- 1GFZ German Research Centre for Geosciences, Section Geomicrobiology, Potsdam, Germany
- 2GFZ German Research Centre for Geosciences, Section Organic Geochemistry, Potsdam, Germany
- 3Institute of Applied Geosciences, Technische Universität Berlin, Berlin, Germany
- 4Institute for Geophysics and Geology, University of Leipzig, Leipzig, Germany
- 5GFZ German Research Centre for Geosciences, Section Climate Dynamics and Landscape Evolution, Potsdam, Germany
- 6Institute of Earth and Environmental Science, University of Potsdam, Potsdam, Germany
The Cheb Basin (CZ) is a shallow Neogene intracontinental basin filled with fluvial and lacustrine sediments that is located in the western part of the Eger Rift. The basin is situated in a seismically active area and is characterized by diffuse degassing of mantle-derived CO2 in mofette fields. The Hartoušov mofette field shows a daily CO2 flux of 23–97 tons of CO2 released over an area of 0.35 km2 and a soil gas concentration of up to 100% CO2. The present study aims to explore the geo–bio interactions provoked by the influence of elevated CO2 concentrations on the geochemistry and microbial community of soils and sediments. To sample the strata, two 3-m cores were recovered. One core stems from the center of the degassing structure, whereas the other core was taken 8 m from the ENE and served as an undisturbed reference site. The sites were compared regarding their geochemical features, microbial abundances, and microbial community structures. The mofette site is characterized by a low pH and high TOC/sulfate contents. Striking differences in the microbial community highlight the substantial impact of elevated CO2 concentrations and their associated side effects on microbial processes. The abundance of microbes did not show a typical decrease with depth, indicating that the uprising CO2-rich fluid provides sufficient substrate for chemolithoautotrophic anaerobic microorganisms. Illumina MiSeq sequencing of the 16S rRNA genes and multivariate statistics reveals that the pH strongly influences microbial composition and explains around 38.7% of the variance at the mofette site and 22.4% of the variance between the mofette site and the undisturbed reference site. Accordingly, acidophilic microorganisms (e.g., OTUs assigned to Acidobacteriaceae and Acidithiobacillus) displayed a much higher relative abundance at the mofette site than at the reference site. The microbial community at the mofette site is characterized by a high relative abundance of methanogens and taxa involved in sulfur cycling. The present study provides intriguing insights into microbial life and geo–bio interactions in an active seismic region dominated by emanating mantle-derived CO2-rich fluids, and thereby builds the basis for further studies, e.g., focusing on the functional repertoire of the communities. However, it remains open if the observed patterns can be generalized for different time-points or sites.
Introduction
Due to magmatic activity beneath the Cheb Basin, large-scale degassing of mantle-derived CO2 (>99%) occurs. The diffuse cold gas emanations at the surface (diffuse degassing structures, DDS) can be distinguished as dry and wet mofettes (Kämpf et al., 2013). Mofettes provide insights into life under elevated CO2 concentrations, low pH, and anoxic conditions comparable to the Earth’s ancient atmosphere (Emiliani, 1992; Raven, 1995; Young et al., 2012). Moreover, mofettes are used as model ecosystems for studying the response of soil microorganisms to a potential CO2 leakage of underground carbon capture and storage systems (Krüger et al., 2009, 2011; Frerichs et al., 2013; Morales and Holben, 2013).
CO2 degassing leads to hypoxia and acidification of the soil (Beaubien et al., 2008; Blume and Felix-Henningsen, 2009; Rennert and Pfanz, 2016). Additionally, an increase in metal mobilization was observed, which may affect the availability of soil nutrients (Mehlhorn et al., 2014, 2016). As shown by several studies, these direct influences of elevated CO2 concentrations on the environment are affecting the mofette biota and its biological matter cycling.
First studies on the effects of CO2 on the soil biota were focused on the plant vegetation. These studies point to a decelerated growth, an increased plant C/N ratio but also physiological adaptations that provide advantages in hypoxic or even anoxic environments (Pfanz et al., 2004; Vodnik et al., 2007; Rennert and Pfanz, 2016). A common feature of mofette soils are increased carbon and nitrogen contents (Ross et al., 2000; Rennert et al., 2011). By δ13C analyses of plant and microbial lipids, Oppermann et al. (2010) demonstrated that within a CO2 vent in the Latera Caldera (Central Italy) a substantial amount of geothermal CO2 is incorporated into the microbial, plant, and soil carbon pools. Recently, Beulig et al. (2016) performed radiocarbon analyses and showed that up to 67% of mofette soil carbon content originated from the assimilation of geogenic CO2 via plant primary production and microbial CO2 fixation. The authors pointed out that the almost undegraded organic material found in the mofette soil is facilitated by the permanent exclusion of meso- to macroscopic eukaryotes rather than an impaired biochemical potential for soil organic matter decomposition. Complementary, Nowak et al. (2015) estimated through combined δ14C and δ13C isotope mass balances that around 8–27% of the bulk soil organic matter (SOM) derived from microorganisms. DNA stable isotope probing allowed the identification of chemolithoautotrophic microorganisms such as methanogenic archaea and acetogens as well as sulfate reducing bacteria (SRB) to be involved in the assimilation of CO2 (Oppermann et al., 2010; Beulig et al., 2015). However, a quantification of cbbL genes, encoding for the large subunit of RuBisCO, a carboxylase which is of crucial importance for carbon assimilation in chemolithoautotrophic microbes, revealed that only a part of the autotrophic CO2-fixing microorganisms could adapt to the very high CO2 concentrations found in a mofette in Slovenia (Videmšek et al., 2009).
In the last decade, several studies have focused on the impact of elevated CO2 concentrations on the microbial community structure in mofettes. A shift to anaerobic/microaerophilic and acidophilic community compositions has been reported in CO2 mofette soils located near the Laacher See (Germany) (Krüger et al., 2009; Krüger et al., 2011), the Cheb Basin (Czech Republic) (Beulig et al., 2015), and in Stavešinci (Slovenia) (Videmšek et al., 2009). Furthermore, at the Laacher See site, the abundance of several functional and group-specific gene markers revealed a decrease in Geobacteraceae and an increase in SRBs in the vent center and biomarker analysis revealed a predominance of Thaumarchaeota as possible indicator organisms for elevated CO2 concentrations in soils (Frerichs et al., 2013). Also for a mofette in Latera, Italy, it was shown that strictly anaerobic SRBs are abundant in mofettes, whereby the ATP biomass and total bacterial cell counts decreased (Beaubien et al., 2008). The highest sulfate reducing activity was observed in the center of the vent. Also Methanogenic archaea showed higher activities in the center of the vent compared to a transition zone site. Contrary to the results from Frerichs et al. (2013) in the Laacher See mofette, Geobacteraceae were in Latera mainly found at the CO2 vent and only minor quantities were found at the reference site (Oppermann et al., 2010).
So far, only a few population datasets from dry mofettes are based on high throughput sequencing. The 454 pyrosequencing analyses of 16S rRNA genes from a natural mofette in central-southern Spain revealed that community richness, evenness, and diversity decreased with increasing CO2 flux (Sáenz de Miera et al., 2014). An increase in abundance was thereby observed for OTUs related to the Chloroflexi phylum. Interestingly, Beulig et al. (2015) also showed that besides Methanoregulaceae, unclassified Chloroflexi might be involved in acetogenesis by DNA-SIP. The second 16S rRNA pyrosequencing dataset was established by Beulig et al. (2015) for a mofette located in the Plesná floodplain in the Cheb Basin. The community was dominated by methanogens (e.g., Methanosarcinales and Methanomicrobiales) and subdivision 1 Acidobacteria, which likely thrived under stable hypoxia and acidic pH. Recently, Krauze et al. (2017) investigated wet mofettes in the Cheb Basin by Illumina 16S rRNA amplicon sequencing and found a unique microbial community highly adapted to the anoxic conditions in the mineral and thermal waters and highlighted the connection between the groundwater or mineral waters and the deep biosphere. Deeper insights into the influence of CO2 on the microbial community within the top 40 cm of a mofette in the Cheb Basin were gained by a metatranscriptomic and metagenomic approach (Beulig et al., 2016). One outcome of the study was that transcripts related to methanogenesis (mcr) and sulfate reduction (dsr, cys, apr) were remarkably increased in frequency.
However, until now, microbiological studies have focused only on near-surface soil layers (<70 cm) of dry mofettes, and deeper sediments have not yet been analyzed in detail. The present study aims to characterize the influence of elevated CO2 concentrations inside a CO2 conduit on the geochemistry, microbial abundance, and community composition. Furthermore, this study intends to determine significant community-shaping environmental factors. To study the influence of mantle-derived CO2, two 3-m drillings were performed, one of which was located in the center of the DDS and the other at the undisturbed border of the mofette field. To unravel the community structures a high resolution sampling (every 5 to 10 cm) and Illumina 16S rRNA gene amplicon sequencing was conducted.
Materials and Methods
Site Selection, Description, and Sampling
The drilling campaign was conducted in September 2015 in the Hartoušov Mofette Field (HMF; 50°07′58′′N, 12°27′46′′E; Figure 1B). The study site is located in the Cheb Basin (NW Bohemia, Czechia), a shallow Neogene intracontinental basin filled with fluvial and lacustrine sediments (≤350 m thick; Figure 1A). The basin formed at the intersection of the Eger Rift (Kopecký, 1979) and the Regensburg–Leipzig–Rostock fault zone (Bankwitz et al., 2003b; Geissler et al., 2004) where four Quaternary volcanoes existed (Mrlina et al., 2009; Rohrmüller et al., 2018). The western Eger Rift has been well studied regarding structure of the lithosphere, seismic activity, sedimentology, and fault characteristics (Dobeš et al., 1986; Bucha, et al, 1990; Špičáková et al., 2000; Bankwitz et al., 2003a; Kämpf et al., 2005, 2007, 2013; Flechsig et al., 2008; Fischer et al., 2014; Nickschick et al., 2015; Bussert et al., 2017). The seismic activity in this area occurs as “earthquake swarms,” which are typically numerous small earthquakes at upper crustal depths that cluster in time and space (Fischer et al., 2014). Earthquake swarms usually occur in volcanic areas, geothermal fields, and ocean ridges, whereas intraplate earthquake swarms that are not connected to active volcanism are present in continental rifts, such as the Rio Grande Rift, the Kenya Rift, and the western Eger Rift (Ibs-von Seht et al., 2008). Due to magmatic activity beneath the Cheb Basin, large-scale degassing of mantle-derived CO2 (>99%) occurs, and traces of gases such as He, N2, Ar, and CH4 are emitted (Weinlich et al., 1999; Kämpf et al., 2013; Hrubcová et al., 2017; Bräuer et al., 2018). The gas migrates through the upper lithospheric mantle and the crust to the surface and mixes with water of deep thermal and shallow groundwater aquifers (Bussert et al., 2017). Mofettes are local degassing phenomena that often occur as larger DDS on the scale of up to a few square kilometers. They are controlled by fluid migration in fault zones (Girault and Perrier, 2014; Nickschick et al., 2015) or in volcano-hydrothermal areas (Chiodini et al., 2008; Girault et al., 2014; Inguaggiato et al., 2017). The investigated dry mofette is part of the Hartoušov mofette field (HMF or DDS Hartoušov) according to Kämpf et al. (2013), which covers an area of approximately 350,000 m2 of grassland with two small ponds (2–6m2) close to the river Plesná. A total of 23–97 tons of CO2 have been estimated to be released daily in the HMF (Figure 1B; Nickschick et al., 2015).
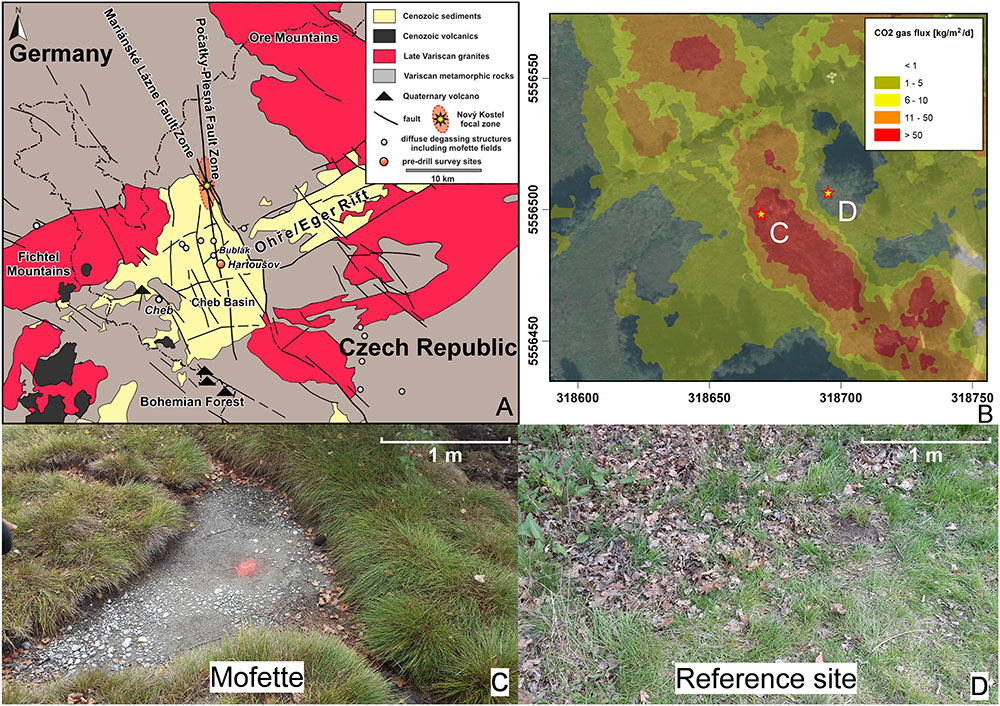
FIGURE 1. Geological map of the Cheb Basin (modified from Flechsig et al., 2008) (A), CO2 gas flux in the Hartoušov mofette field (modified from Nickschick et al., 2015, coordinates in UTM 33N) (B), surface at the mofette (C) and the reference site (D). The drilling locations are marked by stars (C = mofette site; D = reference site) in the CO2 gas flux map (B).
The drilling sites were located along a NW-SE-oriented profile that is perpendicular to one of the main degassing areas of the HMF (Figure 1B). The CO2 soil gas flux was repeatedly measured in June, August, September, and October 2012 along a 55-m profile that consisted of 11 stations (P1 to P11) with 5 m between each of them (Nickschick et al., 2015). The CO2 soil gas flux measurements were performed by the accumulation chamber method, which uses a LiCOR 820 infrared gas analyzer for CO2 discharge quantification and two accumulation chambers (West Systems, Italy). The profile encompasses low, medium, and high CO2 soil degassing spots at the soil surface with strong degassing in the center of the profile (Nickschick et al., 2015). Places of high CO2 soil gas flux form small hummocks (Flechsig et al., 2008).
Two 3-m cores were retrieved by hammered drilling using a motor-driven hammer (Wacker Neuson, Germany). The cores were taken from a dry mofette near station P6 and an undisturbed reference site in the direct vicinity of station P2 (Nickschick et al., 2015; Supplementary Table S1 and Figures 1C,D). The mean CO2 soil gas flux of the mofette amounted to 27,961.6 g m-2 per day, while the CO2 soil gas flux of the reference site amounted to 8.1 g m-2 per day (Nickschick et al., 2015). At the mofette site, which has a size of about 2 m2, the growth of vegetation is hindered by continual CO2 degassing (Figure 1C). A pond about 4 m away from the sampled dry mofette is irregularly filled with groundwater or meteoric water (Supplementary Figure S1).
Each core was subsampled in technical triplicates in the topmost 1 m in intervals of 5 cm and below in intervals of 10 cm. The inner part of the core material was subsampled using an ethanol flamed spatula. The core material for molecular biological analyses was immediately stored at -20°C after subsampling.
Geochemical Analysis
Pore water content in the sediment samples was too low to gain a sufficient amount of water for ion chromatographic analyses; therefore, a leaching procedure was applied in accordance with (Blume et al., 2011). Five grams of sediment were suspended in 25 mL of freshly autoclaved deionized water, shaken for 90 min in an anaerobic workstation (Don Whitley Scientific Limited, West Yorkshire, United Kingdom), and then centrifuged using airtight centrifuge tubes to remove solids. The water content of the fresh sediment was calculated from the difference in weight after being dried at 75°C for 2 days. The pH and the conductivity of the pore water were analyzed with a Multi 3420 SET G digital measuring instrument (WTW, Weilheim, Germany).
Total organic carbon (TOC) and δCorg values were measured using an elemental analyzer (NC 2500 Carlo Erba) coupled with a ConFlowIII interface on a DeltaPlusXL mass spectrometer (Thermo Fischer Scientific). Around 3 mg of sample material were weighed in unfolded Ag-capsules, added with 20% HCl, heated for 3 h at 75°C, enfolded in the Ag-capsules, and measured. The calibration of δCorg was performed by certified isotope standards (USGS24, CH-7) and proofed by an internal soil reference sample (Boden3). The isotopic composition is given in δCorg notation relative to a standard: δ(‰) = [(Rsample–Rstandard)/Rstandard] × 1000. The ratio (R) and standard for carbon is 13C/12C and VPDB (Vienna PeeDee Belemnite).
The cation and anion concentrations in leached pore water were analyzed using ion chromatography (IC) (Sykam Chromatography, Eresing, Germany) according to Vuillemin et al. (2016) protocol. For cations, the IC system consisted of a S5300 sample injector (Sykam), a 4.6 mm × 200 mm ReproSil CAT column (Dr. Maisch HPLC, Ammerbuch-Entringen, Germany), and a S3115 conductivity detector (Sykam). The eluent was 5 mM H2SO4, and the eluent flow rate was set at 1 mL min-1. The column oven temperature was 45°C. A Cation Multi-Element IC-standard (Carl Roth) was diluted ten times for calibration. Samples and standards were measured in technical triplicates. For anions, the suppressed IC system consisted of a SeQuant SAMS anion IC suppressor (EMD Millipore, Billerica, MA, United States), a S5200 sample injector, a 3.0 mm × 250 mm lithocholic acid (LCA) 14 column, and a S3115 conductivity detector (all Sykam). The eluent was 5 mM Na2CO3 with 20 mg L-1 4-hydroxybenzonitrile and 0.2% methanol. The eluent flow rate was set at 1 mL min-1, and the column oven temperature was set at 50°C. A multi-element anion standard (Sykam) was diluted ten times for calibration. Samples and standards were measured in technical triplicates.
DNA Extraction and Purification
The total genomic DNA was isolated by the FastDNATM SPIN Kit for soil and the FastPrep®Instrument (MP Biomedicals, Santa Ana, CA, United States) with some protocol modifications. The FastPrep® Instrument homogenizing time was set to 30 s, and the speed was set to 5.5 m s-1. The mixing time of Binding Matrix and DNA crude extract solution was extended to 20 min. The Genomic DNA Clean & ConcentratorTM-10 (Zymo Research, Irvine, CA, United States) was utilized to remove humic acids and other substances that may have inhibited the PCR reaction. Three DNA isolations were extracted from each of the 44 sediment samples (22 samples from each core) as technical triplicates. In total, 132 samples were processed.
Quantitative PCR
The total bacterial abundance (16S rRNA gene) and the functional genes of sulfate-reducing bacteria (SRB) (dsrB gene) and methanogenic archaea (mcrA gene) were determined by a quantitative polymerase chain reaction (qPCR). The qPCR Master Mix consisted of 12.5 μl iTaqTM Universal SYBR® Green Supermix (Thermo Fisher Scientific Inc., United States), 8.5 μl PCR water, 0.5 μl forward primer (20 μM), 0.5 μl reverse primer (20 μM), and 3 μl template. The quantification of the bacterial 16S rRNA gene was based on the primer pair of 331F (5′-TCCTACGGGAGGCAG-CAGT-3′) and 797R (5′-GGACTACCAGGGTATCTAATCCTGTT-3′) (Lane, 1991) and followed the protocol of 5 min at 98°C, 40 cycles of 5 s at 98°C, 20 s at 57°C, and 60 s at 72°C. The cloned 16S rRNA gene fragment from E. coli was used as standard. The qPCR efficiency for the 16S rRNA gene quantification was 90.2% and the R2-value of the standard curve line was 0.996. The quantification of the dsrB gene was based on the primer pair of dsr2060F (5′-CAACATCGTYCAYACCCAGGG-3′) and dsr4R (5′-GTGTAGCAGTTACCGCA-3′) (Ben-Dov et al., 2007) and followed the protocol of 10 min at 95°C, 40 cycles of 30 s at 95°C, 60 s at 60°C, 60 s at 72°C. The cloned dsrB gene fragment of Desulfovibrio vulgaris was used as standard. The qPCR efficiency for the dsrB gene quantification was 93.4% and the R2-value of the standard curve line was 0.999. The quantification of the mcrA gene was based on the primer pair of mlas-F (5′-GGTGGTGTMGGDTTCACMCARTA-3′) and mcrA-R (5′-CGTTCATBGCGTAGTTVGGRTAGT-3′) (Steinberg and Regan, 2009) and followed the protocol of 3 min at 95°C, 40 cycles of 5 s at 95°C, 20 s at 58.5°C, 30 s at 72°C, and 3 s at 80°C. The cloned mcrA gene fragment of Methanosarcina barkeri was used as standard. The qPCR efficiency for the mcrA gene quantification was 97.7% and the R2-value of the standard curve line was 0.997.
The qPCR was conducted on a CFX96 real-time thermal cycler (Bio-Rad Laboratories Inc., United States), and the analysis of the quantification data was performed with the CFX ManagerTM software (Bio-Rad Laboratories Inc., United States).
Illumina MiSeq Amplicon Sequencing
The 16S rRNA gene amplified from extracted total genomic DNA was used as a template for the Illumina MiSeq high-throughput sequencing. The PCR reaction solution consisted of 12.5 μl MangoMixTM (Bioline, Taunton, United States), 9.2 μl PCR water, 0.3 μl bovine serum albumin, 0.25 μl forward primer (20 μM), 0.25 μl reverse primer (20 μM), and 2.5 μl template. Unique combinations of barcode-tagged 515F (5′-GTGCCAGCMGCCGCGGTAA-3′) and 806R (5′-GGACTACHVGGGTWTCTAAT-3′) (Caporaso et al., 2011) primers were assigned to each sample. The amplifications were performed on a T100 thermal cycler (Bio-Rad Laboratories Inc., United States) and followed the protocol of 3 min at 95°C, 30 cycles of 30 s at 94°C, 45 s at 56°C, 60 s at 72°C, and a final extension step of 10 min at 72°C. The PCR products were cleaned up with AMPure XP magnetic beads (Beckman Coulter GmbH, Krefeld, Germany). After measuring the DNA concentration (CLARIO star® plate reader, BMG LABTECH GmbH, Ortenberg, Germany), PCR products were pooled in equimolar amounts. The DNA pool was concentrated (Eppendorf Concentrator plus, Eppendorf AG, Hamburg, Germany) to meet the requirement of sequencing (DNA concentration ≥50 ng μl-1). It should be noted that the used MangoMixTM (Bioline, Taunton, United States) does not supply a proof-reading polymerase, which may inflate species richness and interfere with recovery of certain genotypes (Brandariz-Fontes et al., 2015). However, Pereira et al. (2018) showed that the choice of DNA polymerase did not significantly change the community profiling and composition.
Bioinformatics and Statistical Analysis
Sequencing was performed by Eurofins Scientific SE, Luxembourg, on an Illumina MiSeq (2 × 250 bp). Read pairs were merged using PEAR (Zhang et al., 2014). QIIME (Version 1.9.1; Caporaso et al., 2010) was employed for microbiome analysis. More specifically, reads were demultiplexed, and USEARCH (Edgar, 2010) was used for the detection and removal of chimeric sequences. The SILVA database (Version 128; DeSantis et al., 2006) was utilized for open-reference OTU clustering (97% sequence similarity) and taxonomic assignments. Rational taxonomic boundaries have been proposed for the high taxa (that is, genus and above) of the Bacteria and the Archaea on the basis of 16S rRNA gene sequence identities. These are: 94.5% for genus, 86.5% for family, 82.0% for order, 78.5% for class, and 75.0% for phylum (Yarza et al., 2014). Within in this study the taxonomical data was discussed on genus-level and above. Singletons and OTUs assigned to chloroplasts were removed. The data received for the technical triplicates were merged for the downstream analyses. For alpha diversity analyses, the data were rarefied to 14,528 reads per sample. Alpha diversity and evenness were analyzed using the Shannon H index and the Shannon EH index. Beta diversity (PCoA) was determined by calculating the weighted UniFrac distance metric (QIIME), and samples from different depths were clustered and illustrated by 95% confidence ellipses. For multivariate statistics (including canonical correlation analysis, CCA), CANOCO 5 (Šmilauer and Lepš, 2014) and PAST3 (Hammer et al., 2001) were used. Sequencing data were submitted to the European Nucleotide Archive1 under accession numbers ERS 2039641 to ERS 2039772 (Bioproject PRJEB22478).
Results
Stratigraphy and Geochemical Characterization
The reference core consisted primarily of fine- to medium-grained clayey sand that contained dispersed iron mottles (Figure 2A). A gravel-rich sand layer was reached at a depth of 80 to 100 cm, which roughly represented the groundwater level. The mofette core was dominated by humus or peaty sand and occasional by sandy peat. Clay or clayey sand occurred primarily in the topmost 20 cm. In the mofette field, the groundwater level was shallower (25–70 cm) than at the reference site. The very shallow groundwater level was also reflected in the high water content of the sediment (Figure 2B).
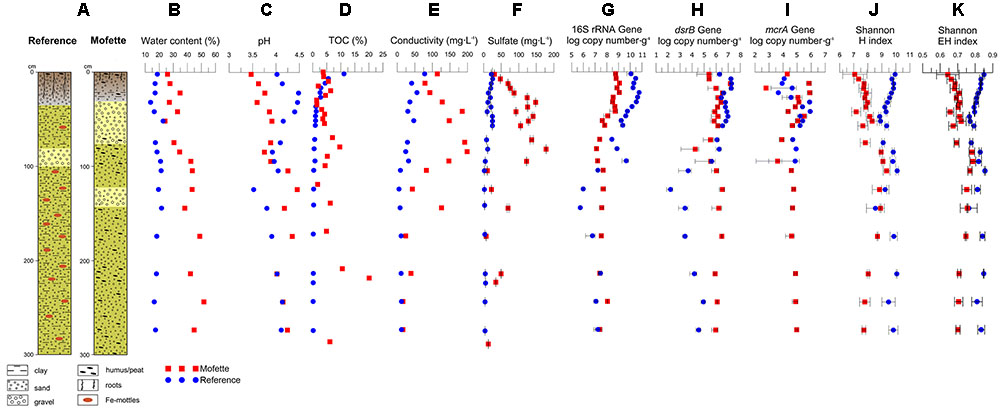
FIGURE 2. Lithological profile of the reference and mofette core (A). The water content (B), pH (C), TOC (D), conductivity (E), sulfate concentration (F), bacterial 16s rRNA gene copy number (G), dsrB gene copy number (H), mcrA gene copy number (I), Shannon H index (J) and Shannon EH index (K) of reference (blue dots) and mofette (red squares) core.
Ion concentrations, conductivity, and pH were obtained by sediment leaching (Figures 2C,E,F). The complete spectrum of measured anions, cations, and δCorg values is provided in the Supplementary Tables S1, S2. The concentrations of sulfur, nitrate, and nitrite were under detection limit. The pH values in the mofette core increased from around 3.5–4.3 down to a depth of 105 cm and varied from 4.0 to 4.6 between 105 and 275 cm in depth. In contrast, the pH values of the reference core ranged from 3.5 to 4.5 without displaying a clear trend. The conductivity of the mofette sediment was substantially higher compared with the reference core. Conductivity in the mofette site increased from 114 mg L-1 at the surface to 199 mg L-1 at a depth of 85 cm and decreased downward to 16 mg L-1 at a depth of 275 cm. A conductivity peak of 126 mg L-1 was measured at a depth of 145 cm. In the reference core, the conductivity decreased from top to bottom from 78 mg L-1 to 12 mg L-1.
The TOC content of the reference site decreased from 11.1% in the topsoil to 0.2% in the deepest sample at 275 cm in depth. The mofette site was characterized by a generally higher TOC content that varied significantly in the organic-rich peat layers (Figure 2D). The highest TOC contents were present at a depth of 224 cm (20.1%) and below the groundwater table at a depth of 82 cm (9.6%). δCorg values were in the range of -25.3 to -28.5‰, and showed no significant differences between mofette and reference site.
In comparison with the reference core, sulfate concentrations were higher across the entire mofette core (Figure 2F). The sulfate most likely stems primarily from the oxidation of pyrite, which at Hartoušov (Flechsig et al., 2008) proved to be abundant in the mofette sites. The highest sulfate concentrations were measured in the top 65 cm of the reference (up to 25.74 mg L-1) and in the top 100 cm of the mofette core (up to 177.92 mg L-1). Sulfate concentrations were ten times higher than other measured anion and cation concentrations in the mofette and had a strong positive correlation with conductivity (R = 0.8019, p < 10-5). Therefore, the high sulfate concentrations seem to be the main reason for the high conductivity.
Abundance of Microorganisms
The bacterial abundance (16S rRNA gene copy numbers) in the mofette core decreased within the upper 50 cm (Figure 2G). However, no decrease in abundance was observed below 50 cm in sediment depth. From 0 to 100 cm in sediment depth, the gene copy numbers of the reference core were one order of magnitude higher than those of the mofette core. The highest bacterial abundance was analyzed at a depth of 12 cm in the mofette core (5.8 × 1010 gene copies g-1 sediment) and at 22 cm in the reference core (1.2 × 109 gene copies g-1 sediment). From 100 to 280 cm in sediment depth, the gene copy numbers in the mofette core partially exceeded those of the reference site.
The abundance of SRB was estimated through the quantification of dsrB gene copies (Figure 2H). The dsrB gene copy numbers in the reference core followed a similar trend to that of the respective 16S rRNA gene copy numbers. The highest dsrB gene copy number (1.9 × 107 gene copies g-1 sediment) was measured at a depth of 12 cm, and the lowest (1.6 × 102 gene copies g-1 sediment) was measured at 125 cm. The dsrB gene copy numbers in the mofette core decreased with depth (1.5 × 107 to 1.8 × 104 gene copies g-1 sediment).
The abundance of methanogens was estimated via the quantification of mcrA gene copies (Figure 2I). Between a depth of 0 and 100 cm, the mcrA gene copy numbers of both the mofette site and the reference site varied in the range of 0 and 8.5 × 105 gene copies g-1 of sediment. No mcrA genes were detected at depths greater than 100 cm at the reference site; however, mcrA gene copy numbers in the mofette site slightly increased with depth (3.9 × 104 gene copies g-1 sediment at a depth of 105 cm to 9.3 × 104 gene copies g-1 sediment at a depth of 275 cm).
Community Structure
In total 10,201,992 sequences were obtained in the 16S rRNA gene library after merging, demultiplexing, filtering, and excluding of chimeric sequences, chloroplast-like sequences, and singletons. The read numbers ranged from between 21,143 and 160,896, with a mean value of 77,287 (Supplementary Table S3). Rarefaction analyses revealed that no sample exhibited a conspicuous increase in its Shannon H index when calculating more than 14,528 sequences per sample (Supplementary Table S4).
Except for the section of sediment between 85 and 145 cm in depth, the reference site showed a higher alpha diversity (Figure 2J and Supplementary Table S4). The alpha diversities of the mofette increased with depth from 0 to 85 cm, remained constant from 85 cm to 145 cm, and then decreased toward the end of the profile. The Shannon index of the mofette displayed a weak positive correlation with pH (R = 0.582, p = 0.0045) and water content (R = 0.497, p = 0.0187) (Supplementary Tables S5, S6). The Shannon EH equitability index was lower throughout the depth sequence at the mofette than at the reference site (Figure 2K), especially at the surface layers and the deepest part.
The relative abundance of each taxon is displayed by the percentage of total sequence reads (Supplementary Table S7). The dominant phyla at both the mofette site and the reference site were Acidobacteria (mofette: 20.4%; reference: 25.6%), Actinobacteria (mofette: 6.7%; reference: 10.9%), Chloroflexi (mofette: 9.4%; reference: 15.8%), Firmicutes (mofette: 14.9%; reference: 4.8%), and Proteobacteria (mofette: 24.9%; reference: 23.7%). The dominant archaeal phyla were Bathyarchaeota (mofette: 1.8%; reference: 1.7%), Euryarchaeota (mofette: <0.1%; reference: 0.4%), and Thaumarchaeota (mofette: 0.3%; reference: 1.0%). Moreover, a larger fraction of unassigned taxa were present in the mofette sediments (4.0%) compared with in the reference sediments (1.0%; Figure 3).
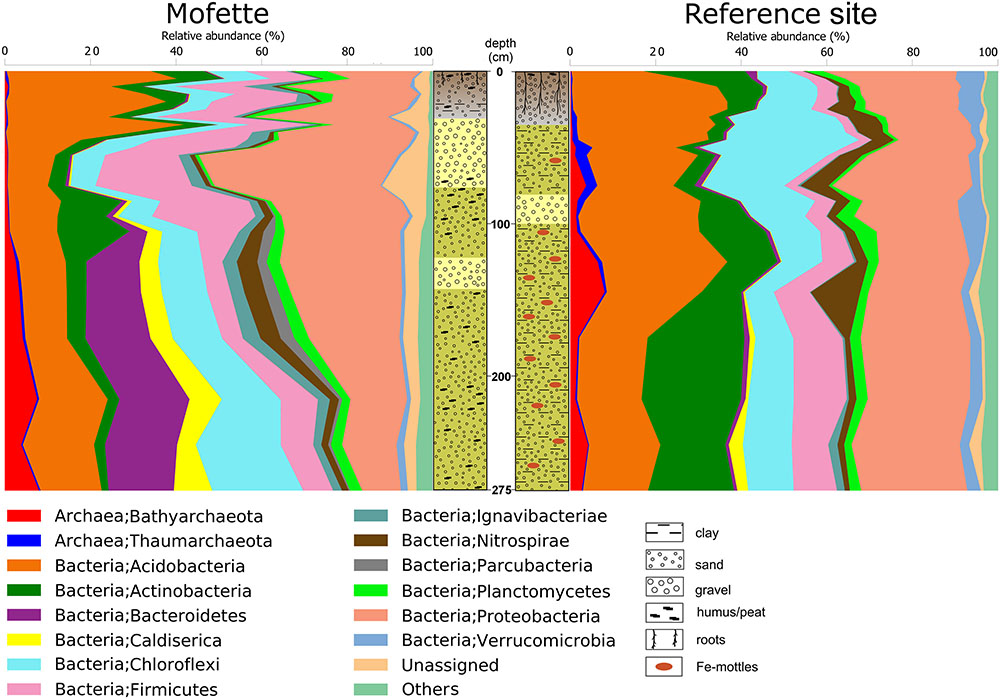
FIGURE 3. Community structures at phylum level (most abundant 14 phyla) and the lithological profiles of the cores.
Beta diversities were obtained by calculating a weighted UniFrac distance metric (Figure 4). A distinct clustering of the microbial communities was observed for both sites. The microbial community structure of the upper sediment (0–95 cm) of the mofette was distinct from the deeper part of the core (100–275 cm), and we therefore defined two distinct clusters. Cluster A includes communities from 0 to 95 cm in depth, and Cluster B includes communities observed at depths between 100 and 275 cm.
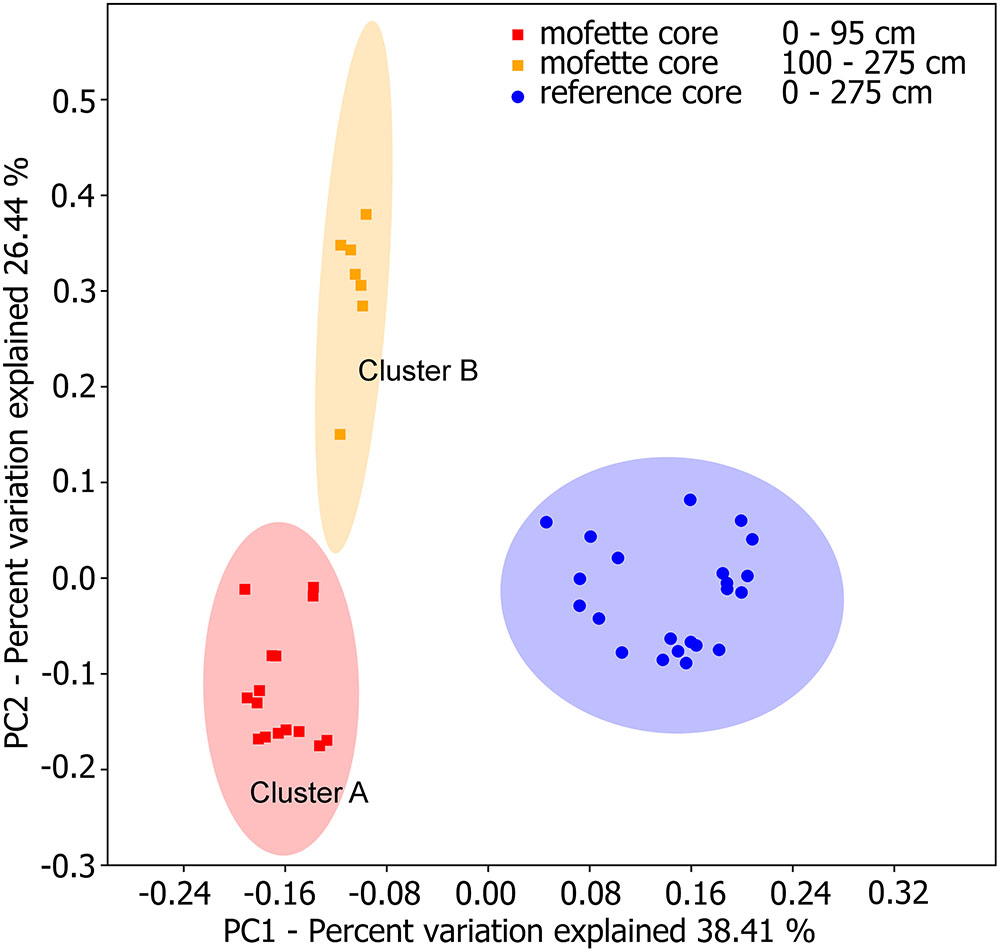
FIGURE 4. PCoA plot calculated by the weighted UniFrac distance of the microbial communities. Mofette samples are indicated by red and orange squares; reference site samples by blue dots. The 95% confidence ellipses indicate three different clusters.
At a depth of 0–100 cm (Cluster A; Supplementary Table S8), the dominant taxa were Acidobacteriaceae (Subgroup 1) (mofette: 12.4%; reference: 10.5%) – which contributed to 5.1% of the dissimilarity – followed by the genera Acidithiobacillus (mofette: 7.1%; reference: 0.5%) – which contributed to 4.6% of the dissimilarity (Figure 5A). At a depth of 100–275 cm (Cluster B; Supplementary Table S9), where the influence of the uprising CO2 was stronger than in the atmospheric- and groundwater-influenced upper part of the depth sequence, significant differences in the community structures were observed (Figure 3). Acidothermus (mofette: 0.5%; reference: 6.8%) and Acidobacteriaceae (Subgroup 1) (mofette: 2.0%; reference: 8.3%) were much more abundant in the reference site, and Sulfurovum (mofette: 4.2%; reference: 0.1%), Anaerolineaceae (mofette: 5.1%; reference: 2.0%), Caldisericum (mofette: 5.5%; reference: 1.2%), Desulfobacca (mofette: 5.7%; reference: 1.4%), Thermoanaerobaculum (mofette: 6.5%; reference: 0.3%), and Bacteroidetes vadin HA17 (mofette: 4.1%; reference: 0.1%) were more abundant in the mofette (Figure 5B).
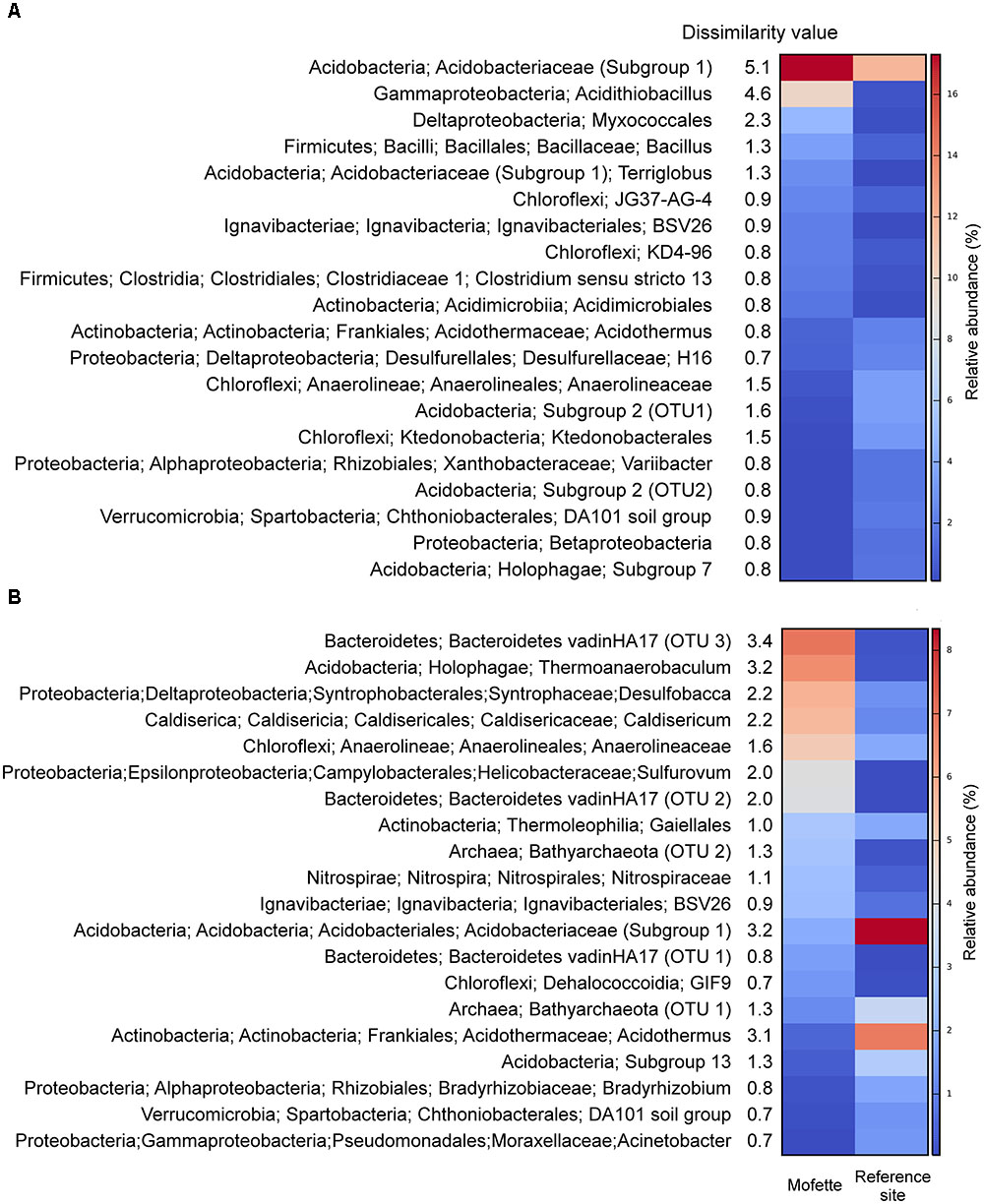
FIGURE 5. Heatmap of the top 20 taxa which explain most of the dissimilarity between the microbial communities of the mofette and the reference site based on the Bray–Curtis dissimilarity values. Cluster A (depths between 0 and 95 cm) (A) and Cluster B (depths between 100 and 275 cm) (B).
In the mofette site, microorganisms potentially involved in sulfur cycling were far more abundant (relative to the entire community) and comprised up to 14.4% of the sequence reads. In contrast, only 2.5% of the sequence reads were involved in sulfur cycling at the reference site. Observed taxa involved in sulfur cycling were sulfide/sulfur oxidizer Acidithiobacillus (mofette: 7.1%; reference: 0.5%) (Kelly and Wood, 2000), Sulfuriferula (mofette: 1.1%; reference: 0.1%) (Watanabe et al., 2015), Sulfurovum (mofette: 1.5%; reference: 0.1%) (Inagaki et al., 2004), SRB Desulfobacca (mofette: 3.1%; reference: 0.9%) (Oude Elferink et al., 1999), and Desulfosporosinus (mofette: 0.8%; reference: 0.2%) (Stackebrandt et al., 1997; Figure 6A). Taxa involved in iron cycling were less abundant in the mofette (1.1%) than at the reference site (1.2%). The iron cycling-related taxa consisted of extremely acidophilic iron-oxidizer Ferrithrix (mofette: 0.18%; reference: 0.01%) (Johnson et al., 2009), acidophilic iron-oxidizer Ferrovum (mofette: 0.72%; reference: 0.05%) (Johnson et al., 2014), Gallionella (mofette: <0.01%; reference: 0.04%) (Hallbeck and Pedersen, 1990), and the aerobic iron-oxidizer Sideroxydans (mofette: 0.20%; reference: 1.14%) (Emerson and Moyer, 1997; Figure 6B). Methanogens were found in a relatively low proportion at both the mofette site and the reference site (0.04 and 0.02%). Uncultured taxa from Rice Cluster II (Großkopf et al., 1998), Methanobacterium (Smith et al., 1997), Methanosaeta (Patel and Sprott, 1990), and Methanosarcina (Zinder et al., 1985) were the most abundant genera and were proportionally more common in the mofette microbial community (0.02, 0.01, 0.01, and <0.01%) in comparison with those of the reference site (<0.01%; Figure 6C).
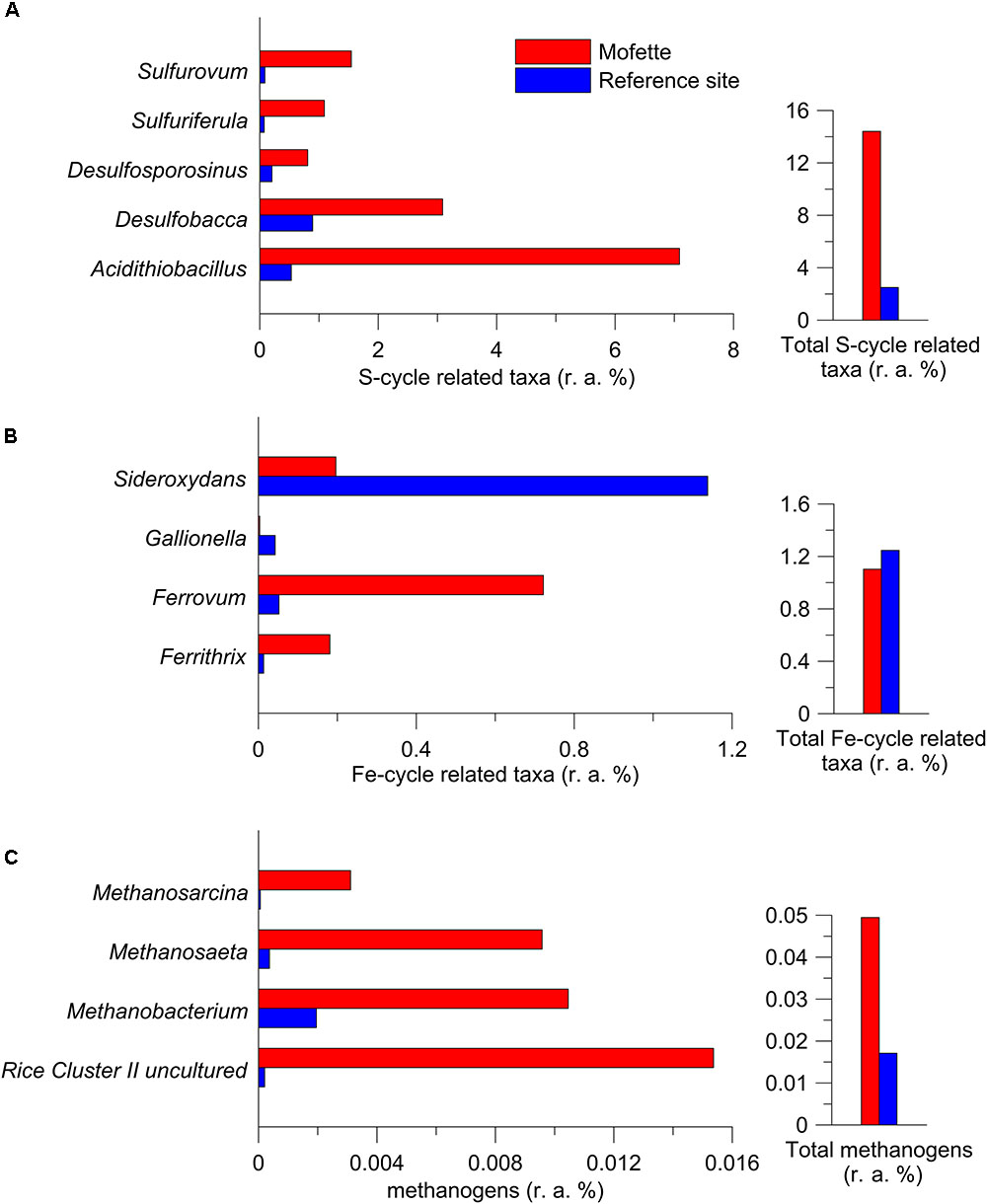
FIGURE 6. Relative abundance (r. a.) of the most abundant taxa and the total fraction involved in the sulfur-cycle (A), iron-cycle (B), and methanogenesis (C).
The mofette and the reference site shared 1,626 taxa, and a small fraction of taxa only occurred in the mofette (138) or the reference site (128). At depths of between 100 and 275 cm, 1,336 taxa were shared, but 184 and 198 taxa were only observed in the mofette and at the reference site, respectively. A total of 1,045 taxa were detected in the deeper part (200–275 cm) of the mofette and the reference site, 124 taxa were detected only at the mofette, and 330 taxa were only detected at the reference site (Supplementary Table S10).
Interestingly, methanogenic archaea – such as Methanosphaerula – were only found in the mofette. Methanoregula was not observed at the reference site between depths of 100–275 cm. Methanosaeta and Methanosarcina were not observed in the deep layers (200–275 cm) of the reference site.
Multivariate Statistics
Canonical-correlation analysis was used to determine community-shaping environmental factors (Figure 7). Among all measured environmental parameters, only those with significant padj-values (Bonferroni corrected <0.05) were included in the analyses (Supplementary Table S11). pH, sulfate concentration, and TOC formed the optimal subset of parameters to explain the observed OTU distribution. The pH value explains 38.7% of the distribution pattern of taxa at the mofette site (Figure 7A) and 16.3% at the reference site (Figure 7B). Sulfate concentration explains 12.7% of the distribution pattern of microorganisms at the mofette (Figure 7A) and 8.4% at the reference site (Figure 7B). The TOC values explain 11.4% of the distribution pattern at the reference site (Figure 7B) and 4.5% at the mofette (Figure 7A). The differences in the community structure of the mofette and the reference site are mainly explained by pH (22.4%), sulfate concentration (10.6%), and TOC (9.9%) (Figure 7C).
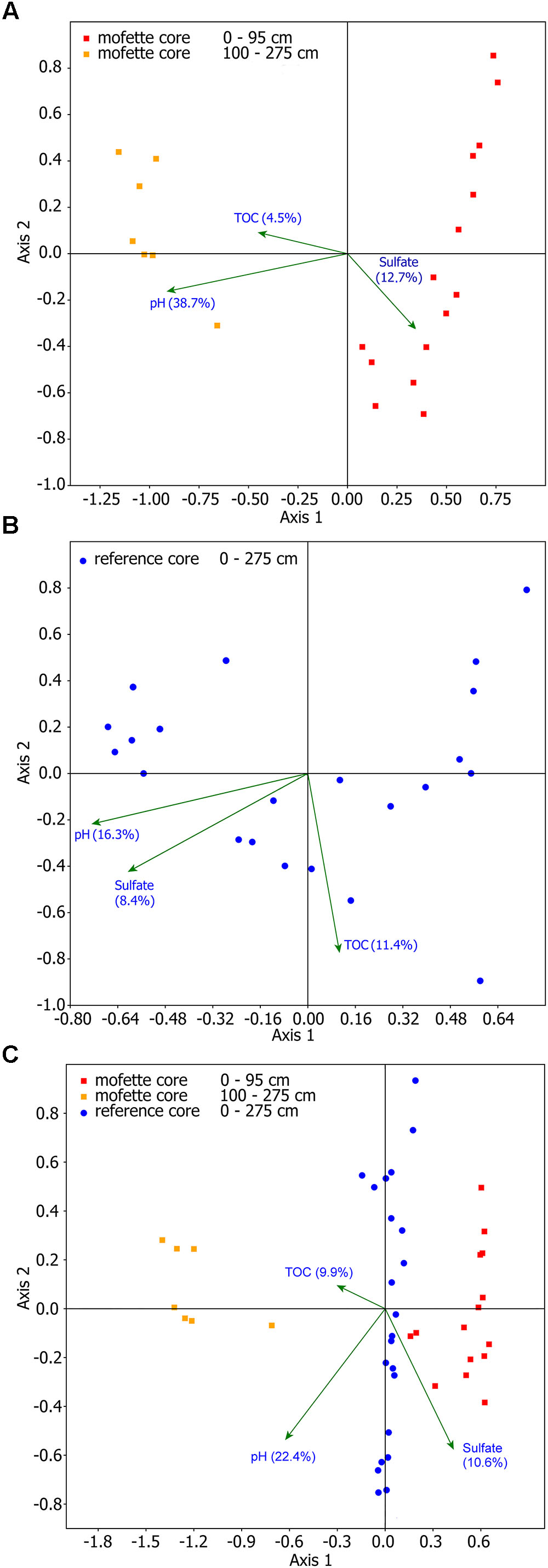
FIGURE 7. CCA analyses of the microbial communities of the mofette (A), reference site (B), and for both (C). The environmental parameters were selected based on the forward-selection method with significant padj-values (Bonferroni corrected <0.05).
The 16S rRNA gene copy numbers at the reference site are positively correlated with pH (R = 0.709, p = 0.0002), whereas at the mofette site, a significant negative correlation with pH (R = -0.655, p = 0.0009) was observed (Figure 2G) (Supplementary Tables S5, S6). No significant correlation with any measured environmental parameter was found for the abundance of the dsrB or mcrA genes.
Discussion
Dry mofettes – such as the HMF – allow for an investigation of geo–bio interactions that result from the permanent degassing of mantle-derived CO2. In the uppermost soil layers – which have been the focus of other studies (Krüger et al., 2009; Blagodatskaya et al., 2010; Sáenz de Miera et al., 2014; Beulig et al., 2015) – the conditions are not necessarily permanent strictly anoxic, which also allows aerobic or microaerophilic heterotrophic microorganisms to grow. The input of oxygen can derive from meteoric water as well as horizontally via groundwater flow. The sampling depth and lithological profile are therefore of major importance in unraveling the influence of the CO2 on the microbial communities. For the first time, the present study provides insights into the community structure in 3-m-deep sediments of a mofette and a nearby reference site. The combination of geochemical analyses and Illumina MiSeq high-throughput sequencing of 16S rRNA genes reveals the complexity of geo–bio interactions in the CO2-influenced habitat.
We observed a strong influence of the emanating CO2-rich fluid on crucial soil parameters, such as pH, water content, and ion composition (Figure 2). The relatively low pH may be an indication for the influence of CO2 also at the reference site, and reflects the characteristics of the lithological profile. However, the CO2 flux measurements at the surface of the reference site did not reveal any mofette activity; this is supported by the typical TOC profile and low sulfate concentration and conductivity. Both sampling sites were located in a wet land area (floodplain) with a high groundwater level. We assume that depending on the season (and flooding) an influence by CO2-rich groundwater at both sites is possible. It is known that the groundwater level at the HMF may shift around 20 cm per day (Nickschick et al., 2017). Within the first 80 cm of the depth profile the pH of the reference site (pH > 4) was higher than at the mofette site (pH < 4). At the reference site the groundwater level is situated within a gravel-rich sand layer at a depth of 80 to 100 cm. The groundwater, which may migrate horizontally, presumably causes a decrease of the pH due to dissolved CO2. Thereby, the fine- to medium-grained clayey sand in the reference site might function as a natural vertical barrier for the groundwater and CO2 flow, especially in depths deeper than the core sequence. In the mofette, the groundwater level was shallower (25–70 cm) than at the reference site. On the other hand the pH in the mofette was highest in the gravel-rich layer between depths of 130–140 cm. The high conductivity and sulfate concentration (Figure 2) may indicate that mineral water from greater depth is admixed with groundwater in the mofette. Mineral water found in the same mofette in a depth of ca. 82 m had a pH of 6.4 and a sulfate concentration 1470 mg L-1 (Bussert et al., 2017).
The different microbial community structures found in each depth and each core at the reference site and in the mofette cannot be explained by the variances in the lithology of the core material, however, the lithological setting is from a major importance for both habitats in greater depth (78 m) where a cap-rock like carbonate-rich layer largely seals the CO2-rich aquifer and allows for a channelized CO2 degassing (Bussert et al., 2017). Instead, the pH, TOC, and sulfate concentration formed the optimal subset of parameters to explain the abundance and distribution of the taxa at both sites. Multivariate statistical analyses and qPCR results revealed that at the reference site, the microbial abundance was positively correlated with pH (R = 0.709, p = 0.0002), while a significant negative correlation was observed at the mofette site (R = -0.655, p = 0.0009; Figures 2C,G and Supplementary Tables S5, S6). Therefore, several taxa of the microbial community at the mofette site seem to be adapted to the acidic conditions. However, the diversity and the evenness at the mofette site are positively correlated with the pH (R = 0.582, p = 0.0044, and R = 0.550, p = 0.008; Supplementary Tables S5, S6), indicating that it is mainly specialists that can withstand the extreme environmental conditions. It is remarkable that solely the pH value explains 38.7% of the distribution pattern of taxa at the mofette site (Figure 7A). Since the pH value at the reference site is hardly affected by the emanating CO2, it only explains 16.3% of the OTU distribution (Figure 7B). However, since the pH is generally co-correlated with CO2 concentration, it is necessary to consider the fact that the abundance of autotrophic microorganisms in the mofette site is also positively correlated with the amount of CO2-rich emanating fluids. The results of the geochemical analyses and the 16S sequencing lead to the assumption that in addition to pH, sulfate also plays an important role in microbial matter cycling under anaerobic conditions in the mofette site. The sulfate concentration – which is five to fifteen times higher in the mofette site – explains 12.7% of the distribution pattern of microorganisms at the mofette (Figure 7A). In comparison with the mofette, the sulfate concentration at the reference site explains only 8.4% of the community structure (Figure 7B).
In comparison with the reference site, the TOC values were up to 100 times higher in the peat layers of the mofette (Figures 2A,D). While pH and ion concentrations are directly influenced by the emanating CO2, the high TOC content at the mofette site can be explained by the consequences of acidification and anoxia on mesoscopic or macroscopic eukaryotes involved in the degradation of complex organic matter; additionally it was shown that acetogenesis is a prominent process in mofettes (Beaubien et al., 2008; Beulig et al., 2015, 2016; Fernández-Montiel et al., 2016). The TOC values explain 11.4% of the distribution pattern at the reference site (Figure 7B) and only 4.5% at the mofette (Figure 7A). Moreover, the top 10 abundant taxa at the reference site were heterotrophic microorganisms (Supplementary Tables S8, S9). In contrast, the high abundance of chemolithoautotrophic taxa in the mofette highlights the importance of an autotrophic- rather than a heterotrophic lifestyle in habitats with strongly elevated CO2 concentrations (Oppermann et al., 2010). Autotrophic microorganisms can fix significant amounts of carbon from geogenic CO2 (Nowak et al., 2015), whereas organic substrates remain undegraded and accumulate in the sediment. An interesting side effect can be seen in a long-term perspective because such an enormous accumulation of organic substances in sediments may be the precondition for the development of a paleo organic layer at a late stage.
The bacterial 16S rRNA gene copy numbers in the mofette varied from 105 to 109 copies g-1 of sediment. The highest gene copy numbers were found in the topsoil of the mofette (1.2 × 109 gene copies g-1 at a depth of 12 cm; Figure 2G). While the abundance of microorganisms at the reference site followed a classical trend and decreased with depth, the abundance of microorganisms in the mofette did not decrease with depth beyond 50 cm. The present study suggests for the first time that at a depth greater than 100 cm, the microbial abundance in the mofette even partially exceeded that of the reference site. This trend was also observed for SRB and methanogenic archaea (Figures 2H,I). As discussed later, presumably the uprising CO2-rich fluid feeds the ecosystem from underneath with substrates such as CO2 and sulfate. However, compared with the reference site, the gene copy numbers at the mofette were about one order of magnitude lower for the uppermost 100 cm. Higher cell numbers in the surface soil of the reference site can be explained by the predominance of aerobic processes and a rather moderate pH. In general, the gene copy numbers per gram of subsurface sediment at the HMF were similar to soils with highly elevated CO2 concentrations (109 to 1010 copies g-1 sediment) (Beaubien et al., 2008; Krüger et al., 2009; Oppermann et al., 2010; Frerichs et al., 2013; Beulig et al., 2015; Fernández-Montiel et al., 2016). The top 40 cm of the mofette site at the HMF was an exception, for here, the gene copies were one order of magnitude lower (108 to 109 copies g-1 sediment) compared with the Plesná floodplain site, which is located 1.8 km to the NW (Beulig et al., 2015). Reasons for the discrepancy could be the higher CO2 flux at the HMF or different soil characteristics (Kämpf et al., 2013). Additionally, seasonal effects have to be considered. In April (Beulig et al., 2015), the surface water level is often higher, and substrates might be more easily accessible in comparison with September, when the HMF drilling took place.
Both diversity and evenness were lower at the mofette than at the reference site (Figures 2J,K). A lowered diversity was also observed at the La Sima mofette in Spain (Sáenz de Miera et al., 2014). The low evenness indicates a dominance of specialists, such as anaerobic acidophilic and acido-tolerant taxa in the mofette community. In the mofette, the alpha diversity and the evenness were higher at depths between 85 and 125 cm. This shift can be explained by the surface water table and the admixture of surrounding aerobic communities.
The results of the beta diversity of the community highlight the assumption that only the deeper sediments are almost unaffected by oxygen. The PCoA plot of the weighted UniFrac distance metric revealed that the mofette harbors two distinct communities (Figure 4). One cluster includes communities from shallow depths between 0 and 95 cm (Cluster A), and the other cluster includes communities in the deep anoxic sediments from 100 to 275 cm (Cluster B). The microbial communities from the reference site cluster apart from all mofette samples, which highlights the fact that the mofette community is strongly influenced by the degassing phenomenon. Site-specific effects – such as a low pH and changes in ion composition – do not sufficiently explain the differences between Cluster A and Cluster B, but the oxygen availability could be identified as the major stress factor. In the mofette, the oxygen-dependent depth gradient was clearly reflected in the antagonistic shift of the relative abundance of aerobic and anaerobic taxa in Cluster A and Cluster B. The relative abundance of obligate and facultative anaerobic microorganisms affiliated with Bacteroidetes, Bathyarchaeota, Caldiserica, and Parcubacteria gradually increased with depth. At the same time, the relative abundance of versatile Proteobacteria strongly decreased with increasing depth (Figure 3). Strictly anaerobic and facultative aerobic microorganisms affiliated with Acidithiobacillus, Clostridiaceae 1, Bacillus, and Ignavibacteriales that were observed in Cluster A were much more abundant at the mofette than at the reference site. In Cluster B, strictly anaerobic microorganisms within the taxa Thermoanaerobaculum, Bacteroidetes vadin HA17, Desulfobacca, Caldisericum, Anaerolineaceae, and Sulfurovum shaped the community and explained most of the differences (14.5% dissimilarity) between the communities of the deep sediments of the mofette and reference site (Figures 5A,B). Neither in Cluster A nor Cluster B were any of the strictly aerobic taxa more abundant in the mofette than at the reference site, except for Acidobacteriaceae (Subg. 1). Additionally, the family Acidobacteriaceae (Subg. 1) was the most abundant taxa in both study sites (mofette: 12.4%; reference site: 10.5%). Members of this group are heterotrophic, aerobic, or microaerophilic, and some species are facultative anaerobes (Pankratov et al., 2012). Therefore, the occurrence at both sites under both aerobic and anaerobic conditions is plausible. The HMF multivariate statistics indicate a strong negative correlation of the taxon with pH, and the same correlation has been observed by Männistöet al. (2007) in tundra soils. Moreover, the proportion of unassigned taxa (4.0%) was much higher in the mofette compared with the reference site, indicating that mofettes are unique environments that harbor a large fraction of novel, as-of-yet undescribed organisms.
The sulfate-rich mofette with a pH < 4.0 offers ideal growth conditions for obligate acidophilic bacteria such as Acidithiobacillus (Kelly and Wood, 2000). Accordingly, these bacteria’s abundance is positively correlated with the sulfate concentration (R = 0.699, p = 0.0003; Supplementary Tables S5,S6). In both clusters, taxa potentially involved in sulfur cycling had a more substantial proportion (14.4%) and a higher diversity (e.g., of Desulfobacca, Desulfosporosinus, Sulfurovum, and Sulfuriferula) in the mofette in comparison with the reference site (3.0%; Figure 6A). Desulfobacca was also recently found in a Plesná floodplain site (Beulig et al., 2016). A higher abundance of SRB was also observed in CO2 vents (Beaubien et al., 2008) and CO2-affected soils (Frerichs et al., 2013). Striking differences between the mofette and reference site were also observed for taxa potentially involved in iron-cycling. The results lead to the assumption that in addition to sulfate reduction, iron-cycling is also an important feature under aerobic conditions at the reference site and under anaerobic conditions in the mofette. The aerobic or microaerophilic taxa – such as Gallionella and Sideroxydans (Hallbeck and Pedersen, 1990; Emerson and Moyer, 1997) – were more abundant at the reference site, whereby acidophilic obligate and facultative anaerobic genera – such as Ferrithrix and Ferrovum (Johnson et al., 2009; Johnson et al., 2014) – dominated in the mofette (Figure 7B).
The extreme environmental conditions in the mofette also favor the growth of methanogenic archaea. Methanosphaerula (Cadillo-Quiroz et al., 2009) occurred solely in the mofette, whereby other genera – such as Methanoregula (Bräuer et al., 2011), Methanosaeta (Patel and Sprott, 1990), and Methanosarcina (Zinder et al., 1985) – were abundant at all depths in the mofette core but occurred only in low abundances close to the surface in the reference site, presumably because oxygen is spatially depleted by aerobic processes. Other archaea potentially involved in acetogenesis and methane cycling – such as Bathyarchaeota and ammonia-oxidizing Thaumarchaeota affiliated with South African Gold Mine Gp 1 (SAGMCG-1) (Takai et al., 2001) – were found at the mofette and the reference site in almost the same relative abundances (1.7 and 0.2%). These organisms have been found in marine sediments, deep aquifer waters, a CO2 vent, as well as in water from wet mofettes and in thermal water in the Eger region (Kubo et al., 2012; Frerichs et al., 2013; Evans et al., 2015; Krauze et al., 2017).
Additionally, several other microorganisms found in the deep mofette sediments (e.g., Thermoanaerobaculum, Caldisericum, Sulfurovum, and Mobilitalea) have been isolated from hot springs, hydrothermal sediments, or thermal water from a 2.8-km-deep well (Oude Elferink et al., 1999; Inagaki et al., 2004; Losey et al., 2013; Podosokorskaya et al., 2014). These microorganisms most likely derive from the deep biosphere, indicating that the mofette is connected to the deep subsurface via ascending fluids. The environmental conditions in the mofette at 1–3 m in depth are similar to deep sediments or deeply originating thermal waters with respect to the availability of oxygen and ion composition and may therefore also provide adequate growth conditions for such taxa.
The microbial communities from both Cluster A and Cluster B at the HMF share many abundant taxa with the communities described by Sáenz de Miera et al. (2014) in the shallow sediments at the La Sima CO2 gas vent (top 10–20 cm; Supplementary Table S12) and a CO2-influenced floodplain site at Plesná (Beulig et al., 2015). Although the relative abundances of these common taxa display clear differences, they represent an outline of the characteristic core community of CO2-influenced surface habitats. The occurrence of unshared site-specific taxa can be explained by the differing environmental conditions. For example, higher oxygen concentrations (<7%) (Peinado et al., 2009) and historical thermal anomalies might specifically trigger the growth of aerobic or facultative anaerobic thermophiles – such as members of Ktedonobacteria (e.g., Thermogemmatispora) – at the La Sima mofette. The high resemblance of the Cluster A communities to the microbial communities at the CO2-influenced Plesná floodplain site (Beulig et al., 2015) lead to the assumption that the environmental conditions at both mofettes located in the Cheb Basin are rather similar compared with those the La Sima site. The low pH at the HMF and the floodplain particularly favors the growth of acidophilic taxa (e.g., Acidobacteria, Subg. 1), which have a rather low abundance at the La Sima site.
The high relative abundance of sulfate reducers, methanogens as well as further autotrophs indicate that the community is supported by hydrothermal originating substrates, delivered by the mantle-derived CO2. The geochemical data demonstrate that electron acceptors such as sulfate and CO2 are sufficient available. This is in good accordance with the findings from Beulig et al. (2016) who showed that in the mofette transcripts related to methanogenesis (mcr) and sulfate reduction (dsr, cys, apr) were remarkably increased in the frequency. In the Eger Rift, hydrogen, which is a key electron donor in the deep biosphere (Stevens and McKinley, 1995; Anderson et al., 2001; Chapelle et al., 2002; Nealson, 2005; Spear et al., 2005; Hinrichs et al., 2006), becomes available during radiolytic decay in the underlying fissured granite or stress-released during earthquake swarms (Bräuer et al., 2005, 2007).
Conclusion
Our study of a dry CO2 degassing mofette in Hartoušov, NW Bohemia, as central part of a CO2 conduit deepens the knowledge of geo–bio interactions in extreme environments with elevated CO2 concentrations. The mofette ecosystem is characterized by anoxic conditions, a low pH, high TOC content and due to the admixing of mineral waters a relatively high sulfate concentration and conductivity. Our study shows that the exceptional environmental conditions provoke a decrease in diversity and favor the occurrence of anaerobic, acidophilic taxa, whereby sulfate reduction and methanogenesis become distinct processes. However, the deeper mofette sediments alone provide strictly anaerobic conditions, and accordingly, two distinct community clusters were found at different depth intervals. Electron acceptors such as CO2 and sulfate are provided by the permanently ascending fluid which is admixed with deep thermal waters, thereby forming a kind of anoxic deep biosphere habitat close to the surface level. Hydrogen, originating from deep fissured granites may thereby function as an electron donor. This study is limited to taxonomical assignments, further studies focusing on deep mofette sediments should implement metagenomic or transcriptomic approaches to unravel the functional repertoire of the communities.
Author Contributions
All authors have taken part in the interpretation of the results and writing the manuscript. QL wrote the manuscript, performed sampling, pore water analyses, DNA extractions and purification, gene quantification, and bioinformatical based statistical analyses. HK and TN performed CO2 soil gas flux measurements. RB led the drilling campaign and performed sedimentological analyses. FH and PK were involved in statistical analyses. BP performed TOC and isotopic analyses. DW contributed to the interpretation of the results and valuable discussion. MA designed and supervised the study and led the writing of the present manuscript. All authors have taken part in the manuscript revisions and agreed with its scientific content.
Funding
This study was supported by the Deutsche Forschungsgemeins-chaft (DFG) within the framework of the priority program 1006 “International Continental Drilling Program” (ICDP) by a grant to MA (AL 1898/1) and HK (KA 902/9, 16). The 16S rRNA gene amplicon (MiSeq) sequencing was financed through the Helmholtz Research Programmes “Geosystem–The Changing Earth” of the GFZ German Research Centre for Geosciences–Helmholtz Centre Potsdam.
Conflict of Interest Statement
The authors declare that the research was conducted in the absence of any commercial or financial relationships that could be construed as a potential conflict of interest.
Acknowledgments
QL gratefully acknowledges financial support from the China Scholarship Council. The authors would like to thank André Friese and Axel Kitte (GFZ German Research Centre for Geosciences) for their guidance and assistance with anion/cation measurements. Special thanks to Oliver Burckhardt and Axel Kitte (GFZ German Research Centre for Geosciences) for their valuable help during the sampling campaigns and support in the lab.
Supplementary Material
The Supplementary Material for this article can be found online at: https://www.frontiersin.org/articles/10.3389/fmicb.2018.02787/full#supplementary-material
Footnote
References
Anderson, R. T., Chapelle, F. H., and Lovley, D. R. (2001). Comment on “abiotic controls on H2 production from basalt-water reactions and implications for aquifer biogeochemistry”. Environ. Sci. Technol. 35, 1553–1557. doi: 10.1021/es0015996
Bankwitz, P., Bankwitz, E., Brauer, K., Kampf, H., and Storr, M. (2003a). Deformation structures in Plio-and Pleistocene sediments (NW Bohemia, central Europe). Spec. Publ. Geol. Soc. Lon. 216, 73–94. doi: 10.1144/GSL.SP.2003.216.01.06
Bankwitz, P., Schneider, G., Kämpf, H., and Bankwitz, E. (2003b). Structural characteristics of epicentral areas in Central Europe: study case Cheb Basin (Czech Republic). J. Geodyn. 35, 5–32. doi: 10.1016/S0264-3707(02)00051-0
Beaubien, S. E., Ciotoli, G., Coombs, P., Dictor, M., Krüger, M., Lombardi, S., et al. (2008). The impact of a naturally occurring CO2 gas vent on the shallow ecosystem and soil chemistry of a Mediterranean pasture (Latera, Italy). Int. J. Greenhouse Gas Control 2, 373–387. doi: 10.1016/j.ijggc.2008.03.005
Ben-Dov, E., Brenner, A., and Kushmaro, A. (2007). Quantification of sulfate-reducing bacteria in industrial wastewater, by real-time polymerase chain reaction (PCR) using dsrA and apsA genes. Microb. Ecol. 54, 439–451. doi: 10.1007/s00248-007-9233-2
Beulig, F., Heuer, V. B., Akob, D. M., Viehweger, B., Elvert, M., Herrmann, M., et al. (2015). Carbon flow from volcanic CO2 into soil microbial communities of a wetland mofette. ISME J. 9, 746–759. doi: 10.1038/ismej.2014.148
Beulig, F., Urich, T., Nowak, M., Trumbore, S. E., Gleixner, G., Gilfillan, G. D., et al. (2016). Altered carbon turnover processes and microbiomes in soils under long-term extremely high CO2 exposure. Nat. Microbiol. 1:15025. doi: 10.1038/nmicrobiol.2015.25
Blagodatskaya, E., Blagodatsky, S., Dorodnikov, M., and Kuzyakov, Y. (2010). Elevated atmospheric CO2 increases microbial growth rates in soil: results of three CO2 enrichment experiments. Global Change Biol. 16, 836–848. doi: 10.1111/j.1365-2486.2009.02006.x
Blume, H.-P., and Felix-Henningsen, P. (2009). Reductosols: natural soils and technosols under reducing conditions without an aquic moisture regime. J. Plant Nutr. Soil Sci. 172, 808–820. doi: 10.1002/jpln.200800125
Blume, H.-P., Stahr, K., and Leinweber, P. (2011). Bodenkundliches Praktikum: Eine Einführung in pedologisches Arbeiten für Ökologen, Land-und Forstwirte, Geo-und Umweltwissenschaftler. Berlin: Springer-Verlag.
Brandariz-Fontes, C., Camacho-Sanchez, M., Vilà, C., Vega-Pla, J. L., Rico, C., and Leonard, J. A. (2015). Effect of the enzyme and PCR conditions on the quality of high-throughput DNA sequencing results. Sci. Rep. 5:8056. doi: 10.1038/srep08056
Bräuer, K., Kämpf, H., Faber, E., Koch, U., Nitzsche, H.-M., and Strauch, G. (2005). Seismically triggered microbial methane production relating to the Vogtland NW Bohemia earthquake swarm period 2000, Central Europe. Geochem. J. 39, 441–450. doi: 10.2343/geochemj.39.441
Bräuer, K., Kämpf, H., Koch, U., Niedermann, S., and Strauch, G. (2007). Seismically induced changes of the fluid signature detected by a multi-isotope approach (He, CO2, CH4, N2) at the Wettinquelle, Bad Brambach (central Europe). J. Geophys. Res. 112:301. doi: 10.1029/2006JB004404
Bräuer, K., Kämpf, H., Niedermann, S., and Strauch, G. (2018). Monitoring of helium and carbon isotopes in the western Eger Rift area (Czech Republic): relationships with the 2014 seismic activity and indications for recent (2000–2016) magmatic unrest. Chem. Geol. 482, 131–145. doi: 10.1016/j.chemgeo.2018.02.017
Bräuer, S. L., Cadillo-Quiroz, H., Ward, R. J., Yavitt, J. B., and Zinder, S. H. (2011). Methanoregula boonei gen. nov., sp. nov., an acidiphilic methanogen isolated from an acidic peat bog. Int. J. System. Evol. Microbiol. 61, 45–52. doi: 10.1099/ijs.0.021782-0
Bucha, V., Horáček, J., and Malkovský, M. (1990). Palaeomagnetic stratigraphy of the tertiary of the Cheb Basin (W Bohemia). Vìstník Ústředního Ústavu Geologického 65, 267–278.
Bussert, R., Kämpf, H., Flechsig, C., Hesse, K., Nickschick, T., Liu, Q., et al. (2017). Drilling into an active mofette: pilot-hole study of the impact of CO2-rich mantle-derived fluids on the geo–bio interaction in the western Eger Rift (Czech Republic). Sci. Drill. 23, 13–27. doi: 10.5194/sd-23-13-2017
Cadillo-Quiroz, H., Yavitt, J. B., and Zinder, S. H. (2009). Methanosphaerula palustris gen. nov., sp. nov., a hydrogenotrophic methanogen isolated from a minerotrophic fen peatland. Int. J. Syst. Evol. Microbiol. 59, 928–935. doi: 10.1099/ijs.0.006890-0
Caporaso, J. G., Kuczynski, J., Stombaugh, J., Bittinger, K., Bushman, F. D., Costello, E. K., et al. (2010). QIIME allows analysis of high-throughput community sequencing data. Nat. Methods 7, 335–336. doi: 10.1038/nmeth.f.303
Caporaso, J. G., Lauber, C. L., Walters, W. A., Berg-Lyons, D., Lozupone, C. A., Turnbaugh, P. J., et al. (2011). Global patterns of 16S rRNA diversity at a depth of millions of sequences per sample. Proc. Natl. Acad. Sci. U.S.A. 108(Suppl. 1), 4516–4522. doi: 10.1073/pnas.1000080107
Chapelle, F. H., O’Neill, K., Bradley, P. M., Methé, B. A., Ciufo, S. A., Knobel, L. L., et al. (2002). A hydrogen-based subsurface microbial community dominated by methanogens. Nature 415:312. doi: 10.1038/415312a
Chiodini, G., Caliro, S., Cardellini, C., Avino, R., Granieri, D., and Schmidt, A. (2008). Carbon isotopic composition of soil CO2 efflux, a powerful method to discriminate different sources feeding soil CO2 degassing in volcanic-hydrothermal areas. Earth Planet. Sci. Lett. 274, 372–379. doi: 10.1016/j.epsl.2008.07.051
DeSantis, T. Z., Hugenholtz, P., Larsen, N., Rojas, M., Brodie, E. L., Keller, K., et al. (2006). Greengenes, a chimera-checked 16S rRNA gene database and workbench compatible with ARB. Appl. Environ. Microbiol. 72, 5069–5072. doi: 10.1128/AEM.03006-05
Dobeš, M., Hercog, F., and Mazáč, F. (1986). Die geophysikalische untersuchung der hydrogeologischen strukturen im cheb-becken, Sbor geol vìd, Užitá geofyz 21, 117–158.
Edgar, R. C. (2010). Search and clustering orders of magnitude faster than BLAST. Bioinformatics (Oxford, England) 26, 2460–2461. doi: 10.1093/bioinformatics/btq461
Emerson, D., and Moyer, C. (1997). Isolation and characterization of novel iron-oxidizing bacteria that grow at circumneutral pH. Appl. Environ. Microbiol. 63, 4784–4792.
Emiliani, C. (1992). Planet Earth: Cosmology, Geology, and the Evolution of Life and Environment/Cesare Emiliani. Cambridge: Cambridge university press.
Evans, P. N., Parks, D. H., Chadwick, G. L., Robbins, S. J., Orphan, V. J., Golding, S. D., et al. (2015). Methane metabolism in the archaeal phylum Bathyarchaeota revealed by genome-centric metagenomics. Science (New York, N.Y.) 350, 434–438. doi: 10.1126/science.aac7745
Fernández-Montiel, I., Pedescoll, A., and Bécares, E. (2016). Microbial communities in a range of carbon dioxide fluxes from a natural volcanic vent in Campo de Calatrava, Spain. Int. J. Greenhouse Gas Control 50, 70–79. doi: 10.1016/j.ijggc.2016.04.017
Fischer, T., Horálek, J., Hrubcová, P., Vavryčuk, V., Bräuer, K., and Kämpf, H. (2014). Intra-continental earthquake swarms in West-Bohemia and Vogtland: a review. Tectonophysics 611, 1–27. doi: 10.1016/j.tecto.2013.11.001
Flechsig, C., Bussert, R., Rechner, J., Schütze, C., and Kämpf, H. (2008). The hartoušov mofette field in the cheb basin, western eger rift (Czech Republic): a comperative geoelectric, sedimentologic and soil gas study of a magmatic diffuse CO2-degassing structure. Z. Geol. Wiss. 36, 177–193.
Frerichs, J., Oppermann, B. I., Gwosdz, S., Möller, I., Herrmann, M., and Krüger, M. (2013). Microbial community changes at a terrestrial volcanic CO2 vent induced by soil acidification and anaerobic microhabitats within the soil column. FEMS Microbiol. Ecol. 84, 60–74. doi: 10.1111/1574-6941.12040
Geissler, W. H., Kämpf, H., Bankwitz, P., and Bankwitz, E. (2004). The quaternary tephra-tuff deposit of Mýtina (southern rim of the western Eger Graben/Czech Republic): indications for eruption and deformation processes. Z. Geol. Wissenschaften 32, 31–54.
Girault, F., and Perrier, F. (2014). The syabru-bensi hydrothermal system in central Nepal: 2. Modeling and significance of the radon signature. J. Geophys. Res. Solid Earth 119, 4056–4089. doi: 10.1002/2013JB010302
Girault, F., Perrier, F., Crockett, R., Bhattarai, M., Koirala, B. P., France-Lanord, C., et al. (2014). The syabru-bensi hydrothermal system in central Nepal: 1. Characterization of carbon dioxide and radon fluxes. J. Geophys. Res. Solid Earth 119, 4017–4055. doi: 10.1002/2013JB010301
Großkopf, R., Stubner, S., and Liesack, W. (1998). Novel euryarchaeotal lineages detected on rice roots and in the anoxic bulk soil of flooded rice microcosms. Appl. Environ. Microbiol. 64, 4983–4989.
Hallbeck, L., and Pedersen, K. (1990). Culture parameters regulating stalk formation and growth rate of Gallionella ferruginea. J. Gen. Microbiol. 136, 1675–1680. doi: 10.1099/00221287-136-9-1675
Hammer,Ø., Harper, D. A. T., and Ryan, P. D. (2001). PAST-palaeontological statistics, ver. 1.89. Palaeontol. Electr. 4, 1–9.
Hinrichs, K. U., Hayes, J. M., Bach, W., Spivack, A. J., Hmelo, L. R., Holm, N. G., et al. (2006). Biological formation of ethane and propane in the deep marine subsurface. Proc. Natl. Acad. Sci. U.S.A. 103, 14684–14689. doi: 10.1073/pnas.0606535103
Hrubcová, P., Geissler, W. H., Bräuer, K., Vavryčuk, V., Tomek, č., and Kämpf, H. (2017). Active magmatic underplating in western eger rift, central Europe. Tectonics 36, 2846–2862. doi: 10.1002/2017TC004710
Ibs-von Seht, M., Plenefisch, T., and Klinge, K. (2008). Earthquake swarms in continental rifts — A comparison of selected cases in America, Africa and Europe. Tectonophysics 452, 66–77. doi: 10.1016/j.tecto.2008.02.008
Inagaki, F., Takai, K., Nealson, K. H., and Horikoshi, K. (2004). Sulfurovum lithotrophicum gen. nov., sp. nov., a novel sulfur-oxidizing chemolithoautotroph within the epsilon-proteobacteria isolated from Okinawa Trough hydrothermal sediments. Int. J. Syst. Evol. Microbiol. 54, 1477–1482. doi: 10.1099/ijs.0.03042-0
Inguaggiato, S., Cardellini, C., Taran, Y., and Kalacheva, E. (2017). The CO2 flux from hydrothermal systems of the Karymsky volcanic Centre, Kamchatka. J. Volcanol. Geotherm. Res. 346, 1–9. doi: 10.1016/j.jvolgeores.2017.07.012
Johnson, D. B., Bacelar-Nicolau, P., Okibe, N., Thomas, A., and Hallberg, K. B. (2009). Ferrimicrobium acidiphilum gen. nov., sp. nov. and Ferrithrix thermotolerans gen. nov., sp. nov. heterotrophic, iron-oxidizing, extremely acidophilic actinobacteria. Int. J. System. Evol. Microbiol. 59, 1082–1089. doi: 10.1099/ijs.0.65409-0
Johnson, D. B., Hallberg, K. B., and Hedrich, S. (2014). Uncovering a microbial enigma: isolation and characterization of the streamer-generating, iron-oxidizing, acidophilic bacterium “Ferrovum myxofaciens”. Appl. Environ. Microbiol. 80, 672–680. doi: 10.1128/AEM.03230-13
Kämpf, H., Bräuer, K., Schumann, J., Hahne, K., and Strauch, G. (2013). CO2 discharge in an active, non-volcanic continental rift area (Czech Republic): characterisation (δ13C, 3He/4He) and quantification of diffuse and vent CO2 emissions. Chem. Geol. 339, 71–83. doi: 10.1016/j.chemgeo.2012.08.005
Kämpf, H., Geissler, W. H., and Bräuer, K. (2007). “Combined gas-geochemical and receiver function studies of the vogtland/nw bohemia intraplate mantle degassing field, central Europe,” in Mantle Plumes: A Multidisciplinary Approach, eds J. R. R. Ritter and U. R. Christensen (Berlin: Springer), 127–158. doi: 10.1007/978-3-540-68046-8_4
Kämpf, H., Peterek, A., Rohrmüller, J., Kümpel, H. J., and Geissler, W. (2005). “The KTB deep crustal laboratory and the western Eger Graben,” in GeoErlangen 2005: System Earth-Biosphere Coupling/Regional Geology of Central Europe (Erlangen 2005), 2005, eds R. Koch and H.-G. Röhling (Erlangen: Schriftenreihe der Deutschen Gesellschaft für Geowissenschaften), 37–107.
Kelly, D. P., and Wood, A. P. (2000). Reclassification of some species of Thiobacillus to the newly designated genera Acidithiobacillus gen. nov., Halothiobacillus gen. nov. and Thermithiobacillus gen. nov. Int. J. Syst. Evol. Microbiol. 50(Pt 2), 511–516. doi: 10.1099/00207713-50-2-511
Kopecký, L. (1979). Magmatism of the Ohre rift in the Bohemian Massif, its Relationship to the Deep Fault Tectonics and to the Geologic Evolution, and its Ore Mineralisation. Bratislava: Czechoslovak geology and global tectonics, 167–181.
Krauze, P., Kämpf, H., Horn, F., Liu, Q., Voropaev, A., Wagner, D., et al. (2017). Microbiological and geochemical survey of CO2-dominated mofette and mineral waters of the cheb basin, Czech Republic. Front. Microbiol. 8:2446. doi: 10.3389/fmicb.2017.02446
Krüger, M., Jones, D., Frerichs, J., Oppermann, B. I., West, J., Coombs, P., et al. (2011). Effects of elevated CO2 concentrations on the vegetation and microbial populations at a terrestrial CO2 vent at Laacher See, Germany. Int. J. Greenhouse Gas Control 5, 1093–1098. doi: 10.1016/j.ijggc.2011.05.002
Krüger, M., West, J., Frerichs, J., Oppermann, B., Dictor, M.-C., Jouliand, C., et al. (2009). Ecosystem effects of elevated CO2 concentrations on microbial populations at a terrestrial CO2 vent at Laacher See, Germany. Enery Proc. 1, 1933–1939. doi: 10.1016/j.egypro.2009.01.252
Kubo, K., Lloyd, K. G., F Biddle, J., Amann, R., Teske, A., and Knittel, K. (2012). Archaea of the miscellaneous crenarchaeotal group are abundant, diverse and widespread in marine sediments. ISME J. 6, 1949–1965. doi: 10.1038/ismej.2012.37
Lane, D. J. (1991). “16S/23S rRNA sequencing,” in Nucleic Acid Techniques in Bacterial Systematics, eds E. Stackebrandt and M. Goodfellow (Chichester: John Wiley and Sons), 115–175.
Losey, N. A., Stevenson, B. S., Busse, H.-J., Sinninghe Damsté, J. S., Rijpstra, W. I. C., Rudd, S., et al. (2013). Thermoanaerobaculum aquaticum gen. nov., sp. nov., the first cultivated member of acidobacteria subdivision 23, isolated from a hot spring. Int. J. Syst. Evol. Microbiol. 63, 4149–4157. doi: 10.1099/ijs.0.051425-0
Männistö, M. K., Tiirola, M., and Häggblom, M. M. (2007). Bacterial communities in Arctic fjelds of Finnish Lapland are stable but highly pH-dependent. FEMS Microbiol. Ecol. 59, 452–465. doi: 10.1111/j.1574-6941.2006.00232.x
Mehlhorn, J., Beulig, F., Küsel, K., and Planer-Friedrich, B. (2014). Carbon dioxide triggered metal(loid) mobilisation in a mofette. Chem. Geol. 382, 54–66. doi: 10.1016/j.chemgeo.2014.05.027
Mehlhorn, J., Byrne, J. M., Kappler, A., and Planer-Friedrich, B. (2016). Time and temperature dependency of carbon dioxide triggered metal(loid) mobilization in soil. Appl. Geochem. 74, 122–137. doi: 10.1016/j.apgeochem.2016.09.007
Morales, S. E., and Holben, W. E. (2013). Functional response of a near-surface soil microbial community to a simulated underground CO2 storage leak. PLoS One 8:e81742. doi: 10.1371/journal.pone.0081742
Mrlina, J., Kämpf, H., Kroner, C., Mingram, J., Stebich, M., Brauer, A., et al. (2009). Discovery of the first quaternary maar in the bohemian massif, Central Europe, based on combined geophysical and geological surveys. J. Volcanol. Geotherm. Res. 182, 97–112. doi: 10.1016/j.jvolgeores.2009.01.027
Nealson, K. H. (2005). Hydrogen and energy flow as “sensed” by molecular genetics. Proc. Natl. Acad. Sci. U.S.A. 102, 3889–3890. doi: 10.1073/pnas.0500211102
Nickschick, T., Flechsig, C., Meinel, C., Mrlina, J., and Kämpf, H. (2017). Architecture and temporal variations of a terrestrial CO2 degassing site using electric resistivity tomography and self-potential. Int. J. Earth Sci. 106, 2915–2926. doi: 10.1007/s00531-017-1470-0
Nickschick, T., Kämpf, H., Flechsig, C., Mrlina, J., and Heinicke, J. (2015). CO2 degassing in the hartoušov mofette area, Western Eger Rift, imaged by CO2 mapping and geoelectrical and gravity surveys. Int. J. Earth Sci. 104, 2107–2129. doi: 10.1007/s00531-014-1140-4
Nowak, M. E., Beulig, F., Fischer, J., von, Muhr, J., Küsel, K., et al. (2015). Autotrophic fixation of geogenic CO2 by microorganisms contributes to soil organic matter formation and alters isotope signatures in a wetland mofette. Biogeosciences 12, 7169–7183. doi: 10.5194/bg-12-7169-2015
Oppermann, B. I., Michaelis, W., Blumenberg, M., Frerichs, J., Schulz, H. M., Schippers, A., et al. (2010). Soil microbial community changes as a result of long-term exposure to a natural CO2 vent. Geochim. Cosmochim. Acta 74, 2697–2716. doi: 10.1016/j.gca.2010.02.006
Oude Elferink, S. J., Akkermans-van Vliet, W. M., Bogte, J. J., and Stams, A. J. (1999). Desulfobacca acetoxidans gen. nov., sp. nov., a novel acetate-degrading sulfate reducer isolated from sulfidogenic granular sludge. Int. J. System. Evol. Microbiol. 49(Pt 2), 345–350. doi: 10.1099/00207713-49-2-345
Pankratov, T. A., Kirsanova, L. A., Kaparullina, E. N., Kevbrin, V. V., and Dedysh, S. N. (2012). Telmatobacter bradus gen. nov., sp. nov., a cellulolytic facultative anaerobe from subdivision 1 of the acidobacteria, and emended description of Acidobacterium capsulatum Kishimoto et al. 1991. Int. J. System. Evol. Microbiol. 62, 430–437. doi: 10.1099/ijs.0.029629-0
Patel, G. B., and Sprott, G. D. (1990). Methanosaeta concilii gen. nov., sp. nov. (“Methanothrix concilii”) and Methanosaeta thermoacetophila nom. rev., comb. nov. Int. J. System. Evol. Microbiol. 40, 79–82. doi: 10.1099/00207713-40-1-79
Peinado, M., García, J. L., González, E., and Ruiz, A. R. (2009). Itinerarios Ðeográficos y Paisajes Por la Provincia de Ciudad Real. Altagracia: Universidad de Castilla-La Mancha.
Pereira, R. P. A., Peplies, J., Brettar, I., and Hoefle, M. G. (2018). Impact of DNA polymerase choice on assessment of bacterial communities by a Legionella genus-specific next-generation sequencing approach. bioRxiv [Preprint]. doi: 10.1101/247445
Pfanz, H., Vodnik, D., Wittmann, C., Aschan, G., and Raschi, A. (2004). “Plants and geothermal CO2 exhalations — Survival in and adaptation to a high CO2 environment,” in Progress in Botany: Genetics Physiology Systematics Ecology, eds K. Esser, U. Lüttge, W. Beyschlag, and J. Murata (Berlin: Springer),499–538.
Podosokorskaya, O. A., Bonch-Osmolovskaya, E. A., Beskorovaynyy, A. V., Toshchakov, S. V., Kolganova, T. V., and Kublanov, I. V. (2014). Mobilitalea sibirica gen. nov., sp. nov., a halotolerant polysaccharide-degrading bacterium. Int. J. System. Evol. Microbiol. 64, 2657–2661. doi: 10.1099/ijs.0.057109-0
Raven, J. A. (1995). The early evolution of land plants: aquatic ancestors and atmospheric interactions. Bot. J. Scot. 47, 151–175. doi: 10.1080/03746609508684827
Rennert, T., Eusterhues, K., Pfanz, H., and Totsche, K. U. (2011). Influence of geogenic CO2 on mineral and organic soil constituents on a mofette site in the NW Czech Republic. Eur. J. Soil Sci. 62, 572–580. doi: 10.1111/j.1365-2389.2011.01355.x
Rennert, T., and Pfanz, H. (2016). Hypoxic and acidic — Soils on mofette fields. Geoderma 280, 73–81. doi: 10.1016/j.geoderma.2016.06.019
Rohrmüller, J., Kämpf, H., Geiß, E., Großmann, J., Grun, I., Mingram, J., et al. (2018). Reconnaissance study of an inferred quaternary maar structure in the western part of the bohemian massif near neualbenreuth, NE-Bavaria (Germany). Int. J. Earth Sci. 107, 1381–1405. doi: 10.1007/s00531-017-1543-0
Ross, D. J., Tate, K. R., Newton, P. C. D., Wilde, R. H., and Clark, H. (2000). Carbon and nitrogen pools and mineralization in a grassland gley soil under elevated carbon dioxide at a natural CO2 spring. Global Change Biol. 6, 779–790. doi: 10.1046/j.1365-2486.2000.00357.x
Sáenz de Miera, L. E., Arroyo, P., Luis Calabuig, E., de, Falagán, J., and Ansola, G. (2014). High-throughput sequencing of 16S RNA genes of soil bacterial communities from a naturally occurring CO2 gas vent. Int. J. Greenhouse Gas Control 29, 176–184. doi: 10.1016/j.ijggc.2014.08.014
Šmilauer, P., and Lepš, J. (2014). Multivariate Analysis of Ecological Data Using CANOCO 5. Cambridge: Cambridge university press. doi: 10.1017/CBO9781139627061
Smith, D. R., Doucette-Stamm, L. A., Deloughery, C., Lee, H., Dubois, J., Aldredge, T., et al. (1997). Complete genome sequence of Methanobacterium thermoautotrophicum deltaH: functional analysis and comparative genomics. J. Bacteriol. 179, 7135–7155. doi: 10.1128/jb.179.22.7135-7155.1997
Spear, J. R., Walker, J. J., McCollom, T. M., and Pace, N. R. (2005). Hydrogen and bioenergetics in the yellowstone geothermal ecosystem. Proc. Natl. Acad. Sci. U.S.A. 102, 2555–2560. doi: 10.1073/pnas.0409574102
Špičáková, L., Ulićný, D., and Koudelková, G. (2000). Tectonosedimentary evolution of the Cheb Basin (NW Bohemia, Czech Republic) between Late Oligocene and Pliocene: a preliminary note. Stud. Geophys. Geod. 44, 556–580. doi: 10.1023/A:1021819802569
Stackebrandt, E., Sproer, C., Rainey, F. A., Burghardt, J., Päuker, O., and Hippe, H. (1997). Phylogenetic analysis of the genus desulfotomaculum: evidence for the misclassification of desulfotomaculum guttoideum and description of desulfotomaculum orientis as Desulfosporosinus orientis gen. nov., comb. nov. Int. J. System. Evol. Microbiol. 47, 1134–1139. doi: 10.1099/00207713-47-4-1134
Steinberg, L. M., and Regan, J. M. (2009). mcrA-targeted real-time quantitative PCR method to examine methanogen communities. Appl. Environ. Microbiol. 75, 4435–4442. doi: 10.1128/AEM.02858-08
Stevens, T. O., and McKinley, J. P. (1995). Lithoautotrophic microbial ecosystems in deep basalt aquifers. Science 270, 450–455. doi: 10.1126/science.270.5235.450
Takai, K., Moser, D. P., DeFlaun, M., Onstott, T. C., and Fredrickson, J. K. (2001). Archaeal diversity in waters from deep South African gold mines. Appl. Environ. Microbiol. 67, 5750–5760. doi: 10.1128/AEM.67.21.5750-5760.2001
Videmšek, U., Hagn, A., Suhadolc, M., Radl, V., Knicker, H., Schloter, M., et al. (2009). Abundance and diversity of CO2-fixing bacteria in grassland soils close to natural carbon dioxide springs. Microb. Ecol. 58, 1–9. doi: 10.1007/s00248-008-9442-3
Vodnik, D., Maèek, I., Videmšek, U., and Hladnik, J. (2007). The life of plants under extreme CO2. Acta Biol. Sloven. 50:1.
Vuillemin, A., Friese, A., Alawi, M., Henny, C., Nomosatryo, S., Wagner, D., et al. (2016). Geomicrobiological features of ferruginous sediments from lake towuti, Indonesia. Front. Microbiol. 7:1007. doi: 10.3389/fmicb.2016.01007
Watanabe, T., Kojima, H., and Fukui, M. (2015). Sulfuriferula multivorans gen. nov., sp. nov., isolated from a freshwater lake, reclassification of ‘Thiobacillus plumbophilus’ as Sulfuriferula plumbophilus sp. nov., and description of Sulfuricellaceae fam. nov. and Sulfuricellales ord. nov. Int. J. System. Evol. Microbiol. 65, 1504–1508. doi: 10.1099/ijs.0.000129
Weinlich, F. H., Bräuer, K., Kämpf, H., Strauch, G., Tesař, J., and Weise, S. M. (1999). An active subcontinental mantle volatile system in the western Eger rift, Central Europe: gas flux, isotopic (He, C, and N) and compositional fingerprints. Geochim. Cosmochim. Acta 63, 3653–3671. doi: 10.1016/S0016-7037(99)00187-8
Yarza, P., Yilmaz, P., Pruesse, E., Glöckner, F. O., Ludwig, W., Schleifer, K.-H., et al. (2014). Uniting the classification of cultured and uncultured bacteria and archaea using 16S rRNA gene sequences. Nat. Rev. Microbiol. 12, 635–645. doi: 10.1038/nrmicro3330
Young, J. N., Rickaby, R. E. M., Kapralov, M. V., and Filatov, D. A. (2012). Adaptive signals in algal Rubisco reveal a history of ancient atmospheric carbon dioxide. Philos. Total R. Soc. B 367, 483–492. doi: 10.1098/rstb.2011.0145
Zhang, J., Kobert, K., Flouri, T., and Stamatakis, A. (2014). PEAR: a fast and accurate illumina paired-end reAd mergeR. Bioinformatics (Oxford, England) 30, 614–620. doi: 10.1093/bioinformatics/btt593
Keywords: geo–bio interaction, elevated CO2 concentration, paleo-sediment, deep biosphere, acidophilic microorganisms, Acidobacteriaceae, Acidithiobacillus, Acidothermus
Citation: Liu Q, Kämpf H, Bussert R, Krauze P, Horn F, Nickschick T, Plessen B, Wagner D and Alawi M (2018) Influence of CO2 Degassing on the Microbial Community in a Dry Mofette Field in Hartoušov, Czech Republic (Western Eger Rift). Front. Microbiol. 9:2787. doi: 10.3389/fmicb.2018.02787
Received: 13 August 2018; Accepted: 30 October 2018;
Published: 21 November 2018.
Edited by:
Mark Alexander Lever, ETH Zürich, SwitzerlandReviewed by:
Felix Beulig, Aarhus University, DenmarkPhilippe Constant, Institut National de la Recherche Scientifique (INRS), Canada
Copyright © 2018 Liu, Kämpf, Bussert, Krauze, Horn, Nickschick, Plessen, Wagner and Alawi. This is an open-access article distributed under the terms of the Creative Commons Attribution License (CC BY). The use, distribution or reproduction in other forums is permitted, provided the original author(s) and the copyright owner(s) are credited and that the original publication in this journal is cited, in accordance with accepted academic practice. No use, distribution or reproduction is permitted which does not comply with these terms.
*Correspondence: Mashal Alawi, ZW1haWxAbWFzaGFsLWFsYXdpLmRl