- Department of Microbiology and Immunology, University of Western Ontario, London, ON, Canada
Understanding host pathogen interactions is paramount to the development of novel antimicrobials. An important facet of this pursuit is the accurate characterization of pathogen replication within infected host cells. Here we describe the use of a fluorescence-based proliferation assay to identify intracellular populations of replicating bacteria at the subcellular level. Using Staphylococcus aureus as a model Gram-positive bacterial pathogen and macrophages as a model host phagocyte, we demonstrate this assay can be used to reliably identify individual phagocytes that contain replicating bacteria. Furthermore, we demonstrate this assay is compatible with additional cellular probes that enable characterization of cellular compartments in which replicating bacteria reside. Finally, we demonstrate that this assay facilitates the investigation of both Gram-negative and Gram-positive bacteria within host cells.
Introduction
The interaction of macrophages with bacteria often leads to ingestion of the microbe through phagocytosis which culminates with the formation of a membrane-bound organelle containing the microbial prey (Underhill and Ozinsky, 2002; Fairn and Grinstein, 2012). This vacuole, otherwise known as a phagosome, is not antimicrobial per se, however, through a complex sequence of interactions with the endo-lysosomal network, phagosomes mature into phagolysosomes that are markedly acidic and microbicidal (Flannagan et al., 2015). Not surprisingly, many successful pathogens with an intracellular lifestyle have evolved mechanisms to perturb phagolysosome formation or to resist phagolysosomal killing to promote bacterial survival (reviewed in Flannagan et al., 2009; Sarantis and Grinstein, 2012).
The molecular mechanisms employed by bacteria to promote intracellular survival can vary dramatically and, not surprisingly, this can impact on replication kinetics, where replication occurs, and host cell viability. For instance, Listeria monocytogenes and Staphylococcus aureus, which both can replicate within macrophages, do so in entirely different intracellular compartments (i.e., the cytoplasm and phagolysosome, respectively) (Portnoy et al., 1988, 2002; Flannagan et al., 2016, 2018a). In contrast, Staphylococcus lugdunensis, which remains viable within macrophages, fails to replicate altogether (Flannagan et al., 2018b). Due to these interactions, it is essential for investigators to have the tools to accurately establish whether phagocytosed bacteria replicate and to establish in which subcellular niche replications occurs. Routinely this entails performing phagocytosis or cell invasion assays followed by lysis and plating for colony forming units at various times post-infection (Edwards and Massey, 2011; VanCleave et al., 2017). While this approach will indicate whether a pathogen demonstrates robust growth, unique differences between individual host cells will be lost. Moreover, if the pathogen growth occurs in a subset of cells, proliferation may go unrecognized as colony counts are averaged over an entire host cell population.
To obtain further insight into the host-pathogen interaction, fluorescence microscopy is often employed which can permit visualization of infected cells and aid in the localization of bacteria to subcellular niches. Using approaches such as this we, and others, have characterized the intracellular compartment in which phagocytosed S. aureus resides within macrophages (Jubrail et al., 2015; Tranchemontagne et al., 2015; Flannagan et al., 2016). However, through investigation of S. aureus within macrophages we uncovered difficulty in accurately distinguishing bacteria that are replicating from those that are not. Therefore, we have developed a fluorescence-based bacterial proliferation assay that allows for the accurate identification of intracellular cocci that are proliferating and present this method here (Flannagan et al., 2016, 2018a,b). This assay is based on the principle that bacteria that are covalently labeled with a finite amount of a fluorescent compound or fluorophore will remain fluorescently labeled so long as they fail to replicate (Figure 1). In contrast, bacteria of the same starting population that commence replicating will eventually appear negative for fluorescence because continued cell division results in dilution of the fluorescent compound between daughter cells (Figure 1). Importantly, this assay can be utilized in parallel with additional cellular probes such as LysotrackerTM staining or LAMP-1 immunofluorescence to identify and characterize the niche in which replicating bacteria reside (Flannagan et al., 2016, 2018a,b). Here we describe this assay in detail and provide salient examples of its application to the characterization of S. aureus in context of the aforementioned cellular probes. Furthermore, we demonstrate that this approach can in principle also be employed for the analysis of Gram-negative bacteria. This approach should be applicable to the study of a variety of Gram-positive and Gram-negative bacterial pathogens.
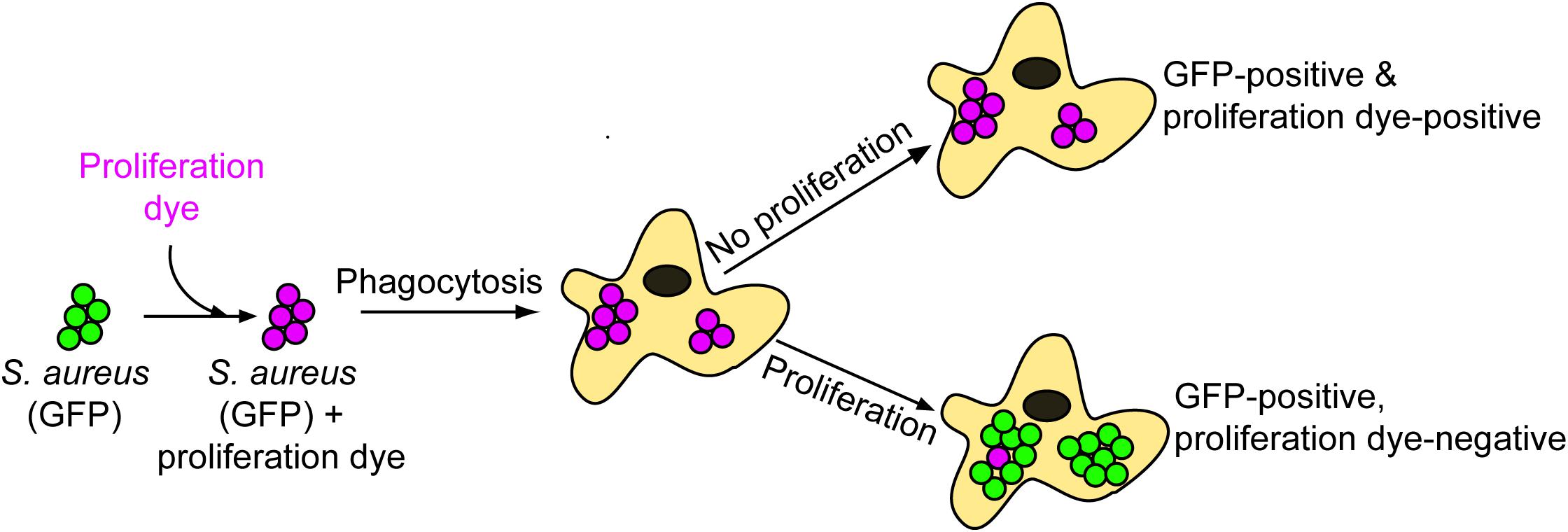
Figure 1. Schematic representation of the eFluor-proliferation assay. When setting up an infection GFP bacteria (or a red fluorescent protein) are incubated with amine-reactive eFluorTM-670 rendering the bacteria positive for both fluorophores. These double positive bacteria are incubated with macrophages and after phagocytosis and treatment with gentamicin, infected macrophages are imaged by fluorescence microscopy. The appearance of bacteria that are GFP-positive yet eFluorTM-670-negative indicates that bacterial replication has occurred. In contrast, bacteria that remain positive for both fluorophores have not replicated. Through replication, the proliferation dye becomes increasingly diluted between daughter cells and is eventually undetectable.
Materials and Methods
Maintenance and Growth of S. aureus USA300 for Cellular Infection
Staphylococcus aureus strain USA300 LAC, cured of its endogenous antibiotic resistance plasmid, was maintained as a frozen stock in tryptic soy broth with 15% (v/v) glycerol. This strain was also transformed with one of a few constitutive GFP-expression vectors (described in Table 1) that have previously been described to render the entire bacterial population GFP-positive. From the frozen stock S. aureus USA300 was streaked onto Tryptic Soy Broth plates containing 1.5% (w/v) agar and antibiotics as appropriate and incubated at 37°C overnight (∼ 16 h) until isolated colonies were visible. This plate, kept at room temperature, was used to initiate over-night broth cultures of S. aureus for a period of 3 days where after that a new streak plate was created from the frozen bacterial stock. Overnight cultures of S. aureus were started from an isolated colony that was inoculated into a 20 mL sterile glass test tube containing 5 mL TSB with antibiotics as needed. Subsequently the culture was incubated at 37°C with continuous shaking at 250 rpm for ∼16 h yielding an overnight culture with an optical density at 600 nm (OD600 nm) of ∼8 to 10. For growth of S. aureus in the presence of antibiotics the following concentrations were used: erythromycin and lincomycin, 3 and 20 μg/mL, respectively; chloramphenicol, 12 μg/mL.
Labeling of S. aureus With the Fluorescent Proliferation Dye
To begin to set up an infection, 1 mL of the over-night culture of GFP-expressing S. aureus in TSB is centrifuged at 18000 ×g for 1 min. After removal of the supernatant the bacterial pellet is re-suspended in 1 mL of sterile saline (0.9% w/v NaCl in ddH20) and centrifuged again. Next, the resulting pellet is re-suspended in 1 mL of sterile saline containing 1.25 μm eBioscienceTM Cell Proliferation Dye eFluorTM 670 (Thermo Fisher Scientific, cat No. 65-0840-85; eFluorTM-670) and incubated at room-temperature for 5 min. Note: eFluorTM-670 is diluted into saline from a stock solution immediately prior to use and before re-suspending the washed bacterial pellet. The eFluorTM-670 compound is an amine reactive dye that will non-specifically label any protein or structure on the cell surface of S. aureus that contains primary amines. After eFluorTM-670 labeling the bacterial cells are pelleted (the pellet may now appear blue in color to the naked eye) and the supernatant removed. To quench any unreacted dye and wash the cells the resulting bacterial pellet is re-suspended in 1 mL of bacterial culture media such as TSB, which contains an abundance of protein and peptides, and is incubated for 2 min at room-temperature. After centrifugation, the bacteria are washed once with sterile saline and then re-suspended in 1 mL of serum-free RPMI-1640 (Wisent Inc.) that has been brought to room-temperature. Next the OD600nm of this 1 mL bacterial suspension is measured and is used to generate a 1 mL suspension of bacteria that is diluted to an OD600nm equivalent of 0.5 or ∼4.4 × 108 CFU/mL for wild-type S. aureus USA300. For each strain employed, the concentration of cells for an OD600nm of 0.5 should be confirmed as the bacterial cell count is required to set up infections at an appropriate multiplicity of infection (MOI).
For macrophage infections, an MOI of 10 bacteria per macrophage is routinely employed in our laboratory. Due to the voracious capacity of macrophages to phagocytose, an MOI of more than 10 typically results in leukocytes that are engorged with bacteria at very early times after infection. These infections are performed in 12-well tissue culture plates containing ∼6.0 × 105 macrophages per well (setup described below) meaning that 6.0 × 106 bacteria are added per well containing macrophages. To generate a single suspension containing GFP-expressing S. aureus USA300 labeled with eFluorTM-670, typically, 163 μL of the labeled bacterial suspension at OD600nm 0.5 is added to 12 mL of serum-free RPMI that has been warmed to 37°C. This suspension is used to infect macrophages as described below.
Growth and Fluorescent Proliferation Dye Labeling of Gram-Negative Bacteria
Gram-negative bacteria including Citrobacter rodentium, Escherichia coli and Yersinia pseudotuberculosis (described in Table 1) were cultured from bacterial glycerol stocks maintained at -80°C. Each bacterium was streaked from a frozen stock onto LB agar plates and grown at 37°C. Next, an isolated colony from each bacterial species was inoculated into 20 mL sterile glass test tubes containing 5 mL of LB broth and grown at 37°C with shaking at 250 rpm overnight. Where appropriate E. coli carrying the GFP expression vector pFPV25.1 (Valdivia and Falkow, 1996) was cultured in the presence of ampicillin (100 μg/mL).
To label Gram-negative bacteria, 500 μL of the overnight culture was pelleted by centrifugation and washed 1× with sterile saline [0.9% (w/v) NaCl in ddH2O]. The cells were again pelleted and washed with 1 mL of 1× PBS pH 8.0. The resulting cell pellet was then re-suspended in 1 mL PBS pH 8.0 containing 2.5 μm of eBioscienceTM Cell Proliferation Dye eFluorTM 670 and incubated for 10 min at room temperature. Technical note: labeling of Gram-negative bacteria was more efficient in PBS at pH 8.0 than in our saline solution. After 10 min, the bacteria were pelleted, washed 1× in sterile LB to quench any unreacted dye, and were then analyzed or used experimentally.
Macrophage Culture
Routinely, infections in our laboratory are performed in primary murine and human macrophages or immortalized macrophage cell lines.
1. Immortalized macrophage cell lines. For infections using the immortalized murine macrophage-like cell line RAW 264.7 (ATCC® TIB-71TM), cells are maintained without antibiotics in RPMI supplemented with 2 mM Glutamine, 25 mM HEPES [4-(2-hydroxyethyl)-1-piperazineethanesulfonic acid] and 5% (v/v) non-heat inactivated fetal bovine serum (Wisent Inc.). HEPES buffer is included in the tissue culture medium so that pH maintenance is relatively insensitive to fluctuations in atmospheric CO2. Consequently, the pH of the medium and organellar pH can be maintained in the presence (i.e., within an incubator) or absence (i.e., on the bench top or on the microscope) of constant CO2. Antibiotics are purposefully omitted to avoid introducing potentially confounding effects of antibiotic exposure on phagocytosed bacteria as macrophages will regularly consume antibiotic through constitutive pinocytosis (Bohdanowicz et al., 2013; Canton et al., 2016; Flannagan et al., 2016). RAW 264.7 macrophages are routinely grown in T25 vented tissue culture flasks at 37°C in the presence of 5% CO2 and humidity of ∼90%. When RAW cells reach ∼70% confluence in a T25 flask, macrophages are split by scraping as per the instructions provided by the ATCC for this cell line. To set up infection assays a 12-well tissue culture plate containing a single sterile 18 mm glass coverslip (Electron Microscopy Sciences, No. 1) in each well is used. Next, a uniform suspension of RAW macrophages is created by adding scraped RAW macrophages, at a density of ∼4.8 × 106 cells per 12 mL of RPMI with FBS. To each well, 1 mL of this suspension (∼4.0 × 105 cells) is added and the plate is incubated overnight (∼ 16 to 18 h) for infection the next day. The RAW cell doubling time is quite fast and so by the following day each well of a 12-well dish will contain RAW macrophages that are ∼55–60% confluent or ∼5.5 to 6.0 × 105 cells per cover glass. Scraping cells to passage them inevitably causes some cell death which accounts for the slightly reduced final number of cells.
2. Primary bone marrow-derived macrophages. In some instances, it may be necessary to perform infections in primary human or murine bone-marrow derived macrophages. Bone-marrow derived macrophages may be particularly useful as this will permit the use of knock-out mice which can allow for investigation into host factors that influence the ability of S. aureus to survive and/or replicate intracellularly. For the isolation of bone marrow-derived macrophages we follow standard procedures whereby the murine femur and tibia are flushed into a 50 mL conical tube with sterile 1× PBS and the bone marrow cells collected (Weischenfeldt and Porse, 2008; Davis, 2013). After centrifugation at 500 ×g the resulting bone-marrow cell pellet is re-suspended in 3 mL sterile ACK red cell lysis buffer (150 mM NH4Cl, 10 mM KHCO3, 0.1 mM EDTA, pH 7.4) for 2 min and then diluted 16-fold by addition of sterile 1× PBS. This diluted cell suspension is passed through a 70 μm nylon mesh cell strainer and then subject to centrifugation at 500 × g to collect the bone marrow cells. The resulting cell pellet is re-suspended 4 mL sterile serum-free RPMI and the density of viable cells is determined using trypan blue staining. Next, the cells are diluted in RPMI containing 10% (v/v) non-heat inactivated FBS supplemented with 1× pen-strep (Wisent) and recombinant murine M-CSF (10 ng/mL; PeproTech®) such that 1.0 × 106 cells are added per well of a 12-well tissue culture dish containing sterile cover glass as described above. The culture medium is replaced on day 2 and day 5 where on day 5 antibiotics are omitted. Macrophages are used for infection between day 7 and day 10 and are infected as described below for RAW macrophages at an MOI of 10 bacteria per macrophage.
3. Primary human macrophages. To derive primary human macrophages peripheral blood monocytes are isolated from healthy volunteers in accordance with protocols approved by the University of Western Ontario Research Ethics Board. Freshly isolated blood is over-laid on Lympholyte-poly cell separation medium (Cedarlane Laboratories) according to the manufacturer’s instructions. In brief, the suspension is centrifuged at 500 ×g for 30 min without centrifugal braking. The mononuclear band of cells is removed and transferred to a fresh 50 mL conical tube and topped up to a final volume of 50 mL with sterile 1× PBS. Mononuclear cells are again centrifuged at 500 × g for 10 min and the resulting cell pellet is re-suspended in serum-free RPMI. Next, the density of viable cells is determined by trypan blue staining and counting using a hemocytometer. The cells are diluted such that ∼5.0 × 105 cells in a 300 μL volume will be plated into each well of a 12-well tissue culture plate already containing sterile 18 mm glass coverslips. Care is taken to add only 300 μL directly onto each coverglass without causing the cell suspension to run all over the well. This is to ensure maximal recovery of monocytes which preferentially adhere to the glass surface. After 1 h incubation at 37°C in 5% CO2 incubator the plate containing adhered monocytes is washed twice with 2 mL sterile 1× PBS per wash. Finally, to each well containing adhered monocytes, 1 mL of RPMI supplemented with 10% (v/v) non-heat inactivated FBS, 1× penicillin-streptomycin solution, and either recombinant human M-CSF for M0 polarized macrophages or recombinant human GM-CSF for M1 polarized macrophages (each at 10 ng/mL, PeproTech®). After 5 days at 37°C in a humidified 5% CO2 incubator the cells are washed twice with 2 mL sterile 1× PBS and the medium replaced with the same growth medium, however, antibiotics are omitted. In addition, the medium to derive M1 polarized macrophages is supplemented recombinant human interferon-γ (10 ng/mL, PeproTech®) and 250 ng/mL lipopolysaccharide (Santa Cruz Biotechnology®). Alternatively, one can derive M2 polarized macrophages at this time by adding to the M-CSF containing medium interleukin-4 (10 ng/mL, PeproTech®). At day 7 to 10 the macrophages are ready to be used for phagocytosis of S. aureus which is performed as described below using an MOI of 10 bacteria per macrophage.
Infection Assays
To infect these cells 1 mL of the diluted suspension of GFP-expressing eFluorTM-670 labeled S. aureus (described above) is added to each well of a 12-well plate containing macrophages. To synchronize phagocytosis the plate is centrifuged immediately at 277 ×g for 2 min at room temperature and then incubated at 37°C in the presence of 5% CO2 for 30 min. We prefer to synchronize phagocytosis by centrifugation without exposing S. aureus or macrophages to the shock of 4°C to avoid untoward effects of cold exposure such as de-polymerization of macrophage microtubules (Breton and Brown, 1998; Lodish et al., 2000) as microtubules can contribute to phagocytosis (Allen and Aderem, 1996; Gilberti and Knecht, 2015). After 30 min the media containing bacteria is aspirated and replaced with warmed serum-free RPMI containing gentamicin (100 μg/mL) for 1 h. After incubation with gentamicin each well containing macrophages is washed twice with a 2-mL volume of sterile 1× PBS each time and then incubated further with warmed RPMI supplemented with 5% (v/v) FBS without gentamicin at 37°C in 5% CO2.
For infection of RAW 264.7 macrophages with GFP-expressing E. coli ML35 bacteria, the RAW cells were prepared as described above. Infections were also performed exactly as described for S. aureus, however, E. coli was added to RAW macrophages at an MOI of 100 because this strain is non-pathogenic.
Fluorescent Staining of Macrophages
In many instances, it is desirable to characterize the subcellular niche in which phagocytosed bacteria reside. Routinely, when investigating the interaction of Staphylococci with macrophages we employ a variety of fluorescence-based staining that is compatible with eFluorTM-670 labeling of S. aureus. Different stains and fluorescent markers are used for delineation of the cell membrane and subcellular compartments, as described below.
1. Plasmalemma staining. To simply delineate the plasmalemma of the macrophages and label extracellular cocci just prior to macrophage fixation, wheat germ agglutinin (WGA) conjugated with tetramethylrhodamine (TMR) is diluted in 1× PBS at a concentration of 1 μg/mL and incubated for 2 min at room temperature prior to rinsing twice with 1 mL of PBS. After rinsing, TMR-WGA stained cells are fixed with 4% (v/v) PFA for 20 min prior to rinsing with 1× PBS and fluorescence imaging.
2. Phagolysosome staining. To determine whether phagocytosed S. aureus reside within mature, acidic phagolysosomes a variety of procedures can be performed, however, we routinely perform LysotrackerTM staining and immunostaining of host cell lysosome-associated membrane protein-1 (LAMP-1).
2a. LysotrackerTM Staining. For LysotrackerTM staining macrophages already infected with S. aureus are incubated with 250 nM LysotrackerTM probe diluted in serum-free RPMI for a total of 5 to 7 min prior to washing away the probe. Macrophages are then imaged immediately while alive to avoid the introduction of artifacts through fixation and/or immunostaining. Live imaging of cells can be done with the cells bathed in either RPMI containing 25 mM HEPES or alternatively live cell imaging buffer (150 mM NaCl, 5 mM KCl, 1 mM MgCl2, 100 μm EGTA, 2 mM CaCl2, and 20 mM HEPES, pH 7.4) can be used. LysotrackerTM staining can be performed at any time during an infection, however, staining should always be performed immediately before imaging that specific time point.
2b. LAMP-1 Immunostaining. To analyze the cellular distribution of LAMP-1 with respect to the S. aureus- containing phagosome we routinely perform immunostaining of either murine or human macrophages to detect endogenous LAMP-1. Here, macrophages are first fixed with 4% (v/v) paraformaldehyde for 20 min at room temperature prior to permeabilization of PFA-fixed cells with 100% methanol pre-chilled to -20°C. While methanol can also be used in some instances for fixation, methanol is not compatible with GFP, and if used without PFA fixation first will distort the GFP chromophore and compromise fluorescence. After 3 min incubation at -20°C the fixed and permeabilized cells are washed once with 1× PBS and then incubated for at least 3 h in 1 mL of human plasma from primary macrophage isolations that is kept frozen. Due to the presence of protein A on the surface of S. aureus, human plasma is used to block non-specific protein A binding of anti-LAMP-1 or secondary antibodies as it contains milligram quantities of IgG per mL. If the bacterium under investigation does not express protein A or another IgG-binding protein, blocking can be done in 5% (w/v) skim milk reconstituted in 1× PBS instead of human plasma. After blocking, detection of murine LAMP-1 is through rat-anti-mouse LAMP-1 antibody (clone 1D4B) whereas detection of human LAMP-1 requires use of mouse-anti-human LAMP-1 antibody (clone H4A3). Anti-LAMP-1 antibodies are purchased as supernatant from the Developmental Studies Hybridoma Bank (DSHB) and H4A3 was deposited to the DSHB by J. T. August and J. E. K. Hildreth and 1D4B by J. T. August. To stain LAMP-1 the appropriate antibody clone is diluted 1:100 in PBS containing 50% (v/v) human plasma and incubated for 1 h at room temperature. After serially washing the cells, primary antibody binding is detected by 45 min incubation with secondary goat anti-rat or goat anti-mouse CyTM3-conjugated antibody (Jackson Immunoresearch) diluted in PBS containing 50% (v/v) human plasma as appropriate. CyTM3-conjugated secondary antibodies are employed as the spectra of this dye is compatible with GFP (expressed by S. aureus) and far-red FluorTM-670 (labeling un-replicated bacteria).
Fluorescence Microscopy
Wide-field fluorescence microscopy is performed on a Leica DMI6000 B inverted microscope equipped with 40× (NA 1.3), 63× (NA 1.4), and 100× (NA 1.4) oil immersion PL-Apo objectives, a Leica 100-W Hg high-pressure light source, and a Photometrics Evolve 512 Delta EM-CCD camera. The EM-CCD camera provides superior sensitivity for photon detection. This microscope is also outfitted with an objective warmer and an enclosed heated stage insert with CO2 perfusion (Live Cell Instruments). This microscope is equipped with the ET-Sedat-quad 89000 series excitation and emission filter set (Chroma Technologies) for DAPI (4′,6-diamidino-2-phenylindole), GFP/FITC, Cy3/Alexa 555, and Cy5/Alexa 647 imaging. For live-cell imaging, coverslips carrying macrophages were placed in a magnetic imaging chamber and bathed in SF-RPMI buffered with Na bicarbonate and 25 mM HEPES. Live-cell imaging employed a heated stage and objective warmer set to 37°C and in the presence of 5% CO2 as necessary.
Images were acquired as z-series using the Leica LAS X microscope software package. In FIJI (Schindelin et al., 2012) the raw imaging data for a given series were Z projected under the stacks feature where the projected image is the sum of three consecutive slices. After the Z projection tool is used, images are split into individual channels, contrast enhanced and cropped prior to image overlaying/merging. Importantly, the gamma is never altered, and fluorescence intensity measurements, if made, are only done on raw data with background subtraction.
Ethics Statement
Blood was obtained, with informed and written permission, only from healthy adult volunteers, in compliance with protocol 109059 approved by the Office of Research Ethics at the University of Western Ontario. All animal protocols (protocol 2017-028) were reviewed and approved by the University of Western Ontario Animal Use Subcommittee, a subcommittee of the University Council on Animal Care. Protocols adhered to guidelines set out by the Canadian Council on Animal Care.
Results
Detection of Replicating Intracellular Bacteria
The phagocytic and microbicidal capacity of macrophages can be heterogeneous complicating the detection of intracellular replicating S. aureus. The need to accurately identify intracellularly proliferating bacteria underpins our ability to understand host-pathogen interactions at the cellular level. Through our investigation of the interaction between macrophages and methicillin-resistant S. aureus strain USA300 we have realized that accurately identifying replicating bacteria within the host cell can be complicated. This is, in part, due to heterogeneity between macrophages in terms of the number of bacteria that might reside in any given phagocyte despite the use of a single multiplicity of infection. To illustrate this, phagocytosis assays of GFP-expressing S. aureus strain USA300 that is co-labeled with the proliferation dye eFluorTM-670 were performed using murine bone marrow derived macrophages (BMDMs) (Figure 2). Here, at 12 h post-infection it is evident that every BMDM can ingest S. aureus to some extent, however, there is significant variation in the number of GFP-positive cocci that reside in each macrophage. In the absence of the proliferation dye, it might be concluded that some of the macrophages in the top panel contain replicating S. aureus because they contain many more cocci at 12 h post-infection as compared to the surrounding phagocytes (Figure 2, top panels). However, through co-labeling GFP-expressing S. aureus with eFluorTM-670 it is evident that these intracellular cocci have not replicated as they remain proliferation dye positive. In contrast, at the same time point there exist instances whereby macrophages contain intracellular GFP-positive S. aureus cocci that are devoid of the proliferation dye eFluorTM-670 indicating these bacteria grew within the macrophage (Figure 2, bottom panels). Importantly, the heterogeneity with which macrophages ingest S. aureus or continue to ingest cocci off the cover glass occurs not only in primary murine macrophages but is also observed when phagocytosis assays are performed using primary human M-CSF-derived macrophages (Figure 3).
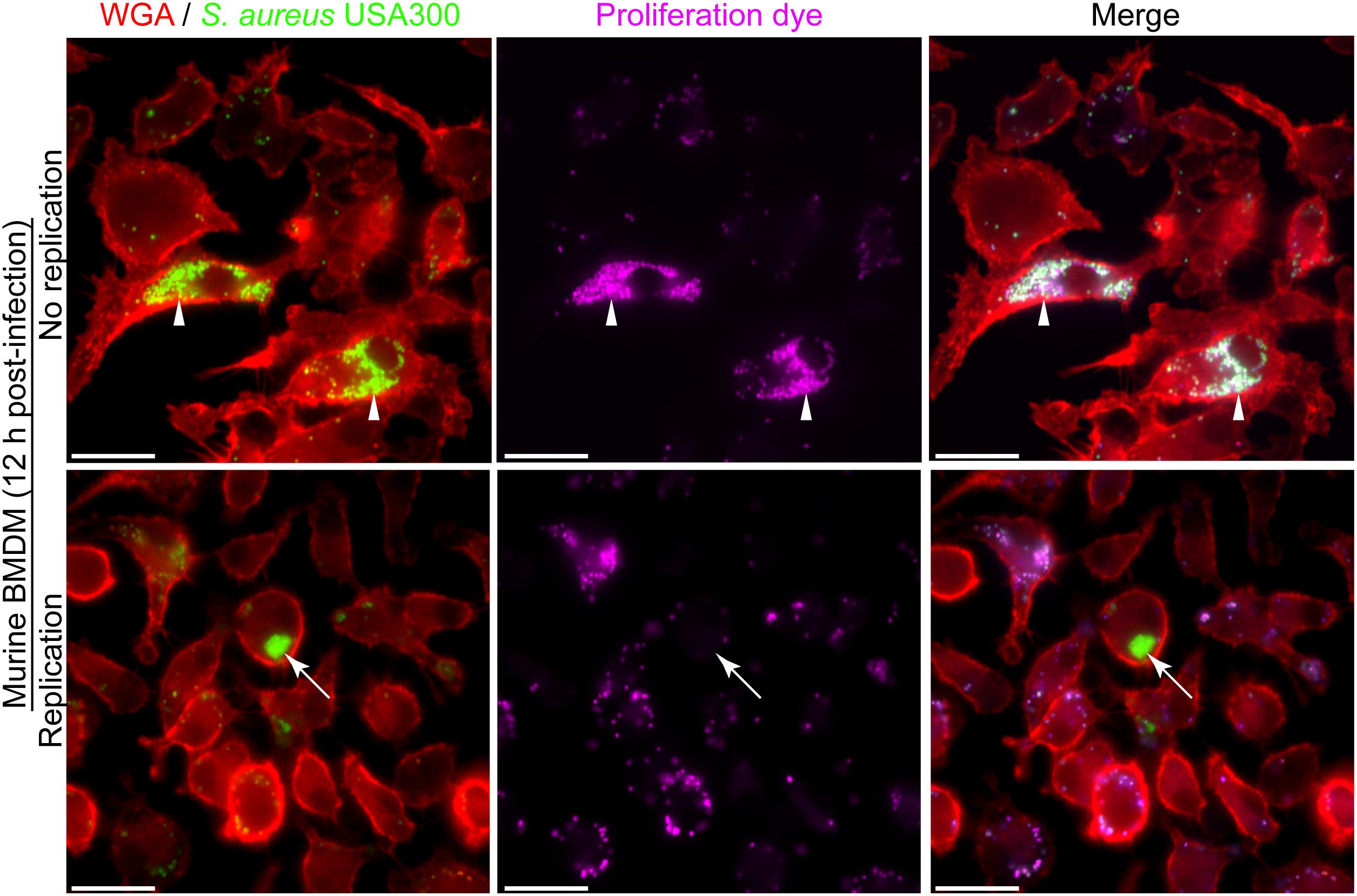
Figure 2. The presence of large populations of intracellular bacteria does not accurately demonstrate the presence of replicating bacteria. The representative images are of TMR-WGA labeled murine bone-marrow-derived macrophages (BMDM, in red) that were incubated with GFP-expressing S. aureus USA300 (in green) labeled with eFluorTM-670 (pseudo-colored pink) at an MOI of 10. Images were acquired at 12 h post-infection. Arrow heads in the top panels indicate macrophages containing many S. aureus cocci, however, these bacteria are eFluorTM-670 positive and therefore have not replicated. White arrows point to GFP-positive yet eFluorTM-670 negative S. aureus indicating these bacteria have indeed replicated intracellularly. These data represent observations made from more than six independent experiments. Scale bar is ∼10 μm.
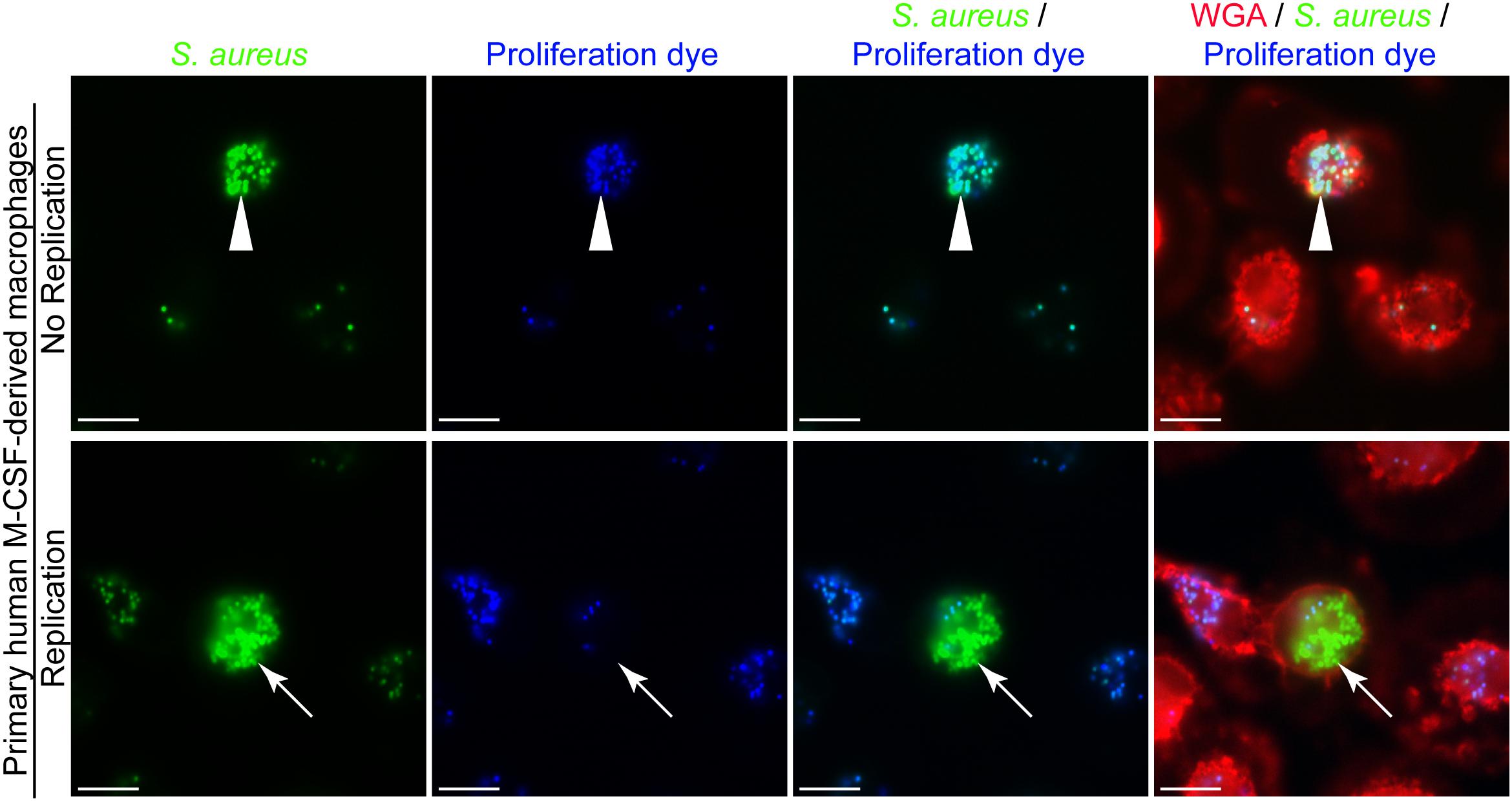
Figure 3. Heterogeneity in the number of intracellular bacteria occurs in primary human M-CSF-derived macrophages. Primary human M-CSF-derived macrophages derived from an otherwise healthy donor were infected with S. aureus after 7 days of differentiation. Macrophages are marked with TMR-WGA (in red) while S. aureus USA300 is expressing GFP (in green). At the outset of the infection bacteria were co-labeled with eFluorTM-670 proliferation dye (pseudo-colored blue). These representative images were taken at 12 h post-infection and represent common events from multiple (>6) independent experiments. The white arrow head points to a primary phagocyte containing many cocci while the surrounding cells contain few. These bacteria are also marked with eFluorTM-670 proliferation dye at this time-point. White arrows point to a phagocyte that contains many GFP-positive cocci that are eFluorTM-negative indicating these bacteria have replicated. In contrast the surrounding cells contain few GFP-positive cocci that are also eFluorTM-670-positive. Scale bar is ∼10 μm.
Loss of Proliferation Dye Fluorescence Requires Bacterial Replication
Again, the proliferation assay we describe functions akin to lymphocyte proliferation assays using carboxyfluorescein diacetate succinimidyl ester (CFSE) where labeled lymphocytes initially appear intensely fluorescent, however, through successive cell division become increasingly weaker for the CFSE signal (Quah et al., 2007). Instead of CFSE we have employed eFluorTM-670 and conjugate this fluorescent probe to S. aureus bacteria also expressing GFP. To demonstrate the utility of the eFluorTM-670 dye for the detection of proliferating bacteria we performed a series of experiments to demonstrate that only living bacteria that can undergo cell division become proliferation dye negative over time. To this end we labeled live GFP-expressing S. aureus USA300 with eFluorTM-670 exactly as described in materials and methods. These bacteria were then either left untreated or subject to paraformaldehyde fixation to inactivate the bacteria and render them unable to proliferate. Next, these live and inactivated bacteria that were positive for both GFP and eFluorTM-670 were inoculated into TSB and cultured for 8 h at 37°C. At the start of this experiment samples of each culture were taken and imaged by fluorescence microscopy which revealed that at the outset all GFP-positive bacteria were indeed positive for far-red eFluorTM-670 fluorescence (Figure 4A). Importantly, by 8 h post-inoculation GFP-positive yet eFluorTM-670 negative bacteria were only evident in the “active” culture where S. aureus could undergo cell division (Figure 4A). These observations were entirely consistent with the notion that S. aureus labeled with eFluorTM-670 could only become negative for the proliferation dye through bacterial replication. From this analysis, it is evident that loss of eFluorTM-670 fluorescence occurs through division of S. aureus cocci.
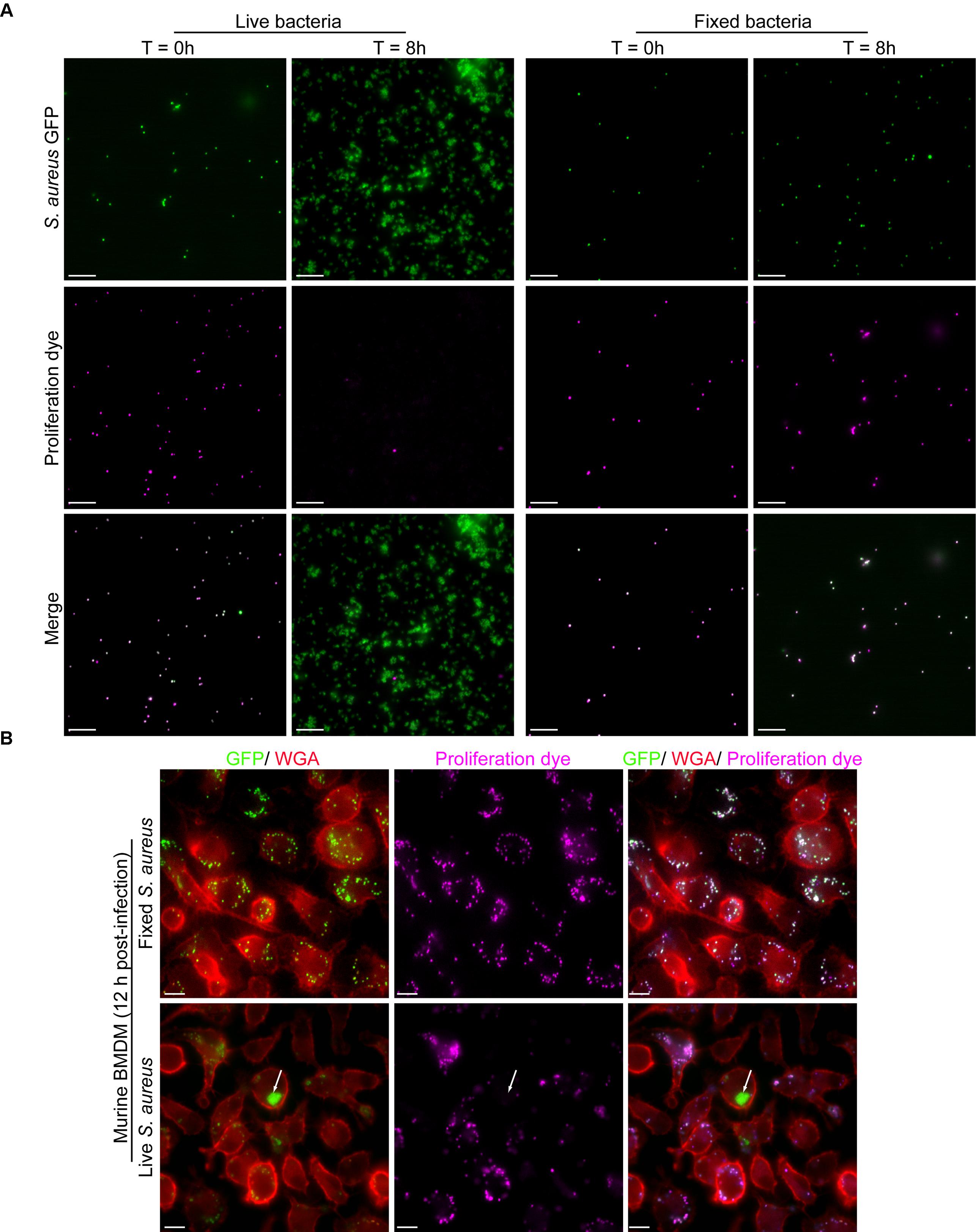
Figure 4. Proliferation dye can accurately identify bacteria that have grown in vitro and within murine bone marrow-derived macrophages. In (A) GFP-expressing S. aureus were co-labeled with eFluorTM-670 proliferation as described in Materials and Methods. In addition, some of these cells were inactivated by PFA fixation. After inoculation of TSB images of the live and fixed bacteria were acquired at time 0 and 8 h post-inoculation. Scale bar is ∼10 μm. In (B) murine bone marrow-derived macrophages (BMDM) were infected with live and fixed GFP-expressing S. aureus that were marked with eFluorTM-670 proliferation dye. Macrophages in red were labeled with TMR-WGA right before fixation. These representative images of macrophages containing fixed (top row) or live (bottom row) S. aureus were acquired at 12 h post-infection. In the top row, all GFP-positive cocci are eFluorTM-670 positive whereas in the bottom row at 12 post-infection GFP-positive yet proliferation dye negative bacteria can be detected (white arrow). These data represent observations from more than ten independent experiments. Scale bar is ∼10 μm.
To demonstrate that this also transpires intracellularly we performed macrophage infections using primary murine bone marrow-derived macrophages. Here macrophages were incubated with live GFP-expressing S. aureus USA300 or fixed GFP-positive S. aureus that were also eFluorTM-670-positive. Here at 12 h post-infection only eFluor-negative bacteria can be detected for live S. aureus whereas all fixed bacteria, at this time point, maintained the proliferation dye (Figure 4B). Taken together these data reveal that in the phagolysosome of macrophages the eFluorTM-670 proliferation dye is stable and that only replicating cells can become negative for the dye long after infection.
Fluorescence-Based Proliferation Analysis Is Compatible With Additional Phagosomal Fluorescent Probes
To demonstrate the utility of the eFluorTM-670 dye for the identification of S. aureus growing within macrophages in the context of additional cellular probes we first performed macrophage infections in parallel with LAMP-1 immunofluorescence. Previously we have demonstrated that S. aureus fails to replicate immediately after phagocytosis and instead commences proliferating hours after infection (Flannagan et al., 2016). Consistent with these earlier observations, we find again that at 1.5 h post-infection, all S. aureus cocci are GFP and eFluorTM-670 positive indicating they have not proliferated (Figure 5, top panels). In contrast, by 12 h post-infection GFP-positive cocci that are eFluorTM-670 negative are readily detectable and easily distinguished from those bacteria that remain eFluorTM-670 positive (Figure 5, bottom panels). In addition, through LAMP-1 immunostaining of these samples it is evident that S. aureus, even when replicating (i.e., GFP-positive yet eFluorTM-670 negative), can be confined to LAMP-1 positive compartments (Figure 5).
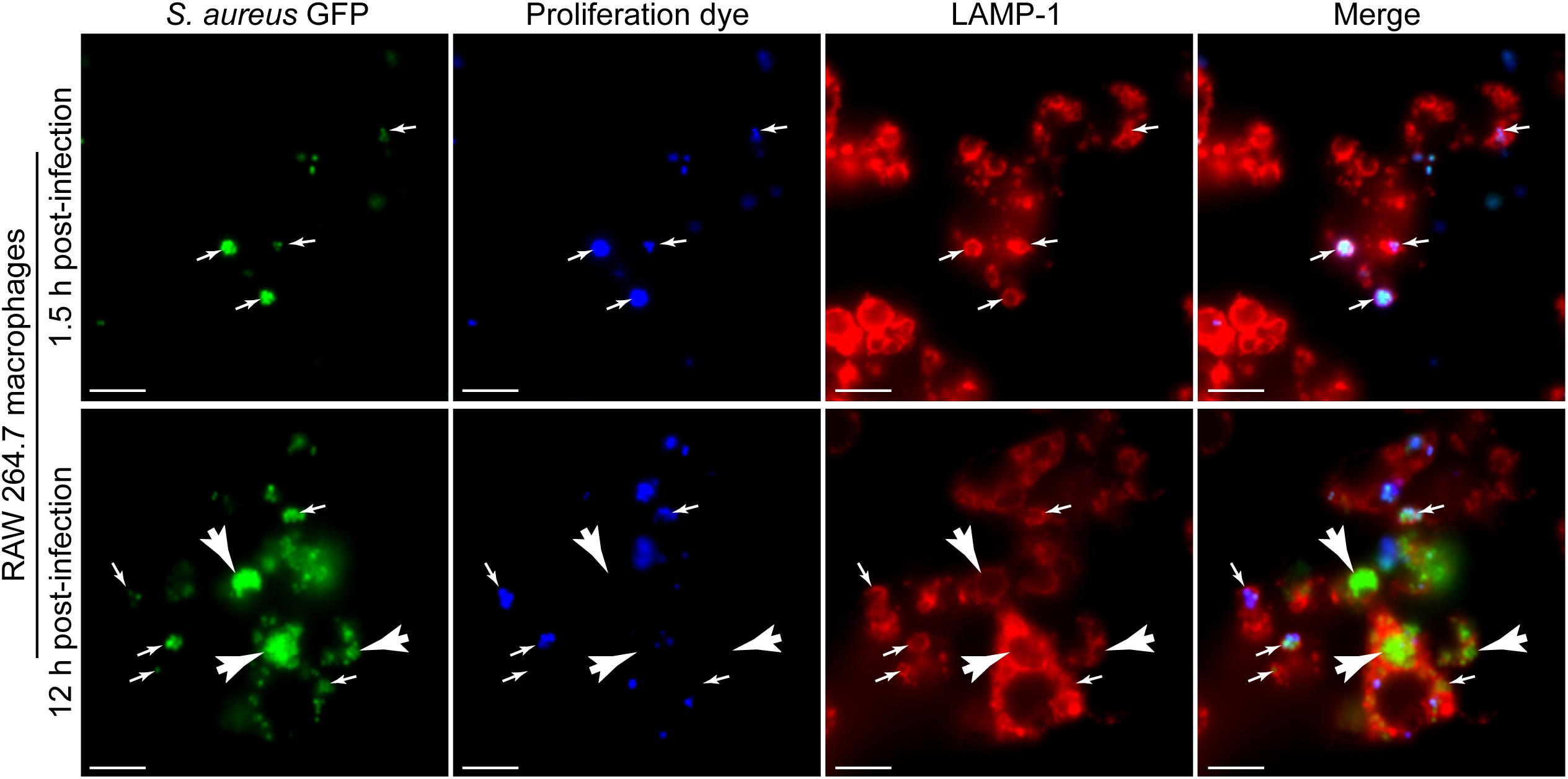
Figure 5. Fluorescence proliferation dye can be used to identify bacteria replicating in lysosome associated membrane protein-1 (LAMP-1) positive compartments. The representative images depict RAW 264.7 macrophages infected with GFP-expressing S. aureus USA300 (in green) co-labeled with eFluorTM-670 (in blue) that are also immune-stained for endogenous LAMP-1 protein (in red). Images are of cells at 1.5 and 12 h post-infection and are representative of data normally obtained from greater than ten independent experiments. Small white arrows point to GFP-positive cocci that are eFluorTM-670 positive and that are demarcated by LAMP-1 fluorescence. White arrow heads point to GFP-positive yet proliferation dye negative S. aureus that are LAMP-1 positive. Scale bar equals 10 μm. Note: The image series shown for the 12 h time point is presented elsewhere in an alternate form (Copyright © American Society for Microbiology; Flannagan et al., 2018a).
To further investigate the niche in which S. aureus is replicating it is desirable to employ additional fluorescent probes such as LysotrackerTM Red DND-99. With these additional probes, important information about the interaction of the replicating pathogen with the host cell can be gained. To demonstrate the compatibility of LysotrackerTM staining with the eFluorTM-670 fluorescence-based proliferation assay we performed macrophage infections where at 12 h post-infection phagocytes were also stained with LysotrackerTM staining (Figure 6A). From this procedure it is evident that GFP-expressing S. aureus that are eFluor-negative can be detected within LysotrackerTM-positive vacuoles (Figure 6A). Here we perform LysotrackerTM staining only on live cells and image immediately after staining. It is important to consider how the LysotrackerTM probe is being used and how stained cells are processed for imaging. This is done to limit artifacts that can be introduced by fixation and or permeabilization. Indeed, LysotrackerTM is not a fixable probe and its retention and cellular distribution is sensitive to fixation and/or permeabilization of cells after staining. To demonstrate this, we performed image analysis of LysotrackerTM stained RAW macrophages that were not manipulated, were pre-treated with the weak base ammonium chloride, or were fixed and or permeabilized after lysotracker staining (Figure 6B). As expected when stained immediately prior to imaging while alive it is evident that RAW macrophages possess a profusion of acidic compartments that robustly accumulate the acidotropic dye (Figure 6B). In contrast, when macrophages are pre-treated with 40 mM NH4Cl for 10 min prior to LysotrackerTM staining and live cell imaging, it is evident that accumulation of the probe is severely compromised (Figure 6B). When 20 min fixation with paraformaldehyde is performed immediately after LysotrackerTM staining and right before imaging it is evident that the cellular distribution of LysotrackerTM differs from living cells despite there being retention of fluorescence. For instance, in live cells LysotrackerTM prominently accumulates within large spacious vacuoles present with the macrophage cytoplasm, however, in fixed cells these vacuolar structures are devoid of the LysotrackerTM probe (Figure 6B). Moreover, when permeabilization is performed on LysotrackerTM stained cells as would be done to detect phagocytosed bacteria or host proteins through antibody staining, the LysotrackerTM probe is lost entirely from the cell. Taken together these data reveal that LysotrackerTM staining is compatible with the eFluorTM-670 fluorescent proliferation dye, however, care should be taken to avoid fixation and/or permeabilization.
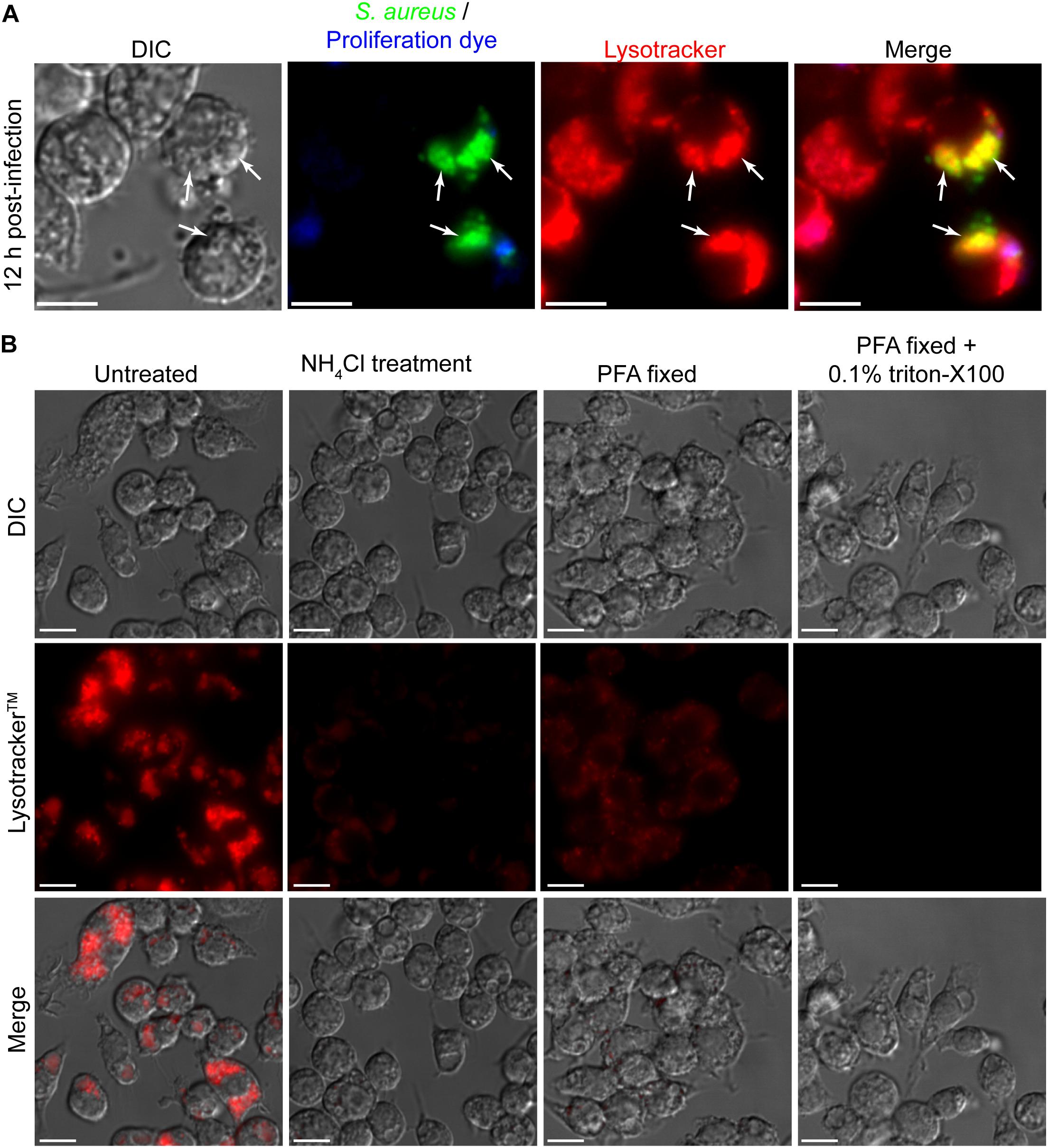
Figure 6. Cellular probes such as LysotrackerTM Red DND-99 are compatible with the fluorescent reporter of proliferation dye. In (A) RAW 264.7 macrophages containing live S. aureus were stained with LysotrackerTM and imaged by live cell fluorescence microscopy. At the outset of infection GFP-expressing bacteria were labeled with eFluorTM-670 and here at 12 h post-infection GFP-positive yet eFluorTM-670 negative bacteria can be detected (white arrows). These bacteria also co-localize with LysotrackerTM. Scale bar is ∼10 μm. In (B) RAW macrophages that were uninfected were stained with LysotrackerTM and imaged either live, after fixation, or after fixation and permeabilization. These representative images were acquired under the same acquisition parameters to allow for direct comparison of fluorescence intensities and represent observations made from more than six independent experiments. In one instance, live RAW cells were first treated with 40 mM of the weak base NH4Cl prior to lysotracker staining. Scale bars equal ∼10 μm.
Fluorescence-Based Proliferation Assays Can Be Employed to Analyze the Growth of Gram-Negative Bacteria
Based on the amine reactivity of the eFluorTM-670 dye we predicted that this probe could also be used to mark and identify replicating Gram-negative bacteria. To test this notion, we utilized select Gram-negative bacteria including Y. pseudotuberculosis, C. rodentium, and E. coli DH5a and tested whether we could detect the eFluorTM-670 dye in association with the bacteria by fluorescence microscopy. Initial attempts to label these bacteria following the protocol we described for S. aureus revealed that while the dye could indeed label some of the bacteria many bacterial cells remained unmarked (data not shown). Clearly the protocol that we employed for S. aureus was less efficient for these Gram-negative bacteria. To overcome this, we performed eFluorTM-670 labeling in sterile 1× PBS set at pH 8.0 as this buffer has been previously employed to react N-hydroxysuccinimidobiotin with Y. pseudotuberculosis bacteria (Sarantis et al., 2012). Using this buffer, it was evident that C. rodentium, Y. pseudotuberculosis, and E. coli DH5α could be labeled with eFluorTM-670 proliferation dye under the conditions employed here (Figure 7A). Interestingly, the extent to which each bacterial species labeled appeared to differ as some C. rodentium and primarily elongated E. coli DH5α cells appeared to remain refractory to the dye. In contrast, virtually every Y. pseudotuberculosis bacillus was marked with eFluorTM-670 (Figure 7A). Nevertheless, it was evident from these experiments that Gram-negative bacteria could in principle be labeled with the proliferation dye, however, optimization of this procedure would have to be performed for each genus, species and strain being analyzed.
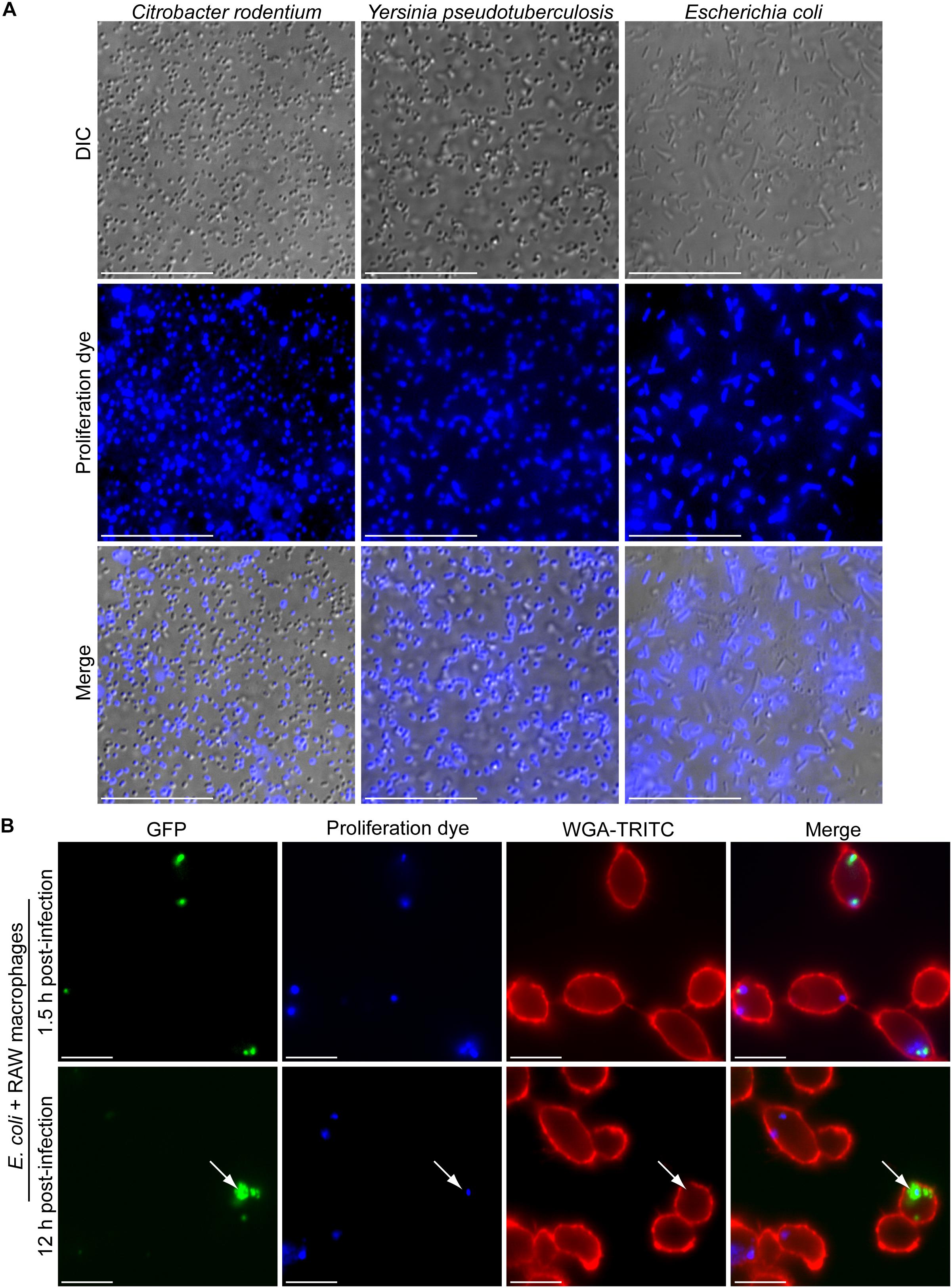
Figure 7. Gram-negative bacteria are amenable to fluorescence-based proliferation assays. In (A) the micrographs depict C. rodentium, Y. pseudotuberculosis, and E. coli DH5α bacteria that were incubated with eFluorTM-670 proliferation dye for a period of 10 min. In (B) the fluorescent micrographs depict intracellular E. coli ML35 expressing GFP (in green) that are co-labeled with the eFluorTM-670 (in blue). At 1.5 h post-infection (top row) and 12 h post-infection (bottom row) eFluorTM-670 positive bacteria can be detected. The white arrow points to a rare instance where GFP-positive yet eFluorTM-670-negative E. coli could be detected within RAW macrophages marked with TRITC-WGA (in red). These micrographs are representative of two independent experiments. Scale bars are ∼10 μm.
Next, we tested whether we could detect eFluorTM-670 dye on Gram-negative bacteria having been phagocytosed by macrophages and whether the loss of the dye from the bacteria could inform us on whether the bacteria had replicated. To this end we employed E. coli ML35 carrying the GFP expression vector pFPV25.1 and performed phagocytosis assays using RAW macrophages after labeling the bacteria with eFluorTM-670 as described above. E. coli ML35 has previously been shown to label with eFluorTM-670 proliferation dye and has been used in a heat-inactivated form for phagocytosis assays (Yin et al., 2016). Using live E. coli ML35 it was evident that at 1.5 h post-infection the E. coli bacteria could be detected within RAW macrophages and were GFP and eFluorTM-670 positive (Figure 7B). Interestingly, at 12 h post-infection many eFluorTM-670-positive E. coli appeared GFP-negative or were poorly GFP-fluorescent, consistent with intracellular killing of these bacteria. Despite this, some instances where GFP-bright E. coli that were now devoid of eFluorTM-670 fluorescence could be observed indicating these bacteria have replicated (Figure 7B). In support of this, these eFluorTM-negative E. coli, were also found as GFP-positive foci within macrophages, indicating they represent a nidus of replicating bacteria.
Discussion
Here we present a fluorescence-based method for the accurate identification of S. aureus bacteria that can replicate within host cells. Moreover, because this assay is compatible with additional cellular probes (i.e., LysotrackerTM and LAMP-1 immunofluorescence) it is possible to identify and characterize the subcellular niches in which S. aureus proliferates. To date, we have employed this assay to characterize the replication of S. aureus and S. lugdunensis within macrophages (Flannagan et al., 2016, 2018a,b), however, this assay should be easily applied to the study of many bacterial pathogens including Gram-positive and Gram-negative bacteria. In support of this we have demonstrated that at least some Gram-negative bacteria can be labeled and that proliferation assays can be performed whilst E. coli, for example, is inside RAW macrophages. The utility of this dye for Gram-negative bacteria is perhaps not surprising considering the fact that eFluorTM-670 reacts with primary amines on the bacterial cell surface and N-hydroxysuccinimide esters, which also react with primary amines, have been employed to chemically modify the cell surface of Gram-negative bacteria such as Y. pseudotuberculosis and Burkholderia cenocepacia (Flannagan et al., 2012; Sarantis et al., 2012). Nevertheless, optimization for labeling distinct bacterial species and/or strains will be necessary.
The fluorescent proliferation assay presented here employed GFP-expressing S. aureus, however, from our use of additional probes (i.e., LysotrackerTM Red DND-99 and CyTM3 fluorescence) it should be evident that the spectra of the eFluorTM-670 probe is compatible with both red and green fluorophores. With this versatility, experiments can be performed with bacteria that express red fluorescent proteins such as mCherry or mRFP and will still be compatible with cellular probes with spectra comparable to GFP/FITC (e.g., FITC-dextran). In addition, the images presented here were acquired by wide-field fluorescence microscopy with an EM-CCD camera meaning this analysis does not require the use of confocal microscopy. Nevertheless, consideration must be given to the use of appropriate excitation and emission filter sets and the excitation and emission spectra of the probes being used.
The proliferation assay has been a powerful tool that has enabled the characterization of intracellular Staphylococci (Flannagan et al., 2016, 2018a,b), however, it is important to note that there are limitations. For instance, we have found that this proliferation analysis is best suited for fluorescence microscopy where individual cells can be visualized and analyzed. Attempts to accurately assess bacterial proliferation by flow cytometry have failed. This is because macrophages can contain multiple phagosomes where the bacteria may or may not be replicating. For instance, a macrophage having ingested several cocci which reside in three different phagosomes may at 12 h post-infection have bacteria replicating in only one of those phagosomes. If analyzed by flow cytometry this cell would be detected as GFP and eFluorTM-positive and the observation that one phagosome was permissive to bacterial growth would be lost. Furthermore, sorting cells based on GFP intensity (i.e., GFP bright cells at 12 h would contain replicating bacteria) may erroneously exaggerate the extent to which the bacteria have replicated. This is illustrated in Figure 2 which was why we began to employ this assay using the eFluorTM-670 dye. Regardless of these limitations we believe this assay is a powerful tool that allows for the identification replicating bacteria and, when used in parallel with other cellular probes allows for the identification and characterization of the subcellular niche in which bacterial replication occurs.
In summary, the proliferation assay presented here is a useful tool that enables accurate identification of intracellularly growing bacteria. Moreover, through additional cellular probes, important information pertaining to the intracellular niche in which bacteria replicate can be obtained. Despite this, caution should be exercised to ensure that probes such as LysotrackerTM are used appropriately.
Author Contributions
All authors contributed significantly to this work. RF conducted each experiment, analyzed the data, and prepared the manuscript. DH analyzed the data and edited the manuscript.
Funding
Funding for this work comes from a Canadian Institutes of Health Research Project Grant awarded to DH and from a Cystic Fibrosis Canada research grant awarded to RF and DH.
Conflict of Interest Statement
The authors declare that the research was conducted in the absence of any commercial or financial relationships that could be construed as a potential conflict of interest.
Acknowledgments
The authors wish to thank David W. Watson for carefully reviewing this manuscript.
References
Allen, L. A., and Aderem, A. (1996). Molecular definition of distinct cytoskeletal structures involved in complement- and Fc receptor-mediated phagocytosis in macrophages. J. Exp. Med. 184, 627–637. doi: 10.1084/jem.184.2.627
Bohdanowicz, M., Schlam, D., Hermansson, M., Rizzuti, D., Fairn, G. D., Ueyama, T., et al. (2013). Phosphatidic acid is required for the constitutive ruffling and macropinocytosis of phagocytes. Mol. Biol. Cell 24, 1700–1712. doi: 10.1091/mbc.E12-11-0789
Breton, S., and Brown, D. (1998). Cold-induced microtubule disruption and relocalization of membrane proteins in kidney epithelial cells. J. Am. Soc. Nephrol. 9, 155–166.
Canton, J., Schlam, D., Breuer, C., Gütschow, M., Glogauer, M., and Grinstein, S. (2016). Calcium-sensing receptors signal constitutive macropinocytosis and facilitate the uptake of NOD2 ligands in macrophages. Nat. Commun. 7:11284. doi: 10.1038/ncomms11284
Davis, B. K. (2013). Isolation, culture, and functional evaluation of bone marrow-derived macrophages. Methods Mol. Biol. 1031, 27–35. doi: 10.1007/978-1-62703-481-4-3
Edwards, A. M., and Massey, R. C. (2011). Invasion of human cells by a bacterial pathogen. J. Vis. Exp. 49:2693. doi: 10.3791/2693
Fairn, G. D., and Grinstein, S. (2012). How nascent phagosomes mature to become phagolysosomes. Trends Immunol. 33, 397–405. doi: 10.1016/j.it.2012.03.003
Flannagan, R. S., Cosío, G., and Grinstein, S. (2009). Antimicrobial mechanisms of phagocytes and bacterial evasion strategies. Nat. Rev. Microbiol. 7, 355–366. doi: 10.1038/nrmicro2128
Flannagan, R. S., Heit, B., and Heinrichs, D. E. (2016). Intracellular replication of Staphylococcus aureus in mature phagolysosomes in macrophages precedes host cell death, and bacterial escape and dissemination. Cell. Microbiol. 18, 514–535. doi: 10.1111/cmi.12527
Flannagan, R. S., Jaumouillé, V., Huynh, K. K., Plumb, J. D., Downey, G. P., Valvano, M. A., et al. (2012). Burkholderia cenocepacia disrupts host cell actin cytoskeleton by inactivating Rac and Cdc42. Cell. Microbiol. 14, 239–254. doi: 10.1111/j.1462-5822.2011.01715.x
Flannagan, R. S., Kuiack, R. C., McGavin, M. J., and Heinrichs, D. E. (2018a). Staphylococcus aureus uses the GraXRS regulatory system to sense and adapt to the acidified phagolysosome in macrophages. mBio 9:e01143-18. doi: 10.1128/mBio.01143-18
Flannagan, R. S., Watson, D. W., Surewaard, B. G. J., Kubes, P., and Heinrichs, D. E. (2018b). The surreptitious survival of the emerging pathogen Staphylococcus lugdunensis within macrophages as an immune evasion strategy. Cell. Microbiol. 20:e12869. doi: 10.1111/cmi.12869
Flannagan, R. S., Heit, B., and Heinrichs, D. E. (2015). Antimicrobial mechanisms of macrophages and the immune evasion strategies of Staphylococcus aureus. Pathogens 4, 826–868. doi: 10.3390/pathogens4040826
Gilberti, R. M., and Knecht, D. A. (2015). Macrophages phagocytose nonopsonized silica particles using a unique microtubule-dependent pathway. Mol. Biol. Cell. 26, 518–529. doi: 10.1091/mbc.E14-08-1301
Gries, C. M., Sadykov, M. R., Bulock, L. L., Chaudhari, S. S., Thomas, V. C., Bose, J. L., et al. (2016). Potassium uptake modulates Staphylococcus aureus metabolism. mSphere 1:e0125-16. doi: 10.1128/mSphere.00125-16
Jubrail, J., Morris, P., Bewley, M. A., Stoneham, S., Johnston, S. A., Foster, S. J., et al. (2015). Inability to sustain intraphagolysosomal killing of Staphylococcus aureus predisposes to bacterial persistence in macrophages. Cell. Microbiol. 18, 80–96. doi: 10.1111/cmi.12485
Lodish, H., Berk, A., Zipursky, S. L., Matsudaira, P., Baltimore, D., and Darnell, J. (2000). Molecular Cell Biology, 4th Edn. Available at: https://www.ncbi.nlm.nih.gov/books/NBK21522/
Portnoy, D. A., Auerbuch, V., and Glomski, I. J. (2002). The cell biology of Listeria monocytogenes infection: the intersection of bacterial pathogenesis and cell-mediated immunity. J. Cell Biol. 158, 409–414. doi: 10.1083/jcb.200205009
Portnoy, D. A., Jacks, P. S., and Hinrichs, D. J. (1988). Role of hemolysin for the intracellular growth of Listeria monocytogenes. J. Exp. Med. 167, 1459–1471. doi: 10.1084/jem.167.4.1459
Quah, B. J. C., Warren, H. S., and Parish, C. R. (2007). Monitoring lymphocyte proliferation in vitro and in vivo with the intracellular fluorescent dye carboxyfluorescein diacetate succinimidyl ester. Nat. Protoc. 2, 2049–2056. doi: 10.1038/nprot.2007.296
Sarantis, H., Balkin, D. M., De Camilli, P., Isberg, R. R., Brumell, J. H., and Grinstein, S. (2012). Yersinia entry into host cells requires Rab5-dependent dephosphorylation of PI(4,5)P 2 and membrane scission. Cell Host Microbe 11, 117–128. doi: 10.1016/j.chom.2012.01.010
Sarantis, H., and Grinstein, S. (2012). Subversion of phagocytosis for pathogen survival. Cell Host Microbe 12, 419–431. doi: 10.1016/j.chom.2012.09.001
Sayedyahossein, S., Xu, S. X., Rudkouskaya, A., McGavin, M. J., McCormick, J. K., and Dagnino, L. (2015). Staphylococcus aureus keratinocyte invasion is mediated by integrin-linked kinase and Rac1. FASEB J. 29, 711–723. doi: 10.1096/fj.14-262774
Schindelin, J., Arganda-Carreras, I., Frise, E., Kaynig, V., Longair, M., Pietzsch, T., et al. (2012). Fiji: an open-source platform for biological-image analysis. Nat. Methods 9, 676–682. doi: 10.1038/nmeth.2019
Tranchemontagne, Z. R., Camire, R. B., O’Donnell, V. J., Baugh, J., and Burkholder, K. M. (2015). Staphylococcus aureus strain USA300 perturbs acquisition of lysosomal enzymes and requires phagosomal acidification for survival inside macrophages. Infect. Immun. 84, 241–253. doi: 10.1128/IAI.00704-15
Underhill, D., and Ozinsky, A. (2002). Phagocytosis of microbes: complexity in action. Annu. Rev. Immunol. 20, 825–852. doi: 10.1146/annurev.immunol.20.103001.114744
Valdivia, R. H., and Falkow, S. (1996). Bacterial genetics by flow cytometry: rapid isolation of Salmonella typhimurium acid-inducible promoters by differential fluorescence induction. Mol. Microbiol. 22, 367–378. doi: 10.1046/j.1365-2958.1996.00120.x
VanCleave, T. T., Pulsifer, A. R., Connor, M. G., Warawa, J. M., and Lawrenz, M. B. (2017). Impact of gentamicin concentration and exposure time on intracellular Yersinia pestis. Front. Cell. Infect. Microbiol. 7:505. doi: 10.3389/fcimb.2017.00505
Weischenfeldt, J., and Porse, B. (2008). Bone marrow-derived macrophages (BMM): isolation and applications. Cold Spring Harb. Protoc. 2008:pdb.prot5080. doi: 10.1101/pdb.prot5080
Keywords: phagocytosis, Staphylococcus, phagolysosome, fluorescence, microscopy, macrophage
Citation: Flannagan RS and Heinrichs DE (2018) A Fluorescence Based-Proliferation Assay for the Identification of Replicating Bacteria Within Host Cells. Front. Microbiol. 9:3084. doi: 10.3389/fmicb.2018.03084
Received: 10 October 2018; Accepted: 29 November 2018;
Published: 12 December 2018.
Edited by:
Sven Hammerschmidt, University of Greifswald, GermanyReviewed by:
Jorunn Pauline Cavanagh, University Hospital of North Norway, NorwaySuzan Rooijakkers, University Medical Center Utrecht, Netherlands
Copyright © 2018 Flannagan and Heinrichs. This is an open-access article distributed under the terms of the Creative Commons Attribution License (CC BY). The use, distribution or reproduction in other forums is permitted, provided the original author(s) and the copyright owner(s) are credited and that the original publication in this journal is cited, in accordance with accepted academic practice. No use, distribution or reproduction is permitted which does not comply with these terms.
*Correspondence: David E. Heinrichs, deh@uwo.ca