- 1Food Safety and Enteric Pathogens Research Unit, United States Department of Agriculture, National Animal Disease Center, Agricultural Research Service, Ames, IA, United States
- 2Crop Production and Pest Control Research Unit, United States Department of Agriculture, Agricultural Research Service, West Lafayette, IN, United States
- 3GlycoAnalytics Core, University of California, San Diego, San Diego, CA, United States
- 4Ruminant Diseases and Immunology Research Unit, Agricultural Research Service, United States Department of Agriculture, National Animal Disease Center, Ames, IA, United States
Campylobacter jejuni is a leading cause of bacterial foodborne illness in humans worldwide. However, C. jejuni naturally colonizes poultry without causing pathology where it resides deep within mucus of the cecal crypts. Mucus may modulate the pathogenicity of C. jejuni in a species-specific manner, where it is pathogenic in humans and asymptomatic in poultry. Little is known about how intestinal mucus from different host species affects C. jejuni gene expression. In this study we characterized the growth and transcriptome of C. jejuni NCTC11168 cultured in defined media supplemented with or without mucus isolated from avian (chicken or turkey) or mammalian (cow, pig, or sheep) sources. C. jejuni showed substantially improved growth over defined media, with mucus from all species, showing that intestinal mucus was an energy source for C. jejuni. Seventy-three genes were differentially expressed when C. jejuni was cultured in avian vs. mammalian mucus. Genes associated with iron acquisition and resistance to oxidative stress were significantly increased in avian mucus. Many of the differentially expressed genes were flanked by differentially expressed antisense RNA asRNA, suggesting a role in gene regulation. This study highlights the interactions between C. jejuni and host mucus and the impact on gene expression, growth and invasion of host cells, suggesting important responses to environmental cues that facilitate intestinal colonization.
IMPORTANCE
Campylobacter jejuni infection of humans is an important health problem world-wide and is the leading bacterial cause of foodborne illnesses in U.S. The main route for exposure for humans is consumption of poultry meat contaminated during processing. C. jejuni is frequently found in poultry, residing within the mucus of the intestinal tract without causing disease. It is not clear why C. jejuni causes disease in some animals and humans, while leaving birds without symptoms. To understand its activity in birds, we characterized C. jejuni responses to poultry mucus to identify genes turned on in the intestinal tract of birds. We identified genes important for colonization and persistence within the poultry gut, turned on when C. jejuni was exposed to poultry mucus. Our findings are an important step in understanding how C. jejuni responds and interacts in the poultry gut, and may identify ways to reduce C. jejuni in birds.
Introduction
Campylobacter jejuni is an important human pathogen, causing up to 400 million infections a year world-wide (Ruiz-Palacios, 2007). According to the CDC, Campylobacter ranked the highest among the causes of foodborne illnesses in U.S. in 2016 (Marder, 2017). Enteritis from Campylobacter results in moderate to severe fever, abdominal pain, and diarrhea. Infections can also lead to serious complications, such as Guillain Barré syndrome (Epps et al., 2013). C. jejuni is considered an avian commensal and poultry are the main reservoir for human exposure, however some infections originate from livestock (Epps et al., 2013). Understanding how C. jejuni interacts within the host-intestinal environment will provide insights into how C. jejuni is so apt at colonizing the avian gut, and thus, may identify targets for limiting commensal carriage.
C. jejuni colonizes the avian intestinal tract, with densities reaching 1010 colony forming units (CFUs) per gram of intestinal contents (Young et al., 2007). Despite colonizing at such a high level, naturally colonized C. jejuni doesn't cause disease in poultry nor does it adhere or invade the intestinal epithelium (Larson et al., 2008). C. jejuni preferentially colonizes the mucus layer that lines the intestinal tract, where it resides, deep within the cecal crypts of birds (Epps et al., 2013). Its motility and helical shape aids C. jejuni movement through the thick mucus layer to reach these mucosal microenvironments favorable for growth (Lertsethtakarn et al., 2011; Stahl et al., 2016). Once there, C. jejuni has to survive harsh environmental conditions that include oxidative and nitrosative stress, iron limitation, and host defense peptides (Hofreuter, 2014). Mucus colonization is required by C. jejuni to cause disease. Previous studies suggest that host intestinal mucus, rather than host epithelial cells, impact Campylobacter phenotypes (Alemka et al., 2010; Ganan et al., 2013). In addition, C. jejuni is chemotactic for mucin and its components, such as L-fucose (Hugdahl et al., 1988), and chicken mucus impacts DNA supercoiling and motility (Shortt et al., 2016). When exposed to purified intestinal mucin from many different host sources, C. jejuni displays tropism for and preferential binding to avian mucins (Naughton et al., 2013). Furthermore, purified chicken mucus has been shown to reduce C. jejuni adherence and invasion to intestinal epithelial cells in vitro, compared to human mucus (Byrne et al., 2007). Campylobacter's interactions with host mucins are highly specific, even showing differences among mucins isolated from different chicken intestinal compartments (Alemka et al., 2010; Naughton et al., 2013). These specific responses to avian mucus suggest adaptations to colonization and persistence within the avian gut.
In this study we characterized C. jejuni NCTC 11168 growth, invasion of intestinal epithelial cells, and transcriptome after exposure to purified mucus from avian and mammalian hosts. Avian and mammalian mucus sources differentially impacted gene expression that may reflect Campylobacter's ability to colonize each animal intestinal tract, including the upregulation of iron acquisition and oxidative stress genes in avian mucus. Non-coding antisense RNAs were differentially detected between avian and mammalian hosts, and may be in response to environmental cues. These asRNA may be evidence of pervasive transcription in C. jejuni because they appeared to be generated by transcriptional read-through events that cross gene boundaries. These data suggest that C. jejuni alters its gene expression in the presence of avian mucus in ways that may benefit intestinal colonization. Understanding the molecular mechanisms of how C. jejuni colonizes mucus may suggest approaches to prevent colonization.
Methods
Mucus Source and Isolation
Intestinal mucus was isolated from the small intestine (duodenum, jejunum, and ileum) of healthy chickens, turkeys, pigs, sheep, or cows euthanized at the National Animal Disease Center, Ames Iowa, in accordance with a protocol approved by the Institutional Animal Care and Use Committee. Animal sources of mucus included: 10 turkeys (2 years old), 20 chickens (14 weeks old), 5 sheep (8 months old), five pigs (ranged from 2 to 4 years old), and one cow (around 2 years old). The mucosal surfaces were gently rinsed with sterile PBS to remove ingesta, and subsequently scraped with sterile microscope slides for collection of mucus. Mucus from respective animal species was pooled and lyophilized. Each mucus prep was purified to enrich the mucus glycans, as previously described (Martens et al., 2008). Briefly, lyopholized crude mucosal scrapings were suspended at 25 g/L in 100 mM Tris pH 7.4. Proteinase K was added (100 mg/L) to each sample and incubated 16–20 h at 65°C. Samples were then centrifuged at 21,000 × g for 30 min at 4°C and the supernatant was saved. NaOH was added to 0.1 M and NaBH4 to 1M and the solution was incubated 16–20 h at 55°C. HCl (12M) was added until pH reached 7.0. Mucus preps were centrifuged at 21,000 × g for 30 min at 4°C and supernatants were saved and filtered stepwise to clarify (5 μm → 3 μm → 1 μm → 0.65 μm → 0.45 μm → 0.22 μm). Mucus preps were dialyzed at 1 kDa cutoff against ddH2O to remove salts. Tris was added to the glycans at 50 mM (pH 7.4) and passed over DEAE-Cellulose (CAS 9013-34-7, Santa Cruz Biotechnology) columns equilibrated in 50 mM Tris pH 7.4 and the flow-through was collected. The resulting fractions were dialyzed at 1 kDa cutoff against ddH2O and lyophilized for downstream use.
Mucus Composition
Glycan analysis of each mucus preparation was performed at the University of California, San Diego Glycotechnology Core facility. Analysis of the monosaccharide and sialic acid components of the glycans was done using high performance anion exchange chromatography with pulsed amperometric detection (HPAEC-PAD) and Reverse phase HPLC Fluorescence, respectively. Profiling of isolated glycans was done by MALDI-TOF and analyzed using the GlycoWorkbench Software Tool (Damerell et al., 2012).
Bradford protein assays were performed on mucus from each host to quantitate the total protein in each sample (Bradford, 1976). A total of 10 μg of each mucus preparation was run on SDS gel and stained with Coomassie blue and then SYPRO Ruby. Mass spectroscopy was performed, as described previously (Reinhardt et al., 2012), on each host mucus preparation (10% wt/vol) to characterize the proteins present in the mucus glycan preparation.
Iron quantification was performed for each host mucus sample using the EnzyX Iron (II) colorimetric assay (Assay Biotechnology Sunnyvale, CA). Samples were run at 1, 2, and 10% wt/vol (w/v), following the manufacturer's recommendations.
C. jejuni Growth on Mucus
C. jejuni NCTC 11168 was obtained from the American Type Culture Collection (ATCC 700819) and grown at 42°C under microaerophilic conditions (85% N2, 5% O2, and 10% CO2) in MCLMAN (medium cysteine leucine methionine aspartic acid niacinamide) broth medium (Alazzam et al., 2011) for maintenance. Growth curves were generated (O.D.600) by culturing C. jejuni NCTC11168 using the Bioscreen growth curve system (Growth Curves USA, Piscataway, NJ), with measurements taken each hour for 60 h. Cultures (10 replicates of each medium) were grown in 300 μl MCLMAN medium supplemented with 0.5% (w/v) purified mucus from chickens, turkeys, pigs, sheep, or cows, as well as MCLMAN without mucus.
Adherence and Invasion of Host Epithelial Cells
Human embryonic INT-407 intestinal epithelial cells were used to test adherence and invasion by C. jejuni (Negretti and Konkel, 2017), with the following modifications. In some experiments, INT-407 cells were infected with C. jejuni and turkey or pig mucus was added only during the time of infection, and in others, mucus was present while culturing C. jejuni, as well as during the infection. The day before the assay, 1.5 × 105 INT-407 cells were cultured at 37°C in 5% CO2 environment in wells of a 24 well plate using DMEM with 10% FBS containing penicillin and streptomycin (1X) and 10 mM HEPES buffer. For each treatment, INT-407 cells were plated in quadruplicate. The next day, cells were washed 3X in HBSS buffer containing calcium and magnesium prior to adding the inoculum. C. jejuni was grown on Mueller-Hinton plates at 42°C in a microaerophilic gas environment. At least 5 colonies were inoculated into the broth phase of biphasic liquid and agar (2% w/v) MCLMAN media. In some cases, the liquid phase of the biphasic media contained 0.5% (w/v) turkey or pig mucus. Inoculum was statically cultured for 48 h at 42°C in a microaerophilic gas environment. Motility of the inoculum was assessed by dark field microscopy. The inocula were prepared by adjusting the OD540 to 0.03 (3 × 107 cfu/mL) in HBSS containing 1% BSA (fraction V) with or without the addition of 0.5% (w/v) turkey or pig mucus. Adherence and invasion was also tested without the addition of mucus to the inocula. A MOI of approximately 100:1 was used by adding 500 μL of the inocula to each well. Infection was synchronized by centrifuging the plate at 800 × g for 5 min at room temperature. Cells were incubated for 3 h at 37°C with 5% CO2 cells were then washed 3X in 1mL of HBSS and lysed with the addition of 200 μL of Triton X-100 for 5 min at 37°C. Cell associated C. jejuni (adherent and invaded) were enumerated by serial dilution and culture on Mueller-Hinton plates. After 3 h incubation, invasion was measured by removing the media from cells plated and inoculated in the same manner as described above, washing cells 3X with HBSS, and adding 1 mL of DMEM containing 1% FBS and 250 μg/mL gentamicin back to each well. Cells were incubated for 3 h and then washed 3X in HBSS. Cells were lysed as described above, bacteria were enumerated, and reported as the number of invaded bacteria.
mRNA Isolation and Sequencing
C. jejuni was grown, while shaking under microaerophillic conditions, in 5.0 ml of MCLMAN liquid medium supplemented with 0.5% (w/v) of each host mucus and MCLMAN without mucus (four C. jejuni culture replicates of each). After a period of 24 h, the cultures were removed from the incubator and RNAprotect (QIAGEN, Germantown, MD) was added to each culture followed by RNA extraction using the RNeasy extraction kit (QIAGEN), following the manufacturer's instructions. DNA was removed using Turbo DNase (Ambion, Austin, TX). Total RNA quality was assessed with the 2100 Bioanalyzer, using RNA Nano chips (Agilent Tech., Santa Clara, CA). Ribosomal RNA was removed using the RiboZero system according to the manufacturer's instructions (Illumina, San Diego, CA), to enrich for total mRNA. Libraries were constructed using directional TruSeq RNA library kit and were sequenced on a HiSeq 2500 sequencer using the 100-cycle, high output mode (Illumina Inc., San Diego, CA).
Full Length mRNA Sequencing
Messenger RNA used for RNA-Seq analysis from C. jejuni grown on chicken mucus was sequenced using PacBio's long read technology to evaluate if antisense RNA resulted from pervasive transcription extending from adjacent upregulated mRNA. Total RNA was isolated as described above. Transcripts were polyadenylated followed by removal of rRNA using the RiboZero kit (Illumina, San Diego, CA, United States). After rRNA depletion, cDNA was synthesized and amplified using SMARTer cDNA system (Clontech Laboratories, Mountain View, CA, United States), and low and high molecular weight fractions were selected using the BluePippin instrument (Sage Science, Beverly, MA, United States). Low and high molecular weight libraries were sequenced using PACBIO RS II sequencer, implementing the Iso-Seq pipeline (Pacific Biosciences, Menlo Park, CA, United States).
Sequence Analysis
Sequence data was imported into CLC Genomic workbench V 8.0, and mapped to the C. jejuni NCTC 11168 reference sequence NC_002163 (Gundogdu et al., 2007). Reads were iteratively mapped to the genome in the following order: Mapped to the gene regions in the sense orientation, then the unmapped reads were mapped in the gene regions in the antisense orientation, and finally any remaining reads were mapped to intergenic regions. Expression values were calculated using only reads that mapped uniquely to a single location on the reference genome. Empirical analysis of differential gene expression was performed using the EdgeR statistical test, implemented in CLC Genomic workbench (Robinson et al., 2010). The data were normalized by scaling to the mean for graphical representations and presentation. A custom Pearl script was written to identify and bin all examples of differentially expressed genes [fold change >4.0, False Discovery Rate corrected (FDR) p < 0.01] in the sense orientation that were adjacent in the genome to differentially expressed genes in the antisense orientation. The criteria for these antisense-sense associations required the differentially expressed gene to be either the same gene as the antisense gene, or adjacent to it in the genome. If an association was identified, the next gene in the genome was evaluated. This continued until our fold change and FDR p-value threshold was not met.
Comparisons With Publicly Available Data
To improve our understanding of the relationship between asRNA, iron acquisition, and oxidative stress genes, data from a study evaluating PerR (global regulator for oxidative stress response) or Fur (global regulator for iron acquisition) single or double knockouts on C. jejuni 11168 gene expression under iron limited or replete conditions was analyzed (Butcher et al., 2015). Briefly, the cDNA libraries of the transcripts had been generated in a strand specific manner by the authors, but the antisense data was not evaluated for proximity or linkages to the sense transcripts. After downloading the reads from NCBI (accession number SRP044881), the directional reads were mapped and analyzed as described above. Antisense RNA and gene expression within our dataset were identified as Fur or PerR repressed based on comparisons with the results from the published gene knockout study.
Data Presentation
Analysis was performed using CLC Genomic workbench V 8.0 and PRIMER7 software v7.0.13 (Gorley and Clarke, 2008). PAST statistical analysis software (Hammer et al., 2001) was used for Permutational multivariate analysis of variance (PERMANOVA) tests. Differences with a False Discovery Rate (FDR) corrected P-value < 0.01 with at least a 4-fold change in gene expression was considered to be significant. Data are deposited in NCBI's Short Read Archive (SRA) associated with BioProject PRJNA411826.
Results
Growth Curves
C. jejuni grown in MCLMAN broth supplemented with intestinal mucus from various species (0.5% w/v) showed a significant enhancement in growth and terminal O.D. (P < 0.001) compared to defined media alone, suggesting that mucus components were used as a growth substrate (Figure 1). The terminal O.D.s varied slightly among mucus sources, but no significant differences were detected between avian or mammalian mucus. Cultures supplemented with mucus reached terminal stationary phase after 28 h of growth, while it took 38 h on MCLMAN without mucus.
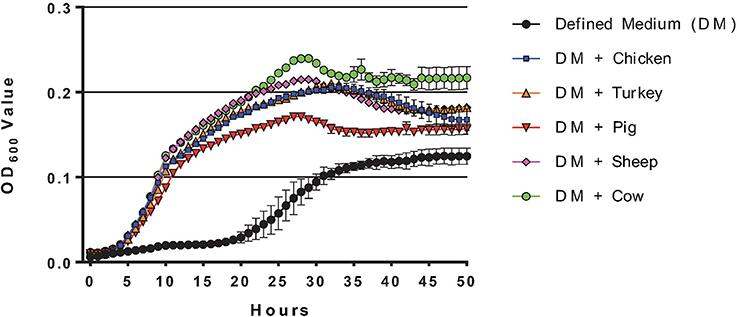
Figure 1. Growth curves of C. jejuni grown with and without mucus. Growth curves were generated from C. jejuni NCTC11168 grown in MCMAN defined media with, or without 0.5 % wt/vol mucus isolated from the small intestines of different animal species (Chicken, Turkey, Cow, Sheep, or Pig). Measurements were collected using a Bioscreen growth curve system (Growth Curves USA, Piscataway, NJ), with measurements taken each hour from 300 μl cultures. Standard errors are indicated for data points along the curve.
Mucus Effect of Cell-Association and Invasion of Intestinal Epithelial Cells
Addition of pig mucus [0.5% (w/v)] during the incubation stage of INT-407 cells with C. jejuni significantly enhanced (P < 0.001) the number of cell-associated and cell-invaded C. jejuni, compared to C. jejuni exposure with turkey mucus or without mucus (Figures 2A,B). In subsequent experiments, C. jejuni were cultured for 48 h in a defined media biphasic culture containing 0.5% (w/v) pig or turkey mucus, or no mucus in the liquid phase. Mucus was also present during the incubation stage of the assay. Culturing C. jejuni in either pig or turkey mucus significantly enhanced cell association and invasion, as compared to a control lacking mucus (Figures 2C,D). The number of invaded C. jejuni cultured in the presence of pig mucus was significantly different (P = 0.0013) compared to C. jejuni cultured in turkey mucus (Figure 2D).
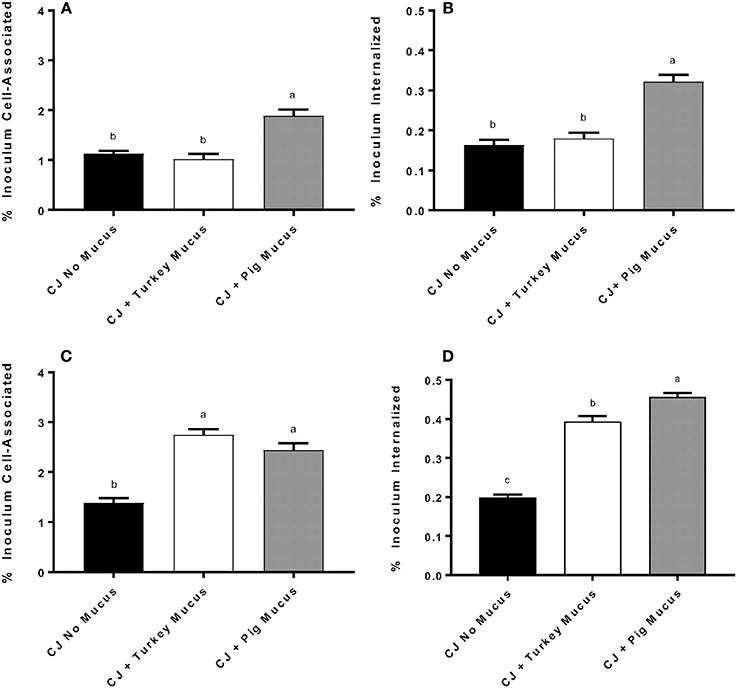
Figure 2. The animal source of mucus and its presence during growth or growth and adherence affect C. jejuni adherence and invasion of INT-407 cells. C. jejuni NCTC 11168 was cultured in defined medium with 0.5% (w/v) pig (CJ + pig mucus) or turkey (CJ + turkey mucus) mucus, or without mucus (CJ no mucus) and INT-407 cells were infected without mucus present during adherence (A) or invasion (B) phases. In other experiments, turkey or pig mucus was added to C. jejuni for growth in defined medium and during adherence (C) and invasion (D) phases. The MOI in each experiment was approximately 100:1. Data represent the mean ± SEM % cell-associated (A,C) or internalized (B,D) of four replicates and compared to the inoculum. Data were statistically analyzed using one-way ANOVA followed by a post-hoc multiple comparisons test (Tukey). Different letters in each panel represent significant differences (P < 0.05) in adherence or invasion of INT-407 cells by C. jejuni treated with or without mucus.
Mucus Composition
The crude mucus processing liberated mucus glycans from the protein backbone and enriched for O-linked neutral glycans (Martens et al., 2008). The total amount of protein present in each of the mucus preparations was low as determined by Bradford assays after purification [percentage/dry weight: chicken (0.12%), cow (0.15%), pig (0.08%), sheep (0.13%), and turkey (0.09%)], and compositional and structural analysis identified glycan sialic acid and differences between the avian and mammalian mucus (Table S1 and Figure S1). In each of the mucus samples, Fucose, N-Acetyl-galactosamine, N-Acetyl-glucosamine, Galactose, Glucose, and Mannose were present as glycan components. The sialic acids N-Glycolylneuraminic acid and N-Acetylneuraminic acid were both present in the glycans from cow, pig, and sheep mucus but N-Acetylneuraminic acid was the only sialic acid present in the chicken and turkey mucus (Table S1). Iron was not detectable in any of the mucus extractions by colorimetric assay (data not shown). The protein composition of each preparation was determined by MS/MS analysis (Table S2 and Figure S2), however no mucus proteins were identified, suggesting that glycans make up a large portion of the dry weight of the material isolated from each mucus (Figure S1).
C. jejuni Transcriptome
In total, 417,448,571 sequence reads were obtained after quality filtration. 311,343,359 reads (74.6%) were mapped to protein coding regions in the sense orientation, 14,659,287 reads (3.5%) mapped to intergenic regions and 843,531 reads (0.2%) mapped to protein coding regions in the antisense orientation (Table 1).
Defined Media vs. All Mucus
Gene expression profiles from C. jejuni grown on defined media or defined media supplemented with intestinal mucus from different hosts significantly differed [Table S3, P < 0.001, Permutational multivariate analysis of variance test (PERMANOVA)]. A principal component analysis plot (PCA) of all transcriptomes showed differences in total transcriptomes between C. jejuni grown in the presence of mucus compared to without mucus (Figure 3A). The increased growth rate of all the mucus-containing cultures likely contributed to many of the expression differences observed. The mucus-containing cultures were in the late-log growth phase at the 24-h sampling, while the defined media were still in early-log phase. Because of these growth-rate differences, discussion of genes differentially expressed between mucus-containing and defined media will be limited to genes known to be impacted by the presence of mucus. A set of genes, part of the fucose utilization genetic island present in several C. jejuni strains including NCTC11168, were up regulated (9–19 fold) for all the mucus cultures. This gene cluster (Cj0481-Cj0489) includes fucose utilization proteins and a transporter.
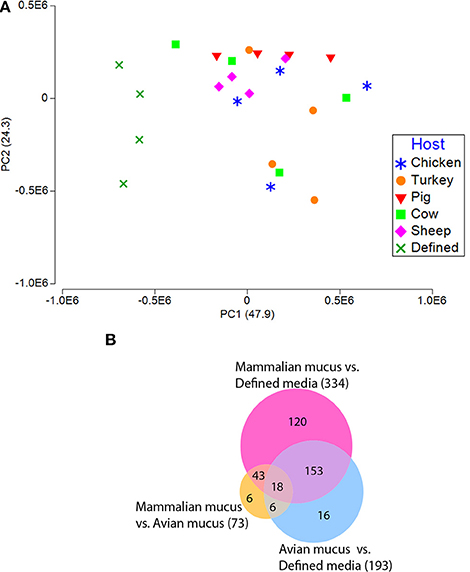
Figure 3. Transcriptomic profiles (sense) from C. jejuni grown on defined media with and without mucus isolated from avian and mammalian sources. (A) PCA plot generated from gene expression data of reads mapping to C. jejuni NCTC11168 coding regions of the reference genome in the sense orientation. (B) Venn diagram shows the number of differentially expressed genes (fdr p < 0.01, with > 4-fold difference) for all comparisons of avian mucus, mammalian mucus, and defined media.
Differences Among Avian and Mammalian Host Mucus
Mucus-containing C. jejuni cultures were each in the late-log growth phase at the 24-h sampling for transcriptomic analysis (Figure 1). PCA plots of the C. jejuni transcriptomes didn't show clear separation among mucus from different hosts (Figure 3A). Despite overlap in expression profiles, there were 73 genes differentially expressed between C. jejuni grown in avian (chicken and turkey) and mammalian (pig, cow, and sheep) mucus (Table 2, Figure 3B). Most of the genes differentially expressed had increased expression in the avian mucus (71 genes). The 2 genes that were upregulated in the mammalian mucus were rrc and fdxa (both associated with oxidative stress response) and both were ~14-fold higher when compared to the avian mucus. Most of the C. jejuni genes upregulated in the avian mucus are involved in iron acquisition, oxidative response, or were membrane proteins. The genes that were most increased in the avian mucus, including Cj1383c, chuC, Cj0177, exbD1, kata, are involved in iron acquisition and oxidative stress response (all were over 100-fold higher in the avian mucus compared to mammalian mucus). In all, there were 35 genes associated with iron acquisition, 10 oxidative stress genes, 13 putative membrane proteins, and 13 genes of various functions (such as the energy taxis response gene cetB), upregulated in C. jejuni due to avian mucus, compared to mammalian mucus (Table 2).
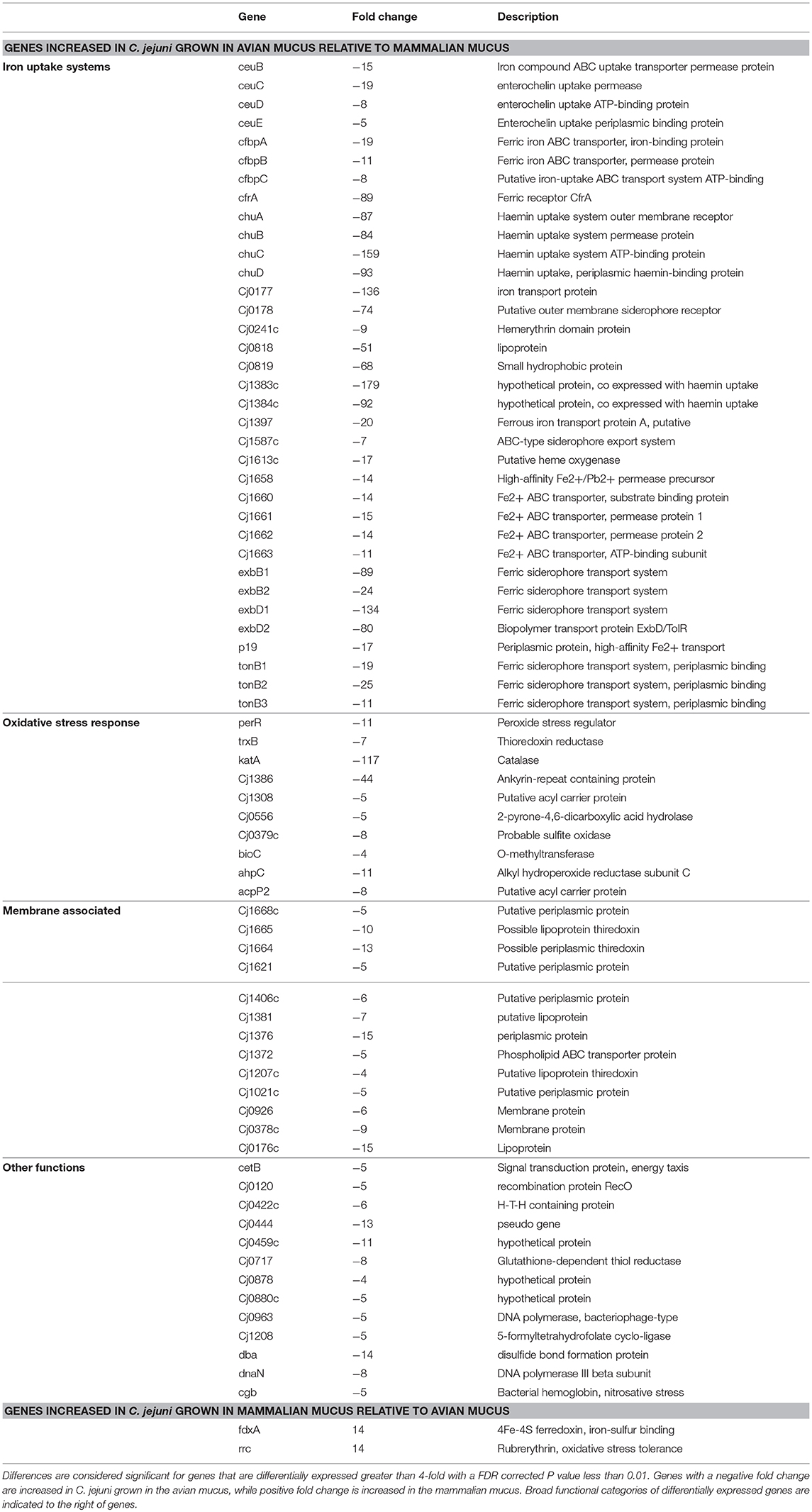
Table 2. Genes differentially expressed between avian mucus (chicken and turkey) and mammalian mucus (cow, pig, and sheep).
Differences Among Each Host Mucus
All comparisons among C. jejuni grown on different host mucus identified genes differentially expressed between each animal mucus, with the exception of cow mucus compared to pig mucus, which had no significant differences (Table S4). Other than the defined media alone, chicken mucus was the strongest driver of C. jejuni gene expression differences, yielding 157, 152, 115, and 113 genes differentially expressed from cow, pig, sheep, and turkey mucus, respectively. The turkey mucus also diverged from the mammalian mucus resulting in 46, 49, and 47 differentially expressed genes from cow, pig, and sheep mucus, respectively. While there were no detected differences between cow and pig mucus, they had 23 and 21 genes differentially expressed with sheep mucus, respectively. Many of the genes identified with these comparisons reflect the avian or mammalian origin of the mucus. For example, iron acquisition and oxidative stress genes were again increased in C. jejuni grown in the chicken or turkey mucus, compared to pig, cow or sheep mucus. Some of these iron-associated genes were increased ~1,000 or ~2,000 fold (see chicken comparisons with cow or pig mucus, Table S4) in the avian mucus. The same 2 genes (rrc and fdxa) that were upregulated in the mammalian mucus when compared to avian, were also increased in the individual mammalian host comparisons with each avian host. Surprisingly, many of the same iron acquisition genes that were increased in the avian mucus, were also increased in the sheep mucus (i.e., chuABD, tonB2, exbD2, exbB2, cfbpAB, p19, ceuB, etc), when sheep was compared to the other mammalian mucus (pig and cow), highlighting differences among animal species.
Antisense RNAs
Antisense sequences were separated from the C. jejuni transcriptome for parallel analysis. These transcripts were identified in the directional RNA-seq dataset by mapping the reads to genes in the antisense orientation. In all, 641 asRNAs, that mapped to the noncoding strand of genes, were identified in the dataset (had at least 10 reads). These asRNAs expression patterns segregated by source of mucus (host) in the C. jejuni growth media (Figure 4A). The C. jejuni grown on avian mucus (chicken or turkey) had asRNA expression profiles (PERMANOVA test) significantly different from the mammalian mucus' (cow, pig, or sheep); and both were different from that of the defined media, (p < 0.01), but there were no differences within groups (avian or mammalian). Many asRNAs were differentially present among C. jejuni grown in each host mucus as well as between defined media compared to all mucus types (Figure 4B). In many cases the asRNAs displayed atypical patterns, mapping to only portions of genes and intergenic regions.
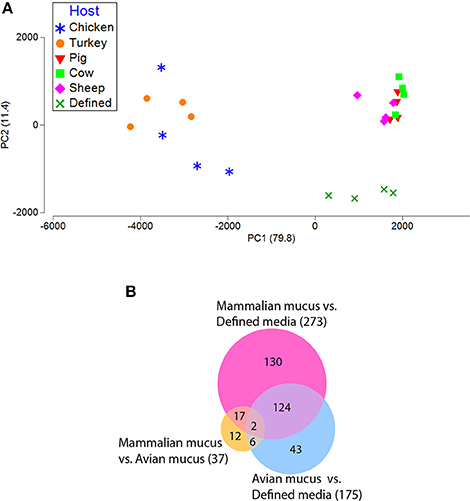
Figure 4. Antisense transcriptomic profiles among C. jejuni grown on avian mucus (chicken or turkey), mammalian mucus (cow, pig, or sheep), and the basal defined media without mucus. (A) PCA plot generated from antisense reads (map to non-coding strand of gene) from C. jejuni grown on defined media with and without mucus isolated from different host sources. (B) Venn diagram shows significant differences for all comparisons of avian mucus, mammalian mucus, and defined media for the antisense portion of the data (fdr p < 0.01, with > 4 fold difference).
Two C. jejuni genes (prfA and flip) had many antisense reads (relative to other asRNA) that mapped to the noncoding stand in the avian mucus cultures, and were also significantly increased in the avian mucus, compared to the mammalian mucus. Upon closer examination, it was observed that prfA and flip, while not differentially expressed in the sense orientation, were adjacent to differentially expressed genes in the sense orientation, within the same samples (Cj1613c next to prfA and Cj0819 next to fliP). Global analysis of all of the sense and antisense data showed that many asRNAs that were upregulated in the avian transcriptomes, were adjacent coding mRNAs that were upregulated in those same samples (Table 3). This phenomenon was not only observed in the avian samples, but was often associated with upregulated genes.
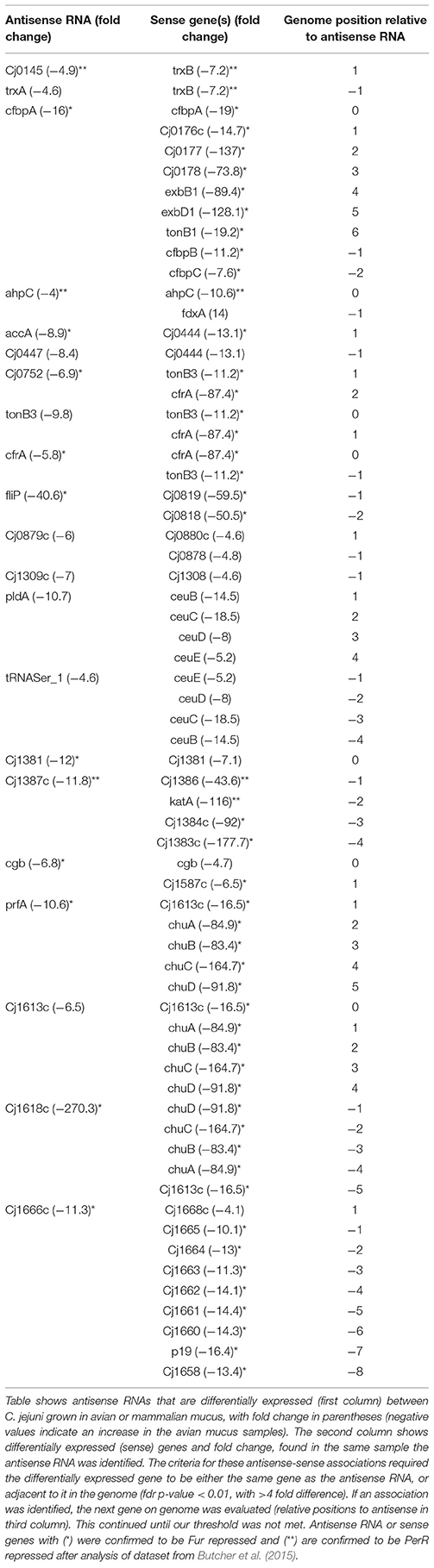
Table 3. Association between differentially expressed antisense reads with adjacent differentially expressed genes between C. jejuni grown on avian or mammalian mucus.
In addition to the positive associations among antisense and sense RNAs described above, negative associations among asRNA and their adjacent sense mRNA was observed with mucus comparisons with the defined media. The eight-gene fucose utilization operon (Cj0481-Cj0489), upregulated in all mucus cultures, had antisense reads mapping to the adjacent transcriptional regulator for the operon, Cj0480c, in the defined media cultures. Antisense RNA that mapped to Cj0480c was significantly increased while the rest of the operon was downregulated in the defined media. The close association between C. jejuni asRNAs and differentially expressed genes may suggest that these non-coding transcrips are associated to regulatory activities.
Many of the asRNAs upregulated in the avian mucus in this study were adjacent to genes associated with iron acquisition or oxidative stress response. To evaluate the role of the transcriptional regulators for iron acquisition (Fur) and oxidative stress response (PerR), public data were retrieved and re-analyzed from a report by Butcher et al. (2015) to evaluate the association between the asRNA and genes part of the Fur or PerR regulatory networks. In their study, Butcher et al. characterized C. jejuni NCTC11168 wild type, ΔFur, ΔPerR, and ΔFurΔPerR mutants under both iron-replete and iron-limited conditions to identify the Fur and PerR regulons. In doing so, they identified the Fur or PerR regulated genes under iron limited and iron replete conditions. The researchers used strand-specific RNA-seq, allowing the sense or antisense nature of the RNAs to be preserved in the data. We identified many of the same asRNAs, observed in our dataset, in our re-analysis of their data, and confirmed the associations of the asRNA in our study are part of the Fur or PerR regulons for iron acquisition or oxidative stress protection, respectively. The differentially expressed genes and asRNAs shared with our study are noted with an asterisk on Table 3.
Pervasive Transcription
We suspected that the detected asRNAs might be caused by delayed termination of mRNA, resulting in transcriptional read-through because the differentially expressed antisense reads correspond to genes arranged in the opposite direction. Read-through of RNA polymerase would create transcripts with an antisense portion if transcription continues into adjacent genes arranged in the opposite orientation. To test this, one of the replicates of C. jejuni grown in chicken mucus was subjected to full length cDNA sequencing on a PacBio instrument to evaluate the nature of the observed antisense RNAs. While the sequencing depth was low, we obtained sequences spanning regions where asRNA were adjacent to sense mRNAs. The most abundant asRNAs in the avian RNA-seq data mapped to the opposite stand of prfA, adjacent to the upregulated gene Cj1613c in the genome. Long reads spanning gene boundaries were observed in this region, suggesting that some antisense RNAs were being generated by failure of transcriptional termination to occur at gene boundaries, continuing into the non-coding stand of the open reading frame of the adjacent gene (Figure S3).
This transcriptional read-through did not always occur and did not appear to be proportional to transcriptional levels. For example, porA was the most highly expressed gene across all transcriptomes (1,151,741 reads average per sample, compared to 65,688 average reads mapping to Cj1613c in the avian samples). Immediately downstream of porA is dnaJ, in the opposite orientation as porA (similar to orientation of Cj1613c to prfA). Despite this, dnaJ only has on average only 5 antisense reads mapping to it in our dataset, compared to 6,264 antisense reads mapping to prfA in the avian mucus C. jejuni transcriptomes.
Discussion
In this study, we described C. jejuni transcriptional responses to avian or mammalian intestinal mucus from different host species. Niche adaptation is expected for host-associate microbes and C. jejuni's preferred environment is the mucus along the mucosa of the avian gut (Epps et al., 2013). Several studies have identified phenotypic responses of C. jejuni to mucus' or mucins, but a full understanding of how C. jejuni responds to these environmental cues is still lacking (Ganan et al., 2013; Naughton et al., 2013; Shortt et al., 2016). Avian mucus was shown to modulate pathogenicity by reducing C. jejuni cellular adherence and invasion (Alemka et al., 2012), and our findings where mucus was added only during inoculation period concur with these prior findings. However, our adherence and invasion results differ from previous work when C. jejuni was cultured in the presence of either mammalian or avian mucus. This may be explained by the different intestinal cell lines used, as we used the INT-407 cell line, which is highly sensitive to Campylobacter adherence and invasion (Monteville et al., 2003). In addition, the previous study only addressed the effect of adding mucus to a rich tissue culture medium during the adherence and invasion assay and used up to 20% mucus (w/v) compared to 0.5% in the present study. Incubating INT-407 cells with C. jejuni with or without mucus in a nutrient deficient medium (HBSS with 1% BSA) was less likely to affect gene expression of Campylobacter when cultured in the defined medium with mucus. C. jejuni is chemotactic to and preferentially binds avian mucus over mucus from other hosts, but despite the prevalence in the avian gut, C. jejuni isn't associated with pathology in avian hosts (Naughton et al., 2014). In fact, C. jejuni recognizes and binds multiple glycan structures found within mucus, including terminal mannose, N-acetylneuraminic acid, galactose and fucose (chemotaxis and flagellar genes were upregulated in the mucus cultures in this study, but just below the 4-fold cutoff applied) (Day et al., 2009). Adaptations to the host environment are important for pathogen and commensal survival within the animal intestinal tract. We observed genes important for survival in this intestinal niche to be upregulated when C. jejuni is exposed to mucus.
Many genes were upregulated in the defined (minimal) media (208 genes), many of these differences may be related to the different growth phases of C. jejuni in defined media (early log) compared to the different mucus medias (each were late log), because C. jejuni is known to alter its expression profiles in each growth phase (Wright et al., 2009). Of the 33 genes increased in the mucus cultures, those increased the greatest were the genes part of the fucose utilization operon (Cj0481-Cj0489), a response shared regardless of mucus source. These genes have been shown to be necessary for growth on fucose which is an abundant sugar found in mucin (Muraoka and Zhang, 2011), and C. jejuni NCTC11168 is one of several C. jejuni strains known to have this operon and to utilize fucose (Muraoka and Zhang, 2011; Stahl et al., 2011). Strains of C. jejuni from human and avian sources show affinities for fucose, suggesting its importance for persistence in the gut (Day et al., 2013). Despite the broad importance of fucose for colonization of the gut, fucose permease (fucP) knockout mutants have a colonization defect in mammals but not in the avian gut, suggesting additional substrates support C. jejuni colonization of the avian gut (Stahl et al., 2011).
Many genes upregulated in C. jejuni grown in avian mucus, compared to mammalian mucus, have been shown to be essential for colonization of the avian gut. Specifically genes associated with resistance to oxidative stress (e.g., perR, katA, ahpC, trxB) and iron (heme) binding and transport (e.g., ceuBCDE, cfbABC, chuABCD, exbB1, exbB2, exbD1, exbD2, p19, tonB1, tonB2, tonB3) (Hofreuter, 2014; Butcher et al., 2015) were significantly increased in the avian mucus cultures. Interestingly, not all iron transport genes were increased in the avian mucus. FeoB, involved in ferrous iron acquisition wasn't differentially expressed (1.4-fold difference with the mammalian mucus) (Naikare et al., 2006). Studies with knockout mutants have established that loss of genes involved in protection against oxidative and nitrosative stress, and iron acquisition reduce or eliminate C. jejuni colonization of the chicken gut (Palyada et al., 2004, 2009). C. jejuni similarly responds to bile, perhaps as an environmental cue, upregulating oxidative stress response genes after exposure to bile salts (Negretti et al., 2017). Many of the genes upregulated in avian mucus were also identified in a previous transcriptomic study of C. jejuni in chickens,in vivo (Taveirne et al., 2013). These include the oxidative and nitrosative stress response genes katA and cgb, and iron acquisition genes chuABCD and exbB-2, and exbD (Taveirne et al., 2013). Iron acquisition genes CJJ81176_1649 (Cj1658) and p19 were similarly upregulated in C. jejuni strain 81-176 when exposed to chicken cecal extracts from chicken or fecal extracts from humans (Liu et al., 2018). Many of these same iron acquisition and oxidative stress pathways are also upregulated in C. jejuni during human infection (Crofts et al., 2018).
Dual oxidase (DUOX2) (El Hassani et al., 2005) and nicotinamide-adenine dinucleotide phosphate (NADPH) oxidase 1 (NOX1) (Szanto et al., 2005) are reactive oxygen species (ROS)-producing NADPH oxidases highly expressed in the lower intestinal epithelium, and serve as one of the innate effector mechanisms to prevent bacterial invasion of the mucosa. The ROS generated from DUOX2 and NOX1 alter capsule synthesis in C. jejuni, reducing its virulence (Corcionivoschi et al., 2012). C. jejuni uses heme-containing enzymes to counter the effects of host-derived hydrogen peroxide defenses (Alvarez et al., 2016). The interaction of mucus shed into the small intestinal lumen and C. jejuni may serve as an early signal to upregulate genes involved in resistance to oxidative stress, and adapt C. jejuni to survive within cecal mucus (Beery et al., 1988), in close proximity to ROS-generating epithelium.
Other mucosal colonizers have been characterized with similar responses to mucus, by upregulating essential colonization pathways. Transcriptomic comparisons from mucus-associated and intestinal lumen associated Escherichia coli from monocolonized mice showed very different functional pathways being upregulated in each environment (Li et al., 2015). While pathways such as glycerol metabolism and fatty acid degradation were upregulated in the lumen, genes belonging to the Fur regulon, including iron uptake and mobilization genes, and oxidative stress response genes were upregulated in the mucus. C. jejuni has also been shown to respond to microbial-derived short chain fatty acids within the intestinal lumen by modulating gene expression, leading to colonization (34).
Pseudomonas aeruginosa, an opportunistic pathogen and potential colonizer of mucus in the respiratory tract of cystic fibrosis patients, upregulates iron acquisition genes, peroxide-detoxifying enzymes and small regulatory RNAs when exposed to human respiratory mucus in vitro (Cattoir et al., 2013; Gi et al., 2015). Many of the genes upregulated in C. jejuni in the presence of avian mucus (katA, catalase; ahpC, alkyl hydroperoxide reductase subunit C; trxB, thioredoxin; Cj1386c, ankyrin containing protein) have homologus genes upregulated in P aeruginosa in human mucus (Cattoir et al., 2013). Cj1386c is downstream from katA and enhances catalase activity by trafficking heme to catalase (Flint et al., 2012). It is unclear if the upregulation of katA and Cj1386 creates and iron demand, resulting in the upregulation of iron acquisition genes observed in this study. Oddly, superoxide dismutase (sodB) was not changed in C. jejuni in the avian mucus.
Here we show that antisense reads from C. jejuni grown in mucus from different host sources correlate with their respective host mucus environment [(avian; chicken or turkey), mammalian; (cow, pig, or sheep), or defined media; Figures 4A,B]. Several reports have described the existence of asRNAs in C. jejuni transcriptomes and have suggested roles in gene regulation because of the lack of many known transcriptional regulators in C. jejuni (Taveirne et al., 2013; Butcher et al., 2015). Antisense RNAs have also been observed upregulated in C. jejuni in vivo, suggesting important regulatory rolls in the intestinal environment compared to standard laboratory media (Taveirne et al., 2013). Of the 37 differentially expressed asRNA between avian and mammalian mucus cultures, 21 are adjacent to genes that were also differentially expressed. Most of these genes are regulated by one of two global regulators for iron acquisition or oxidative stress, Fur and PerR, respectively. Understanding this response is complicated by the overlapping regulons of Fur and PerR. We confirmed the association of these asRNA to the Fur or PerR regulons by re-analyzing the strand-specific data from a previously published study that evaluated C. jejuni NCTC11168 wild type, ΔFur, and ΔPerR under both iron-replete and iron-limited conditions (Butcher et al., 2015). Both of these regulators repress expression of genes in their respective regulon. Data generated by Butcher et al. (Butcher et al., 2015) showed that ΔFur, or ΔPerR mutants failed to repress asRNA production (upregulated in mutants), as well as the genes belonging to each regulon, when compared to wild type C. jejuni NCTC11168. Most of the asRNA and adjacent differentially expressed genes in our study appeared in their dataset (Table 3). How these asRNAs may associated with adjacent genes, and why they are impacted by avian mucus is not clear.
Information about longer transcripts or transcriptional read through is lost with the short reads obtained from RNA-seq. To examine long transcripts, we sequenced the mRNA from one of the samples using the PacBio platform to see if the observed asRNAs were small elements or part of a longer transcripts. We confirmed that some of these asRNAs are part of larger transcripts that span gene boundaries (low sequencing depth prevented us from evaluating all asRNA). With broad use of strand-specific RNA-seq, there have been many other reports of bacterial transcription crossing gene boundaries and starting or terminating mid-gene, including the non-coding strand resulting in antisense transcripts (Wade and Grainger, 2014; Bidnenko et al., 2017). This non-canonical transcription has been named “pervasive transcription” and has been observed in all domains of life (Berretta and Morillon, 2009; Lybecker et al., 2014). The role of pervasive transcription in bacteria is unknown and resulting transcripts remain uncharacterized, but it has been observed in many bacterial species (Lybecker et al., 2014; Wade and Grainger, 2014). Whether the identified asRNA results from pervasive transcription or have regulatory functions requires further experiments.
Conclusion
C. jejuni has a commensalistic relationship with its avian hosts, yet can be pathogenic in the mammalian intestinal tract, including humans. In the avian gut, C. jejuni colonizes mucus in the intestinal crypts, without pathology or disease in the host. Previous studies suggest intestinal mucus may contribute to the different responses of C. jejuni in the diverse gut environments. We showed that avian mucus modulates C. jejuni adherence and invasion of host cells, and impacts transcriptomic response, different from that of C. jejuni grown in mammalian mucus. Many of the genes upregulated when C. jejuni was grown in avian mucus, are essential for colonization of the avian gut. Understanding how C. jejuni responds to and interacts with the avian gut environment may identify intervention targets for reducing C. jejuni prevalence in the food chain.
Author Contributions
TL conceived and designed the study, as well as in drafted the manuscript. TL, BC, LL, JL, TR, and MS performed the experiments and collected data. TL, GC, and TC analyzed and interpreted the data. TC and MS revised the paper for important content.
Conflict of Interest Statement
The authors declare that the research was conducted in the absence of any commercial or financial relationships that could be construed as a potential conflict of interest.
Acknowledgments
We would like to thank Brian Brunelle and Timothy Johnson for helpful scientific advice. We are grateful to the excellent animal caretakers at the NADC. Mention of trade names or commercial products in this publication is solely for the purpose of providing specific information and does not imply recommendation or endorsement by the US Department of Agriculture. USDA is an equal opportunity provider and employer.
Supplementary Material
The Supplementary Material for this article can be found online at: https://www.frontiersin.org/articles/10.3389/fmicb.2018.03215/full#supplementary-material
References
Alazzam, B., Bonnassie-Rouxin, S., Dufour, V., and Ermel, G. (2011). MCLMAN, a new minimal medium for Campylobacter jejuni NCTC 11168. Res. Microbiol. 162, 173–179. doi: 10.1016/j.resmic.2010.09.024
Alemka, A., Corcionivoschi, N., and Bourke, B. (2012). Defense and adaptation: the complex inter-relationship between Campylobacter jejuni and mucus. Front. Cell Infect. Microbiol. 2:15. doi: 10.3389/fcimb.2012.00015
Alemka, A., Whelan, S., Gough, R., Clyne, M., Gallagher, M. E., Carrington, S. D., et al. (2010). Purified chicken intestinal mucin attenuates Campylobacter jejuni pathogenicity in vitro. J. Med. Microbiol. 59, 898–903. doi: 10.1099/jmm.0.019315-0
Alvarez, L. A., Kovacic, L., Rodriguez, J., Gosemann, J. H., Kubica, M., Pircalabioru, G. G., et al. (2016). NADPH oxidase-derived H2O2 subverts pathogen signaling by oxidative phosphotyrosine conversion to PB-DOPA. Proc. Natl. Acad. Sci. U.S.A. 113, 10406–10411. doi: 10.1073/pnas.1605443113
Beery, J. T., Hugdahl, M. B., and Doyle, M. P. (1988). Colonization of gastrointestinal tracts of chicks by Campylobacter jejuni. Appl. Environ. Microbiol. 54, 2365–2370.
Berretta, J., and Morillon, A. (2009). Pervasive transcription constitutes a new level of eukaryotic genome regulation. EMBO Rep. 10, 973–982. doi: 10.1038/embor.2009.181
Bidnenko, V., Nicolas, P., Grylak-Mielnicka, A., Delumeau, O., Auger, S., Aucouturier, A., et al. (2017). Termination factor Rho: from the control of pervasive transcription to cell fate determination in Bacillus subtilis. PLoS Genet. 13,e1006909. doi: 10.1371/journal.pgen.1006909
Bradford, M. M. (1976). A rapid and sensitive method for the quantitation of microgram quantities of protein utilizing the principle of protein-dye binding. Anal. Biochem. 72, 248–254. doi: 10.1016/0003-2697(76)90527-3
Butcher, J., Handley, R. A., Van Vliet, A. H., and Stintzi, A. (2015). Refined analysis of the Campylobacter jejuni iron-dependent/independent Fur- and PerR-transcriptomes. BMC Genomics 16:498. doi: 10.1186/s12864-015-1661-7
Byrne, C. M., Clyne, M., and Bourke, B. (2007). Campylobacter jejuni adhere to and invade chicken intestinal epithelial cells in vitro. Microbiology 153, 561–569. doi: 10.1099/mic.0.2006/000711-0
Cattoir, V., Narasimhan, G., Skurnik, D., Aschard, H., Roux, D., Ramphal, R., et al. (2013). Transcriptional response of mucoid Pseudomonas aeruginosa to human respiratory mucus. MBio 3, e00410–00412. doi: 10.1128/mBio.00410-12
Corcionivoschi, N., Alvarez, L. A., Sharp, T. H., Strengert, M., Alemka, A., Mantell, J., et al. (2012). Mucosal reactive oxygen species decrease virulence by disrupting Campylobacter jejuni phosphotyrosine signaling. Cell Host Microbe 12, 47–59. doi: 10.1016/j.chom.2012.05.018
Crofts, A. A., Poly, F. M., Ewing, C. P., Kuroiwa, J. M., Rimmer, J. E., Harro, C., et al. (2018). Campylobacter jejuni transcriptional and genetic adaptation during human infection. Nat. Microbiol. 3, 494–502. doi: 10.1038/s41564-018-0133-7
Damerell, D., Ceroni, A., Maass, K., Ranzinger, R., Dell, A., and Haslam, S. M. (2012). The GlycanBuilder and GlycoWorkbench glycoinformatics tools: updates and new developments. Biol. Chem. 393, 1357–1362. doi: 10.1515/hsz-2012-0135
Day, C. J., Tiralongo, J., Hartnell, R. D., Logue, C. A., Wilson, J. C., Von Itzstein, M., et al. (2009). Differential carbohydrate recognition by Campylobacter jejuni strain 11168: influences of temperature and growth conditions. PLoS ONE 4,e4927. doi: 10.1371/journal.pone.0004927
Day, C. J., Tram, G., Hartley-Tassell, L. E., Tiralongo, J., and Korolik, V. (2013). Assessment of glycan interactions of clinical and avian isolates of Campylobacter jejuni. BMC Microbiol 13,228. doi: 10.1186/1471-2180-13-228
El Hassani, R. A., Benfares, N., Caillou, B., Talbot, M., Sabourin, J.-C., Belotte, V., et al. (2005). Dual oxidase2 is expressed all along the digestive tract. Am. J. Physiol. 288, G933–G942. doi: 10.1152/ajpgi.00198.2004
Epps, S. V., Harvey, R. B., Hume, M. E., Phillips, T. D., Anderson, R. C., and Nisbet, D. J. (2013). Foodborne Campylobacter: infections, metabolism, pathogenesis and reservoirs. Int. J. Environ. Res. Public Health 10, 6292–6304. doi: 10.3390/ijerph10126292
Flint, A., Sun, Y. Q., and Stintzi, A. (2012). Cj1386 is an ankyrin-containing protein involved in heme trafficking to catalase in Campylobacter jejuni. J Bacteriol 194, 334–345. doi: 10.1128/JB.05740-11
Ganan, M., Martinez-Rodriguez, A. J., Carrascosa, A. V., Vesterlund, S., Salminen, S., and Satokari, R. (2013). Interaction of Campylobacter spp. and human probiotics in chicken intestinal mucus. Zoonoses Public Health 60, 141–148. doi: 10.1111/j.1863-2378.2012.01510.x
Gi, M., Lee, K.-M., Kim, S. C., Yoon, J.-H., Yoon, S. S., and Choi, J. Y. (2015). A novel siderophore system is essential for the growth of Pseudomonas aeruginosa in airway mucus. Sci. Rep. 5,14644. doi: 10.1038/srep14644
Gorley, A. M., and Clarke, K. (2008). PERMANOVA + for PRIMER: Guide to Software and Statistical Methods. Plymouth: PRIMER-E.
Gundogdu, O., Bentley, S. D., Holden, M. T., Parkhill, J., Dorrell, N., and Wren, B. W. (2007). Re-annotation and re-analysis of the Campylobacter jejuni NCTC11168 genome sequence. BMC Genomics 8,162. doi: 10.1186/1471-2164-8-162
Hammer, Ø., Harper, D., and Ryan, P. (2001). Paleontological Statistics Software: Package for Education and Data Analysis. Palaeontologia Electronica.
Hofreuter, D. (2014). Defining the metabolic requirements for the growth and colonization capacity of Campylobacter jejuni. Front. Cell Infect. Microbiol. 4,137. doi: 10.3389/fcimb.2014.00137
Hugdahl, M. B., Beery, J. T., and Doyle, M. P. (1988). Chemotactic behavior of Campylobacter jejuni. Infect. Immun. 56, 1560–1566.
Larson, C. L., Shah, D. H., Dhillon, A. S., Call, D. R., Ahn, S., Haldorson, G. J., et al. (2008). Campylobacter jejuni invade chicken LMH cells inefficiently and stimulate differential expression of the chicken CXCLi1 and CXCLi2 cytokines. Microbiology 154, 3835–3847. doi: 10.1099/mic.0.2008/021279-0
Lertsethtakarn, P., Ottemann, K. M., and Hendrixson, D. R. (2011). Motility and chemotaxis in Campylobacter and Helicobacter. Annu. Rev. Microbiol. 65, 389–410. doi: 10.1146/annurev-micro-090110-102908
Li, H., Limenitakis, J. P., Fuhrer, T., Geuking, M. B., Lawson, M. A., Wyss, M., et al. (2015). The outer mucus layer hosts a distinct intestinal microbial niche. Nat. Commun. 6,8292. doi: 10.1038/ncomms9292
Liu, M. M., Boinett, C. J., Chan, A. C. K., Parkhill, J., Murphy, M. E. P., and Gaynor, E. C. (2018). Investigating the Campylobacter jejuni transcriptional response to host intestinal extracts reveals the involvement of a widely conserved iron uptake system. MBio 9. doi: 10.1128/mBio.01347-18
Lybecker, M., Bilusic, I., and Raghavan, R. (2014). Pervasive transcription: detecting functional RNAs in bacteria. Transcription 5,e944039. doi: 10.4161/21541272.2014.944039
Marder, E. P. (2017). Incidence and trends of infections with pathogens transmitted commonly through food and the effect of increasing use of culture-independent diagnostic tests on surveillance—Foodborne diseases active surveillance network, 10 US Sites, 2013–2016. MMWR Morb. Mortal. Wkly. Rep. 66, 397–403. doi: 10.15585/mmwr.mm6615a1
Martens, E. C., Chiang, H. C., and Gordon, J. I. (2008). Mucosal glycan foraging enhances fitness and transmission of a saccharolytic human gut bacterial symbiont. Cell Host Microbe 4, 447–457. doi: 10.1016/j.chom.2008.09.007
Monteville, M. R., Yoon, J. E., and Konkel, M. E. (2003). Maximal adherence and invasion of INT 407 cells by Campylobacter jejuni requires the CadF outer-membrane protein and microfilament reorganization. Microbiology 149, 153–165. doi: 10.1099/mic.0.25820-0
Muraoka, W. T., and Zhang, Q. (2011). Phenotypic and genotypic evidence for L-fucose utilization by Campylobacter jejuni. J. Bacteriol. 193, 1065–1075. doi: 10.1128/JB.01252-10
Naikare, H., Palyada, K., Panciera, R., Marlow, D., and Stintzi, A. (2006). Major role for FeoB in Campylobacter jejuni ferrous iron acquisition, gut colonization, and intracellular survival. Infect. Immun. 74, 5433–5444. doi: 10.1128/IAI.00052-06
Naughton, J., Duggan, G., Bourke, B., and Clyne, M. (2014). Interaction of microbes with mucus and mucins: recent developments. Gut Microbes 5, 48–52. doi: 10.4161/gmic.26680
Naughton, J. A., Marino, K., Dolan, B., Reid, C., Gough, R., Gallagher, M. E., et al. (2013). Divergent mechanisms of interaction of Helicobacter pylori and Campylobacter jejuni with mucus and mucins. Infect. Immun. 81, 2838–2850. doi: 10.1128/IAI.00415-13
Negretti, N. M., Gourley, C. R., Clair, G., Adkins, J. N., and Konkel, M. E. (2017). The food-borne pathogen Campylobacter jejuni responds to the bile salt deoxycholate with countermeasures to reactive oxygen species. Sci. Rep. 7,15455. doi: 10.1038/s41598-017-15379-5
Negretti, N. M., and Konkel, M. E. (2017). Methods to study Campylobacter jejuni adherence to and invasion of host epithelial cells. Methods Mol. Biol. 1512, 117–127. doi: 10.1007/978-1-4939-6536-6_11
Palyada, K., Sun, Y. Q., Flint, A., Butcher, J., Naikare, H., and Stintzi, A. (2009). Characterization of the oxidative stress stimulon and PerR regulon of Campylobacter jejuni. BMC Genomics 10,481. doi: 10.1186/1471-2164-10-481
Palyada, K., Threadgill, D., and Stintzi, A. (2004). Iron acquisition and regulation in Campylobacter jejuni. J. Bacteriol. 186, 4714–4729. doi: 10.1128/JB.186.14.4714-4729.2004
Reinhardt, T. A., Lippolis, J. D., Nonnecke, B. J., and Sacco, R. E. (2012). Bovine milk exosome proteome. J. Proteomics 75, 1486–1492. doi: 10.1016/j.jprot.2011.11.017
Robinson, M. D., Mccarthy, D. J., and Smyth, G. K. (2010). edgeR: a Bioconductor package for differential expression analysis of digital gene expression data. Bioinformatics 26, 139–140. doi: 10.1093/bioinformatics/btp616
Ruiz-Palacios, G. M. (2007). The health burden of Campylobacter infection and the impact of antimicrobial resistance: playing chicken. Clin. Infect. Dis. 44, 701–703. doi: 10.1086/509936
Shortt, C., Scanlan, E., Hilliard, A., Cotroneo, C. E., Bourke, B., and C, T. O. (2016). DNA supercoiling regulates the motility of Campylobacter jejuni and is altered by growth in the presence of chicken mucus. MBio 7,e01227–16. doi: 10.1128/mBio.01227-16
Stahl, M., Friis, L. M., Nothaft, H., Liu, X., Li, J., Szymanski, C. M., et al. (2011). l-Fucose utilization provides Campylobacter jejuni with a competitive advantage. Proc. Natl. Acad. Sci. U.S.A. 108, 7194–7199. doi: 10.1073/pnas.1014125108
Stahl, M., Frirdich, E., Vermeulen, J., Badayeva, Y., Li, X., Vallance, B. A., et al. (2016). The helical shape of Campylobacter jejuni promotes in vivo pathogenesis by aiding transit through intestinal mucus and colonization of crypts. Infect. Immun. 84, 3399–3407. doi: 10.1128/IAI.00751-16
Szanto, I., Rubbia-Brandt, L., Kiss, P., Steger, K., Banfi, B., Kovari, E., et al. (2005). Expression of NOX1, a superoxide-generating NADPH oxidase, in colon cancer and inflammatory bowel disease. J. Pathol. 207, 164–176. doi: 10.1002/path.1824
Taveirne, M. E., Theriot, C. M., Livny, J., and Dirita, V. J. (2013). The complete Campylobacter jejuni transcriptome during colonization of a natural host determined by RNAseq. PLoS ONE 8,e73586. doi: 10.1371/journal.pone.0073586
Wade, J. T., and Grainger, D. C. (2014). Pervasive transcription: illuminating the dark matter of bacterial transcriptomes. Nat. Rev. Microbiol. 12, 647–653. doi: 10.1038/nrmicro3316
Wright, J. A., Grant, A. J., Hurd, D., Harrison, M., Guccione, E. J., Kelly, D. J., et al. (2009). Metabolite and transcriptome analysis of Campylobacter jejuni in vitro growth reveals a stationary-phase physiological switch. Microbiology 155, 80–94. doi: 10.1099/mic.0.021790-0
Keywords: Campylobacter jejuni, mucus, gene expression, RNAseq, antisense RNA
Citation: Looft T, Cai G, Choudhury B, Lai LX, Lippolis JD, Reinhardt TA, Sylte MJ and Casey TA (2019) Avian Intestinal Mucus Modulates Campylobacter jejuni Gene Expression in a Host-Specific Manner. Front. Microbiol. 9:3215. doi: 10.3389/fmicb.2018.03215
Received: 10 October 2018; Accepted: 11 December 2018;
Published: 07 January 2019.
Edited by:
Axel Cloeckaert, Institut National de la Recherche Agronomique (INRA), FranceReviewed by:
Michael Konkel, Washington State University, United StatesBilly Bourke, Our Lady's Children's Hospital, Ireland
Copyright © 2019 Looft, Cai, Choudhury, Lai, Lippolis, Reinhardt, Sylte and Casey. This is an open-access article distributed under the terms of the Creative Commons Attribution License (CC BY). The use, distribution or reproduction in other forums is permitted, provided the original author(s) and the copyright owner(s) are credited and that the original publication in this journal is cited, in accordance with accepted academic practice. No use, distribution or reproduction is permitted which does not comply with these terms.
*Correspondence: Torey Looft, VG9yZXkuTG9vZnRAdXNkYS5nb3Y=