- 1Centre of New Technologies, University of Warsaw, Warsaw, Poland
- 2College of Inter-Faculty Individual Studies in Mathematics and Natural Sciences, University of Warsaw, Warsaw, Poland
- 3Department of Drug Chemistry, Faculty of Pharmacy with the Laboratory Medicine Division, Medical University of Warsaw, Warsaw, Poland
- 4Department of Bacterial Genetics, Institute of Microbiology, Faculty of Biology, University of Warsaw, Warsaw, Poland
- 5Unit of Bacterial Genome Plasticity, Department of Genomes and Genetics, Pasteur Institute, Paris, France
- 6Genomic Medicine, Medical University of Warsaw, Warsaw, Poland
The search for new, non-standard targets is currently a high priority in the design of new antibacterial compounds. Bacterial toxin–antitoxin systems (TAs) are genetic modules that encode a toxin protein that causes growth arrest by interfering with essential cellular processes, and a cognate antitoxin, which neutralizes the toxin activity. TAs have no human analogs, are highly abundant in bacterial genomes, and therefore represent attractive alternative targets for antimicrobial drugs. This study demonstrates how artificial activation of Escherichia coli mazEF and hipBA toxin–antitoxin systems using sequence-specific antisense peptide nucleic acid oligomers is an innovative antibacterial strategy. The growth arrest observed in E. coli resulted from the inhibition of translation of the antitoxins by the antisense oligomers. Furthermore, two other targets, related to the activities of mazEF and hipBA, were identified as promising sites of action for antibacterials. These results show that TAs are susceptible to sequence-specific antisense agents and provide a proof-of-concept for their further exploitation in antimicrobial strategies.
Introduction
Antibiotics are essential medicines used to prevent and treat otherwise incurable bacterial infections (Coates and Hu, 2007). However, the combination of widespread usage of antibiotics and bacterial evolution have decreased their efficacy, and stimulated the emergence of antibiotic-resistant bacteria (Fair and Tor, 2014). The traditional answer to this problem has been the introduction of new, improved versions of existing antibiotics that can kill the resistant bacterial mutants. However, simple molecular variants of commonly used antibiotics are becoming less effective in overcoming bacterial resistance mechanisms (Ventola, 2015a). The identification of new non-traditional targets for antimicrobials is therefore critical in combating infectious diseases caused by evolving pathogens (Ventola, 2015b).
Many bacteria contain toxin–antitoxin systems (TAs), usually composed of two genes that encode (i) a stable toxin that targets an essential cellular process, and (ii) a labile antitoxin that counteracts the activity of the toxin (Harms et al., 2018). These genetic modules are widely distributed in bacterial genomes, including clinical pathogens, but are not found in eukaryotes (Hayes and Van Melderen, 2011; Fernandez-Garcia et al., 2016; Lee and Lee, 2016). This makes them promising targets for the development of novel antibacterials (Kirkpatrick et al., 2016).
TAs have been divided into six classes, depending on the nature and the mode of action of the antitoxin molecule (Page and Peti, 2016). Type II TAs have been the most extensively characterized (Ma et al., 2015; Chan et al., 2016; Rocker and Meinhart, 2016). In this class, both the toxin and the antitoxin are proteins (Lobato-Márquez et al., 2016). Two particularly well studied type II TAs are mazEF (Hazan et al., 2004; Schuster et al., 2013; Tripathi et al., 2014) and hipBA (Schumacher et al., 2009; Germain et al., 2013; Kaspy et al., 2013). In the mazEF TAs family, the MazF toxin is an endoribonuclease, which cleaves mRNA at three-, five-, or seven-base recognition sequences in different bacteria resulting in translation inhibition (Yamaguchi and Inouye, 2009; Yamaguchi et al., 2012; Schifano et al., 2016). This cleavage occurs independently of both the ribosome and translation, and it is inhibited by the MazE antitoxin (Kędzierska and Hayes, 2016). In the hipBA TAs family, the HipA toxin targets the glutamyl-tRNA synthetase (GltX) involved in tRNA synthesis (Germain et al., 2013). The overproduction of HipA affects the integrity of E. coli cells and induces their lysis (Kędzierska and Hayes, 2016). The HipA toxin is neutralized by forming a complex with the HipB antitoxin (Wen et al., 2014). The mazEF and the hipBA loci are present in the chromosomes of many bacteria (Fu et al., 2009; Rothenbacher et al., 2012; Fernandez-Garcia et al., 2016), but their function has mainly been studied in E. coli (Hazan et al., 2004; Schumacher et al., 2009).
The artificial activation of TAs has potential as an antimicrobial strategy (Lioy et al., 2010; Shapiro, 2013; Verma et al., 2015; Kim et al., 2018). To test this concept we have used antisense oligonucleotides to try to alter TAs activity and arrest the growth of bacteria (Figure 1). Antisense oligonucleotides bind to nucleic acids in a sequence-specific manner by Watson-Crick base pairing, and can affect the function of targeted mRNAs and silence genes (Dias and Stein, 2002). Due to the rapid degradation of natural oligonucleotides in the intracellular environment, chemically modified oligonucleotides, such as peptide nucleic acids (PNA), have been successfully used as silencing agents (Rasmussen et al., 2007). PNA is a DNA analog with a pseudo-peptide backbone composed of repeating N-(2-aminoethyl) glycine units linked by amide bonds (Nielsen et al., 1991). PNA oligomers show enhanced nuclease and protease resistance, improved binding affinity with natural nucleic acids, and negligible toxicity to eukaryotic cells (Good et al., 2001). Typically, antibacterial PNAs have been targeted against mRNAs of essential genes (Good et al., 2001; Nekhotiaeva et al., 2004; Patenge et al., 2013) or functional ribosomal sites (Hatamoto et al., 2009; Górska et al., 2016; Kulik et al., 2017). Earlier problems with the delivery of PNAs to bacterial cells have been solved by conjugating them to various carriers, such as cell penetrating peptides (CPP) (Abushahba et al., 2016) or vitamin B12 (Równicki et al., 2017).
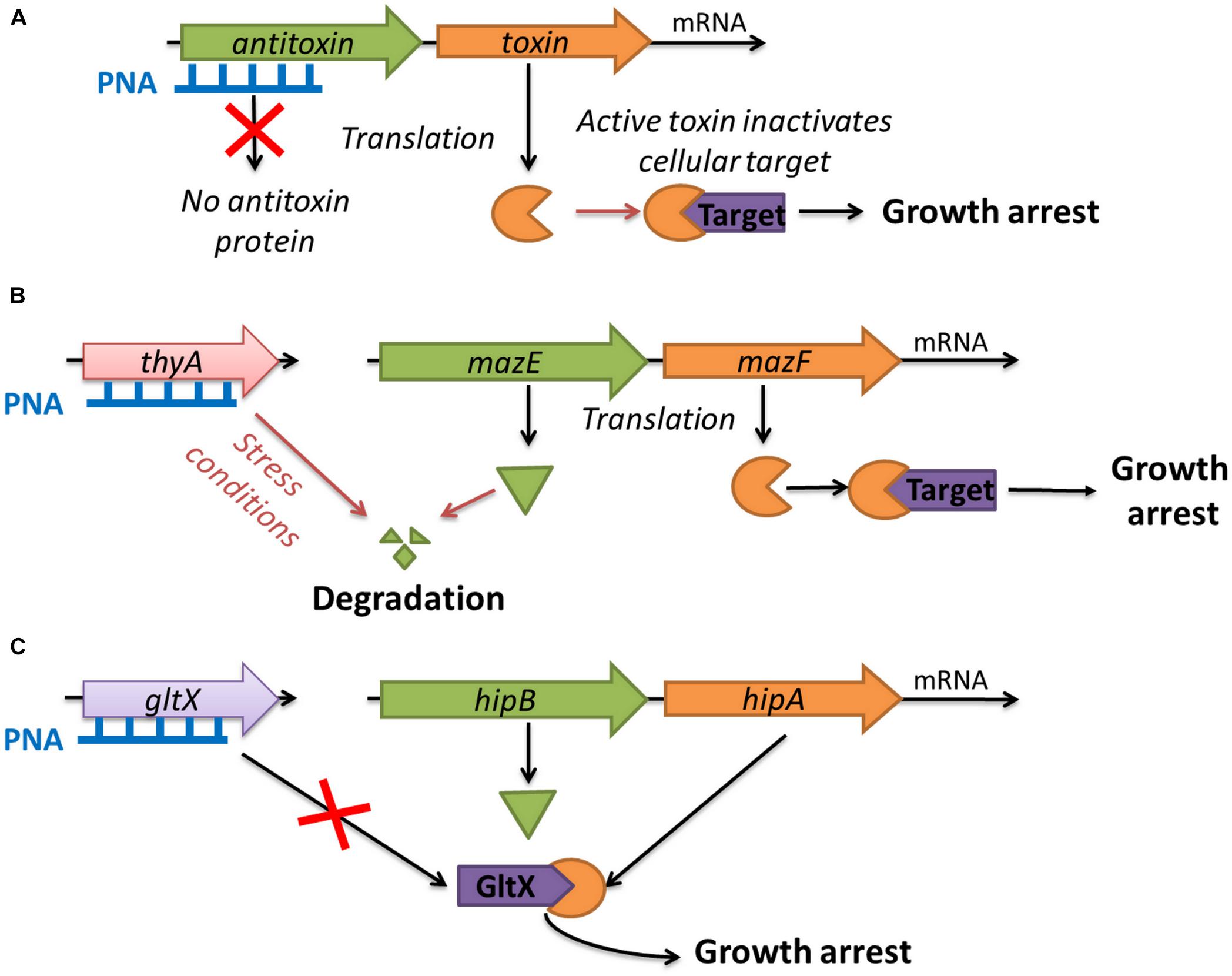
FIGURE 1. Strategies for using toxin–antitoxin systems as a target for antibacterials: (A) artificial activation of MazF and HipA toxins by antisense PNA targeted at the antitoxin genes; (B) indirect activation of MazF toxin by inducing thymine starvation through silencing of the thyA gene; (C) blocking the expression of the gltX gene which mimics the action of the HipA toxin.
In this study, we have investigated three innovative strategies related to the use of TAs and associated proteins as targets for antibacterial molecules (Figure 1). To the best of our knowledge, such approaches have not been reported so far. The first strategy was the artificial activation of the E. coli MazF and HipA toxins by using antisense PNA oligomers to inhibit the translation of the corresponding antitoxins (MazE and HipB, respectively, Figure 1A). The second strategy involved the indirect activation of the MazF toxin by inducing thymine starvation. This was achieved by silencing the thyA gene encoding thymidylate synthase, an enzyme involved in folic acid metabolism, which has been shown to interfere with mazEF-mediated growth inhibition (Figure 1B) (Engelberg-Kulka et al., 2005). The third strategy was to mimic the action of the HipA toxin by silencing the gltX gene encoding its cellular target glutamyl-tRNA synthase (Figure 1C).
To test these novel strategies, we first designed and synthesized antisense PNAs and evaluated their antimicrobial effectiveness by determining the minimal inhibitory concentration (MIC) for three strains of E. coli: a K-12 wild-type strain, the pathogenic strain O157:H7 (Łoś et al., 2008) and the extended spectrum β-lactamase-producing strain WR3551/98 (Baraniak, 2002) (Supplementary Table 1). Second, we examined the decay of the target mRNAs after treatment with different PNA concentrations, using reverse transcription real-time quantitative PCR (RT-qPCR). Third, we checked for any synergistic interactions between the mazEF-targeted PNAs and selected antibiotics (trimethoprim and sulfamethoxazole) by determining the fractional inhibitory concentration (FIC). Finally, we assessed the cytotoxicity of the oligonucleotides to eukaryotic cells in an assay using human embryonic kidney-293 cells (HEK-293).
Results
Selection of Targets for PNA Oligomers
Based on the predicted secondary and tertiary structures of the targeted mRNAs, four antisense PNA sequences were designed (Table 1). Their complementary sequences overlapped the most favorable mRNA sites that covered the translation start codon (Dryselius et al., 2003; Deere et al., 2005) and lacked stable secondary structures such as stem-loops or hairpins (Rasmussen et al., 2007).
To validate the sequence homology of the PNA target sites in E. coli O157:H7 and WR3551/98, these regions were amplified by PCR using specific primers (Supplementary Table 2) and sequenced. All sequences were identical in the three E. coli strains, excluding any possibility that mutation of the mRNA target sites for the PNAs might cause decreased susceptibility. To deliver the PNAs into cells, we used a cell-penetrating peptide (KFF)3K, which was covalently linked to each PNA oligomer via an AEEA (aminoethoxyethoxyacetic acid) linker. (KFF)3K is the most widely used cell-penetrating peptide for achieving PNA entry into E. coli cells (Eriksson et al., 2002; Bendifallah et al., 2006).
As a negative control, a random PNA (PNAnc) unrelated to the target sequences was used (Table 1). See Materials and Methods for detailed synthesis protocols.
Escherichia coli Growth Inhibition by Sequence-Specific PNAs
To evaluate the potential of antisense PNAs to inhibit the growth of E. coli strains, the minimal inhibitory concentrations (MIC) of the compounds were determined. All tested PNAs inhibited E. coli growth. First, the MICs for the (KFF)3K-PNAs targeted to antitoxins were measured.
The MIC of the anti-mazE PNA was 16 μM for all three E. coli strains (Figure 2, top), while the anti-hipB PNA prevented the growth of E. coli K-12 at a concentration of 8 μM and E. coli WR3551/98 at 16 μM (Figure 2, bottom). In E. coli O157:H7, the antimicrobial activity of anti-hipB PNA was observed only up to 8 h of exposure (Figure 2, bottom). Therefore, the MIC was recorded as being >16 μM.
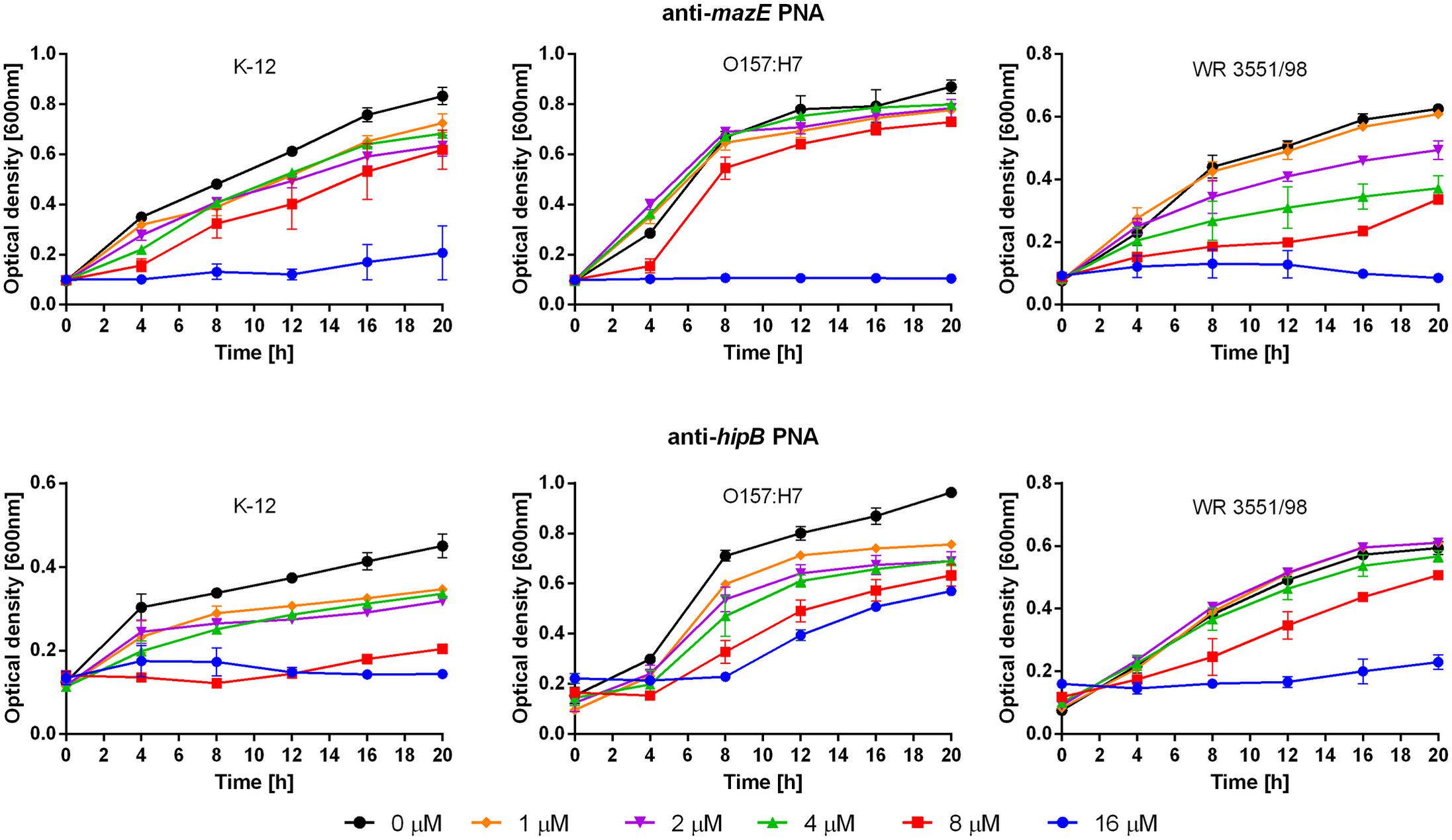
FIGURE 2. Growth inhibition after treatment with the anti-mazE (top) and anti-hipB (bottom) PNAs. Error bars represent the mean ± SEM, n = 3. For all strains the differences between 0 and 16 μM concentrations at 20 h are statistically significant with P < 0.001.
Next, the antisense PNAs to the two TAs-associated target genes were tested (Table 1). The anti-thyA PNA had lower activity against E. coli K-12 (MIC > 16 μM) than against the strains O157:H7 and WR3551/98 (MIC = 16 μM) (Figure 3, top). After treatment with anti-gltX PNA, the growth of E. coli K-12, O157:H7, and WR3551/98 was prevented at MICs of 4, 16, and 1 μM, respectively (Figure 3, bottom).
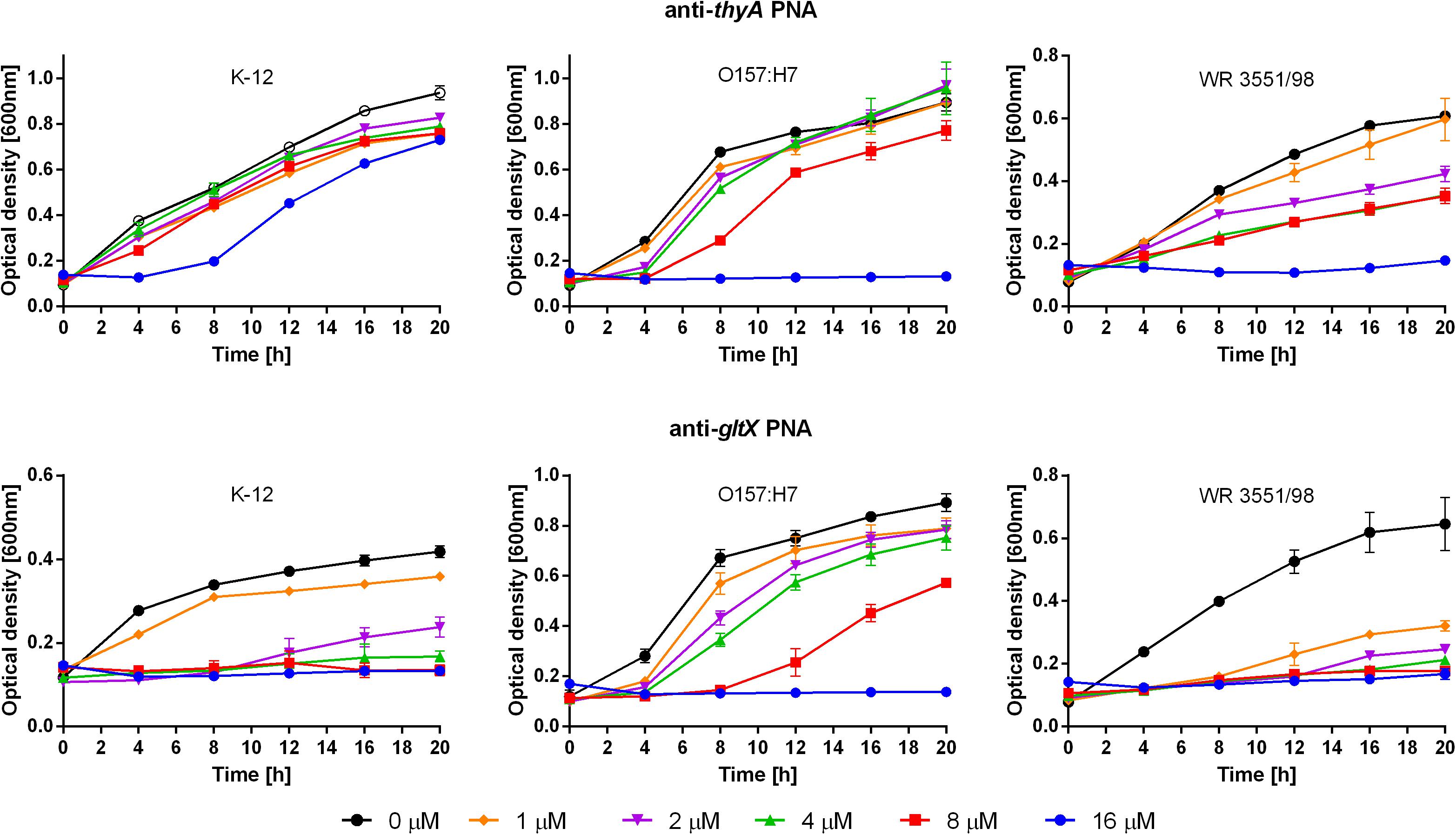
FIGURE 3. Growth inhibition after treatment with the anti-thyA (top) and anti-gltX (bottom) PNAs. Error bars represent the mean ± SEM, n = 3. For all strains the differences between 0 and 16 μM concentration at 20 h are statistically significant with P < 0.001.
To control for specificity of the interaction of the sequence-specific PNAs, the non-complementary PNAnc was tested (Table 1). No growth inhibition was observed following the addition of the (KFF)3K-PNAnc at concentrations of up to 16 μM (Supplementary Figure 1A). Addition of the (KFF)3K peptide alone at concentrations above 16 μM also failed to cause any significant reduction in E. coli growth (Supplementary Figure 1B).
To confirm that the observed growth inhibition was a consequence of toxin action, we used E. coli mutants lacking either the mazF (K-12 MG1655 ΔmazF) or hipA (JW1500-2 ΔhipA) toxin genes (Supplementary Table 1). The ΔmazF mutant was treated with anti-mazE PNA and the ΔhipA mutant with anti-hipB PNA. No growth inhibition of these mutant strains was observed following treatment with the corresponding PNAs at concentrations of up to 16 μM (Supplementary Figure 2). This result verified that the activity of the MazF and HipA toxins was the main cause of growth inhibition after treatment with the specific PNAs.
Effect of PNAs on Corresponding Gene Transcripts
We further tested whether the reduction in growth of E. coli upon treatment with sequence-specific PNAs was reflected in the changes of the corresponding gene transcript levels. To determine the level of the targeted mRNAs, the quantitative reverse transcription polymerase chain reaction (qRT-PCR) was used (Figure 4). E. coli K-12 cultures were treated with sub-MIC concentrations of each respective PNA and the level of mRNAs was compared to untreated control samples. Upon treatment with 8 μM anti-mazE PNA, the amount of mazE transcript was reduced to 60% (Figure 4). The level of thyA mRNA was reduced to 55% after treatment with 16 μM anti-thyA PNA. Similarly, the level of hipB and gltX transcripts was diminished to 40 and 35%, respectively, after treatment with sub-MIC concentrations (4 μM anti-hipB and 1 μM anti-gltX) of corresponding PNAs (Figure 4). We also monitored the mRNA levels of the mazF and hipA toxin genes. Treatment with PNA conjugates targeting these antitoxin genes did not influence the relative mazF and hipA mRNA levels detected by qRT-PCR (Supplementary Figure 3).
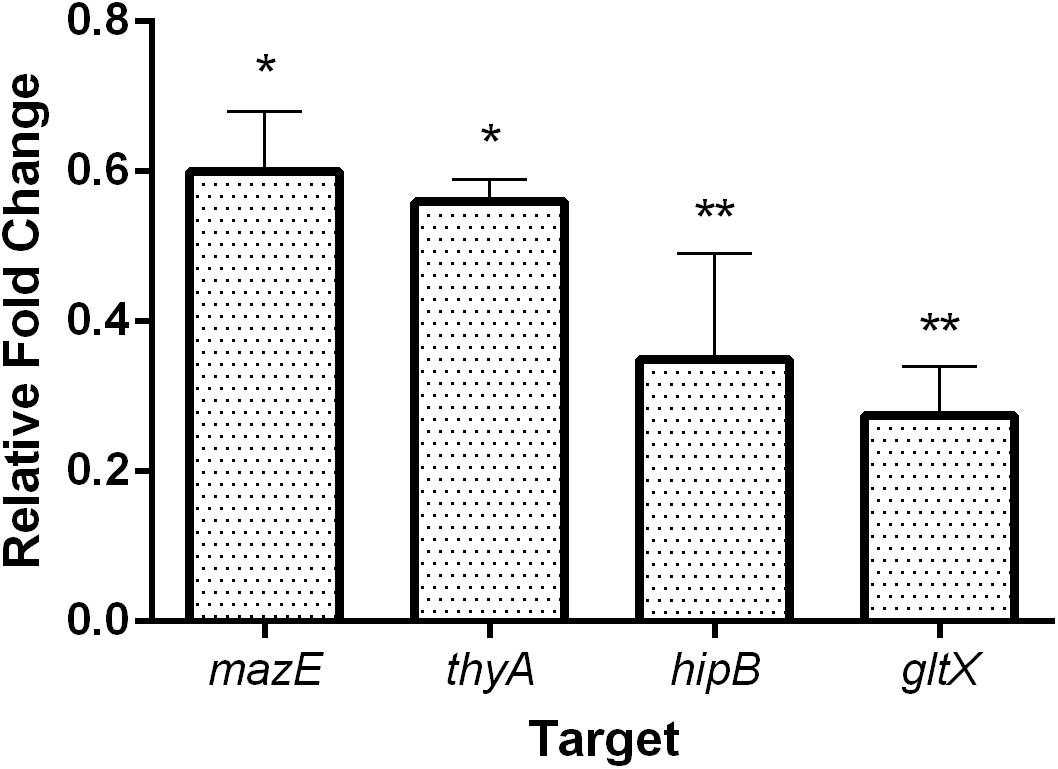
FIGURE 4. The effect of PNA treatment on the abundance of the targeted mRNA transcripts in E. coli K-12. The graph shows the relative expression fold change compared to respective untreated control samples. Error bars represent the mean ± SEM, n = 2. ∗P < 0.1, ∗∗P < 0.05.
Antimicrobial Synergy Between PNAs and Antibiotics
It has been demonstrated that the antibiotics trimethoprim and sulfonamide induce thymine starvation by inhibiting folic acid metabolism, and this triggers mazEF-mediated cell death in E. coli (Engelberg-Kulka et al., 2004). Because folic acid metabolism inhibitors and the mazEF-targeted PNAs (anti-mazE and anti-thyA, Table 1) have related targets (Figure 5A), we sought to determine whether they act synergistically against E. coli O157:H7. The combinatory effects of antibiotics and PNAs were examined in checkerboard assays and quantified by the calculation of FIC indices. Following this protocol, the MIC for each compound was determined independently, and subsequently this was repeated for each concentration of the PNA combined with each concentration of the antibiotic (Figure 5B). The combinations of anti-mazE PNA/trimethoprim (Figure 5B1) and PNAs/sulfamethoxazole (Figures 5B2, B4) presented an interaction of indifference. However, the combination of anti-thyA PNA with trimethoprim resulted in a highly synergistic interaction (FICi = 0.31; Figure 5B3).
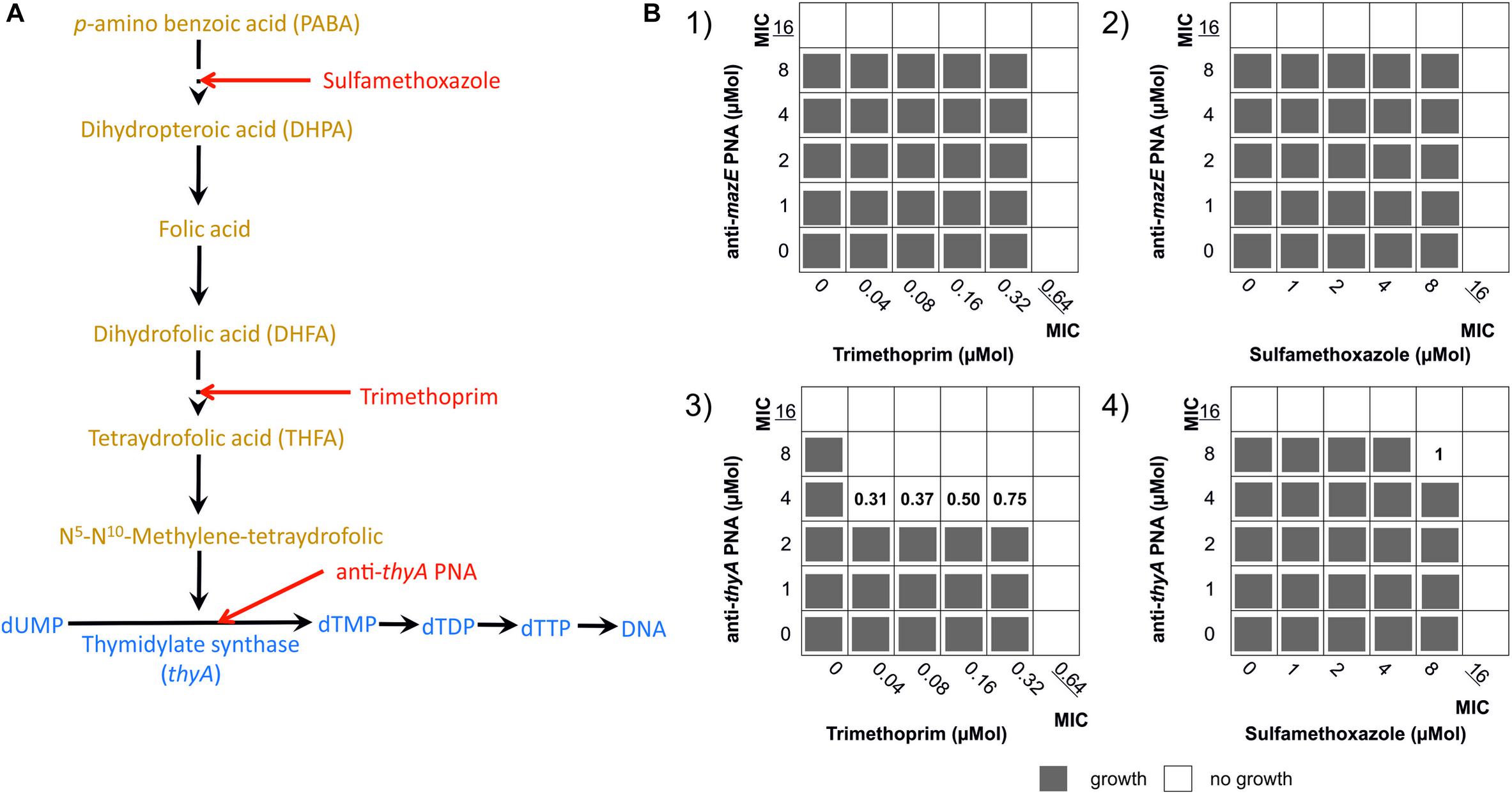
FIGURE 5. Effect of combining treatments that interfere with folic acid metabolism on the growth of E. coli O157:H7. (A) The main steps in the metabolism of folic acid (orange) and thymine (blue). Red arrows mark the points of action of sulfamethoxazole, trimethoprim, and (KFF)3K-PNA anti-thyA. Based on Engelberg-Kulka et al. (2004). (B) Checkerboard tests showing the FIC indices calculated for the combinations of (B1) trimethoprim/anti-mazE PNA, (B2) sulfamethoxazole/anti-mazE PNA, (B3) trimethoprim/anti-thyA PNA, and (B4) sulfamethoxazole/anti-thyA PNA. Results evaluated after 12 h of incubation.
Influence of PNAs on Human Cells
The cytotoxic effect of the tested (KFF)3K-PNA conjugates on human embryonic kidney cells 293 (HEK-293) was evaluated. For these experiments the (KFF)3K-PNA conjugates were applied at a concentration of 32 μM. No significant impact on cell viability was observed after 24 or 48 h of treatment compared to untreated control cells (Figure 6).
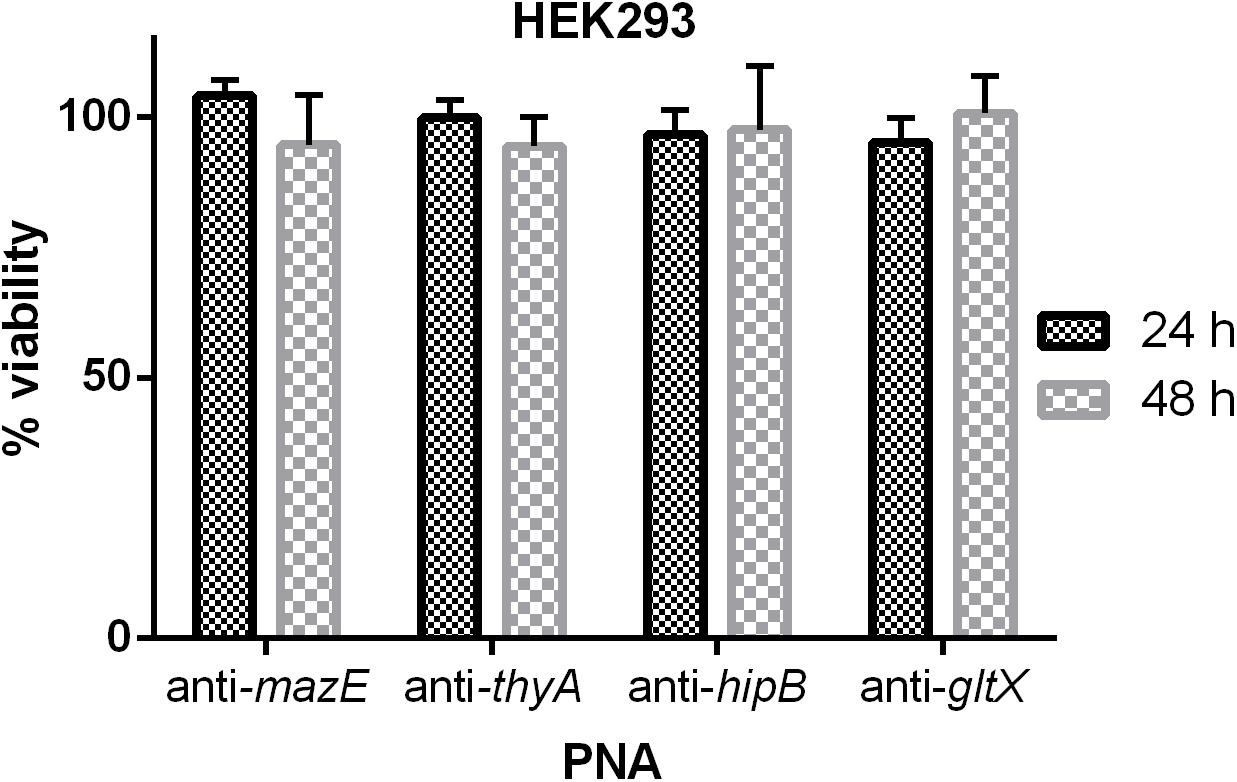
FIGURE 6. The cytotoxic effect of PNAs at a concentration of 32 μM on HEK-293 cells. The results are presented as the % viability in comparison to untreated control cells. Error bars represent the mean ± SD; n = 3.
Discussion
The exploitation of TAs in antibacterial strategies via artificial activation of the toxin has been proposed (Shapiro, 2013; Williams and Hergenrother, 2014; Chan et al., 2015), but there have been no experimental data to support this idea until now. The results of this study provide a proof-of-concept for the use of bacterial TAs as novel targets for antimicrobial compounds. Operons encoding the targeted TAs, mazEF and hipBA, conserved in both laboratory and pathogenic E. coli strains (Fernandez-Garcia et al., 2016), were found to be promising candidates for antisense-based antibacterials. A reduction in E. coli growth was obtained by inhibiting the expression of the mazE and hipB antitoxins using PNA oligomers targeting their mRNAs.
To the best of our knowledge, artificial activation of toxins by antisense oligonucleotides has never been attempted as an antibacterial strategy (Narenji et al., 2017). PNAs have been tested as antibacterials, typically by targeting the expression of essential genes. The TAs genes are non-essential, and this might explain why the MICs obtained in this study are slightly higher than those previously reported for PNAs (Dryselius et al., 2003, 2005; Nikravesh et al., 2007). On the other hand, these MIC values are comparable with those reported for cases where essential sites on ribosomal RNA were targeted (Hatamoto et al., 2009; Górska et al., 2016; Kulik et al., 2017).
Furthermore, we tested PNAs specific for two additional targets related to the activities of mazEF and hipBA. First, we targeted the thyA gene in order to induce thymine starvation and as a consequence indirectly activate the MazF toxin. The other target was the gltX gene encoding glutamyl-tRNA synthase, the enzyme inhibited by HipA toxin. The assumption was that lowering the concentration of GltX should result in fatal effects for the bacterial cell, similar to those observed in the case of the HipA-mediated inactivation. Both approaches were successful, showing that multiple antimicrobial strategies might be based on the action of TAs.
With the PNAs targeted to inhibit the translation of the thyA and gltX transcripts, E. coli WR3551/98 turned out to be the most susceptible strain. This observation is consistent with a previous report that extended-spectrum beta-lactamase (ESBL)-producing E. coli strains are more susceptible to an anti-rpoD PNA than the wild-type strain (Bai et al., 2012). Furthermore, the obtained MICs for the anti-thyA and anti-gltX PNAs are generally comparable with those reported for PNAs targeting essential genes. For example, in the studies employing E. coli K-12, the MIC of a PNA targeting the acpP gene was 1 μM (Nikravesh et al., 2007), while those of PNAs against the fabI and fabD genes were both 3 μM (Dryselius et al., 2005). Overall, E. coli O157:H7 was the least susceptible to the PNAs tested in this study. This observation may be due to the diverse genetic background of the E. coli strains and consequently different expression levels of the target genes in response to respective PNAs. Another possible reason could be due to the differences in the structure of the lipopolysaccharide in E. coli strains, which is a known limiting factor for antimicrobial PNA action (Good et al., 2000).
Antisense silencing using PNA in bacteria leads to translational repression via steric blocking of the ribosome assembly on the mRNA (Rasmussen et al., 2007). As a consequence, such non-translated transcripts are degraded (Dryselius et al., 2006; Goh et al., 2009). Therefore, the effectiveness of antisense silencing can be estimated by quantifying mRNA abundance (Kurupati et al., 2007). Note, that the qRT-PCR experiments were performed on the E. coli K-12 strain and not on O157:H7 or WR3551/98 strains. This was mostly because the yield and quality of the RNA (which are critical in qRT-PCR analysis), isolated from E. coli O157:H7, were much lower than the RNA isolated form E. coli K-12. Although there are differences in the response of the tested E. coli strains to different PNA oligomers, the tendency is similar in the O157:H7 and WR3551/98 strains as compared to K-12. We thus used wild-type E. coli K-12 as a background in all qRT-PCR experiments.
We detected a significant reduction in the levels of the targeted mRNAs upon treatment with the complementary PNAs (Figure 4), confirming their sequence-specific binding to these transcripts. Similar observations have been reported in various bacterial species, e.g., in Streptococcus pyogenes where the application of anti-gyrA PNA decreased the gyrA mRNA level to about 50% of the untreated control value (Patenge et al., 2013). In E. coli the level of acpP mRNA was diminished to about 60% of the control following treatment with anti-acpP PNA (Nikravesh et al., 2007). To demonstrate the absence of off-target activity of the tested PNAs, the levels of the mRNAs for the mazF and hipA toxin genes were monitored after treatment with PNA conjugates targeting the corresponding antitoxin genes. The transcript levels of these toxin genes remained unchanged as compared to the untreated control samples (Supplementary Figure 3). Since the antitoxin and toxin genes are organized in operons, we presume that disruption of translation of the former does not totally prevent translation of the latter. Although the genes are co-transcribed as a single mRNA, the toxin gene carries its own ribosome binding site located within the upstream antitoxin gene (Chan et al., 2012).
The use of synergistic antibiotic/non-antibiotic combinations is a promising option for maximizing the therapeutic effect against problematic drug-resistant pathogens (Schneider et al., 2017). Moreover, the combination of agents makes the emergence of resistance in bacteria less likely (Doern, 2014). The in vitro effectiveness of the combined application of antisense PNAs and traditional antibiotics has already been demonstrated for several bacterial pathogens such as Campylobacter jejuni (Mu et al., 2013), Streptococcus pyogenes (Patenge et al., 2013), Staphylococcus aureus, and E. coli (Dryselius et al., 2005). However, in all these reports, the authors described synergistic antimicrobial effects for the combinations of drugs sharing the same targets. In addition, Patenge et al. (2013) also showed synergy between agents independent of the targeted pathways.
The combination of trimethoprim and sulfamethoxazole (co-trimoxazole) is commonly used in clinical treatment because of their impedance of two sequential steps in folic acid synthesis (Figure 5A) (Bushby, 1975; Harvey, 1982). Since both agents trigger mazEF-mediated growth inhibition (Engelberg-Kulka et al., 2004), synergistic interactions with the mazEF-targeted PNAs were expected. However, we found strong synergy only for the combination of anti-thyA PNA and trimethoprim (Figure 5B3). The combination of the same anti-thyA PNA and sulfamethoxazole resulted in an interaction of indifference (Figure 5B4). This observation is interesting with regard to the mechanism of synergy. All three agents act on the same metabolic pathway (Figure 5A), but the synergistic effect was observed only for two combinations, i.e., trimethoprim/sulfamethoxazole and trimethoprim/anti-thy PNA. Dryselius et al. (2005) tested combinations of PNAs and drugs against the folate biosynthesis pathway in E. coli and S. aureus. Surprisingly, they found synergy when sulfamethoxazole, which targets the dihydropteroate synthase (FolP), was combined with anti-folP PNA. However, they did not observe synergy when the anti-folP PNA was combined with trimethoprim, which targets dihydrofolate reductase (FolA). Similarly, the combination of anti-folA PNA and trimethoprim showed synergy but the sulfamethoxazole/anti-folA PNA combination showed indifference (Dryselius et al., 2005). Further investigation is required to understand these drug interactions, but the results of this and previous studies show how combinations of PNAs and antibiotics can be applied to help decipher mechanisms of drug action.
PNA oligonucleotides are generally considered to have negligible toxicity for eukaryotic cells (Good et al., 2001; Evers et al., 2015). However, whether or not PNAs elicit toxic effects is highly dependent on their sequence composition and the presence of a transporter vehicle (Pandey et al., 2009; Rozners, 2012; Zeng et al., 2016). We demonstrated that treatment of HEK-293 cells with the designed PNAs at a concentration of 32 μM did not cause significant cytotoxicity. This observation is consistent with those reported previously (Sharma et al., 2017). However, another study found that 2-aminopyridine-modified PNAs and their peptide conjugates were not cytotoxic up to 10 μM, but toxicity occurred at higher concentrations (Hnedzko et al., 2017). For this reason, confirmation that PNA-peptide conjugates are not toxic to mammalian cells is crucial before their therapeutic use is considered.
Two potential undesirable effects of artificial activation of TAs are bacterial persistence and biofilm formation (Lewis, 2007; Díaz-Orejas et al., 2017). The precise mechanism of persister formation is currently unknown (Formation et al., 2017), but according to previous reports, only overexpression of the toxin induces persistence (Pedersen et al., 2002; Lewis, 2008; Harrison et al., 2009; Wood et al., 2013). The PNAs employed in the present study did not lead to overexpression of the toxin (Supplementary Figure 3), so we speculate that the risk of dormant cell formation is relatively low. On the other hand, there is evidence that toxin–antitoxin modules are not the only factors that contribute to the formation of persister cells (Fisher et al., 2017). The deletion of multiple TAs reduces persisters but does not fully abolish their presence (Kint et al., 2012; Chowdhury et al., 2016). It is possible that there is heterogeneity within the persister population, with several different pathways contributing to the formation of persister cells. Furthermore, we speculate that the artificial activation of E. coli TAs by antisense PNA oligomers could induce the stringent response. This phenomenon is a bacterial response to stress conditions, e.g., amino-acid starvation (Cashel et al., 1996). The stringent response has been described as an important mechanism for the persister formation as a reaction to a diauxic growth (Amato and Brynildsen, 2015). However, how the formulation of persister cells is connected to this stress response is still unknown. Persister cells are present in each population prior to any treatment with antibacterial agent. Therefore, further investigation is required to determine the exact roles of central metabolism and toxin–antitoxin modules in the formation of persisters. In the future, we plan to determine whether artificial activation of TAs by PNAs influences persister formation and if it triggers the stringent response.
Materials and Methods
Target Site Selection
The genome sequence of E. coli K-12 was obtained from the KEGG database (Ogata et al., 1999). For secondary and tertiary structure prediction of the targeted mRNAs, the Mfold (Zuker, 2003), and RNAComposer (Popenda et al., 2012) software were used, respectively. To ensure target specificity of PNAs, the target sites were examined for uniqueness within the E. coli K-12 genome using the on-line search tools GenoList (Lechat et al., 2008) and RiboScanner (Górska et al., 2016), with special consideration given to the start codon region that includes the consensus Shine-Dalgarno sequence.
Reagents and Conditions for (KFF)3K-AEEA-PNA Synthesis
Commercial reagents and solvents were used as received from the supplier. NovaSyn®TG Sieber resin for PNA synthesis was obtained from Merck and Fmoc/Bhoc-protected PNA monomers (Fmoc-PNA-A(Bhoc)-OH, Fmoc-PNA-G(Bhoc)-OH, Fmoc-PNA-C(Bhoc)-OH, Fmoc-PNA-T-OH) from Panagene and Link Technologies Ltd. Fmoc-AEEA-OH was purchased from Link Technologies Ltd. Nα-Fmoc protected L-amino acids (Fmoc-Lys(Boc)-OH, Fmoc-Phe-OH) were obtained from Sigma-Aldrich. Preparative chromatography was performed using C18 reversed-phase silica gel 90 Å (Sigma-Aldrich) with redistilled water and HPLC grade MeCN as eluents with the addition of HPLC grade trifluoroacetic acid (TFA) (0.1% v/v solution). The following conditions were used for HPLC: column – Eurospher II 100–5 C18, 250 mm Å – 4.6 mm with a precolumn; pressure – 230 bar; flow rate – 4.5 ml/min; room temperature; detection – UV/Vis at a wavelength of 267 nm.
Synthesis of (KFF)3K-AEEA-PNA Conjugates
The conjugates of (KFF)3K with PNA connected via an aminoethylethanolamine linker (AEEA) were in-house synthesized manually using the Fmoc solid-phase methodology at 10 μmol scale. The syntheses were conducted using 9-Fmoc-amino-xanthen-3-yloxy TG resin with amine group loading of 190 μmol/g. This resin carries a linker, which yields a C-terminal amide upon TFA cleavage of the PNA. In all syntheses, Lys was the first monomer attached to the resin. PNA oligomers were synthesized using a 2.5-fold molar excess of the Fmoc/Bhoc-protected PNA monomers. To activate the monomers, we used a 2-(1H-7-azabenzotriazole-1-yl)-1,1,3,3-tetramethyluronium hexafluorophosphate (HATU), N-methylmorpholine (NMM), and 2,6-lutidine (0.7:1:1.5) mixture in DMF/NMP (1:1, v/v) solution. Coupling of the activated compounds was carried out two times for 30 min each. The Fmoc group was removed with 20% (v/v) piperidine in DMF (2 mm × 2 min). After the PNA oligomer synthesis and N-terminal Fmoc deprotection, Fmoc-AEEA-OH was attached to the N-terminus. Then, the N-terminal Fmoc was removed, and amino acids were coupled to yield the (KFF)3K peptide. We used a 3-fold and 1.5-fold molar excess of Fmoc-AEEA-OH and the Fmoc-protected amino acids (Fmoc-Lys-(Boc)-OH, Fmoc-Phe-OH) in the respective couplings performed two times for each substrate (for 60 and 40 min, respectively). The reagents were activated with HATU and the addition of HOAt and collidine (1:1:2) and N,N-dimethylpyridin-4-amine (DMAP) as a catalyst, in DMF/NMP (1:1, v/v) solution. The Fmoc groups of Fmoc-AEEA-OH and amino acids were deprotected using 20% (v/v) piperidine in DMF in two cycles (5 and 15 min). All of the protecting groups were removed and cleavage of the (KFF)3K-AEEA-PNA conjugates from the resin was accomplished by treatment with a TFA/triisopropylsilane/m-cresol (95:2.5:2.5; v/v/v) mixture for 60 min. The crude products were precipitated with cold diethyl ether, collected by centrifugation, decanted, lyophilized, and subsequently purified by RP-HPLC. The HPLC methods and molecular masses are shown in Supplementary Table 3, and the RP-HPLC chromatograms and MS spectra of the purified products in Supplementary Figures 4–8.
Bacterial Strains and Growth Conditions
The strains used in this study are listed in Supplementary Table 1. All strains were grown in LB or LA medium at 37°C. When necessary, the media were supplemented with kanamycin (50 μg/ml), chloramphenicol (20 μg/ml), sucrose (10% w/v), or diaminopimelic acid (DAP, 0.3 mM). For susceptibility tests, the strains were grown in cation-adjusted Mueller Hinton Broth (Difco) at 37°C with shaking.
PCR for Sequencing
Bacterial gDNA was extracted from cell pellets using a Bacterial & Yeast Genomic DNA Purification Kit (EURx, Poland) according to the manufacturer’s instructions. Based on the E. coli K-12 MG1655 genome sequence (RefSeq assembly accession: GCF_000005845.2) appropriate oligonucleotide primers were designed (Supplementary Table 2) for the amplification of fragments of the genes overlapping the targeted sequences. The PCR products were purified and sequenced.
Genetic Manipulations and Strain Construction
DNA manipulations were performed using standard procedures, and according to the manufacturer’s instructions included in kits for DNA isolation and PCR (Phusion, Thermo Fisher Scientific). Deletion of the E. coli K-12 MG1655 mazF gene was performed using the gene replacement method (Pósfai et al., 1999). The plasmid for the mutagenesis [pDS132-mazFKm was generated by the Gibson assembly procedure (Gibson et al., 2009)]. The DNA fragments used for the assembly were amplified by PCR using the following primer pairs: (i) mazF1/mazF2 and mazF3/mazF4 – amplification of DNA fragments flanking the mazF gene using MG1655 genomic DNA as template, (ii) KML/KMR – amplification of the kanamycin resistance gene from plasmid pMSB1 (Dziewit et al., 2011), and (iii) pds132X/pds132Y – amplification of mobilizable vector pDS132 carrying the sacB gene enabling counter-selection with sucrose (Philippe et al., 2004). The assembled plasmid was transformed into E. coli DH5αλpir cells, carrying the pir gene required for pDS132 replication. Isolated pDS132-mazFKm plasmid DNA was then used to transform E. coli strain β2163 (carrying the pir gene) and a single transformant was used as a donor in a bi-parental mating with strain MG1655 (without the pir gene) as the recipient. The ΔmazF mutant colonies (in which the mazF gene was replaced by the kanamycin resistance gene following homologous recombination between the flanking regions) were selected on LA medium containing kanamycin and sucrose. The deletion was confirmed by PCR using primers LmazFspr/RmazFspr and sequencing of the amplified DNA fragment.
Minimal Inhibitory Concentrations
The minimal inhibitory concentrations (MICs) of the antibiotics, (KFF)3K-PNAs and free (KFF)3K were determined by broth microdilution according to Clinical and Laboratory Standards Institute method M07-A10 (CLSI, 2015). Each MIC was scored as the lowest concentration that prevented visibly detectable growth. Cells were cultured in Mueller-Hinton Broth (MHB) to exponential phase and diluted to ∼5 × 105 CFU/ml. Aliquots of the cell suspensions were added to the wells of sterile 96-well plates containing dilutions of the tested agents and these plates were incubated at 37°C in a microplate reader for 20 h. During incubation, the optical density at 600 nm (OD600) of the culture in each well was measured at 10-min intervals. Before each measurement, the plate was briefly shaken to suspend the cells. Each experiment was performed on at least three independent biological replicates.
RNA Isolation and Quantitative Reverse Transcription Polymerase Chain Reaction
RNA was isolated from 4 μl × 100 μl samples of E. coli K-12 cultures incubated for 6-h with sub-MIC concentrations of each respective antisense (KFF)3K-PNA oligomer (8 μM for anti-mazE, 4 μM for anti-hipB, 16 μM for anti-thyA, and 1 μM for anti-gltX). The cells were collected by centrifugation, and total bacterial RNA was isolated using a High Pure RNA Isolation Kit (Roche Molecular Systems), following the protocol provided by the manufacturer. All qPCR procedures were performed according to the MIQE guidelines (Bustin et al., 2009). To detect the expression of the mazE, mazF, thyA, hipB, hipA, and gltX genes, 50 ng of the total RNA was reverse transcribed to generate cDNA using a Maxima First Strand cDNA Synthesis Kit for RT-qPCR with dsDNase (Thermo ScientificTM), as outlined in the manufacturer’s protocol. qPCR was performed using SYBR® Green Master Mix (Bio-Rad Laboratories Inc., United States) according to the supplied instructions. The level of the transcript of the essential gyrA gene was used for normalization (Rocha et al., 2015). The gene-specific primer pairs used for qPCR are listed in Supplementary Table 3. Amplification was performed in a Biorad CFX96 TouchTM Real-Time PCR Detection System (Bio-Rad Laboratories Inc., United States). Relative quantification of gene transcription was performed using the ΔΔCT method (Livak and Schmittgen, 2001). The presented data represent averages of triplicate determinations, performed at least two times. Statistical analyses were performed with one-way ANOVA with Fisher’s test for multiple comparisons. Differences between treated and non-treated control samples were considered not significant (P ≥ 0.05), marginally significant (P < 0.05)∗, or significant (P < 0.01)∗∗.
Synergy With Antibiotics – Fractional Inhibitory Concentrations
The interaction of (KFF)3K-PNAs and antibiotics was examined using a standard checkerboard microdilution method (Figure 5B; Hsieh et al., 1993). Samples of E. coli O157:H7 culture grown in MHB were added to the wells of sterile 96-well plates containing combinations of two agents at various concentrations, and the plates were incubated at 37°C for 12 h. The OD600 of the culture in each well was then determined using a plate reader. To define the nature of the interactions, the Fractional Inhibitory Concentration Indices (FICi) were calculated for each well with the equation FICi = FICA + FICB = (CA/MICA) + (CB/MICB), where MICA and MICB are the MICs of compounds A and B alone, respectively, and CA and CB are the concentrations of the compounds in combination, respectively. The FICi values were interpreted using a conservative model restricting interpretation to the following interaction categories: synergy (FICi ≤ 0.5), additive (FICi > 0.5–1.0), and indifference (1.0–4.0) (Doern, 2014).
Cytotoxicity Assay
HEK-293 cells were cultured in DMEM medium with high glucose (Lonza) supplemented with 10% FBS (Biowest) and Penicillin–Streptomycin (50 U/ml, Gibco) at 37°C in 5% CO2. The cells were seeded at 5 × 104 or 105 per well in 96-well plates for incubations of 24 or 48 h, respectively. Wells contained the (KFF)3K-PNAs at a final concentration of 32 μM. Cell viability following incubation with the (KFF)3K-PNAs was determined using the standard MTT [3-(4,5-dimethylthiazol-2-yl)-2,5-diphenyltetrazolium bromide] (Merck) assay (Mosmann, 1983) with absorbance at 590 nm measured using a plate reading spectrophotometer (Synergy H1MFDG, Biotek). Each experiment was performed for three independent biological replicates. Statistical significance compared to untreated control samples was determined using the Mann-Whitney U test. All results were considered as statistically significant if P < 0.05.
Data Availability Statement
The raw data supporting the conclusions of this manuscript will be made available by the authors, without undue reservation, to any qualified researcher.
Author Contributions
MR designed the PNA sequences, performed the MIC, RT-qPCR, and FIC experiments, and analyzed the data. TP synthesized the PNA oligomers and PNA-peptides. JC, DB, and MR designed and prepared the E. coli K-12 MG1655 ΔmazF mutant. MK examined the cytotoxic effect of (KFF)3K-PNAs on eukaryotic cells. JT and DB supervised the overall project. MR, TP, and JT wrote the manuscript. All authors discussed the results and revised the manuscript.
Funding
We acknowledge support from the National Science Centre, Poland (PRELUDIUM 2017/25/N/NZ1/01578 to MR; SYMFONIA 2014/12/W/ST5/00589 to JT, MR, TP, and MK), the Ministry of Science and Higher Education through the CeNT, University of Warsaw intramural grant DSM #501-D313-86-0117000-02, and Polish-U.S. Fulbright Commission (to JT and MR).
Conflict of Interest Statement
The authors declare that the research was conducted in the absence of any commercial or financial relationships that could be construed as a potential conflict of interest.
Acknowledgments
We thank A. Szalewska-Pałasz and A. Krawczyk-Balska for providing the E. coli O157:H7 and WR3551/98 strains, respectively. JT would like to thank K. Jażdżewski for helpful discussions.
Supplementary Material
The Supplementary Material for this article can be found online at: https://www.frontiersin.org/articles/10.3389/fmicb.2018.02870/full#supplementary-material
References
Abushahba, M. F. N., Mohammad, H., Thangamani, S., Hussein, A. A. A., and Seleem, M. N. (2016). Impact of different cell penetrating peptides on the efficacy of antisense therapeutics for targeting intracellular pathogens. Sci. Rep. 6:20832. doi: 10.1038/srep20832
Amato, S. M., and Brynildsen, M. P. (2015). Persister heterogeneity arising from a single metabolic stress. Curr. Biol. 25, 2090–2098. doi: 10.1016/j.cub.2015.06.034
Bai, H., You, Y., Yan, H., Meng, J., Xue, X., Hou, Z., et al. (2012). Antisense inhibition of gene expression and growth in gram-negative bacteria by cell-penetrating peptide conjugates of peptide nucleic acids targeted to rpoD gene. Biomaterials 33, 659–667. doi: 10.1016/j.biomaterials.2011.09.075
Baraniak, A. (2002). Ceftazidime-hydrolysing CTX-M-15 extended-spectrum beta-lactamase (ESBL) in Poland. J. Antimicrob. Chemother. 50, 393–396. doi: 10.1093/jac/dkf151
Bendifallah, N., Rasmussen, F. W., Zachar, V., Ebbesen, P., Nielsen, P. E., and Koppelhus, U. (2006). Evaluation of cell-penetrating peptides (CPPs) as vehicles for intracellular delivery of antisense peptide nucleic acid (PNA). Bioconjug. Chem. 17, 750–758. doi: 10.1021/bc050283q
Bushby, S. R. M. (1975). Synergy of trimethoprim-sulfamethoxazole. Can. Med. Assoc. J. 112, 638–668.
Bustin, S. A., Benes, V., Garson, J. A., Hellemans, J., Huggett, J., Kubista, M., et al. (2009). The MIQE guidelines: minimum information for publication of quantitative real-time PCR experiments. Clin. Chem. 55, 611–622. doi: 10.1373/clinchem.2008.112797
Cashel, M., Gentry, D. R., Hernandez, V. J., and Vinella, D. (1996). The stringent response. Escherichia coli and Salmonella. Cell Mol. Biol. 2, 913–931. doi: 10.1128/IAI.00193-11
Chan, W. T., Balsa, D., and Espinosa, M. (2015). One cannot rule them all: are bacterial toxins-antitoxins druggable? FEMS Microbiol. Rev. 39, 522–540. doi: 10.1093/femsre/fuv002
Chan, W. T., Espinosa, M., and Yeo, C. C. (2016). Keeping the wolves at bay: antitoxins of prokaryotic type II toxin-antitoxin systems. Front. Mol. Biosci. 3:9. doi: 10.3389/fmolb.2016.00009
Chan, W. T., Moreno-Córdoba, I., Yeo, C. C., and Espinosa, M. (2012). Toxin-antitoxin genes of the Gram-positive pathogen Streptococcus pneumoniae: so few and yet so many. Microbiol. Mol. Biol. Rev. 76, 773–791. doi: 10.1128/MMBR.00030-12
Chowdhury, N., Kwan, B. W., and Wood, T. K. (2016). Persistence increases in the absence of the alarmone guanosine tetraphosphate by reducing cell growth. Sci. Rep. 6:20519. doi: 10.1038/srep20519
CLSI (2015). Methods for Dilution Antimicrobial Susceptibility Tests for Bacteria That Grow Aerobically. Approved Standard, 9th Edn. Wayne, PA: CLSI,
Coates, A. R. M., and Hu, Y. (2007). Novel approaches to developing new antibiotics for bacterial infections. Br. J. Pharmacol. 152, 1147–1154. doi: 10.1038/sj.bjp.0707432
Deere, J., Iversen, P., and Geller, B. L. (2005). Antisense phosphorodiamidate morpholino oligomer length and target position effects on gene-specific inhibition in Escherichia coli. Antimicrob. Agents Chemother. 49, 249–255. doi: 10.1128/AAC.49.1.249-255.2005
Dias, N., and Stein, C. A. (2002). Antisense oligonucleotides: basic concepts and mechanisms. Mol. Cancer Ther. 1, 347–355.
Díaz-Orejas, R., Espinosa, M., and Yeo, C. C. (2017). The importance of the expendable: toxin-antitoxin genes in plasmids and chromosomes. Front. Microbiol. 8:1479. doi: 10.3389/fmicb.2017.01479
Doern, C. D. (2014). When does 2 plus 2 equal 5? A review of antimicrobial synergy testing. J. Clin. Microbiol. 52, 4124–4128. doi: 10.1128/JCM.01121-14
Dryselius, R., Aswasti, S. K., Rajarao, G. K., Nielsen, P. E., and Good, L. (2003). The translation start codon region is sensitive to antisense PNA inhibition in Escherichia coli. Oligonucleotides 13, 427–433. doi: 10.1089/154545703322860753
Dryselius, R., Nekhotiaeva, N., and Good, L. (2005). Antimicrobial synergy between mRNA- and protein-level inhibitors. J. Antimicrob. Chemother. 56, 97–103. doi: 10.1093/jac/dki173
Dryselius, R., Nikravesh, A., Kulyté, A., Goh, S., and Good, L. (2006). Variable coordination of cotranscribed genes in Escherichia coli following antisense repression. BMC Microbiol. 6:97. doi: 10.1186/1471-2180-6-97
Dziewit, L., Adamczuk, M., Szuplewska, M., and Bartosik, D. (2011). DIY series of genetic cassettes useful in construction of versatile vectors specific for Alphaproteobacteria. J. Microbiol. Methods 86, 166–174. doi: 10.1016/j.mimet.2011.04.016
Engelberg-Kulka, H., Hazan, R., and Amitai, S. (2005). mazEF: a chromosomal toxin-antitoxin module that triggers programmed cell death in bacteria. J. Cell Sci. 118, 4327–4332. doi: 10.1242/jcs.02619
Engelberg-Kulka, H., Sat, B., Reches, M., Amitai, S., and Hazan, R. (2004). Bacterial programmed cell death systems as targets for antibiotics. Trends Microbiol. 12, 66–71. doi: 10.1016/j.tim.2003.12.008
Eriksson, M., Nielsen, P. E., and Good, L. (2002). Cell permeabilization and uptake of antisense peptide-peptide nucleic acid (PNA) into Escherichia coli. J. Biol. Chem. 277, 7144–7147. doi: 10.1074/jbc.M106624200
Evers, M. M., Toonen, L. J. A., and van Roon-Mom, W. M. C. (2015). Antisense oligonucleotides in therapy for neurodegenerative disorders. Adv. Drug Deliv. Rev. 87, 90–103. doi: 10.1016/j.addr.2015.03.008
Fair, R. J., and Tor, Y. (2014). Antibiotics and bacterial resistance in the 21st century. Perspect. Med. Chem. 6, 25–64. doi: 10.4137/PMC.S14459
Fernandez-Garcia, L., Blasco, L., Lopez, M., Bou, G., Garcia-Contreras, R., Wood, T., et al. (2016). Toxin-antitoxin systems in clinical pathogens. Toxins 8, 1–23. doi: 10.3390/toxins8070227
Fisher, R. A., Gollan, B., and Helaine, S. (2017). Persistent bacterial infections and persister cells. Nat. Rev. Microbiol. 15, 453–464. doi: 10.1038/nrmicro.2017.42
Formation, A. P., Shan, Y., Gandt, A. B., Rowe, S. E., Deisinger, J. P., Conlon, B. P., et al. (2017). ATP-dependent persister formation in Escherichia coli. mBio 8:e2267-16. doi: 10.1128/mBio.02267-16
Fu, Z., Tamber, S., Memmi, G., Donegan, N. P., and Cheung, A. L. (2009). Overexpression of MazF Sa in Staphylococcus aureus induces bacteriostasis by selectively targeting mRNAs for cleavage. J. Bacteriol. 191, 2051–2059. doi: 10.1128/JB.00907-08
Germain, E., Castro-Roa, D., Zenkin, N., and Gerdes, K. (2013). Molecular mechanism of bacterial persistence by HipA. Mol. Cell 52, 248–254. doi: 10.1016/j.molcel.2013.08.045
Gibson, D. G., Young, L., Chuang, R.-Y., Venter, J. C., Hutchison, C. A., and Smith, H. O. (2009). Enzymatic assembly of DNA molecules up to several hundred kilobases. Nat. Methods 6, 343–345. doi: 10.1038/nmeth.1318
Goh, S., Boberek, J. M., Nakashima, N., Stach, J., and Good, L. (2009). Concurrent growth rate and transcript analyses reveal essential gene stringency in Escherichia coli. PLoS One 4:e6061. doi: 10.1371/journal.pone.0006061
Good, L., Awasthi, S. K., Dryselius, R., Larsson, O., and Nielsen, P. E. (2001). Bactericidal antisense effects of peptide-PNA conjugates. Nat. Biotechnol. 19, 360–364. doi: 10.1038/86753
Good, L., Sandberg, R., Larsson, O., Nielsen, P. E., and Wahlestedt, C. (2000). Antisense PNA effects in Escherichia coli are limited by the outer-membrane LPS layer. Microbiology 146, 2665–2670. doi: 10.1099/00221287-146-10-2665
Górska, A., Markowska-Zagrajek, A., Równicki, M., and Trylska, J. (2016). Scanning of 16S ribosomal RNA for peptide nucleic acid targets. J. Phys. Chem. B 120, 8369–8378. doi: 10.1021/acs.jpcb.6b02081
Harms, A., Brodersen, D. E., Mitarai, N., and Gerdes, K. (2018). Toxins, targets, and triggers: an overview of toxin-antitoxin biology. Mol. Cell 70, 768–784. doi: 10.1016/j.molcel.2018.01.003
Harrison, J. J., Wade, W. D., Akierman, S., Vacchi-Suzzi, C., Stremick, C. A., Turner, R. J., et al. (2009). The chromosomal toxin gene yafQ is a determinant of multidrug tolerance for Escherichia coli growing in a biofilm. Antimicrob. Agents Chemother. 53, 2253–2258. doi: 10.1128/AAC.00043-09
Harvey, R. J. (1982). Synergism in the folate pathway. Rev. Infect. Dis. 4, 255–260. doi: 10.1093/clinids/4.2.255
Hatamoto, M., Nakai, K., Ohashi, A., and Imachi, H. (2009). Sequence-specific bacterial growth inhibition by peptide nucleic acid targeted to the mRNA binding site of 16S rRNA. Appl. Microbiol. Biotechnol. 84, 1161–1168. doi: 10.1007/s00253-009-2099-0
Hayes, F., and Van Melderen, L. (2011). Toxins-antitoxins: diversity, evolution and function. Crit. Rev. Biochem. Mol. Biol. 46, 386–408. doi: 10.3109/10409238.2011.600437
Hazan, R., Sat, B., and Engelberg-kulka, H. (2004). Escherichia coli mazEF -mediated cell death is triggered by various stressful conditions. Genet. Mol. Biol. 186, 3663–3669. doi: 10.1128/JB.186.11.3663
Hnedzko, D., Mcgee, D. W., Karamitas, Y. A., and Rozners, E. (2017). Sequence-selective recognition of double-stranded RNA and enhanced cellular uptake of cationic nucleobase and backbone-modified peptide nucleic acids. RNA 23:58. doi: 10.1261/rna.058362
Hsieh, M. H., Yu, C. M., Yu, V. L., and Chow, J. W. (1993). Synergy assessed by checkerboard a critical analysis. Diagn. Microbiol. Infect. Dis. 16, 343–349. doi: 10.1016/0732-8893(93)90087-N
Kaspy, I., Rotem, E., Weiss, N., Ronin, I., Balaban, N. Q., and Glaser, G. (2013). HipA-mediated antibiotic persistence via phosphorylation of the glutamyl-tRNA-synthetase. Nat. Commun. 4:3001. doi: 10.1038/ncomms4001
Kędzierska, B., and Hayes, F. (2016). Emerging roles of toxin-antitoxin modules in bacterial pathogenesis. Molecules 21:E790. doi: 10.3390/molecules21060790
Kim, D. H., Kang, S. M., Park, S. J., Jin, C., Yoon, H. J., and Lee, B. J. (2018). Functional insights into the Streptococcus pneumoniae HicBA toxin-antitoxin system based on a structural study. Nucleic Acids Res. 46, 6371–6386. doi: 10.1093/nar/gky469
Kint, C. I., Verstraeten, N., Fauvart, M., and Michiels, J. (2012). New-found fundamentals of bacterial persistence. Trends Microbiol. 20, 577–585. doi: 10.1016/j.tim.2012.08.009
Kirkpatrick, C. L., Martins, D., Redder, P., Frandi, A., Mignolet, J., Chapalay, J. B., et al. (2016). Growth control switch by a DNA-damage- inducible toxin–antitoxin system in Caulobacter crescentus. Nat. Microbiol. 1:16008. doi: 10.1038/nmicrobiol.2016.8
Kulik, M., Markowska-Zagrajek, A., Wojciechowska, M., Grzela, R., Wituła, T., and Trylska, J. (2017). Helix 69 of Escherichia coli 23S ribosomal RNA as a peptide nucleic acid target. Biochimie 138, 32–42. doi: 10.1016/j.biochi.2017.04.001
Kurupati, P., Tan, K. S. W., Kumarasinghe, G., and Poh, C. L. (2007). Inhibition of gene expression and growth by antisense peptide nucleic acids in a multiresistant beta-lactamase-producing Klebsiella pneumoniae strain. Antimicrob. Agents Chemother. 51, 805–811. doi: 10.1128/AAC.00709-06
Lechat, P., Hummel, L., Rousseau, S., and Moszer, I. (2008). GenoList: an integrated environment for comparative analysis of microbial genomes. Nucleic Acids Res. 36, 469–474. doi: 10.1093/nar/gkm1042
Lee, K. Y., and Lee, B. J. (2016). Structure, biology, and therapeutic application of toxin-antitoxin systems in pathogenic bacteria. Toxins 8:E305. doi: 10.3390/toxins8100305
Lewis, K. (2007). Persister cells, dormancy and infectious disease. Nat. Rev. Microbiol. 5, 48–56. doi: 10.1038/nrmicro1557
Lewis, K. (2008). Multidrug tolerance of biofilms and persister cells. Curr. Top. Microbiol. Immunol. 322, 107–131.
Lioy, V. S., Rey, O., Balsa, D., Pellicer, T., and Alonso, J. C. (2010). A toxin-antitoxin module as a target for antimicrobial development. Plasmid 63, 31–39. doi: 10.1016/j.plasmid.2009.09.005
Livak, K. J., and Schmittgen, T. D. (2001). Analysis of relative gene expression data using real- time quantitative PCR and the 2-DDCT method. Methods 25, 402–408. doi: 10.1006/meth.2001.1262
Lobato-Márquez, D., Díaz-Orejas, R., and García-del Portillo, F. (2016). Toxin-antitoxins and bacterial virulencea. FEMS Microbiol. Rev. 40, 592–609. doi: 10.1093/femsre/fuw022
Łoś, J. M., Golec, P., Wȩgrzyn, G., Wȩgrzyn, A., and Łoś, M. (2008). Simple method for plating Escherichia coli bacteriophages forming very small plaques or no plaques under standard conditions. Appl. Environ. Microbiol. 74, 5113–5120. doi: 10.1128/AEM.00306-08
Ma, Y., Zhu, Y., Li, W., Liu, F., Lv, N., Li, J., et al. (2015). Characteristics of persister cells and the diversity of type II toxin - antitoxin system in Acinetobacter baumannii. Wei Sheng Wu Xue Bao Acta Microbiol. Sin. 55, 949–958.
Mosmann, T. (1983). Rapid colorimetric assay for cellular growth and survival: application to proliferation and cytotoxicity assays. J. Immunol. Methods 65, 55–63. doi: 10.1016/0022-1759(83)90303-4
Mu, Y., Shen, Z., Jeon, B., Dai, L., and Zhang, Q. (2013). Synergistic effects of anti-CmeA and anti-CmeB peptide nucleic acids on sensitizing Campylobacter jejuni to antibiotics. Antimicrob. Agents Chemother. 57, 4575–4577. doi: 10.1128/AAC.00605-13
Narenji, H., Gholizadeh, P., Aghazadeh, M., Rezaee, M. A., Asgharzadeh, M., and Kafil, H. S. (2017). Peptide nucleic acids (PNAs): currently potential bactericidal agents. Biomed. Pharmacother. 93, 580–588. doi: 10.1016/j.biopha.2017.06.092
Nekhotiaeva, N., Awasthi, S. K., Nielsen, P. E., and Good, L. (2004). Inhibition of Staphylococcus aureus gene expression and growth using antisense peptide nucleic acids. Mol. Ther. 10, 652–659. doi: 10.1016/j.ymthe.2004.07.006
Nielsen, P. E., Egholm, M., Berg, R. H., and Buchardt, O. (1991). Sequence-selective recognition of DNA by strand displacement with a thymine-substituted polyamide. Science 254, 1497–1500. doi: 10.1126/science.1962210
Nikravesh, A., Dryselius, R., Faridani, O. R., Goh, S., Sadeghizadeh, M., Behmanesh, M., et al. (2007). Antisense PNA accumulates in Escherichia coli and mediates a long post-antibiotic effect. Mol. Ther. 15, 1537–1542. doi: 10.1038/sj.mt.6300209
Ogata, H., Goto, S., Sato, K., Fujibuchi, W., Bono, H., and Kanehisa, M. (1999). KEGG: kyoto encyclopedia of genes and genomes. Nucleic Acids Res. 27, 29–34. doi: 10.1093/nar/27.1.29
Page, R., and Peti, W. (2016). Toxin-antitoxin systems in bacterial growth arrest and persistence. Nat. Chem. Biol. 12, 208–214. doi: 10.1038/nchembio.2044
Pandey, V. N., Upadhyay, A., and Chaubey, B. (2009). Prospects for antisense peptide nucleic acid (PNA) therapies for HIV. Expert Opin. Biol. Ther. 9, 975–989. doi: 10.1517/14712590903052877
Patenge, N., Pappesch, R., Krawack, F., Walda, C., Mraheil, M. A., Jacob, A., et al. (2013). Inhibition of growth and gene expression by PNA-peptide conjugates in Streptococcus pyogenes. Mol. Ther. Nucleic Acids 2:e132. doi: 10.1038/mtna.2013.62
Pedersen, K., Christensen, S. K., and Gerdes, K. (2002). Rapid induction and reversal of a bacteriostatic condition by controlled expression of toxins and antitoxins. Mol. Microbiol. 45, 501–510. doi: 10.1111/j.1540-5915.2006.00123.x
Philippe, N., Alcaraz, J. P., Coursange, E., Geiselmann, J., and Schneider, D. (2004). Improvement of pCVD442, a suicide plasmid for gene allele exchange in bacteria. Plasmid 51, 246–255. doi: 10.1016/j.plasmid.2004.02.003
Popenda, M., Szachniuk, M., Antczak, M., Purzycka, K. J., Lukasiak, P., Bartol, N., et al. (2012). Automated 3D structure composition for large RNAs. Nucleic Acids Res. 40:e112. doi: 10.1093/nar/gks339
Pósfai, G., Kolisnychenko, V., Bereczki, Z., and Blattner, F. R. (1999). Markerless gene replacement in Escherichia coli stimulated by a double-strand break in the chromosome. Nucleic Acids Res. 27, 4409–4415. doi: 10.1093/nar/27.22.4409
Rasmussen, L. C. V., Sperling-Petersen, H. U., and Mortensen, K. K. (2007). Hitting bacteria at the heart of the central dogma: sequence-specific inhibition. Microb. Cell Fact. 6:24. doi: 10.1186/1475-2859-6-24
Rocha, D. J. P., Santos, C. S., and Pacheco, L. G. C. (2015). Bacterial reference genes for gene expression studies by RT-qPCR: survey and analysis. Antonie Van Leeuwenhoek 108, 685–693. doi: 10.1007/s10482-015-0524-1
Rocker, A., and Meinhart, A. (2016). Type II toxin: antitoxin systems. More than small selfish entities? Curr. Genet. 62, 287–290. doi: 10.1007/s00294-015-0541-7
Rothenbacher, F. P., Suzuki, M., Hurley, J. M., Montville, T. J., Kirn, T. J., Ouyang, M., et al. (2012). Clostridium difficile MazF toxin exhibits selective, not global, mRNA cleavage. J. Bacteriol. 194, 3464–3474. doi: 10.1128/JB.00217-12
Równicki, M., Wojciechowska, M., Wierzba, A. J., Czarnecki, J., Bartosik, D., Gryko, D., et al. (2017). Vitamin B12 as a carrier of peptide nucleic acid (PNA) into bacterial cells. Sci. Rep. 7:7644. doi: 10.1038/s41598-017-08032-8
Rozners, E. (2012). Recent advances in chemical modification of peptide nucleic acids. J. Nucleic Acids 2012:518162. doi: 10.1155/2012/518162
Schifano, J. M., Cruz, J. W., Vvedenskaya, I. O., Edifor, R., Ouyang, M., Husson, R. N., et al. (2016). tRNA is a new target for cleavage by a MazF toxin. Nucleic Acids Res. 44:gkv1370. doi: 10.1093/nar/gkv1370
Schneider, E. K., Reyes-Ortega, F., Velkov, T., and Li, J. (2017). Antibiotic–non-antibiotic combinations for combating extremely drug-resistant Gram-negative ‘superbugs’. Essays Biochem. 61, 115–125. doi: 10.1042/EBC20160058
Schumacher, M. A., Piro, K. M., Xu, W., Hansen, S., Lewis, K., and Brennan, R. G. (2009). Molecular mechanisms of HipA mediated multidrug tolerance and its neutralization by HipB. Science 323, 396–401. doi: 10.1126/science.1163806
Schuster, C. F., Park, J. H., Prax, M., Herbig, A., Nieselt, K., Rosenstein, R., et al. (2013). Characterization of a MazEF toxin-antitoxin homologue from Staphylococcus equorum. J. Bacteriol. 195, 115–125. doi: 10.1128/JB.00400-12
Shapiro, S. (2013). Speculative strategies for new antibacterials: all roads should not lead to Rome. J. Antibiot. 66, 371–386. doi: 10.1038/ja.2013.27
Sharma, P., Teymournejad, O., and Rikihisa, Y. (2017). Peptide nucleic acid knockdown and intra-host cell complementation of ehrlichia type IV secretion system effector. Front. Cell. Infect. Microbiol. 7:228. doi: 10.3389/fcimb.2017.00228
Tripathi, A., Dewan, P. C., Siddique, S. A., and Varadarajan, R. (2014). MazF-induced growth inhibition and persister generation in escherichia coli. J. Biol. Chem. 289, 4191–4205. doi: 10.1074/jbc.M113.510511
Ventola, C. L. (2015a). The antibiotic resistance crisis. Part 1: causes and threats. Pharm. Ther. 40, 277–283.
Ventola, C. L. (2015b). The antibiotic resistance crisis. Part 2: management strategies and new agents. Pharm. Ther. 40, 344–352.
Verma, S., Kumar, S., Gupta, V. P., Gourinath, S., Bhatnagar, S., and Bhatnagar, R. (2015). Structural basis of Bacillus anthracis MoxXT disruption and the modulation of MoxT ribonuclease activity by rationally designed peptides. J. Biomol. Struct. Dyn. 33, 606–624. doi: 10.1080/07391102.2014.899924
Wen, Y., Behiels, E., and Devreese, B. (2014). Toxin-antitoxin systems: their role in persistence, biofilm formation, and pathogenicity. Pathog. Dis. 70, 240–249. doi: 10.1111/2049-632X.12145
Williams, J., and Hergenrother, P. (2014). Artificial activation of toxin-antitoxin systems as an antibacterial strategy. Trends Microbiol. 20, 291–298. doi: 10.1016/j.tim.2012.02.005.Artificial
Wood, T. K., Knabel, S. J., and Kwan, B. W. (2013). Bacterial persister cell formation and dormancy. Appl. Environ. Microbiol. 79, 7116–7121. doi: 10.1128/AEM.02636-13
Yamaguchi, Y., and Inouye, M. (2009). Chapter 12 mRNA Interferases, Sequence-Specific Endoribonucleases from the Toxin-Antitoxin Systems, 1st Edn. Amsterdam: Elsevier Inc., doi: 10.1016/S0079-6603(08)00812-X
Yamaguchi, Y., Nariya, H., Park, J. H., and Inouye, M. (2012). Inhibition of specific gene expressions by protein-mediated mRNA interference. Nat. Commun. 3, 606–607. doi: 10.1038/ncomms1621
Zeng, Z., Han, S., Hong, W., Lang, Y., Li, F., Liu, Y., et al. (2016). A tat-conjugated peptide nucleic acid tat-PNA-DR inhibits hepatitis B virus replication in vitro and in vivo by targeting LTR direct repeats of HBV RNA. Mol. Ther. Nucleic Acids 5:e295. doi: 10.1038/mtna.2016.11
Keywords: antimicrobial strategies, bacterial toxin–antitoxin systems, peptide nucleic acid (PNA), antisense oligonucleotides, Escherichia coli mazEF and hipBA targets
Citation: Równicki M, Pieńko T, Czarnecki J, Kolanowska M, Bartosik D and Trylska J (2018) Artificial Activation of Escherichia coli mazEF and hipBA Toxin–Antitoxin Systems by Antisense Peptide Nucleic Acids as an Antibacterial Strategy. Front. Microbiol. 9:2870. doi: 10.3389/fmicb.2018.02870
Received: 13 September 2018; Accepted: 08 November 2018;
Published: 26 November 2018.
Edited by:
Natalia V. Kirienko, Rice University, United StatesReviewed by:
Ilana Kolodkin-Gal, Weizmann Institute of Science, IsraelChew Chieng Yeo, Sultan Zainal Abidin University, Malaysia
Copyright © 2018 Równicki, Pieńko, Czarnecki, Kolanowska, Bartosik and Trylska. This is an open-access article distributed under the terms of the Creative Commons Attribution License (CC BY). The use, distribution or reproduction in other forums is permitted, provided the original author(s) and the copyright owner(s) are credited and that the original publication in this journal is cited, in accordance with accepted academic practice. No use, distribution or reproduction is permitted which does not comply with these terms.
*Correspondence: Joanna Trylska, am9hbm5hQGNlbnQudXcuZWR1LnBs