral immune response coordination is required. The humoral immune response to intracellular bacteria is a relatively new concept, with the common belief before 2004 being that humoral (antibody) immunity protected against extracellular bacteria and cellular immunity protected against intracellular pathogens (Casadevall, 2003, 2004). However, the use of B cell deficient mice and the identification of monoclonal antibodies have shed new light on the remarkable complexity of antibody mediated immunity (AMI) (Casadevall and Pirofski, 2006). Many studies have now shown that the appearance of serum antibodies strongly correlates with chlamydial clearance (Casadevall and Pirofski, 2006). Furthermore, the presence of IgA within human vaginal secretions correlates with chlamydial clearance (Brunham et al., 1983). However, it is still well-established that a combined humoral and cellular mediated immune response is required for complete protection from chlamydial infection and disease progression (Williams et al., 1987; Ramsey et al., 1988). The cellular immune response requires the recruitment of macrophages, dendritic cells, natural killer cells and CD4/CD8 T cells to the mucosal site of infection (Brunham and Rey-Ladino, 2005; Vasilevsky et al., 2014). The primary cytokine involved in chlamydial clearance is interferon gamma (IFNγ). Stimulated through the interleukin (IL) 12 cytokine pathway (Trinchieri, 2003; Eyerich et al., 2010), in humans, IFNγ restricts the growth cycle of Chlamydia by depleting tryptophan through the indoleamine 2,3-dioxygenase (IDO) pathway. IFNγ also suppresses inflammation at the site of infection through the downregulation of the Th2 immune response, characterized by IL4 and IL10 (Natividad et al., 2005). Failure to downregulate Th2 results in negative feedback, depleting IFNγ (Holland et al., 1996). IL12 also stimulates cytokines TNFα and IL22 involved in epithelial protective immunity (Trinchieri, 2003; Eyerich et al., 2010; Zhao et al., 2015). In addition to the stimulatory effects on inflammation by IL12, evidence indicates that regulatory T cells (Tregs) balance this response through counter-inflammatory pathways (Faal et al., 2006).
Clearly, there is an incredibly complex interaction between chlamydia and the host immune system. It is not possible to monitor all aspects of the immune response during each vaccine trial, so the reported protection achieved from each trial must be summarized and inferred from the data available. As such, for the purposes of this review, trials demonstrating reduced disease pathology when challenged and achieving a decrease in chlamydial shedding rates (compared to controls) were denoted as having achieved partial protection, regardless of the immune response measured (humoral or cellular). Any study reporting no disease pathology and no detectable chlamydial organisms after challenge infection was denoted as having achieved full protection. Finally, trials failing to control the onset of disease pathology were denoted as no protection.
The purpose of this review is to highlight and summarize the breadth of chlamydial based vaccine trials performed to date and, based on this summary, recommend where future vaccine trial effort should be focused.
Overview of Chlamydia Vaccine Trials
Over the last 71 years (from 1946 to 2017), there have been a large number of documents (1,489) reported in the scientific literature relating to Chlamydia vaccine trials. A literature search of the “Scopus” database using keywords “Chlamydia AND vaccine” and limiting the results to full and short communications performed on living hosts to test any form of vaccine targeted toward any species of Chlamydia identifies 220 vaccine trials. This represents an incredible body of work that has encompassed different chlamydial species, vaccine formulations (Figure 2) and approaches. Interestingly, the past 10 years have shown the greatest interest in Chlamydia vaccine research, with an average of 12 vaccine studies per year. To understand where the Chlamydia vaccine field currently stands, it is worth breaking down the 220 trials to evaluate what has been done and how successful they were. All the studies referred to are listed in Supplementary Table S1.
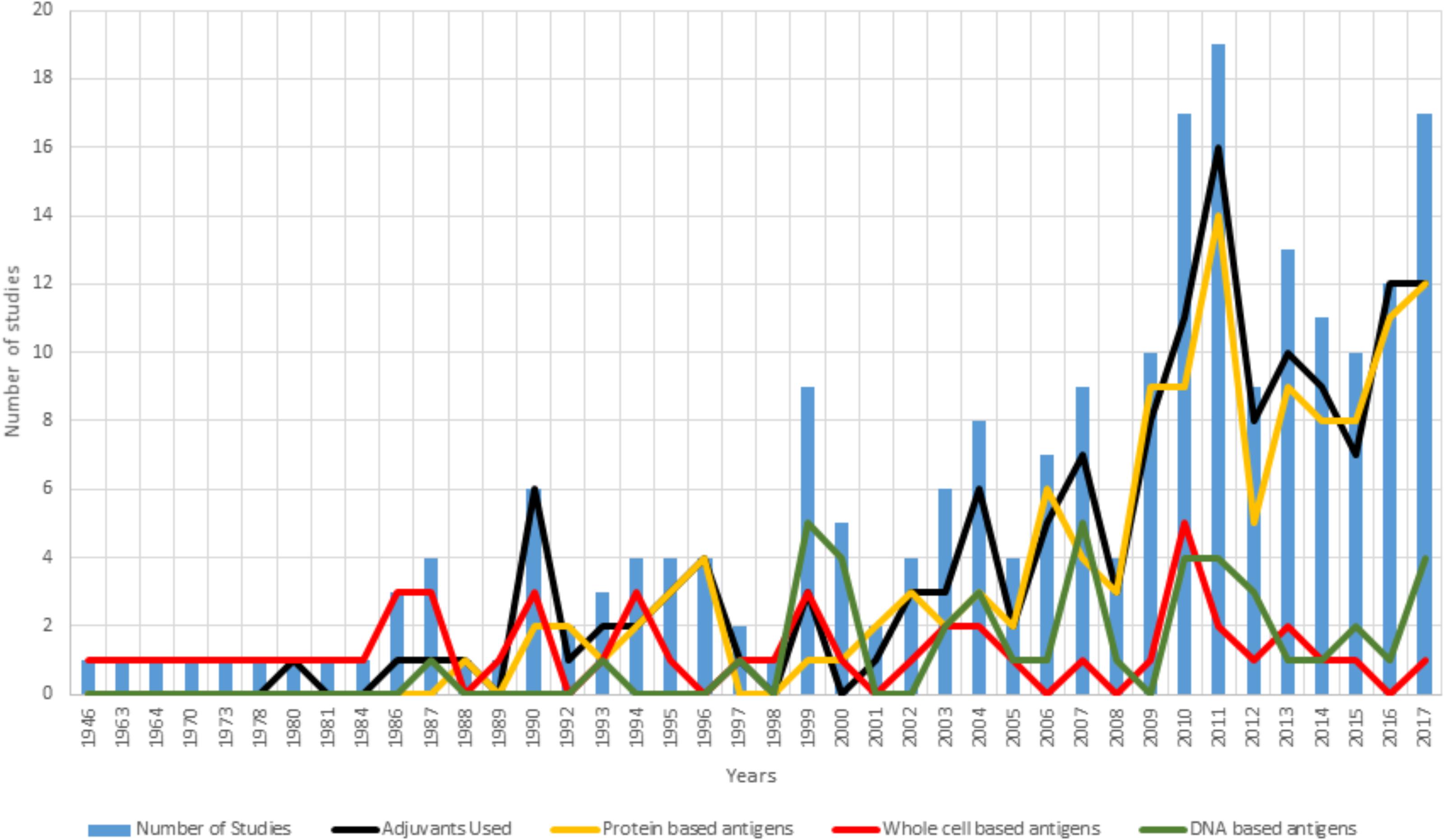
Figure 2. Summary of vaccine formulations between 1946 and 2017. For each year examined, the blue bars represent the number of vaccine trials published that year. Overlayed on the trial numbers is a break down the vaccine formulation tested by inclusion of an adjuvant (black line) and the use of either a protein-based antigen (yellow line), a whole cell antigen (attenuated or in-active) (red line) or a DNA-based antigen (green line).
Chlamydia and Host Species Targeted
From the 220 vaccine trials reported, eight chlamydial species and 12 host species have been targeted (Table 2 and Supplementary Table S1): The most targeted chlamydial species, C. muridarum, has been studied in 77 vaccine trials (35.0%) using mice (77 trials) and non-human primates (2 trials) as the host. C. trachomatis has been used in 67 vaccine trials (30.5%) tested in mice, non-human primates, pigs, guinea pigs, rabbits and a single human vaccine trial. C. psittaci has been used in 23 vaccine trials (10.5%) within a range of different hosts, including, mice, sheep, birds, Guinea pigs and cats. Vaccines to C. pneumoniae (6.4%) have only been tested in mice, vaccines to C. pecorum (5.5%) have been examined in koalas, mice and sheep, vaccines to C. abortus (5.0%) have been trialed in mice, cattle, sheep and pigs, vaccines to C. caviae (0.5%) have only been tested in guinea pigs and vaccines to C. felis (0.5%) have only been trialed in cats. Finally, nine trials (4.1%) have tested vaccines targeted for cross-species protection with a final 5 trials (2.3%) having used the resulting disease name (psittacosis or trachoma) and not a bacterial name as the identifier. This summary reveals that a staggering 85% of vaccine trials have been performed in substitute hosts and is concentrated around developing vaccines for humans. Overall human vaccine trials have been performed in mice, non-human primates, guinea pigs, rabbits and pigs. The only other vaccine trials conducted in a surrogate host were for the development of a sheep vaccine (Table 2). The majority (69.7%) of matched chlamydial species/infected host trials have being conducted within only two species, koalas and sheep. This indicates a major weakness in current Chlamydia vaccine research.
Target Antigens
There have been many different forms of antigen used in vaccine formulation. Traditionally, elementary bodies (EBs) (either live, formalin-fixed, or UV-inactivated) and crude outer membrane preparations were the antigens of choice. More recently (since the 1990’s), antigens have expanded to include the use of recombinant proteins, synthetic peptides, expression vectors and naked DNA.
For the first 40 years of Chlamydia vaccine research, the only published antigens used were whole-cell preparations of either live attenuated bacteria (23 studies) or inactivated bacteria (29 studies) (Table 3 and Supplementary Table S1). Inactivation methods for Chlamydia EBs consisting of 45% UV, 41% formalin, 10% heat, and 4% other techniques. The first protein-based antigens appeared in 1988 and steadily increased to 130 studies by 2017 (57.7% of all vaccine studies) (Table 3). Despite the successes of whole cell vaccines, safety concerns and the relative costs around the production of a whole cell vaccine has led to significant research being focused on the production of a protein-based vaccine.
There have been three different types of protein-based vaccines used; 18.5% (24 studies) used crude outer membrane protein preparations, 41.5% (54 studies) used purified recombinant outer membrane proteins and 54.6% (71 studies) used over 143 individual and mixed recombinant and synthetic peptides (Table 3 and Supplementary Table S1). From this array of antigens, MOMP has emerged as the most tested protein (78 trials using MOMP and 71 using other antigenic targets). DNA sequencing and computational analysis has indicated that MOMP is the protein most likely responsible for the success observed in whole cell trials (Stephens et al., 1987; Stephens et al., 1998). The MOMP of Chlamydia species is approximately 40 kDa in size, with five genetically conserved domains and four variable domains that are used to determine the serovar within each species (Stephens et al., 1987, 1998; Stevens et al., 2010). These domains contain multiple T-cell and B-cell epitopes that have been shown to induce T-cell immunity and neutralizing antibodies (Caldwell and Perry, 1982; Baehr et al., 1988; Ortiz et al., 1996; Pal et al., 2005). Other chlamydial proteins utilized as antigenic targets have included the polymorphic membrane proteins (PMPs), a collection of surface exposed proteins with highly conserved regions (ideal for inducing cross genotype recognition) and are characterized as autotransporter adhesion molecules involved in early chlamydial infection processes (Vasilevsky et al., 2016). The chlamydial heat shock protein has also been utilized as an antigenic target for multiple trials and has been shown to induce a strong inflammatory response (Morrison, 1991). Another protein tested as an antigenic target has been the chlamydia protease-like activity factor (CPAF), which is a cytosol secreted protein with proposed virulence potential (Li et al., 2007).
In 1987, the first study to use a plasmid-based vaccine was published (Taylor and Prendergast, 1987). This study achieved negative results and no further plasmid-based work was reported for 12 years. In 1999, three groups published five vaccine trials using plasmids, with four showing partial protection (Brunham and Zhang, 1999; Pal et al., 1999; Vanrompay et al., 1999b; Zhang et al., 1999) (Table 3). These trials stimulated Chlamydia plasmid-based vaccine research, launching 26 studies over the next 17 years (Table 3 and Supplementary Table S1). There have also been 13 trials (5.5% of vaccine trials) involving naked DNA or adapted virus-based antigens (with 83% of these using either full or partial omp gene sequences) occurring sporadically since 1993 (Table 2 and Supplementary Table S1).
Adjuvants
Within the 220 chlamydial vaccine trials conducted, 73 studies reported no adjuvant present in the vaccine. The majority of these adjuvant-free formulations were for vaccines using either whole-cells or plasmid antigen sources (73%). Of the 147 trials that used an adjuvant, 10 different adjuvants were used in 5 or more distinct trials, with the most utilized adjuvant being CpG oligodeoxynucleotides (all classes) (31%) (Supplementary Table S1). The use of adjuvants in combination with protein-based vaccines (peptides or full-length MOMP) appears to improve vaccine effectiveness, with vaccine trials reporting a 24% increase in achieving partial protection (from 58 to 82%) and a 6% increase in achieving full protection (0 – 6%). Within the C. pecorum koala vaccine trials, a triple adjuvant mixture has been trialed with notable success. This adjuvant mixture contains a synthetic host defense peptide IDR-1002, known to be anti-inflammatory (Wu et al., 2017), a synthetic polyphosphazene polyelectrolyte poly [di (sodiumcarboxylatoethylphenoxy) phosphazene] (PCEP), a protein carrier shown to have immune-stimulating properties through acid functionalities (Andrianov et al., 2004) and polycytidylic acid (Poly I:C), a synthetic analog of double stranded ribonucleic acid that is recognized by toll-like receptor 3 (TLR3) and upregulates cytokines involved in Th2 immune responses (Alexopoulou et al., 2001; Matsumoto et al., 2002; Yamamoto et al., 2003; Cheng et al., 2011a). An advantage of this triple adjuvant formulation is that it allows for a single dose vaccine. Sadly, with over 50 different adjuvants having been tried in different vaccine formulations, all having unique mechanisms of action, only limited conclusions can be made by comparison.
Vaccine Delivery Sites
The methods for vaccine delivery within the published 220 vaccine studies were either systemically, mucosally or a combination of both (Table 4 and Supplementary Table S1). Systemic vaccination has involved 23 studies that used multiple body sites and 107 studies that used a single body site for vaccination, with the most common site being subcutaneous (51.4%), followed by intramuscular (29.9%), intraperitoneal (7.5%), intravenous (4.7%), epidermis (<2%), intra-abdominal (<2%), intradermal (<2%), and transcutaneous (<2%) (Table 4). Mucosal vaccination has involved two studies using multiple mucosal sites and 45 studies have used a single mucosal site, with the most common site being nasal (73.3%), followed by oral (11.1%), ocular (8.9%), vaginal (4.4%), and gastrointestinal (2.2%) (Table 4). Finally, 43 studies have used a combined approach with both systemic and mucosal vaccinations involving sites listed above (Table 4).
Vaccine Results, No Challenge
Of the 44 studies that did not include a post-vaccine Chlamydia challenge, 18 studies were performed in mice, 11 studies in koalas, four studies in sheep, and one or two in birds, pigs, humans, non-human primates and rabbits. In some of these studies, such as with the human and koala subjects, ethical restrictions most likely prevented the study design from including a challenge component (i.e., deliberate infection). This type of restriction will continue to be a limitation for vaccine design in some hosts and helps explain why the Chlamydia mouse model remains a popular research tool. The results of these no challenge trials varied and were dependant on the in vitro tests performed on collected samples, predominately focused on Chlamydia specific antibody responses (IgG and IgA). However, overall, 86% of the trials achieved results indicating vaccination induced a measurable antibody mediated immune response.
Chlamydia Challenge Trials
Post vaccination studies predominately use challenge trials to test the effectiveness of the trial vaccine, however, as challenge trials are only performed in the short term (<1 year), they only provide information on short term immunity. Observations of long lasting immunity (> 1 year) are lacking in chlamydial vaccine research. From 220 vaccine studies, 176 (80%) employed a Chlamydia challenge post-vaccination. These studies involved 159 mucosal challenge routes including urogenital (vaginal, ovarian, uterine, penile, and urethral) (57.1%), nasal (27.3%), ocular (8.7%), oral (0.6%), and multiple sites (4.3%) (Table 5 and Supplementary Table S1). Of the 17 studies that used systemic routes, challenge sites included intraperitoneal (47.1%), subcutaneous (29.4%), intradermal (17.6%), and intra-cerebral (5.9%) (Table 4 and Supplementary Table S1). There are no studies to date that have used a combined mucosal and systemic challenge method. This preference for mucosal-based challenge delivery methods is likely because studies are trying to replicate the natural infection routes of many Chlamydia species.
Of the challenge-based vaccine studies, five different vaccination/challenge methods were implemented; (1) mucosal route of vaccination with a mucosal challenge (41 studies), (2) mucosal route of vaccination with systemic challenge (2 studies), (3) systemic route of vaccination with a mucosal challenge (81 studies), (4) systemic route of vaccination with a systemic challenge (15 studies) and (5) mixed route of vaccination with a mucosal challenge (37 studies). The most common type of vaccine was trials on mice using protein-based vaccines and CpG oligodeoxynucleotides (all classes) adjuvants with 39 trials (16.4%), while the next closest focus was on koalas using a protein-based antigen with 11 trials (5%). From all 176 challenged-based vaccine studies, only 8.5% (15/176) reported complete protection (defined as the absence of pathology and bacterial shedding at challenge site) with 10 of these trials using either a whole cell or MOMP-based antigen (Supplementary Table S1). Interestingly, this group of complete protection trials included three peptide-based studies targeting human C. pneumoniae using the mouse model (Tammiruusu et al., 2007; Thorpe et al., 2007; Li et al., 2010). In fact, 66.7% (102 trials) of challenge trials were performed with chlamydial species infecting non-native hosts, meaning that future work will be needed to confirm the efficacy of tested vaccines in their target hosts. Focusing only on studies that matched chlamydial species to infecting host (73 studies), vaccine trials that used a mucosal route of vaccination achieved a measurable mucosal immune response in 80% (20/25) of trials. This compared to systemic vaccination, which showed 77% (37/48) of trials identifying a measurable mucosal immune response.
Vaccine Trials for Human Chlamydial Infections
The need for a vaccine to manage and reduce human chlamydial disease is well recognized. The prevalence of C. trachomatis is estimated at approximately 4.2% among 15 to 49-year-old men and women, making this pathogen the world’s most reported STI (Newman et al., 2015). C. trachomatis is known to cause serious urogenital and ocular disease outcomes (Garland et al., 1995; Stevens et al., 2010; Menon et al., 2015). However, with up to 80% of infections asymptomatic, most infections are not recognized and transmission to partners is common (Korenromp et al., 2002; Farley et al., 2003; Ljubin-Sternak and Mestrovic, 2014; Menon et al., 2015). C. trachomatis has been reported to have treatment failures (organisms detected after antibiotic treatment has finished) (Jones et al., 1990; Lefevre et al., 1997; Somani et al., 2000; Misiurina et al., 2004; Bhengraj et al., 2010), however no genetic link to macrolide or fluoroquinolone resistance has been reported (Deguchi et al., 2018). Furthermore, chlamydial persistence (organisms present in a non-infectious and non-replicating state) has also been reported in many studies. However, the high rate of individuals being reinfected from undiagnosed partners makes determining whether Chlamydia detected after treatment is the result of persistence, treatment failure or reinfection is complex. Recent studies targeting only actively replicating chlamydial cells have demonstrated that a high percentage of treatment failure cases are only identifying inactive cells (via DNA) as false positives for active infections (Janssen et al., 2016; Phillips et al., 2018). The foundational work necessary for developing a C. trachomatis vaccine for human use has taken place in mouse and non-human primate model systems. Here we discuss the range of vaccine trials used in mouse model trials, the rationality for progression to new complex vaccines and the relative effectiveness of each vaccine make up.
Mouse Model Vaccine Trials
Chlamydia muridarum is known to infect mice and rats causing conjunctivitis, respiratory disease and urogenital disease. The mouse model has been used as a host for vaccination trials because it is an amenable animal model, enabling post vaccination challenge experiments and detailed analysis of immune responses. The main purpose of studying chlamydial vaccines within the mouse model is for future development of a human chlamydial vaccine. Due to differences in the efficiency of certain strains within the mouse model, many studies chose to use C. muridarum as a surrogate for C. trachomatis.
Early mouse model trials for Chlamydia vaccine research started in the late 1940s and were designed to target C. psittaci (known now to be C. muridarum post re-classification of nomenclature) infections using live and inactivated whole cell preparation vaccines (Morgan and Wiseman, 1946). These early trials used rates of chlamydial shedding post challenge as a measure of effectiveness and were successful in achieving some protection from bacterial challenge (Morgan and Wiseman, 1946; Mitzel et al., 1970; Johnson and Hobson, 1986; Rekiki et al., 2004b). However, since the 1990s, the predominant focus of mouse model vaccines has been using C. muridarum and C. trachomatis strains. Live whole cell vaccines have demonstrated the feasibility of inducing a protective response to Chlamydia infections. Several trials have shown that using live chlamydial EBs can induce complete protection through intranasal vaccination (Pal et al., 1994; Peterson et al., 1999; Lu et al., 2002). However, further trials have failed to reproduce these results using inactivated EBs, which is thought to be the result of lowered peptide loading on to dendritic cells of inactivated EBs compared to active EBs (De La Maza et al., 2017).
The first MOMP-based vaccine in mice was by Tuffrey et al. (1992). Using a recombinant MOMP (rMOMP) from C. trachomatis serovar L1 and direct vaccination into the Peyer’s patches or oviducts, the trial failed to reduce colonization or disease development. However, humoral immune responses in plasma anti-MOMP IgG were detected along with trace levels of mucosal anti-MOMP IgA. This disappointing result was repeated in six additional protein-based vaccine trials over the next 20 years, with the only improvement being that the increases in plasma IgG responses had Chlamydia neutralizing effects (Su and Caldwell, 1993; Knight et al., 1995; Igietseme and Murdin, 2000; Shaw et al., 2002; Zheng et al., 2006; Jiang et al., 2015). None of these seven trials used an adjuvant to stimulate immune responses during vaccination, generating a clear indication that an adjuvant-based vaccine would be required for a successful immunological response within a protein-based vaccine.
The first evidence of a protein vaccine inducing protection in mice was by Pal et al. (1997). The vaccine consisted of a detergent prepared chlamydial outer membrane complex (COMC) or denatured MOMP protein in Freund’s incomplete adjuvant. This vaccine, delivered subcutaneously, decreased chlamydial shedding rates and induced protection from disease post-ovarian challenge, with the COMC formulation outperforming the MOMP formulation. The promising results were replicated by Pal et al. (2001) with the MOMP protein, whereby a native MOMP (nMOMP) preparation outperformed the denatured MOMP preparation. Cellular immune responses were enhanced in the nMOMP vaccinated mice and humoral immune responses were slightly higher in the denatured MOMP preparation. These results indicated that conservation of the native protein structure resulted in increased protection from disease and that this is driven by both cellular and humoral immune responses. Pal and colleagues went on to reproduce these results in another three trials testing different adjuvants (CpG and Montanide ISA 720) using nMOMP as the antigen. Furthermore, they also observed similar levels of protection between nMOMP vaccines and live EB vaccines post-intrabursal challenge (Pal et al., 1997, 2005). This was significant, as protein-based vaccines had not performed as well as whole cell vaccines previously. Further mouse model studies into the protective effects of nMOMP have been demonstrated in the respiratory tract (Sun et al., 2009) and the genital tract (Farris et al., 2010; Carmichael et al., 2011; Tifrea et al., 2011). These studies confirm that nMOMP induces protection from disease and decreased bacterial shedding from Chlamydia challenge. They also conclude that a cellular and humoral immune response is required for complete protection.
Although native protein vaccine production is less expensive than whole cell vaccine production, antigen in this format is still relatively costly. As such, significant recent research has been focused on the production of a recombinant MOMP (rMOMP) vaccine (where the desired protein antigen is cloned, expressed and purified from Escherichia coli in large quantities instead of purified directly from cultured chlamydial cells). Since 1992, there have been 38 different vaccine trials using an rMOMP antigen with a total of 14 different adjuvants. The first reports of a successful rMOMP vaccine was reported by Berry et al. (2004) using a cholera toxin and CpG adjuvant through a transdermal vaccination route (Berry et al., 2004). They observed both humoral and cellular immune responses that included anti-chlamydial plasma and mucosal IgG and mucosal IgA and IFNγ-secreting T cells. Together, these responses reduced chlamydial shedding and enhanced protection against pathology (Berry et al., 2004). Further trials generated comparable results using transdermal, oral and intranasal vaccination routes (Hickey et al., 2004, 2009, 2010). Additional vaccination route studies demonstrated that combining both mucosal and systemic vaccination routes induced an enhanced immune response compared to using a mucosal route alone. Ralli-Jain et al. (2010), Carmichael et al. (2011) and Cheng et al. (2014) all observed that vaccinations with rMOMP and adjuvants CpG and Montanide induced the strongest humoral and cellular immune responses (IgG, IgA and neutralization effect and T-cell proliferation) with a dosage regime of a mucosal priming dose, followed by a systemic boosting dose.
Further insights into the required cellular immune response were observed by O’Meara et al. (2013). They tested an rMOMP antigen with a cholera toxin/CpG adjuvant and various vaccination routes (transdermal, sublingual or intranasal) and observed a relationship between the production of IFNγ, TNFα, and IL17 and protection against infection (O’Meara et al., 2013). Further studies in 2016 (using nMOMP or rMOMP antigens with CAF01 and CAF09 adjuvants) also observed a correlation between an increased production of IFNγ and IL17 and low levels of IL4 with significantly reduced bacterial shedding and protection against respiratory pathology (Pal et al., 2017b).
In the last 3 years, there has been a significant focus on identifying the specific chlamydial outer membrane protein epitopes that induce the highest antigenic response, with a particular focus on the MOMP and PMPs. Pal et al. (2017a) completed one of the largest of these trials, comparing the vaccination effects of nine different PMPs from C. trachomatis in mice and challenged with C. muridarum. They identified that, although PMPs C, G and H individually elicited the highest levels of humoral and cellular immune responses and decreased signs of disease of the nine different PMP antigens tested, these results were still lower than the more effective C. muridarum MOMP vaccine response (Pal et al., 2017a). These results show that the complexities involved with inducing a protective response to chlamydial infections involves the recognition of multiple epitopes. This further indicates that the success of a future chlamydial vaccine will probably be dependent on large recombinant proteins such as the MOMP.
Non-human Primate Vaccine Trials
One of the most difficult aspects of Chlamydia vaccine research is replicating mouse vaccine trial results in other host species, specifically non-human primates. Over the last 30 years, there have been only 12 attempts to replicate mouse model trial results in non-human primates. The first set of non-human primate trials utilized whole cell antigenic targets and observed limited to no protection post challenge (Chang et al., 1964; Whittum-Hudson et al., 1986; Taylor et al., 1987). In fact, one study observed increased chlamydial pathology in vaccinated owl monkeys post ocular challenge (MacDonald et al., 1984). However, recent whole cell antigen vaccines have produced promising results. Kari et al. (2009, 2011) vaccinated Cynomolgus macaques (via the ocular route) with a plasmid deficient strain of C. trachomatis and observed increased serum AMI, specifically IgG and IgA, with neutralizing properties, upregulation of both IFNγ and IL12 [from peripheral blood mononuclear cells (PBMC) fractions] and demonstrated protection from ocular challenge in five out of six vaccinated animals (Kari et al., 2009, 2011). These results were repeated in 2014 by the same group, using a systemic vaccination regime (Olivares-Zavaleta et al., 2014). Alternative approaches to reducing EB virulence, such as using a plasmid negative chlamydial strain, failed to induce protection in rhesus macaques (Qu et al., 2015).
Sadly, protein based vaccine trials in non-human primates have shown little to no promise, with six separate trials observing some non-significant increases in both humoral and cell mediated immunity that failed to induce complete protection from disease (Taylor et al., 1987, 1988; Su and Caldwell, 1993; Campos et al., 1995; Kari et al., 2009; Cheng et al., 2011b).
Mouse model trials highlight the complexities involved in producing a vaccine response that will protect from infection and development of disease. Although some success has been achieved through live vaccines and MOMP-based vaccines, failure to reproduce these results in non-human primate models is most likely due to the differences in both host immunity and Chlamydia species genetics. Important differences within the tryptophan synthase genes between C. muridarum and C. trachomatis have the potential to affect how each pathogen will be cleared by IFNγ and IDO pathways in different hosts. Despite this failure, mouse models have demonstrated important characteristics that should be considered in both administration of a Chlamydia vaccine and monitoring of the humoral and cellular immune responses. Together, this research identifies that a successful Chlamydia vaccine will combine a mucosal and systemic dose regime using a MOMP-based protein with an adjuvant. Success will likely be observed through a combination of (a) humoral immune responses including chlamydial specific plasma IgG and mucosal IgG and IgA responses with neutralizing capabilities and; (b) cellular immune responses, including upregulation of IFNγ, TNFα and IL17 and down regulation of IL4 and IL10.
Vaccine Trials for Animal Chlamydial Infections
Beyond the quest to develop a vaccine in mice and non-human primates to eventually combat C. trachomatis infection in humans, there are several chlamydial species of economic and wildlife welfare importance to merit vaccination programs of their own. So far, concerted effort has been focused on C. psittaci and C. pecorum for livestock applications and C. pecorum for disease management in koalas. To date, only two chlamydial vaccines are available commercially (as whole cell vaccine formulations) and they target C. felis in cats and C. abortus in sheep. While some of the lessons learnt with C. trachomatis and C. muridarum in the mouse model can be extrapolated to other chlamydial species and hosts, much work is still needed to tailor specific chlamydial vaccines to their specific purposes.
Livestock Vaccine Trials – C. psittaci Vaccines
Vaccines targeting C. psittaci have been trialed sporadically over the last 70 years with 23 studies occurring between 1978 and 2017. However, caution must be taken when comparing early and recent trials, as changes in 1999 to chlamydial nomenclature significantly altering the number of strains included under this once broad species (Everett et al., 1999; Stephens et al., 2009; Greub, 2010).
Of the 18 trials conducted prior to 1999, 67% utilized whole cell preparations as antigenic targets for use in sheep (six trials), guinea pigs (three trials), mice (two trials), and cats (one trial). These trials achieved partial protection from infection and the majority showed reductions in disease (Nichols et al., 1978; Malaty et al., 1981; Johnson and Hobson, 1986; Rodolakis and Souriau, 1986; Wills et al., 1987; Rank et al., 1990; Wilsmore et al., 1990a,b; Gajdosová et al., 1994; Westbay et al., 1994; Jones et al., 1995). There have been three studies utilizing outer membrane preparations (two in sheep and one in mice) and, although the results were promising, the reductions in disease (noted as abortion rates) were lower when compared to that observed from whole cell preparations (46 and 70% reduction, respectively) (Anderson et al., 1990; Tan et al., 1990; Sandbulte et al., 1996). Finally, in 1999, there were two trials conducted in turkeys utilizing a plasmid-based antigenic target and different vaccination routes (systemic and mucosal), however both these trials failed to induce a chlamydial specific antibody response (Vanrompay et al., 1999a,b).
Post 1999, there have been only five trials of C. psittaci vaccines. These trials utilized recombinant proteins (three trials) or plasmids in different vectors (eukaryotic and viral) (two trials). Results from all five studies observed partial protection from infection and/or disease; however, each utilized a different gene or protein making comparisons difficult (Loots et al., 2006; Qiu et al., 2010; Liu et al., 2015; Liang et al., 2016; Ran et al., 2017).
Livestock Vaccine Trials – C. pecorum Vaccines
There have only been two C. pecorum specific vaccine trials performed in non-koala hosts. Rekiki et al. (2004a), vaccinated mice with a commercial C. abortus (temperature-sensitive mutant) live cell vaccine and challenged with C. pecorum (sheep isolate) (Rekiki et al., 2004a). They reported some protection (as abortion rates and progeny health) post intraperitoneal challenge, however these pathologies are rarely related to C. pecorum infections (Rekiki et al., 2004a). Finally, Desclozeaux et al. (2017a), vaccinated pregnant ewes and lambs with a recombinant PmpG protein from C. pecorum strain IPA (sheep isolate) and a rMOMP from a C. pecorum genotype G strain (koala isolate) (Desclozeaux et al., 2017a). They observed increases in antibody mediated immunity (IgG and IgA) and cellular immunity (IFNγ), however, in vitro neutralization effects were absent and the cell mediated immune response durations appeared short (Desclozeaux et al., 2017a). These studies show some promise in producing an effective C. pecorum vaccine. However, as some or all of the antigenic targets utilized, in both these studies, differed to the infecting chlamydial species or strain of interest, these results should be interpreted with caution, with further studies required replicating natural conditions.
Koala Vaccine Trials
The development of a C. pecorum vaccine for koalas has involved a systematic research program that covers 11 different trials over a period of 7 years (Table 6 and Figure 2). The first koala C. pecorum vaccination trial tested the safety and immunogenicity of recombinant forms of C. muridarum proteins including MOMP, Ribonucleotide reductase (NrdB) and omp85 (CT0512), as well as adjuvant effects of Immunostimulating complex (ISC), Aluminum hydroxide gel (Alhydrogel) and TiterMax Gold (Carey et al., 2010). This first study vaccinated healthy captive female koalas, utilizing a three-dose vaccination regime and tested the effects of each adjuvant over a 270 days period. The results identified strong neutralizing (C. muridarum and C. pneumoniae) plasma derived IgG responses lasting for > 270 days using the ISC and Alhydrogel and cloacal IgG responses lasting for > 270 days in the ISC group (Carey et al., 2010). This study demonstrated that a Chlamydia based vaccine in koalas was possible and induced a significant increase in plasma and mucosal. While the results of the first trial were promising, they utilized antigens from C. muridarum, the mouse pathogen, rather than from the koala pathogen, C. pecorum. The next vaccine trial replaced C. muridarum rMOMP (genotype G) and NrdB with C. pecorum proteins and containing only the adjuvant ISC. This trial included koalas with signs of clinical disease (conjunctivitis and/or cystitis) and a group of healthy koalas receiving only two vaccine doses (all other groups received a three dose vaccination) (Kollipara et al., 2012). This study demonstrated similar plasma and mucosal IgG responses as in Carey et al. (2010) for both MOMP and NrdB, with MOMP eliciting a slightly higher elevation of the humoral immune response compared to NrdB. Interestingly, koalas with current signs of disease showed some clinical signs of improvement post vaccination and the two dose vaccination regime resulted in similar IgG levels as the three dose regime. The success of a koala specific C. pecorum targeted vaccine lead to three additional trials that (1) expanded the vaccine to be multi-variant by including multiple MOMP genotypes within a single vaccine to induce a broad antigenic memory (Kollipara et al., 2013b), (2) observed plasma antibodies derived from koalas immunized with rMOMP produced antibodies to both variable and conserved domains of MOMP (Kollipara et al., 2013a), and 3) found that different vaccination routes (mucosal via intranasal or systemic via sub-cutaneous) had differing affects (Waugh et al., 2015). Collectively, the koala specific C. pecorum vaccine trials indicated that a multivalent rMOMP vaccine, delivered via a subcutaneous and intranasal route, could elicit a cross-protective humoral and cellular immune response in wild koalas, with or without current Chlamydia infections and related signs of disease.
With previous trials observing strong immune responses to vaccination, further studies aimed to determine the vaccines ability to limit disease progression in wild koalas over extended periods (up to 12 months) (Khan et al., 2016b; Waugh et al., 2016b). Over two studies, koalas vaccinated with two doses of an rMOMP mixed genotype C. pecorum vaccine containing ISC adjuvant and were followed for 12 months. Vaccinated koalas had decreases in both C. pecorum infecting loads and disease prevalence at both six and 12 months post vaccination, as well as showing a significant increase in plasma IgG that recognized epitopes within the conserved domains of C. pecorum 6 months post vaccination (Waugh et al., 2016b). When compared to unvaccinated controls, naturally infected vaccinated koalas also had a significant increase in the neutralizing effect of the plasma derived immunoglobulins (Khan et al., 2016b).
One major drawback to the C. pecorum koala vaccine as it was formulated to date was that it required multiple vaccine doses for the ISC adjuvant to induce optimal protection. Given the challenge of a multiple vaccination strategy in a wildlife management program, a redesign of the adjuvant component was undertaken to allow for single dose implementation. In 2014, the mixed genotype rMOMPs were combined with a new adjuvant known as Tri-Adj, developed from the Veterinary Infectious Disease Organization (VIDO), Canada (Khan et al., 2014; Waugh et al., 2016a). The Tri-Adj combines three different components; a synthetic host defense peptide IDR-1002 (known to be anti-inflammatory; Wu et al., 2017), a synthetic polyphosphazene polyelectrolyte poly [di (sodiumcarboxylatoethylphenoxy) phosphazene] (PCEP) [a protein carrier shown to have immune-stimulating properties through acid functionalities (Andrianov et al., 2004)] and polycytidylic acid (Poly I:C) [a synthetic analog of double stranded ribonucleic acid that is recognized by toll-like receptor 3 (TLR3) and upregulates cytokines involved in Th2 immune responses (Alexopoulou et al., 2001; Matsumoto et al., 2002; Yamamoto et al., 2003; Cheng et al., 2011a)]. This new vaccine formulation was tested using a one and two dose regime with healthy koalas (Khan et al., 2014). Overall, comparable humoral and cellular immune responses were observed in both the single and double dose regimes, indicating the efficacy of a single dose C. pecorum vaccine in healthy koalas. Once a single dose regime was established, Khan et al. (2016a) sought to compare specific humoral and cellular immune responses between the old three dose ISC adjuvant regime and the new Tri-Adj single dose regime on free-ranging koalas using the mixed genotype rMOMP antigens. Humoral immune responses (plasma IgG) observed between the two groups showed an increased plasma IgG response from the three dose ISC vaccine group, however, when assessed for neutralizing effects on C. pecorum EBs, there were no differences observed between vaccine groups. As well, there were major differences in the C. pecorum epitopes recognized by plasma IgG from the ISC vaccine and the Tri-Adj vaccine group. The Tri-Adj vaccine group recognized two extra epitopes with a conserved domain and one extra epitope within variable domain compared to the ISC vaccine group. Collectively, these results solidified the advantages of the new Tri-Adj vaccine formulation over the old ISC vaccine formulation.
With the establishment of a single dose vaccine, the most recent trials have aimed at replicating the vaccine’s effectiveness on koalas with current signs of chlamydial disease over an extended time period (6 months) (Waugh et al., 2016a; Desclozeaux et al., 2017b) and testing the utility of expanding the antigenic targets included in the formulation to include PMPs along with rMOMPs (Desclozeaux et al., 2017b). The single dose rMOMP/Tri-Adj vaccine reduced the severity of the conjunctival pathology and C. pecorum DNA shedding in both vaccinated and antibiotic treated koalas and elicited a long lasting response to C. pecorum stimulus (in vitro) (Waugh et al., 2016a; Desclozeaux et al., 2017b). However, lasting protection from C. pecorum reinfection appeared elusive, as both rMOMP and rMOMP/PMP vaccine groups contained koalas with signs of chlamydial disease at a prevalence similar to unvaccinated controls. Further analysis of these C. pecorum strains indicated that these strains contained a large genetic diversity within the variable domain four of the ompA gene, known to contain T and B cell epitopes. Their conclusions noted that these observations indicate a possible pitfall in using a single antigenic region (MOMP) and that considerations should be made to develop a multi-antigenic vaccine with the possibility of including a mucosal administration site in combination with the current sub-cutaneous route.
This systematic series of vaccine trials identifies that with direction and persistence an effective chlamydial vaccine can be achieved within the intended host. Future directions for the koala vaccine may include alterations to vaccine formulations used, such as a second vaccine dose at a mucosal site, but with the foundation of work already completed the effects of the alterations of vaccine effectiveness can be accurately assessed.
Conclusion
This review has identified that, in over 70 years of vaccine research, with many advances in techniques and knowledge of the target species, no single antigen type or target, adjuvant, or route of administration has been established as a clear front-runner for effective vaccination. Extensive mouse model trials indicate that whole cell antigenic targets induce an effective response, protecting from disease and reducing shedding rates. However, replication of these results using more commercially acceptable antigenic preparations has proven difficult. Both mouse and koala trials indicate that MOMP is a highly recognized antigenic target and is a suitable substitute for whole cell targets. However, if MOMP is not combined with an appropriate adjuvant, it is ineffective. There is also evidence indicating a combined systemic and mucosal vaccine delivery is very effective, however, this is likely to depend on the target species, host and adjuvant used. Mouse model trials have been important in understanding the humoral and cellular immune responses required for an effective chlamydial vaccine, unfortunately replication of these responses has failed in larger animals including humans. These failures are likely due to the inherent differences in chlamydial – host interactions, such as IFNγ induction of p47 GTPase in mice and IDO in humans (Bonner et al., 2014).
With future trials utilizing closer related host species (i.e., non-human primates) with focus on the differences in these interactions and specific adjuvant combinations, it is plausible that an effective human vaccine is possible. With the first human phase 1 clinical trials currently underway, this establishes a major milestone for chlamydial vaccine development and will provide answers to many questions related to the effectiveness of the vaccine within the intended host.
Data Availability Statement
All datasets for this study are included in the manuscript and the Supplementary Files.
Author Contributions
SP, BQ, and PT contributed to the conceptualization. SP performed the data curation and analysis. SP, BQ, and PT contributed to construction and writing of the manuscript.
Conflict of Interest Statement
The authors declare that the research was conducted in the absence of any commercial or financial relationships that could be construed as a potential conflict of interest.
Supplementary Material
The Supplementary Material for this article can be found online at: https://www.frontiersin.org/articles/10.3389/fmicb.2019.00070/full#supplementary-material
TABLE S1 | List of all data collected from all trials included in analysis with associated references.
References
Alexopoulou, L., Holt, A. C., Medzhitov, R., and Flavell, R. A. (2001). Recognition of double-stranded RNA and activation of NF-kappaB by Toll-like receptor 3. Nature 413, 732–738. doi: 10.1038/35099560
Anderson, I. E., Tan, T. W., Jones, G. E., and Herring, A. J. (1990). Efficacy against ovine enzootic abortion of an experimental vaccine containing purified elementary bodies of Chlamydia psittaci. Vet. Microbiol. 24, 21–27. doi: 10.1016/0378-1135(90)90047-Y
Andrew, D. W., Hafner, L. M., Beagley, K. W., and Timms, P. (2011). Partial protection against chlamydial reproductive tract infection by a recombinant major outer membrane protein/CpG/cholera toxin intranasal vaccine in the guinea pig Chlamydia caviae model. J. Reprod. Immunol. 91, 9–16. doi: 10.1016/j.jri.2011.06.100
Andrianov, A. K., Svirkin, Y. Y., and LeGolvan, M. P. (2004). Synthesis and biologically relevant properties of polyphosphazene polyacids. Biomacromolecules 5, 1999–2006. doi: 10.1021/bm049745d
Baehr, W., Zhang, Y. X., Joseph, T., Su, H., Nano, F. E., Everett, K. D., et al. (1988). Mapping antigenic domains expressed by Chlamydia trachomatis major outer membrane protein genes. Proc. Natl. Acad. Sci. U.S.A. 85, 4000–4004. doi: 10.1073/pnas.85.11.4000
Balin, B. J., Gérard, H. C., Arking, E. J., Appelt, D. M., Branigan, P. J., Abrams, J. T., et al. (1998). Identification and localization of Chlamydia pneumoniae in the Alzheimer’s brain. Med. Microbiol. Immunol. 187, 23–42. doi: 10.1007/s004300050071
Becker, A., Lutz-Wohlgroth, L., Brugnera, E., Lu, Z. H., Zimmermann, D. R., Grimm, F., et al. (2007). Intensively kept pigs pre-disposed to chlamydial associated conjunctivitis. J. Vet. Med. Ser. A Physiol. Pathol. Clin. Med. 54, 307–313. doi: 10.1111/j.1439-0442.2007.00963.x
Beeckman, D. S. A., and Vanrompay, D. C. G. (2009). Zoonotic Chlamydophila psittaci infections from a clinical perspective. Clin. Microbiol. Infect. 15, 11–17. doi: 10.1111/j.1469-0691.2008.02669.x
Berry, L. J., Hickey, D. K., Skelding, K. A., Bao, S., Rendina, A. M., Hansbro, P. M., et al. (2004). Transcutaneous immunization with combined cholera toxin and CpG adjuvant protects against Chlamydia muridarum genital tract infection. Infect. Immun. 72, 1019–1028. doi: 10.1128/IAI.72.2.1019-1028.2004
Bhengraj, A. R., Vardhan, H., Srivastava, P., Salhan, S., and Mittal, A. (2010). Decreased susceptibility to azithromycin and doxycycline in clinical isolates of Chlamydia trachomatis obtained from recurrently infected female patients in India. Chemotherapy 56, 371–377. doi: 10.1159/000314998
Bommana, S., Walker, E., Desclozeaux, M., Jelocnik, M., Timms, P., Polkinghorne, A., et al. (2018). Molecular and serological dynamics of Chlamydia pecorum infection in a longitudinal study of prime lamb production. PeerJ 6:e4296. doi: 10.7717/peerj.4296
Bonner, C., Byrne, G., and Jensen, R. (2014). Chlamydia exploit the mammalian tryptophan-depletion defense strategy as a counter-defensive cue to trigger a survival state of persistence. Front. Cell. Infect. Microbiol. 4:17. doi: 10.3389/fcimb.2014.00017
Borel, N., Polkinghorne, A., and Pospischil, A. (2018). A review on chlamydial diseases in animals: still a challenge for pathologists? Vet. Pathol. 55, 374–390. doi: 10.1177/0300985817751218
Browning, G. F. (2004). Is Chlamydophila felis a significant zoonotic pathogen? Aust. Vet. J. 82, 695–696.
Brunham, R. C., Kuo, C. C., Cles, L., and Holmes, K. K. (1983). Correlation of host immune response with quantitative recovery of Chlamydia trachomatis from the human endocervix. Infect. Immun. 39, 1491–1494.
Brunham, R. C., and Rey-Ladino, J. (2005). Immunology of Chlamydia infection: implications for a Chlamydia trachomatis vaccine. Nat. Rev. Immunol. 5, 149–161. doi: 10.1038/nri1551
Brunham, R. C., and Zhang, D. J. (1999). Transgene as vaccine for chlamydia. Am. Heart J. 138, S519–S522. doi: 10.1016/S0002-8703(99)70291-7
Burnard, D., Huston, W. M., Webb, J. K., Jelocnik, M., Reiss, A., Gillett, A., et al. (2017). Molecular evidence of Chlamydia pecorum and arthropod-associated Chlamydiae in an expanded range of marsupials. Sci. Rep. 7:12844. doi: 10.1038/s41598-017-13164-y
Cai, Y., Fukushi, H., Koyasu, S., Kuroda, E., Yamaguchi, T., and Hirai, K. (2002). An etiological investigation of domestic cats with conjunctivitis and upper respiratory tract disease in Japan. J. Vet. Med. Sci. 64, 215–219. doi: 10.1292/jvms.64.215
Caldwell, H. D., and Perry, L. J. (1982). Neutralization of Chlamydia trachomatis infectivity with antibodies to the major outer membrane protein. Infect. Immun. 38, 745–754.
Campos, M., Pal, S., O’Brien, T. P., Taylor, H. R., Prendergast, R. A., and Whittum-Hudson, J. A. (1995). A chlamydial major outer membrane protein extract as a trachoma vaccine candidate. Invest. Ophthalmol. Vis. Sci. 36, 1477–1491.
Carey, A. J., Timms, P., Rawlinson, G., Brumm, J., Nilsson, K., Harris, J. M., et al. (2010). A Multi-subunit chlamydial vaccine induces antibody and cell-mediated immunity in immunized koalas (Phascolarctos cinereus): comparison of three different adjuvants. Am. J. Reprod. Immunol. 63, 161–172. doi: 10.1111/j.1600-0897.2009.00776.x
Carmichael, J. R., Pal, S., Tifrea, D., and De la Maza, L. M. (2011). Induction of protection against vaginal shedding and infertility by a recombinant Chlamydia vaccine. Vaccine 29, 5276–5283. doi: 10.1016/j.vaccine.2011.05.013
Casadevall, A. (2003). Antibody-mediated immunity against intracellular pathogens: two-dimensional thinking comes full circle. Infect. Immun. 71, 4225–4228. doi: 10.1128/IAI.71.8.4225-4228.2003
Casadevall, A. (2004). The methodology for determining the efficacy of antibody-mediated immunity. J. Immunol. Methods 291, 1–10. doi: 10.1016/j.jim.2004.04.027
Casadevall, A., and Pirofski, L. A. (2006). A reappraisal of humoral immunity based on mechanisms of antibody-mediated protection against intracellular pathogens. Adv. Immunol. 91, 1–44. doi: 10.1016/S0065-2776(06)91001-3
Chang, H. L., Chin, H. Y., and Wang, K. C. (1964). Experimental studies on trachoma vaccine in monkeys. Chin. Med. J. 83, 755–762.
Cheng, C., Jain, P., Bettahi, I., Pal, S., Tifrea, D., and de la Maza, L. M. (2011a). A TLR2 agonist is a more effective adjuvant for a chlamydia major outer membrane protein vaccine than ligands to other TLR and NOD receptors. Vaccine 29, 6641–6649. doi: 10.1016/j.vaccine.2011.06.105
Cheng, C., Pal, S., Bettahi, I., Oxford, K. L., Barry, P. A., and De la Maza, L. M. (2011b). Immunogenicity of a vaccine formulated with the Chlamydia trachomatis serovar F, native major outer membrane protein in a nonhuman primate model. Vaccine 29, 3456–3464. doi: 10.1016/j.vaccine.2011.02.057
Cheng, C., Pal, S., Tifrea, D., Jia, Z., and De la Maza, L. M. (2014). A vaccine formulated with a combination of TLR-2 and TLR-9 adjuvants and the recombinant major outer membrane protein elicits a robust immune response and significant protection against a Chlamydia muridarum challenge. Microbes Infect. 16, 244–252. doi: 10.1016/j.micinf.2013.11.009
Chu, J., Zhang, Q., Zuo, Z., El-Ashram, S., Guo, Y., Zhao, P., et al. (2017). Co-infection of Chlamydia psittaci with H9N2, ORT and Aspergillus fumigatus contributes to severe pneumonia and high mortality in SPF chickens. Sci. Rep. 7:13997. doi: 10.1038/s41598-017-14519-1
Daba, M. H., Saleh, O., Al-Arifi, M. N., Gubara, O. A., Al-Hadiya, B. M. H., and Dana, M. H. (2002). The role of infection in atherosclerosis. Saudi Pharm. J. 10, 9–18.
De La Maza, L. M., Zhong, G., and Brunham, R. C. (2017). Update on Chlamydia trachomatis vaccinology. Clin. Vaccine Immunol. 24:e00543-16. doi: 10.1128/CVI.00543-16
De Puysseleyr, L., De Puysseleyr, K., Braeckman, L., Morre, S. A., Cox, E., and Vanrompay, D. (2017). Assessment of Chlamydia suis infection in pig farmers. Transbound. Emerg. Dis. 64, 826–833. doi: 10.1111/tbed.12446
DeGraves, F. J., Kim, T., Jee, J., Schlapp, T., Hehnen, H. R., and Kaltenboeck, B. (2004). Reinfection with Chlamydophila abortus by uterine and indirect cohort routes reduces fertility in cattle preexposed to Chlamydophila. Infect. Immun. 72, 2538–2545. doi: 10.1128/IAI.72.5.2538-2545.2004
Deguchi, T., Hatazaki, K., Ito, S., Kondo, H., Horie, K., Nakane, K., et al. (2018). Macrolide and fluoroquinolone resistance is uncommon in clinical strains of Chlamydia trachomatis. J. Infect. Chemother. 24, 610–614. doi: 10.1016/j.jiac.2018.03.007
Deniset, J. F., Cheung, P. K. M., Dibrov, E., Lee, K., Steigerwald, S., and Pierce, G. N. (2010). Chlamydophila pneumoniae infection leads to smooth muscle cell proliferation and thickening in the coronary artery without contributions from a host immune response. Am. J. Pathol. 176, 1028–1037. doi: 10.2353/ajpath.2010.090645
Desclozeaux, M., Jelocnik, M., Whitting, K., Saifzadeh, S., Bommana, S., Potter, A., et al. (2017a). Safety and immunogenicity of a prototype anti-Chlamydia pecorum recombinant protein vaccine in lambs and pregnant ewes. Vaccine 35, 3461–3465. doi: 10.1016/j.vaccine.2017.03.091
Desclozeaux, M., Robbins, A., Jelocnik, M., Khan, S. A., Hanger, J., Gerdts, V., et al. (2017b). Immunization of a wild koala population with a recombinant Chlamydia pecorum Major Outer Membrane Protein (MOMP) or Polymorphic Membrane Protein (PMP) based vaccine: new insights into immune response, protection and clearance. PLoS One 12:e0178786. doi: 10.1371/journal.pone.0178786
Everett, K. D., Bush, R. M., and Andersen, A. A. (1999). Emended description of the order Chlamydiales, proposal of Parachlamydiaceae fam. nov. and Simkaniaceae fam. nov., each containing one monotypic genus, revised taxonomy of the family Chlamydiaceae, including a new genus and five new species, and standards for the identification of organisms. Int. J. Syst. Bacteriol. 49(Pt 2), 415–440. doi: 10.1099/00207713-49-2-415
Eyerich, S., Eyerich, K., Cavani, A., and Schmidt-Weber, C. (2010). IL-17 and IL-22: siblings, not twins. Trends Immunol. 31, 354–361. doi: 10.1016/j.it.2010.06.004
Faal, N., Bailey, R. L., Jeffries, D., Joof, H., Sarr, I., Laye, M., et al. (2006). Conjunctival FOXP3 expression in trachoma: do regulatory T cells have a role in human ocular Chlamydia trachomatis infection? PLoS Med. 3:e266. doi: 10.1371/journal.pmed.0030266
Farley, T. A., Cohen, D. A., and Elkins, W. (2003). Asymptomatic sexually transmitted diseases: the case for screening. Prev. Med. 36, 502–509. doi: 10.1016/S0091-7435(02)00058-0
Farris, C. M., Morrison, S. G., and Morrison, R. P. (2010). CD4+ T cells and antibody are required for optimal major outer membrane protein vaccine-induced immunity to Chlamydia muridarum genital infection. Infect. Immun. 78, 4374–4383. doi: 10.1128/IAI.00622-10
Gajdosová, E., Kovácová, E., Kazár, J., Kolcunová, A., and Sabó, A. (1994). The immunogenicity of a vaccine against enzootic abortion in sheep. Vet. Med. 39, 589–596.
Garland, S. M., Malatt, A., Tabrizi, S., Grando, D., Lees, M. I., Andrew, J. H., et al. (1995). Chlamydia trachomatis conjunctivitis. Prevalence and association with genital tract infection. Med. J. Aust. 162, 363–366.
Greub, G. (2010). International committee on systematics of prokaryotes. Subcommittee on the taxonomy of the Chlamydiae: minutes of the inaugural closed meeting, 21 March 2009, Little Rock, AR, USA. Int. J. Syst. Evol. Microbiol. 60(Pt 11), 2691–2693. doi: 10.1099/ijs.0.028225-0
Guo, W., Li, J., Kaltenboeck, B., Gong, J., Fan, W., and Wang, C. (2016). Chlamydia gallinacea, not C. psittaci, is the endemic chlamydial species in chicken (Gallus gallus). Sci. Rep. 6:19638. doi: 10.1038/srep19638
Hickey, D. K., Aldwell, F. E., and Beagley, K. W. (2009). Transcutaneous immunization with a novel lipid-based adjuvant protects against Chlamydia genital and respiratory infections. Vaccine 27, 6217–6225. doi: 10.1016/j.vaccine.2009.08.001
Hickey, D. K., Aldwell, F. E., and Beagley, K. W. (2010). Oral immunization with a novel lipid-based adjuvant protects against genital Chlamydia infection. Vaccine 28, 1668–1672. doi: 10.1016/j.vaccine.2009.12.010
Hickey, D. K., Jones, R. C., Bao, S., Blake, A. E., Skelding, K. A., Berry, L. J., et al. (2004). Intranasal immunization with C. muridarum major outer membrane protein (MOMP) and cholera toxin elicits local production of neutralising IgA in the prostate. Vaccine 22, 4306–4315. doi: 10.1016/j.vaccine.2004.04.021
Hogerwerf, L., De Gier, B., Baan, B., and Van Der Hoek, W. (2017). Chlamydia psittaci (psittacosis) as a cause of community-acquired pneumonia: a systematic review and meta-analysis. Epidemiol. Infect. 145, 3096–3105. doi: 10.1017/S0950268817002060
Holland, M. J., Bailey, R. L., Conway, D. J., Culley, F., Miranpuri, G., Byrne, G. I., et al. (1996). T helper type-1 (Th1)/Th2 profiles of peripheral blood mononuclear cells (PBMC); responses to antigens of Chlamydia trachomatis in subjects with severe trachomatous scarring. Clin. Exp. Immunol. 105, 429–435. doi: 10.1046/j.1365-2249.1996.d01-792.x
Igietseme, J. U., and Murdin, A. (2000). Induction of protective immunity against Chlamydia trachomatis genital infection by a vaccine based on major outer membrane protein-lipophilic immune response-stimulating complexes. Infect. Immun. 68, 6798–6806. doi: 10.1128/IAI.68.12.6798-6806.2000
Iramain, R., De Jesús, R., Spitters, C., Jara, A., Jimenez, J., Bogado, N., et al. (2016). Chlamydia pneumoniae, and mycoplasma pneumoniae: are they related to severe asthma in childhood? J. Asthma 53, 618–621. doi: 10.3109/02770903.2015.1116085
Janssen, K. J. H., Hoebe, C. J. P. A., Dukers-Muijrers, N. H. T. M., Eppings, L., Lucchesi, M., and Wolffs, P. F. G. (2016). Viability-PCR shows that NAAT detects a high proportion of DNA from non-viable Chlamydia trachomatis. PLoS One 11:e0165920. doi: 10.1371/journal.pone.0165920
Jelocnik, M., Forshaw, D., Cotter, J., Roberts, D., Timms, P., and Polkinghorne, A. (2014). Molecular and pathological insights into Chlamydia pecorum-associated sporadic bovine encephalomyelitis (SBE) in Western Australia. BMC Vet. Res. 10:121. doi: 10.1186/1746-6148-10-121
Jelocnik, M., Jenkins, C., O’Rourke, B., Barnwell, J., and Polkinghorne, A. (2018). Molecular evidence to suggest pigeon-type Chlamydia psittaci in association with an equine foal loss. Transbound. Emerg. Dis. 65, 911–915. doi: 10.1111/tbed.12817
Jiang, P., Du, W., Xiong, Y., Lv, Y., Feng, J., Zhu, S., et al. (2015). Hepatitis B virus core antigen as a carrier for Chlamydia trachomatis MOMP multi-epitope peptide enhances protection against genital chlamydial infection. Oncotarget 6, 43281–43292. doi: 10.18632/oncotarget.6533
Johnson, F. W. A., and Hobson, D. (1986). Intracerebral infection of mice with ovine strains of Chlamydia psittaci: an animal screening test for the assay of vaccines. J. Comp. Pathol. 96, 497–505. doi: 10.1016/0021-9975(86)90070-8
Jones, G. E., Jones, K. A., Machell, J., Brebner, J., Anderson, I. E., and How, S. (1995). Efficacy trials with tissue-culture grown, inactivated vaccines against chlamydial abortion in sheep. Vaccine 13, 715–723. doi: 10.1016/0264-410X(94)00068-X
Jones, R. B., Van Der Pol, B., Martin, D. H., and Shepard, M. K. (1990). Partial characterization of Chlamydia trachomatis isolates resistant to multiple antibiotics. J. Infect. Dis. 162, 1309–1315. doi: 10.1093/infdis/162.6.1309
Kari, L., Whitmire, W. M., Crane, D. D., Reveneau, N., Carlson, J. H., Goheen, M. M., et al. (2009). Chlamydia trachomatis native major outer membrane protein induces partial protection in nonhuman primates: implication for a trachoma transmission- blocking vaccine. J. Immunol. 182, 8063–8070. doi: 10.4049/jimmunol.0804375
Kari, L., Whitmire, W. M., Olivares-Zavaleta, N., Goheen, M. M., Taylor, L. D., Carlson, J. H., et al. (2011). A live-attenuated chlamydial vaccine protects against trachoma in nonhuman primates. J. Exp. Med. 208, 2217–2223. doi: 10.1084/jem.20111266
Kauffold, J., Melzer, F., Berndt, A., Hoffmann, G., Hotzel, H., and Sachse, K. (2006). Chlamydiae in oviducts and uteri of repeat breeder pigs. Theriogenology 66, 1816–1823. doi: 10.1016/j.theriogenology.2006.04.042
Khan, S. A., Desclozeaux, M., Waugh, C., Hanger, J., Loader, J., Gerdts, V., et al. (2016a). Antibody and cytokine responses of koalas (Phascolarctos cinereus) vaccinated with Recombinant Chlamydial Major Outer Membrane Protein (MOMP) with two different adjuvants. PLoS One 11:e0156094. doi: 10.1371/journal.pone.0156094
Khan, S. A., Polkinghorne, A., Waugh, C., Hanger, J., Loader, J., Beagley, K., et al. (2016b). Humoral immune responses in koalas (Phascolarctos cinereus) either naturally infected with Chlamydia pecorum or following administration of a recombinant chlamydial major outer membrane protein vaccine. Vaccine 34, 775–782. doi: 10.1016/j.vaccine.2015.12.050
Khan, S. A., Waugh, C., Rawlinson, G., Brumm, J., Nilsson, K., Gerdts, V., et al. (2014). Vaccination of koalas (Phascolarctos cinereus) with a recombinant chlamydial major outer membrane protein adjuvanted with poly I: C, a host defense peptide and polyphosphazine, elicits strong and long lasting cellular and humoral immune responses. Vaccine 32, 5781–5786. doi: 10.1016/j.vaccine.2014.08.037
Knight, S. C., Iqball, S., Woods, C., Stagg, A., Ward, M. E., and Tuffrey, M. (1995). A peptide of Chlamydia trachomatis shown to be a primary T-cell epitope in vitro induces cell-mediated immunity in vivo. Immunology 85, 8–15.
Kollipara, A., George, C., Hanger, J., Loader, J., Polkinghorne, A., Beagley, K., et al. (2012). Vaccination of healthy and diseased koalas (Phascolarctos cinereus) with a Chlamydia pecorum multi-subunit vaccine: evaluation of immunity and pathology. Vaccine 30, 1875–1885. doi: 10.1016/j.vaccine.2011.12.125
Kollipara, A., Polkinghorne, A., Beagley, K. W., and Timms, P. (2013a). Vaccination of koalas with a recombinant Chlamydia pecorum major outer membrane protein induces antibodies of different specificity compared to those following a natural live infection. PLoS One 8:e74808. doi: 10.1371/journal.pone.0074808
Kollipara, A., Wan, C., Rawlinson, G., Brumm, J., Nilsson, K., Polkinghorne, A., et al. (2013b). Antigenic specificity of a monovalent versus polyvalent MOMP based Chlamydia pecorum vaccine in koalas (Phascolarctos cinereus). Vaccine 31, 1217–1223. doi: 10.1016/j.vaccine.2012.12.057
Korenromp, E. L., Sudaryo, M. K., de Vlas, S. J., Gray, R. H., Sewankambo, N. K., Serwadda, D., et al. (2002). What proportion of episodes of gonorrhoea and chlamydia becomes symptomatic? Int. J. STD AIDS 13, 91–101. doi: 10.1258/0956462021924712
Kurz, H., Göpfrich, H., Wabnegger, L., and Apfalter, P. (2009). Role of Chlamydophila pneumoniae in children hospitalized for community-acquired pneumonia in Vienna, Austria. Pediatr. Pulmonol. 44, 873–876. doi: 10.1002/ppul.21059
Lefevre, J. C., Lepargneur, J. P., Guion, D., and Bei, S. (1997). Tetracycline-resistant Chlamydia trachomatis in Toulouse, France. Pathol. Biol. 45, 376–378.
Li, J., Guo, W., Kaltenboeck, B., Sachse, K., Yang, Y., Lu, G., et al. (2016). Chlamydia pecorum is the endemic intestinal species in cattle while C. gallinacea, C. psittaci and C. pneumoniae associate with sporadic systemic infection. Vet. Microbiol. 193, 93–99. doi: 10.1016/j.vetmic.2016.08.008
Li, L., Luther, M., MacKlin, K., Pugh, D., Li, J., Zhang, J., et al. (2017). Chlamydia gallinacea: a widespread emerging Chlamydia agent with zoonotic potential in backyard poultry. Epidemiol. Infect. 145, 2701–2703. doi: 10.1017/S0950268817001650
Li, W., Guentzel, M. N., Seshu, J., Zhong, G., Murthy, A. K., and Arulanandam, B. P. (2007). Induction of cross-serovar protection against genital chlamydial infection by a targeted multisubunit vaccination approach. Clin. Vaccine Immunol. 14, 1537–1544. doi: 10.1128/CVI.00274-07
Li, Y., Ahluwalia, S. K., Borovkov, A., Loskutov, A., Wang, C., Gao, D., et al. (2010). Novel Chlamydia pneumoniae vaccine candidates confirmed by Th1-enhanced genetic immunization. Vaccine 28, 1598–1605. doi: 10.1016/j.vaccine.2009.11.046
Liang, M., Wen, Y., Ran, O., Chen, L., Wang, C., Li, L., et al. (2016). Protective immunity induced by recombinant protein IT_p8 of Chlamydia psittaci. Appl. Microbiol. Biotechnol. 100, 6385–6393. doi: 10.1007/s00253-016-7494-8
Liu, S., Sun, W., Chu, J., Huang, X., Wu, Z., Yan, M., et al. (2015). Construction of recombinant HVT expressing PmpD, and immunological evaluation against Chlamydia psittaci and Marek’s disease virus. PLoS One 10:e0124992. doi: 10.1371/journal.pone.0124992
Ljubin-Sternak, S., and Mestrovic, T. (2014). Chlamydia trachomatis and genital mycoplasmas: pathogens with an impact on human reproductive health. J. Pathog. 2014:183167. doi: 10.1155/2014/183167
Loots, K., Vleugels, B., Ons, E., Vanrompay, D., and Goddeeris, B. M. (2006). Evaluation of the persistence and gene expression of an anti-Chlamydophila psittaci DNA vaccine in turkey muscle. BMC Vet. Res. 2:18. doi: 10.1186/1746-6148-2-18
Lu, H., Xing, Z., and Brunham, R. C. (2002). GM-CSF transgene-based adjuvant allows the establishment of protective mucosal immunity following vaccination with inactivated Chlamydia trachomatis. J. Immunol. 169, 6324–6331. doi: 10.4049/jimmunol.169.11.6324
MacDonald, A. B., McComb, D., and Howard, L. (1984). Immune response of owl monkeys to topical vaccination with irradiated Chlamydia trachomatis. J. Infect. Dis. 149, 439–442. doi: 10.1093/infdis/149.3.439
Malaty, R., Dawson, C. R., Wong, I., Lyon, C., and Schachter, J. (1981). Serum and tear antibodies to Chlamydia after reinfection with guinea pig inclusion conjunctivitis agent. Invest. Ophthalmol. Vis. Sci. 21, 833–841.
Masala, G., Porcu, R., Daga, C., Denti, S., Canu, G., Patta, C., et al. (2007). Detection of pathogens in ovine and caprine abortion samples from Sardinia, Italy, by PCR. J. Vet. Diagn. Invest. 19, 96–98. doi: 10.1177/104063870701900116
Matsumoto, M., Kikkawa, S., Kohase, M., Miyake, K., and Seya, T. (2002). Establishment of a monoclonal antibody against human Toll-like receptor 3 that blocks double-stranded RNA-mediated signaling. Biochem. Biophys. Res. Commun. 293, 1364–1369. doi: 10.1016/s0006-291x(02)00380-7
Meijer, A., Brandenburg, A., de Vries, J., Beentjes, J., Roholl, P., and Dercksen, D. (2004). Chlamydophila abortus infection in a pregnant woman associated with indirect contact with infected goats. Eur. J. Clin. Microbiol. Infect. Dis. 23, 487–490. doi: 10.1007/s10096-004-1139-z
Menon, S., Timms, P., Allan, J. A., Alexander, K., Rombauts, L., Horner, P., et al. (2015). Human and pathogen factors associated with Chlamydia trachomatis-related infertility in women. Clin. Microbiol. Rev. 28, 969–985. doi: 10.1128/CMR.00035-15
Misiurina, O. I., Shipitsina, E. V., Finashutina, I. P., Lazarev, V. N., Akopian, T. A., Savicheva, A. M., et al. (2004). Analysis of point mutations in the ygeD, gyrA and parC genes in fluoroquinolones resistant clinical isolates of Chlamydia trachomatis. Mol. Gen. Mikrobiol. Virusol. 3, 3–7.
Mitura, A., Niemczuk, K., Zarȩba, K., Zając, M., Laroucau, K., and Szymańska-Czerwińska, M. (2017). Free-living and captive turtles and tortoises as carriers of new Chlamydia spp. PLoS One 12:e0185407. doi: 10.1371/journal.pone.0185407
Mitura, A., Szymanska-Czerwinska, M., Niemczuk, K., and Anara, J. (2014). Chlamydia in birds-occurrence, new species and zoonotic potential-A review. Bull. Vet. Inst. Pulawy 58, 503–506. doi: 10.2478/bvip-2014-0076
Mitzel, J. R., Wright, G. G., and Swack, N. S. (1970). Cross immunity among strains of Chlamydia psittaci. Proc. Soc. Exp. Biol. Med. 135, 944–946. doi: 10.3181/00379727-135-35176
Morgan, H. R., and Wiseman, R. W. (1946). Growth of psittacosis virus in roller tube tissue culture; use in a vaccine. J. Infect. Dis. 79, 131–133. doi: 10.1093/infdis/79.2.131
Morrison, R. P. (1991). Chlamydial hsp60 and the immunopathogenesis of chlamydial disease. Semin. Immunol. 3, 25–33.
Natividad, A., Wilson, J., Koch, O., Holland, M. J., Rockett, K., Faal, N., et al. (2005). Risk of trachomatous scarring and trichiasis in Gambians varies with SNP haplotypes at the interferon-gamma and interleukin-10 loci. Genes Immun. 6, 332–340. doi: 10.1038/sj.gene.6364182
Newman, L., Rowley, J., Vander Hoorn, S., Wijesooriya, N. S., Unemo, M., Low, N., et al. (2015). Global estimates of the prevalence and incidence of four curable sexually transmitted infections in 2012 based on systematic review and global reporting. PLoS One 10:e0143304. doi: 10.1371/journal.pone.0143304
Nichols, R. L., Murray, E. S., and Nisson, P. E. (1978). Use of enteric vaccines in protection against chlamydial infections of the genital tract and the eye of guinea pigs. J. Infect. Dis. 138, 742–746. doi: 10.1093/infdis/138.6.742
Oehme, A., Musholt, P. B., and Dreesbach, K. (1991). Chlamydiae as pathogens — An overview of diagnostic techniques, clinical features, and therapy of human infections. Klin. Wochenschr. 69, 463–473. doi: 10.1007/bf01649417
Olivares-Zavaleta, N., Whitmire, W. M., Kari, L., Sturdevant, G. L., and Caldwell, H. D. (2014). CD8+ T cells define an unexpected role in live-attenuated vaccine protective immunity against Chlamydia trachomatis infection in macaques. J. Immunol. 192, 4648–4654. doi: 10.4049/jimmunol.1400120
O’Meara, C. P., Armitage, C. W., Harvie, M. C. G., Timms, P., Lycke, N. Y., and Beagley, K. W. (2013). Immunization with a MOMP-based vaccine protects mice against a pulmonary chlamydia challenge and identifies a disconnection between infection and pathology. PLoS One 8:e61962. doi: 10.1371/journal.pone.0061962
Ortiz, L., Demick, K. P., Petersen, J. W., Polka, M., Rudersdorf, R. A., Van der Pol, B., et al. (1996). Chlamydia trachomatis major outer membrane protein (MOMP) epitopes that activate HLA class II-restricted T cells from infected humans. J. Immunol. 157, 4554–4567.
Pal, S., Barnhart, K. M., Wei, Q., Abai, A. M., Peterson, E. M., and De La Maza, L. M. (1999). Vaccination of mice with DNA plasmids coding for the Chlamydia trachomatis major outer membrane protein elicits an immune response but fails to protect against a genital challenge. Vaccine 17, 459–465. doi: 10.1016/S0264-410X(98)00219-9
Pal, S., Favaroni, A., Tifrea, D. F., Hanisch, P. T., Luczak, S. E. T., Hegemann, J. H., et al. (2017a). Comparison of the nine polymorphic membrane proteins of Chlamydia trachomatis for their ability to induce protective immune responses in mice against a C. muridarum challenge. Vaccine 35, 2543–2549. doi: 10.1016/j.vaccine.2017.03.070
Pal, S., Tifrea, D. F., Follmann, F., Andersen, P., and de la Maza, L. M. (2017b). The cationic liposomal adjuvants CAF01 and CAF09 formulated with the major outer membrane protein elicit robust protection in mice against a Chlamydia muridarum respiratory challenge. Vaccine 35, 1705–1711. doi: 10.1016/j.vaccine.2017.02.020
Pal, S., Fielder, T. J., Peterson, E. M., and de la Maza, L. M. (1994). Protection against infertility in a BALB/c mouse salpingitis model by intranasal immunization with the mouse pneumonitis biovar of Chlamydia trachomatis. Infect. Immun. 62, 3354–3362.
Pal, S., Peterson, E. M., and De La Maza, L. M. (2005). Vaccination with the Chlamydia trachomatis major outer membrane protein can elicit an immune response as protective as that resulting from inoculation with live bacteria. Infect. Immun. 73, 8153–8160. doi: 10.1128/IAI.73.12.8153-8160.2005
Pal, S., Theodor, I., Peterson, E. M., and De la Maza, L. M. (1997). Immunization with an acellular vaccine consisting of the outer membrane complex of Chlamydia trachomatis induces protection against a genital challenge. Infect. Immun. 65, 3361–3369.
Pal, S., Theodor, I., Peterson, E. M., and De la Maza, L. M. (2001). Immunization with the Chlamydia trachomatis mouse pneumonitis major outer membrane protein can elicit a protective immune response against a genital challenge. Infect. Immun. 69, 6240–6247. doi: 10.1128/IAI.69.10.6240-6247.2001
Peterson, E. M., You, J. Z., Motin, V., and De La Maza, L. M. (1999). Intranasal immunization with Chlamydia trachomatis, serovar E, protects from a subsequent vaginal challenge with the homologous serovar. Vaccine 17, 2901–2907. doi: 10.1016/S0264-410X(99)00131-0
Phillips, S., Vodstrcil, L. A., Huston, W. M., Lawerence, A., Timms, P., Chen, M. Y., et al. (2018). Detection of Chlamydia trachomatis mRNA using digital PCR as a more accurate marker of viable organism. Eur. J. Clin. Microbiol. Infect. Dis. 37, 2117–2122. doi: 10.1007/s10096-018-3347-y
Pisanu, B., Laroucau, K., Aaziz, R., Vorimore, F., Le Gros, A., Chapuis, J. L., et al. (2018). Chlamydia avium detection from a ring-necked parakeet (Psittacula krameri) in France. J. Exot. Pet Med. 27, 68–74. doi: 10.1053/j.jepm.2018.02.035
Qiu, C., Zhou, J., Cao, X., Lin, G., Zheng, F., and Gong, X. (2010). Immunization trials with an avian chlamydial MOMP gene recombinant adenovirus. Bioeng. Bugs 1, 267–273. doi: 10.4161/bbug.1.4.12115
Qu, Y., Frazer, L. C., O’Connell, C. M., Tarantal, A. F., Andrews, C. W. Jr., O’Connor, S. L., et al. (2015). Comparable genital tract infection, pathology, and immunity in rhesus macaques inoculated with wild-type or plasmid-deficient Chlamydia trachomatis serovar D. Infect. Immun. 83, 4056–4067. doi: 10.1128/iai.00841-15
Ralli-Jain, P., Tifrea, D., Cheng, C., Pal, S., and de la Maza, L. M. (2010). Enhancement of the protective efficacy of a Chlamydia trachomatis recombinant vaccine by combining systemic and mucosal routes for immunization. Vaccine 28, 7659–7666. doi: 10.1016/j.vaccine.2010.09.040
Ramakers, B. P., Heijne, M., Lie, N., Le, T. N., van Vliet, M., Claessen, V. P. J., et al. (2017). Zoonotic Chlamydia caviae presenting as community-acquired pneumonia. N. Engl. J. Med. 377, 992–994. doi: 10.1056/NEJMc1702983
Rampazzo, A., Appino, S., Pregel, P., Tarducci, A., Zini, E., and Biolatti, B. (2003). Prevalence of Chlamydophila felis and feline Herpesvirus 1 in cats with conjunctivitis in northern Italy. J. Vet. Intern. Med. 17, 799–807. doi: 10.1111/j.1939-1676.2003.tb02517.x
Ramsey, K. H., Soderberg, L. S., and Rank, R. G. (1988). Resolution of chlamydial genital infection in B-cell-deficient mice and immunity to reinfection. Infect. Immun. 56, 1320–1325.
Ran, O., Liang, M., Yu, J., Yu, M., Song, Y., and Yimou, W. (2017). Recombinant protein CPSIT_0846 induces protective immunity against Chlamydia psittaci infection in BALB/c mice. Pathog. Dis. 75:ftx018. doi: 10.1093/femspd/ftx018
Rank, R. G., Batteiger, B. E., and Soderberg, L. S. F. (1990). Immunization against chlamydial genital infection in guinea pigs with UV-inactivated and viable chlamydiae administered by different routes. Infect. Immun. 58, 2599–2605.
Reinhold, P., Jaeger, J., Liebler-Tenorio, E., Berndt, A., Bachmann, R., Schubert, E., et al. (2008). Impact of latent infections with Chlamydophila species in young cattle. Vet. J. 175, 202–211. doi: 10.1016/j.tvjl.2007.01.004
Reinhold, P., Jaeger, J., Melzer, F., and Sachse, K. (2005). Evaluation of lung function in pigs either experimentally or naturally infected with Chlamydiaceae. Vet. Res. Commun. 29(Suppl. 1), 125–150. doi: 10.1007/s11259-005-0843-1
Reinhold, P., Sachse, K., and Kaltenboeck, B. (2011). Chlamydiaceae in cattle: commensals, trigger organisms, or pathogens? Vet. J. 189, 257–267. doi: 10.1016/j.tvjl.2010.09.003
Rekiki, A., Bouakane, A., Hammami, S., El Idrissi, A. H., Bernard, F., and Rodolakis, A. (2004a). Efficacy of live Chlamydophila abortus vaccine 1B in protecting mice placentas and foetuses against strains of Chlamydophila pecorum isolated from cases of abortion. Vet. Microbiol. 99, 295–299. doi: 10.1016/j.vetmic.2004.01.010
Rekiki, A., Bouakane, A., and Rodolakis, A. (2004b). Combined vaccination of live 1B Chlamydophila abortus and killed phase I Coxiella burnetii vaccine does not destroy protection against chlamydiosis in a mouse model. Can. J. Vet. Res. 68, 226–228.
Rodolakis, A., and Souriau, A. (1986). Response of goats to vaccination with temperature-sensitive mutants of Chlamydia psittaci obtained by nitrosoguanidine mutagenesis. Am. J. Vet. Res. 47, 2627–2631.
Rogers, D. G., and Andersen, A. A. (2000). Intestinal lesions caused by a strain of Chlamydia suis in weanling pigs infected at 21 days of age. J. Vet. Diagn. Invest. 12, 233–239. doi: 10.1177/104063870001200306
Sachse, K., Grossmann, E., Berndt, A., Schütt, C., Henning, K., Theegarten, D., et al. (2004). Respiratory chlamydial infection based on experimental aerosol challenge of pigs with Chlamydia suis. Comp. Immunol. Microbiol. Infect. Dis. 27, 7–23. doi: 10.1016/S0147-9571(02)00079-6
Sandbulte, J., TerWee, J., Wigington, K., and Sabara, M. (1996). Evaluation of Chlamydia psittaci subfraction and subunit preparations for their protective capacities. Vet. Microbiol. 48, 269–282. doi: 10.1016/0378-1135(95)00166-2
Shaw, J., Grund, V., Durling, L., Crane, D., and Caldwell, H. D. (2002). Dendritic cells pulsed with a recombinant chlamydial major outer membrane protein antigen elicit a CD4+ type 2 rather than type 1 immune response that is not protective. Infect. Immun. 70, 1097–1105. doi: 10.1128/IAI.70.3.1097-1105.2002
Shi, Y., Xia, X., Song, Y., Feng, G., Hu, L., Zhang, X., et al. (2002). Assessment of polymerase chain reaction and serology for detection of Chlamydia pneumoniae in patients with acute respiratory tract infection. Chin. Med. J. 115, 184–187.
Somani, J., Bhullar, V. B., Workowski, K. A., Farshy, C. E., and Black, C. M. (2000). Multiple drug-resistant Chlamydia trachomatis associated with clinical treatment failure. J. Infect. Dis. 181, 1421–1427. doi: 10.1086/315372
Staub, E., Marti, H., Biondi, R., Levi, A., Donati, M., Leonard, C. A., et al. (2018). Novel Chlamydia species isolated from snakes are temperature-sensitive and exhibit decreased susceptibility to azithromycin. Sci. Rep. 8:5660. doi: 10.1038/s41598-018-23897-z
Stephens, R. S., Kalman, S., Lammel, C., Fan, J., Marathe, R., Aravind, L., et al. (1998). Genome sequence of an obligate intracellular pathogen of humans: Chlamydia trachomatis. Science 282, 754–759. doi: 10.1126/science.282.5389.754
Stephens, R. S., Myers, G., Eppinger, M., and Bavoil, P. M. (2009). Divergence without difference: phylogenetics and taxonomy of Chlamydia resolved. FEMS Immunol. Med. Microbiol. 55, 115–119. doi: 10.1111/j.1574-695X.2008.00516.x
Stephens, R. S., Sanchez-Pescador, R., Wagar, E. A., Inouye, C., and Urdea, M. S. (1987). Diversity of Chlamydia trachomatis major outer membrane protein genes. J. Bacteriol. 169, 3879–3885. doi: 10.1128/jb.169.9.3879-3885.1987
Stevens, M. P., Twin, J., Fairley, C. K., Donovan, B., Tan, S. E., Yu, J., et al. (2010). Development and evaluation of an ompA quantitative real-time PCR assay for Chlamydia trachomatis serovar determination. J. Clin. Microbiol. 48, 2060–2065. doi: 10.1128/jcm.02308-09
Su, H., and Caldwell, H. D. (1993). Immunogenicity of a synthetic oligopeptide corresponding to antigenically common T-helper and B-cell neutralizing epitopes of the major outer membrane protein of Chlamydia trachomatis. Vaccine 11, 1159–1166. doi: 10.1016/0264-410X(93)90080-H
Sun, G., Pal, S., Weiland, J., Peterson, E. M., and de la Maza, L. M. (2009). Protection against an intranasal challenge by vaccines formulated with native and recombinant preparations of the Chlamydia trachomatis major outer membrane protein. Vaccine 27, 5020–5025. doi: 10.1016/j.vaccine.2009.05.008
Sykes, J. E. (2001). Feline upper respiratory tract pathogens: Chlamydophila felis. Compend. Contin. Educ. Pract. Vet. 23, 231–240.
Szeredi, L., and Bacsadi, Á. (2002). Detection of Chlamydophila (Chlamydia) abortus and Toxoplasma gondii in smears from cases of ovine and caprine abortion by the streptavidin-biotin method. J. Comp. Pathol. 127, 257–263. doi: 10.1053/jcpa.2002.0591
Tammiruusu, A., Penttilä, T., Lahesmaa, R., Sarvas, M., Puolakkainen, M., and Vuola, J. M. (2007). Intranasal administration of chlamydial outer protein N (CopN) induces protection against pulmonary Chlamydia pneumoniae infection in a mouse model. Vaccine 25, 283–290. doi: 10.1016/j.vaccine.2006.07.043
Tan, T. W., Herring, A. J., Anderson, I. E., and Jones, G. E. (1990). Protection of sheep against Chlamydia psittaci infection with a subcellular vaccine containing the major outer membrane protein. Infect. Immun. 58, 3101–3108.
Taylor, H. R., and Prendergast, R. A. (1987). Attempted oral immunization with chlamydial lipopolysaccharide subunit vaccine. Invest. Ophthalmol. Vis. Sci. 28, 1722–1726.
Taylor, H. R., Whittum-Hudson, J., Schachter, J., Caldwell, H. D., and Prendergast, R. A. (1988). Oral immunization with chlamydial major outer membrane protein (MOMP). Invest. Ophthalmol. Vis. Sci. 29, 1847–1853.
Taylor, H. R., Young, E., MacDonald, A. B., Schachter, J., and Prendergast, R. A. (1987). Oral immunization against chlamydial eye infection. Invest. Ophthalmol. Vis. Sci. 28, 249–258.
Taylor-Brown, A., Bachmann, N. L., Borel, N., and Polkinghorne, A. (2016). Culture-independent genomic characterisation of Candidatus Chlamydia sanzinia, a novel uncultivated bacterium infecting snakes. BMC Genomics 17:710. doi: 10.1186/s12864-016-3055-x
Taylor-Brown, A., and Polkinghorne, A. (2017). New and emerging chlamydial infections of creatures great and small. New Microbes New Infect. 18, 28–33. doi: 10.1016/j.nmni.2017.04.004
Taylor-Brown, A., Spang, L., Borel, N., and Polkinghorne, A. (2017). Culture-independent metagenomics supports discovery of uncultivable bacteria within the genus Chlamydia. Sci. Rep. 7:10661. doi: 10.1038/s41598-017-10757-5
Thorpe, C., Edwards, L., Snelgrove, R., Finco, O., Rae, A., Grandi, G., et al. (2007). Discovery of a vaccine antigen that protects mice from Chlamydia pneumoniae infection. Vaccine 25, 2252–2260. doi: 10.1016/j.vaccine.2006.12.003
Tifrea, D. F., Sun, G., Pal, S., Zardeneta, G., Cocco, M. J., Popot, J. L., et al. (2011). Amphipols stabilize the Chlamydia major outer membrane protein and enhance its protective ability as a vaccine. Vaccine 29, 4623–4631. doi: 10.1016/j.vaccine.2011.04.065
Torres-Mejía, A. M., Blanco-Peña, K., Rodríguez, C., Duarte, F., Jiménez-Soto, M., and Esperón, F. (2018). Zoonotic agents in feral pigeons (Columba livia) from Costa Rica: possible improvements to diminish contagion risks. Vector Borne Zoonotic Dis. 18, 49–54. doi: 10.1089/vbz.2017.2131
Trinchieri, G. (2003). Interleukin-12 and the regulation of innate resistance and adaptive immunity. Nat. Rev. Immunol. 3, 133–146. doi: 10.1038/nri1001
Tuffrey, M., Alexander, F., Conlan, W., Woods, C., and Ward, M. (1992). Heterotypic protection of mice against chlamydial salpingitis and colonization of the lower genital tract with a human serovar F isolate of Chlamydia trachomatis by prior immunization with recombinant serovar L1 major outer-membrane protein. J. Gen. Microbiol. 138, 1707–1715. doi: 10.1099/00221287-138-8-1707
Vanrompay, D., Cox, E., Vandenbussche, F., Volckaert, G., and Goddeeris, B. (1999a). Protection of turkeys against Chlamydia psittaci challenge by gene gun- based DNA immunizations. Vaccine 17, 2628–2635. doi: 10.1016/S0264-410X(99)00053-5
Vanrompay, D., Cox, E., Volckaert, G., and Goddeeris, B. (1999b). Turkeys are protected from infection with Chlamydia psittaci by plasmid DNA vaccination against the major outer membrane protein. Clin. Exp. Immunol. 118, 49–55. doi: 10.1046/j.1365-2249.1999.01024.x
Vasilevsky, S., Greub, G., Nardelli-Haefliger, D., and Baud, D. (2014). Genital Chlamydia trachomatis: understanding the roles of innate and adaptive immunity in vaccine research. Clin. Microbiol. Rev. 27, 346–370. doi: 10.1128/cmr.00105-13
Vasilevsky, S., Stojanov, M., Greub, G., and Baud, D. (2016). Chlamydial polymorphic membrane proteins: regulation, function and potential vaccine candidates. Virulence 7, 11–22. doi: 10.1080/21505594.2015.1111509
Wali, S., Gupta, R., Veselenak, R. L., Li, Y., Yu, J. J., Murthy, A. K., et al. (2014). Use of a guinea pig-specific transcriptome array for evaluation of protective immunity against genital chlamydial infection following intranasal vaccination in guinea pigs. PLoS One 9:e114261. doi: 10.1371/journal.pone.0114261
Walker, E., Jelocnik, M., Bommana, S., Timms, P., Carver, S., and Polkinghorne, A. (2018). Understanding the health and production impacts of endemic Chlamydia pecorum infections in lambs. Vet. Microbiol. 217, 90–96. doi: 10.1016/j.vetmic.2018.03.009
Walker, E., Moore, C., Shearer, P., Jelocnik, M., Bommana, S., Timms, P., et al. (2016). Clinical, diagnostic and pathologic features of presumptive cases of Chlamydia pecorum-associated arthritis in Australian sheep flocks. BMC Vet. Res. 12:193. doi: 10.1186/s12917-016-0832-3
Waugh, C., Austin, R., Polkinghorne, A., and Timms, P. (2016a). Treatment of Chlamydia-associated ocular disease via a recombinant protein based vaccine in the koala (Phascolarctos cinereus). Biologicals 44, 588–590. doi: 10.1016/j.biologicals.2016.09.006
Waugh, C., Khan, S. A., Carver, S., Hanger, J., Loader, J., Polkinghorne, A., et al. (2016b). A prototype recombinant-protein based Chlamydia pecorum vaccine results in reduced chlamydial burden and less clinical disease in free-ranging koalas (Phascolarctos cinereus). PLoS One 11:e0146934. doi: 10.1371/journal.pone.0146934
Waugh, C. A., Timms, P., Andrew, D., Rawlinson, G., Brumm, J., Nilsson, K., et al. (2015). Comparison of subcutaneous versus intranasal immunization of male koalas (Phascolarctos cinereus) for induction of mucosal and systemic immunity against Chlamydia pecorum. Vaccine 33, 855–860. doi: 10.1016/j.vaccine.2014.12.052
Westbay, T. D., Dascher, C. C., Hsia, R. C., Bavoil, P. M., and Zauderer, M. (1994). Dissociation of immune determinants of outer membrane proteins of Chlamydia psittaci strain guinea pig inclusion conjunctivitis. Infect. Immun. 62, 5614–5623.
Whittum-Hudson, J. A., Prendergast, R. A., and Taylor, H. R. (1986). Changes in conjunctival lymphocyte populations induced by oral immunization with Chlamydia trachomatis. Curr. Eye Res. 5, 973–979. doi: 10.3109/02713688608995179
Williams, D. M., Grubbs, B., and Schachter, J. (1987). Primary murine Chlamydia trachomatis pneumonia in B-cell-deficient mice. Infect. Immun. 55, 2387–2390.
Wills, J. M., Gruffydd-Jones, T. J., Richmond, S. J., Gaskell, R. M., and Bourne, F. J. (1987). Effect of vaccination on feline Chlamydia psittaci infection. Infect. Immun. 55, 2653–2657.
Wilsmore, A. J., Izzard, K. A., Dagnall, G. J. R., Wilsmore, B. C., and Woodland, R. M. (1990a). Protection of ewes vaccinated with A22 strain Chlamydia psittaci (ovis) against challenge in pregnancy with homologous and heterologous strains of the organism. Br. Vet. J. 146, 349–353. doi: 10.1016/S0007-1935(11)80028-X
Wilsmore, A. J., Wilsmore, B. C., Dagnall, G. J. R., Izzard, K. A., Woodland, R. M., Dawson, M., et al. (1990b). Clinical and immunological responses of ewes following vaccination with an experimental formalin-inactivated Chlamydia psittaci (ovis) vaccine and subsequent challenge with the live organism during pregnancy. Br. Vet. J. 146, 341–348. doi: 10.1016/S0007-1935(11)80027-8
Wu, B. C., Lee, A. H. Y., and Hancock, R. E. W. (2017). Mechanisms of the innate defense regulator peptide-1002 anti-inflammatory activity in a sterile inflammation mouse model. J. Immunol. 199, 3592–3603. doi: 10.4049/jimmunol.1700985
Yamamoto, M., Sato, S., Hemmi, H., Hoshino, K., Kaisho, T., Sanjo, H., et al. (2003). Role of adaptor TRIF in the MyD88-independent toll-like receptor signaling pathway. Science 301, 640–643. doi: 10.1126/science.1087262
Zhang, D. J., Yang, X., Shen, C., and Brunham, R. C. (1999). Characterization of immune responses following intramuscular DNA immunization with the MOMP gene of Chlamydia trachomatis mouse pneumonitis strain. Immunology 96, 314–321. doi: 10.1046/j.1365-2567.1999.00682.x
Zhao, X., Zhu, D., Ye, J., Li, X., Wang, Z., Zhang, L., et al. (2015). The potential protective role of the combination of IL-22 and TNF-alpha against genital tract Chlamydia trachomatis infection. Cytokine 73, 66–73. doi: 10.1016/j.cyto.2015.01.027
Keywords: Chlamydia, vaccine, MOMP (major outer membrane protein), mice, koala (Phascolarctos cinereus)
Citation: Phillips S, Quigley BL and Timms P (2019) Seventy Years of Chlamydia Vaccine Research – Limitations of the Past and Directions for the Future. Front. Microbiol. 10:70. doi: 10.3389/fmicb.2019.00070
Received: 30 October 2018; Accepted: 15 January 2019;
Published: 31 January 2019.
Edited by:
Robert V. Schoborg, East Tennessee State University, United StatesReviewed by:
Barbara Susanne Sixt, Umeå University, SwedenKenneth Fields, University of Kentucky, United States
Copyright © 2019 Phillips, Quigley and Timms. This is an open-access article distributed under the terms of the Creative Commons Attribution License (CC BY). The use, distribution or reproduction in other forums is permitted, provided the original author(s) and the copyright owner(s) are credited and that the original publication in this journal is cited, in accordance with accepted academic practice. No use, distribution or reproduction is permitted which does not comply with these terms.
*Correspondence: Samuel Phillips, U2FtdWVsLnBoaWxsaXBzQHJlc2VhcmNoLnVzYy5lZHUuYXU=