- 1Campus Food Science, Department of Agricultural and Food Sciences, Alma Mater Studiorum, University of Bologna, Cesena, Italy
- 2Leibniz Institute for Agricultural Engineering and Bioeconomy, Quality and Safety of Food and Feed, Potsdam, Germany
Essential oils (EOs) or their components represent one of the most promising natural, safe, and feasible alternatives to prevent the growth of food-borne pathogens like Listeria monocytogenes and Escherichia coli in food matrices. Although antimicrobial properties of EOs and their components are well-documented, limited and fragmented information is available on the changes induced by these compounds, even at sub-lethal concentrations, in the physiological properties of microbial cells. The aim of this study was to explore the morpho-physiological changes of L. monocytogenes Scott A and E. coli MG 1655 induced after 1 h exposure to different sub-lethal and lethal concentrations of citral, carvacrol, (E)-2-hexenal, and thyme EO. For this purpose, different cell viability parameters such as membrane integrity, esterase activity, and cytoplasmic cell membrane potential were measured by flow cytometry. Flow cytometric data revealed specific response patterns in relation to the strain, the natural antimicrobial and its concentrations. Both the target microbial strains showed an increased cell membrane permeabilization without a loss of esterase activity and cell membrane potential with increasing citral, carvacrol and thyme EO concentrations. By contrast, (E)-2-hexenal did not significantly affect the measured physiological properties of L. monocytogenes Scott A and E. coli MG 1655. The used approach allowed identifying the most effective natural antimicrobials in relation to the microbial target.
Introduction
Consumer’s demand for minimally processed and ready-to-eat foods with a reduced content of synthetic preservatives has stimulated the research of alternative preservation strategies. Essential oils (EOs) or their components represent one of the most promising natural feasible alternatives to improve food safety, shelf-life and quality. Recognized as safe from international food authorities they are traditionally used in food industry as flavor and taste enhancers (Newberne et al., 2000). Their antimicrobial activity and the wide action spectra against several pathogenic and spoilage microorganisms are well-documented and several reviews are available (Burt, 2004; Hyldgaard et al., 2012; Tongnuanchan and Benjakul, 2014; Patel, 2015; Pandey et al., 2017). A wide literature documented their application as natural preservatives also in different food matrices, such as meat (Fratianni et al., 2010; Barbosa et al., 2015; Radha Krishnan et al., 2015), dairy products (Amatiste et al., 2014; Ehsani et al., 2016; Ben Jemaa et al., 2017), minimally processed fruits and vegetables (Patrignani et al., 2015; Siroli et al., 2015b,c) and beverages (Kiskó and Roller, 2005; Chueca et al., 2016). Among the natural antimicrobials, thyme EO, and some components of citrus and officinal EOs, such as citral, carvacrol, and (E)-2-hexenal, are very promising alternatives to traditional preservatives (Ivanovic et al., 2012). In fact, they are widely reported to be able to improve safety and shelf-life of several foods even when used at concentrations lower than their minimal bactericidal concentrations. In fact, their application in foods is subordinated to the usage of concentrations not detrimental for the product sensory properties (Zanini et al., 2014a,b; Silva-Angulo et al., 2015). In general, the cell wall, the cytoplasmic membrane and membrane proteins have been considered as the main targets of EOs and their components (Burt, 2004). However, the EO action mechanisms are reported to vary according to the natural antimicrobial and its concentration, the target species or strain, the food matrix, the storage conditions, etc. (Valero and Francés, 2006; Somolinos et al., 2008, 2010; Patrignani et al., 2013). As described by Burt (2004) and Hyldgaard et al. (2012), the primary site of toxic action of terpenes like citral and carvacrol is represented by the cell membrane due to their hydrophobic properties.
As reported by Somolinos et al. (2010), the exposure to different citral concentrations caused a disruption of the cytoplasmic cell membrane of Escherichia coli BJ4 and BJ4L1. A similar permeabilization effect was also observed for different Listeria monocytogenes strains. As reported by Zanini et al. (2014a,b), the exposure to citral and carvacrol increased the permeabilization of the cytoplasmic cell membrane and potentiated the activity of various antibiotics even at sub-lethal concentrations.
Additionally, the cytosol coagulation and the depletion of the microbial cell proton-motive force have been identified as action mechanisms of EOs (Burt, 2004; Hyldgaard et al., 2012; Patel, 2015). Aldehydes such as hexanal and (E)-2-hexenal are antimicrobials produced by plants and vegetable tissues which are damaged by biotic or abiotic stresses throughout the lipoxygenase pathway to prevent and/or inhibit the growth of plant pathogens (Lanciotti et al., 2004). It has been demonstrated that such tissue show a noticeable activity against several yeasts, molds, Gram-positive and Gram-negative bacterial strains of food interest (Nakamura and Hatanaka, 2002; Trombetta et al., 2002; Zhang et al., 2017) both in model and real food systems (Lanciotti et al., 1999, 2003; Siroli et al., 2014). As reported by Patrignani et al. (2008), (E)-2-hexenal acts as a surfactant and permeates by passive diffusion across the plasma membrane of many microorganisms. After reaching the cytoplasm, the α,β-unsaturated aldehyde is able to react with different nucleophilic groups (Kubo and Fujita, 2001; Lanciotti et al., 2004). Moreover, (E)-2-hexenal may cause cytoplasm coagulation as a result of thiol containing enzyme inhibition (Aiemsaard et al., 2011). The antimicrobial properties of thyme EO depend on its chemical composition and the target microorganism (Kim et al., 1995; Nevas et al., 2004; Sienkiewicz et al., 2011; Picone et al., 2013; Boskovic et al., 2015; Siroli et al., 2015a,c; Swamy et al., 2016).
Thyme EO is constituted of numerous different compounds, but its antimicrobial activity is mainly attributed to carvacrol and thymol. Thymol is structurally similar to carvacrol and they share their cellular targets. Studies have shown that thymol interacts with cell membrane permeability, leading to a depletion of membrane potential, cellular uptake of ethidium bromide, and leakage of potassium ions, ATP, and carboxyfluorescein (Helander et al., 1998; Lambert et al., 2001; Xu et al., 2008). Although literature regarding the action mechanisms of citral, carvacrol, (E)-2-hexenal, and thyme EO has been dramatically increased in the last years, the knowledge on their mechanisms on L. monocytogenes and E. coli is still fragmentary since it is affected by several factors such as concentration, strains, cell physiological state, treatment conditions, microbial interaction with exposure systems, etc.
In addition, antimicrobial activity of EOs and their components are not attributable to a specific mechanism but to the actions toward several cell targets. Moreover, for EOs a holistic approach should be considered, since synergistic actions among present components greatly affects their antimicrobial activities also at very low concentrations (Caccioni et al., 1997), and, consequently, the understanding of their action mechanisms becomes more complex. In addition, a heterogeneity in microbial population resistance to stress is reported to occur as a monomodal Gaussian with a narrow or broad distribution, or as a multimodal distribution comprising subpopulations of similar or vastly different numbers of individuals (Dhar and McKinney, 2007). However, the literature on the behavior of L. monocytogenes and E. coli cell populations exposed to natural antimicrobials is still scarce (Burt, 2004; Bakkali et al., 2008; Hyldgaard et al., 2012).
Flow cytometry represents a reliable and fast tool in food microbiology for the measurements of the changes on physiological single cell properties. By the use of the appropriate fluorescent dyes, it is possible to classify cells into three different categories: metabolically active, intact, or permeabilized cell mixtures (Hewitt and Nebe-Von-Caron, 2004; Johnson et al., 2013). Most common fluorescent dyes used in flow cytometry are fluorescent immune-conjugates and probes for fluorescence in situ hybridization and nucleic acid stains. In addition, several probes capable of measuring the membrane potential as well as cell enzymatic activity, viability, organelles, phagocytosis, development, and other properties are available (Haugland, 1994). Various authors demonstrated the suitability of flow cytometry to study the microbial cell responses even after the exposure to sub-lethal stress conditions (Luscher et al., 2004; Ananta et al., 2005; Berney et al., 2007; Mathys et al., 2007; Sunny-Roberts and Knorr, 2008; Da Silveira and Abee, 2009; Mols et al., 2010; Fröhling et al., 2012; Tamburini et al., 2013; Fröhling and Schlüter, 2015). In fact, this technique provides several information on the whole cell population and its changes during the exposure to stresses and the following recovery during storage. The knowledge of the behavior of the different sub-populations after exposure to natural antimicrobials is fundamental for their further application at industrial level as alternative to traditional preservatives, also to avoid resistance phenomena.
In this framework, the main aim was to investigate the potential of flow cytometry to study the changes of morphological and physiological properties of the food-borne pathogen L. monocytogenes Scott A and the indicator strain E. coli MG 1655, after 1 h exposure to different sub-lethal and lethal concentrations of citral, carvacrol, (E)-2-hexenal and thyme EO in order to clarify their specific action mechanisms and the responses of the whole cell population. For these purposes, different cell viability parameters, such as membrane integrity, esterase activity and cytoplasmic cell membrane potential were measured by flow cytometry.
Materials and Methods
Natural Antimicrobials
Some EOs (citral, carvacrol, and (E)-2-hexenal) used in these experiments were purchased from Sigma-Aldrich (Milan, Italy) while thyme EO was obtained from Flora s.r.l. (Pisa, Italy). Before conducting the experiments, EOs were properly diluted using absolute ethanol (Sigma-Aldrich, Milan, Italy) to prepare 100X EO stock solutions.
Bacterial Strains
Listeria monocytogenes Scott A and E. coli MG 1655 were stored as glass bead cultures at -80°C for long-term preservation. To acclimatize cultures to the experimental conditions, one glass bead of each strain was given to 5 ml of Brain Heart Infusion broth (BHI) (Thermo-fisher, Milan, Italy) and incubated for 24 h without shaking at 37°C. After growth, cells were sub-cultured at 37°C for 24 h in BHI broth.
Exposure to Natural Antimicrobials
In each assay, 250 mL of fresh BHI broth was inoculated with 2.5 mL of bacteria suspension (corresponding to 1% of the final volume) to reach a 4 log CFU/mL concentration and incubated without stirring at 37°C. The growth was monitored by measuring the optical density (OD) at λ = 600 nm. For L. monocytogenes Scott A the exposure was performed in the middle of the exponential growth phase while for E. coli MG 1655 the exposure was performed in the stationary growth phase (OD = 2, λ = 600 nm). For both microbial strains, 200 μL of natural antimicrobial hydro alcoholic stock solutions was added to 20 mL of liquid cultures in order to obtain the concentrations reported in Tables 1, 2. Cultures were incubated for 1 h at 37°C.
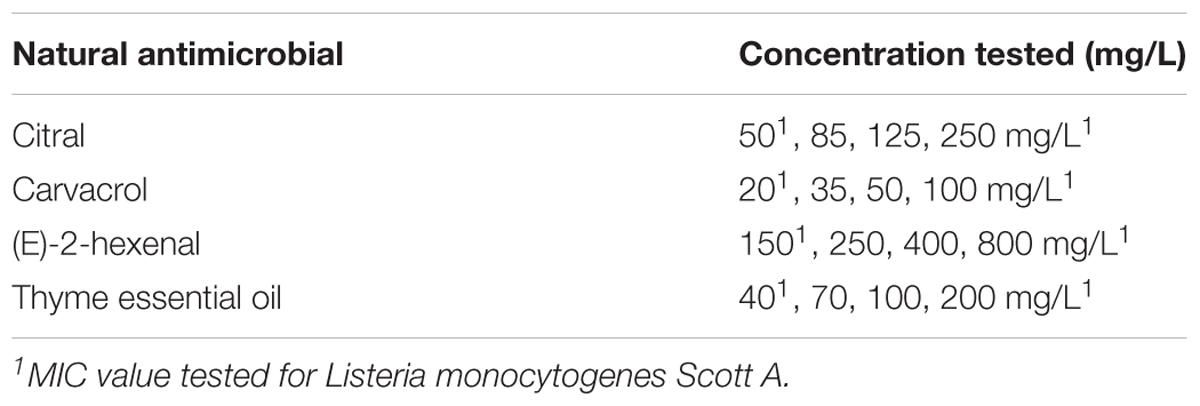
TABLE 1. Essential oils, their components, and relative concentrations, used for the treatments of L. monocytogenes Scott A.
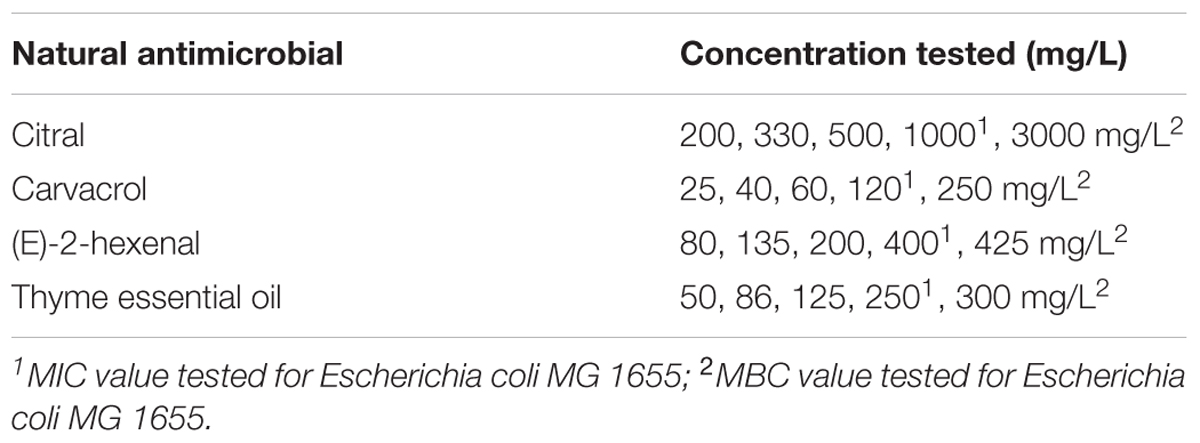
TABLE 2. Essential oils, their components, and relative concentrations, used for the treatments of Escherichia coli MG 1655.
For L. monocytogenes Scott A the natural antimicrobial operative concentrations were determined according to the minimum inhibitory concentration standard method (MIC) as previously described by Siroli et al. (2015a,b). For each selected natural antimicrobial, L. monocytogenes cells were exposed to three sub-lethal concentrations corresponding to the 1/5, 1/3, 1/2 of the MIC value as well as to the MIC values (Table 1). For E. coli MG 1655, the minimum inhibitory concentration (MIC) and minimum bactericidal concentration (MBC) of the selected natural antimicrobials were determined in preliminary studies according to the protocol described by Siroli et al. (2015a,b) (data not shown). E. coli MG 1655 cells were exposed to different natural antimicrobials in sub-lethal concentrations (corresponding to 1/5, 1/3, 1/2 of the MIC values) as well as to the MIC and MBC values (Table 2). After exposure, the total viable cell count of L. monocytogenes Scott A and E. coli MG 1655 was immediately performed. Afterward, bacterial cells were harvested by centrifugation at 3214 × g and 4°C for 15 min, resuspended in 250 μL of phosphate buffered saline (PBS, 50 mM) and centrifuged at 7000 × g and 4°C for 5 min. For the subsequent staining procedures and flow cytometric analysis, L. monocytogenes pellets were resuspended in 100 μL (50 mM) PBS, while E. coli samples were resuspended in 100 μL (50 mM) Tris buffer. For both microorganisms the final cell density of each sample was about 109 cells/mL.
Total Viable Cell Count
The total viable cell count of bacteria after exposure to EOs or their components was determined by plate count methods in duplicate. Samples were serially diluted in microtest plates (96er U-profile, Carl Roth GmbH & Co KG, Germany) using physiological saline buffer (9 g/L NaCl) as dilution solution. 100 μL of each dilution was spread on BHI agar (Thermo Fisher Scientific, Ltd., Milan, Italy) and the growth (colony forming units) was evaluated after 24 h at 37°C.
Flow Cytometric Analysis
All experiments were performed using a CyFlow ML flow cytometer (Sysmex Partec GmbH, Görlitz, Germany) equipped, among others, with a 50 mW blue solid state laser emitting at a wavelength of 488 nm. A photomultiplier with a band pass filter of 536 ± 20 nm was used to collect fluorescence data of thiazole orange (TO), carboxyfluorescein (cF), and green DiOC2(3), while the fluorescence of propidium iodide and red DiOC2(3) was recorded in the photomultiplier with a band pass filter of 620 ± 11 nm. To correct the overlap of one dye’s emission into another dye’s detector, fluorescence signal compensation was performed. Data obtained from each photomultiplier channels were collected as logarithmic signals and analyzed using the FloMax software 3.0 (Sysmex Partec GmbH, Görlitz, Germany). For each sample, one hundred thousand events were measured at a flow rate of approximately 3000 events/sec. According to Fröhling et al. (2012), the density plots obtained by flow cytometric analyses were divided into four regions. Each region is associated with cells revealing different physiological or morphological properties. The average of the percentage values obtained from three density plots was calculated and illustrated as diagrams where the treatment concentration and the percentage of fluorescent cells is displayed by the x-axis and the y-axis, respectively. The different cell parameters investigated during this experimentation were: cell membrane integrity (TO-PI staining), cell membrane potential (DiOC2(3) staining), and cell membrane integrity and esterase activity (cF-PI staining) as indicator of the microbial population viability. Staining procedures were performed as described earlier by Fröhling and Schlüter (2015) with some adaptations to the bacterial species used.
Membrane Integrity
Cell membrane integrity was evaluated after the treatments using a combination of thiazole orange (TO) and propidium iodide (PI) dyes (Sigma-Aldrich KGaA, Darmstadt, Germany). Staining procedures were performed in the dark with some variations depending on the microorganisms tested.
For L. monocytogenes, 20 μL of resuspended pellets was diluted in PBS (50 mM) to a cell density of approximately 106 cells/mL. Thiazole orange (0.2 μM) was added to the samples and incubated for 10 min at room temperature. After incubation, 30 μM propidium iodide was added and samples were analyzed after 5 min.
As described above, E. coli resuspended pellets were diluted in Tris buffer (50 mM) to a cell density of approximately 106 cells/mL. Thiazole orange was added to a final concentration of 2.5 μM and samples were incubated for 15 min at room temperature. Propidium iodide staining was performed as described for L. monocytogenes samples.
Esterase Activity and Membrane Permeabilization
The cell esterase activity and the membrane integrity were evaluated after the exposure to natural antimicrobials using 5(6)-carboxyfluorescein diacetate mixed isomers (cFDA) (Sigma-Aldrich, KGaA, Darmstadt, Germany) and PI. A volume of 60 μL of concentrated L. monocytogenes samples were stained with an equal volume of cFDA (200 μM) stock solution to obtain a final cFDA concentration of 100 μM, incubated at 37°C in a water bath for 5 min and centrifuged at 7000 × g and 4°C for 5 min. Cell pellets were diluted 1:1 in PBS (50 mM). The PI staining procedure was performed as previously described. The cFDA staining procedure for E. coli followed the protocol described for L. monocytogenes with some differences. Cells were incubated with 833 μM cFDA (in 50 mM Tris) at 37°C in a water bath for 45 min and then centrifuged at 7000 × g and 4°C for 5 min. The pellets were resuspended in 60 μL Tris (50 mM) and stained with 30 μM PI. Samples were analyzed after 10 min of incubation.
Membrane Potential
The membrane potential of bacteria cells was measured using 3,3′-diethyloxacarbocyanine iodide [DiOC2(3)] provided by Sigma-Aldrich, Germany. The protocol applied was in agreement with Novo et al. (1999) and Fröhling and Schlüter (2015) with further modifications. L. monocytogenes suspensions were diluted in 50 mM PBS containing 10 mM D-Glucose and 30 μM DiOC2(3) and then incubated for 15 min at room temperature in the dark. Afterward the suspension was centrifuged at 7000 × g and 4°C for 5 min and the pellet was resuspended in 1 mL PBS (50 mM). E. coli staining procedure required a different staining buffer due to the higher complexity of the outer and inner cell membrane. First, samples were suspended in 10 mM D-Glucose, 30 μM DiOC2(3) and EDTA (0.5 mM) Tris buffer (50 mM). After the centrifugation and the incubation time as previously described, E. coli samples were resuspended in 1 mL of Tris (50 mM). After the staining procedure samples were immediately analyzed to detect shifts in the cell membrane potential. The ratio of the mean red to the mean green DiOC2(3)-fluorescence channel value was calculated to investigate changes in the membrane potential. Due to the chosen cytometer settings the red/green DiOC2(3)-fluorescence ratio of depolarized cells was ≤1. It was assumed that the red/green ratio of untreated cells represents the relative membrane potential of intact cells (Novo et al., 1999). A reduction of the red/green ratio reflects the loss of cell membrane potential.
Statistical Analysis
Statistical analyses were performed to evaluate significant differences between samples using the R software (R Core Development Team, 2017). One way-ANOVA with Tukey test with a significance level of 0.05 was used.
Results
Treatment Effects on Total Viable Count of Listeria monocytogenes Scott A
The exposure of L. monocytogenes cells to the natural antimicrobials at different concentrations was performed in the middle of the exponential growth phase (OD = 0.4; λ = 600 nm). In all trials, the cell loads before the treatments were about 8.8 log CFU/mL (Tables 3, 4). After 1 h exposure, the untreated controls and the samples exposed to 1% ethanol showed the same cell counts. Only the 1 h exposure to carvacrol and thyme EO resulted in a significant reduction of the total viable counts at the highest concentration tested (Tables 3, 4). More specifically, the exposure to 100 mg/L of carvacrol which is corresponding to the MIC value, reduced the viable cell load by one logarithmic cycle (7.59 log CFU/mL) (Table 3B). A more severe effect was observed after the exposure to thyme EO, reducing the viable counts to 7.45 log CFU/mL and 5.23 CFU/mL after the exposure to 100 and 200 mg/L, respectively (Table 4D). In contrast, 1 h exposure to citral and (E)-2-hexenal produced no significant effect on cell loads of L. monocytogenes Scott A cells (Tables 3A, 4C).
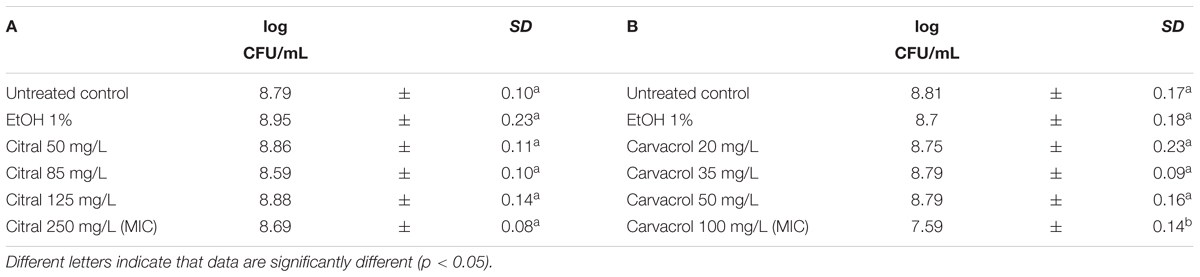
TABLE 3. Total viable counts of Listeria monocytogenes Scott A after 1 h exposure to different concentrations of Citral (A) and Carvacrol (B).
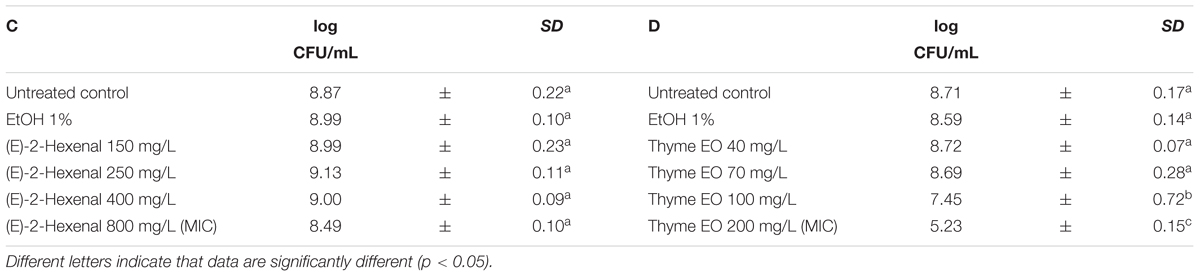
TABLE 4. Total viable counts of Listeria monocytogenes Scott A after 1 h exposure to different concentrations of (E)-2-hexenal (C) and Thyme EO (D).
Treatment Effects on Total Viable Count of Escherichia coli MG 1655
Escherichia coli MG 1655 samples were exposed to EO or their bioactive compounds at the beginning of the stationary growth phase (OD = 2; λ = 600 nm). The cell loads of E. coli before the exposure to the natural antimicrobials ranged between 8.5 and 9.0 log CFU/mL (Tables 5, 6). Analogously to L. monocytogenes, no differences on the cell loads were highlighted between the untreated controls and the samples exposed to 1% EtOH (Tables 5, 6). The highest effect on E. coli was observed after the exposure to citral, even at lowest concentration tested. The exposure to 200 mg/L reduced the viable cell load to 7.22 log CFU/mL. Increasing concentrations reduced the total viable counts to values ranging between 6.60 and 6.12 CFU/mL. The exposure to the citral MBC concentration caused a reduction of cell loads below the detection limit (Table 5A). Also thyme EO and carvacrol treatments significantly decreased the total viable cell loads. A reduction of three logarithmic cycles were observed after the exposure to carvacrol MBC concentration (250 mg/L), while an exposure to thyme EO MIC and MBC concentrations reduced cell load to 6.52 and 5.59 log CFU/mL, respectively (Tables 5B, 6D). (E)-2-hexenal exposure did not affect the cell loads of E. coli (Table 6C).
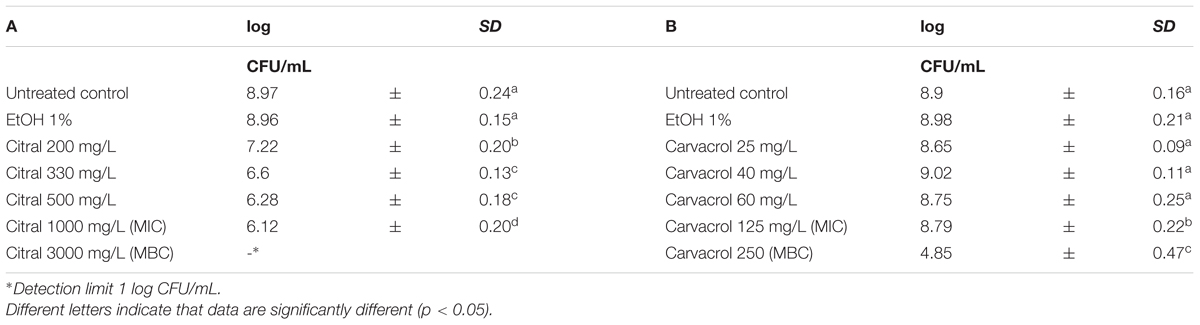
TABLE 5. Total viable count of Escherichia coli MG 1655 after 1 h exposure to Citral (A) and Carvacrol (B).
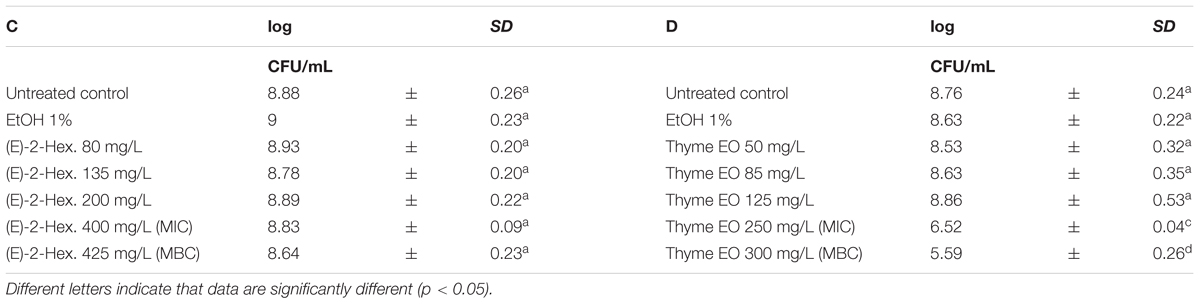
TABLE 6. Total viable count of Escherichia coli MG 1655 after 1 h exposure to (E)-2-hexenal (C) and Thyme EO (D).
Treatment Effects on the Membrane Integrity of Listeria monocytogenes Scott A
The exposure of L. monocytogenes Scott A to different natural antimicrobials caused an augment of the fractions with cells having slightly permeabilized cell membranes (PI + TO stained cells) and permeabilized cell membranes (PI stained cells). The distribution of stained cells varied according to different treatments and concentrations used. The percentage of L. monocytogenes Scott A with intact cell membranes remained almost constant (above 80%) after exposure to 1% ethanol in all the performed trials (Figure 1). The different concentrations of citral (Figure 1A) increased the percentages of cells with slightly permeabilized cell membranes. The magnitude of the damaging effect rose with increasing citral concentrations. In fact, at the highest concentration (250 mg/L) 60% of stained cells showed a slightly permeabilized cell membrane. A value of about 3% cells with permeabilized cell membrane was observed in all the conditions independently on the severity of chemical stress applied (citral concentration). A similar pattern was observed for carvacrol (Figure 1B). All concentrations tested induced a significant reduction in the population with intact cell membranes. In particular, while in the control samples the population with intact cell membranes was about 80%, this value decreased to 25% in the samples exposed to 100 mg/L carvacrol (MIC value). Simultaneously, the percentage of cells with slightly permeabilized cell membranes increased from 12 to 50% augmenting the carvacrol concentrations. The exposure to the MIC carvacrol concentration also raised the percentage of cells with permeabilized cell membranes up to 15%. Compared to the untreated control, the exposure to (E)-2-hexenal had no significant effect on the cell membrane integrity of L. monocytogenes Scott A (Figure 1C). Thyme EO had the highest effect on the cell membrane integrity compared to the other natural antimicrobials tested. The percentage of slightly permeabilized and completely permeabilized cells increased with the treatment concentrations, and the exposure to 200 mg/L of thyme EO (MIC concentration) induced a complete membrane permeabilization in more than 90% of the cell population (Figure 1D). Except for Thyme EO exposure, the percentage values of unstained cells/cell fragments were lower than 3% independently on the antimicrobial and its concentration (Figure 1).
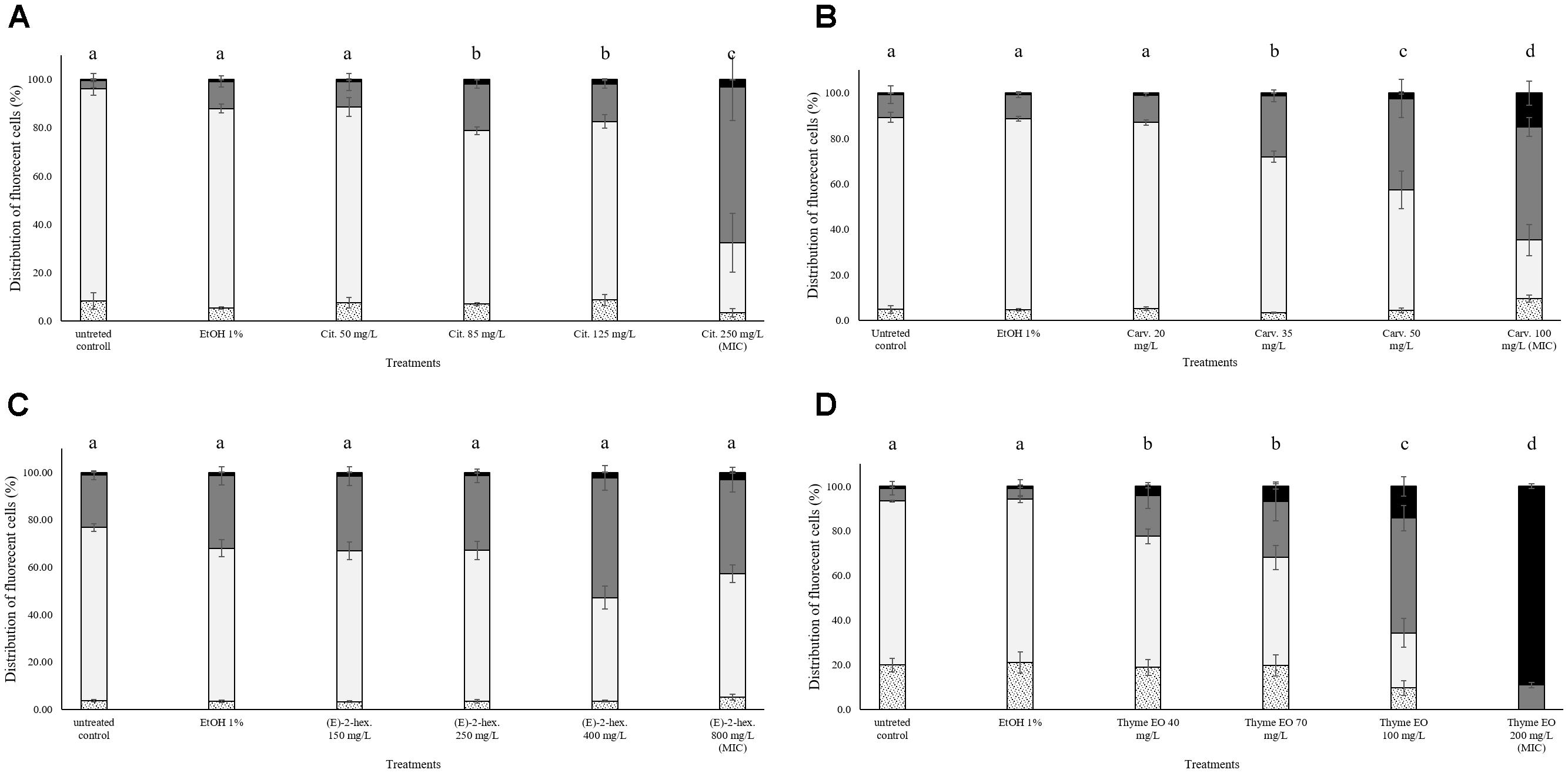
FIGURE 1. Membrane integrity of Listeria monocytogenes Scott A after 1 h exposure to different concentrations of natural antimicrobials: Citral (A), Carvacrol (B), (E)-(2)-hexenal (C), and thyme EO (D). White bars with dots represent cell fragments or unstained cells; White bars represent cells with intact cell membrane; Gray bars represent cells with slight cell membrane permeabilization; Black bars represent cells with (complete) cell membrane permeabilization. Different letters indicate that data are significantly different (p < 0.05).
Treatment Effects on the Esterase Activity and Membrane Integrity of Listeria monocytogenes Scott A
No effects on the esterase activity of L. monocytogenes cells were evidenced after the exposure to natural antimicrobials or their bioactive compounds. As shown in Figure 2, the percentage of fluorescent cells with intact cell membranes and esterase activity was constantly above 80% with the only one exception of thyme EO 200 mg/L exposure. The MIC thyme EO exposure caused a complete permeabilization of the cells, however, these cells still showed esterase activity (Figure 2D).
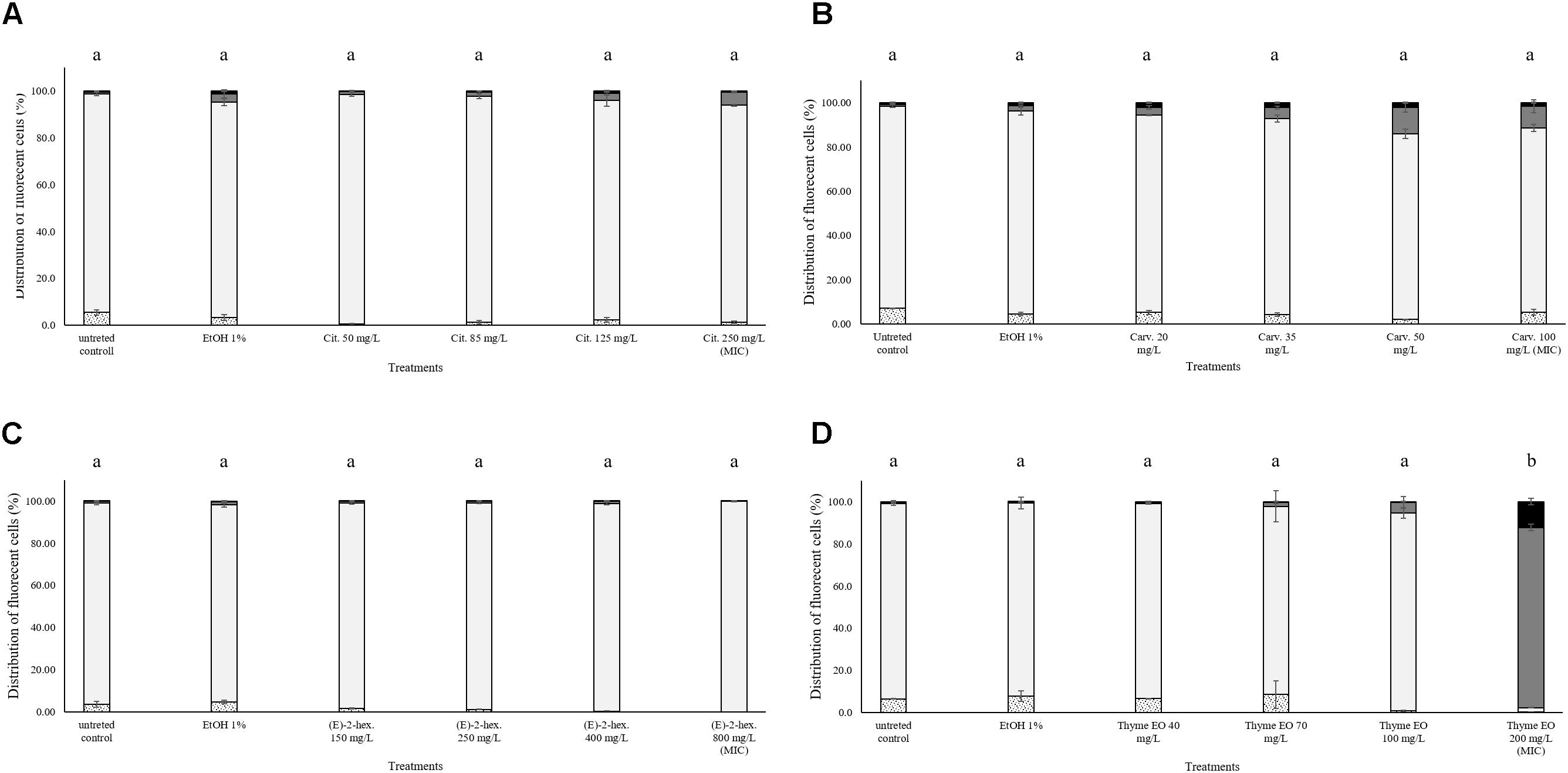
FIGURE 2. Esterase activity and membrane integrity of L. monocytogenes Scott A after 1 h exposure to natural antimicrobials: Citral (A), Carvacrol (B), (E)-(2)-hexenal (C), and thyme EO (D). White bars with dots represent cell fragments or unstained cells; White bars represent cells with intact cell membrane and esterase activity; Gray bars represent cell membrane permeabilization but still existing esterase activity; Black bars represent cells with cell membrane permeabilization but without esterase activity. Different letters indicate that data are significantly different (p < 0.05).
Treatment Effects on the Cell Membrane Potential of Listeria monocytogenes Scott A
The measurement of the relative membrane potential using 3,3′-Diethyloxacarbocyanine iodide DiOC2(3) showed no significant differences between untreated and treated cells of L. monocytogenes Scott A. In fact, red/green ratios were always lower than the value 1 independently on the treatments and concentrations used (Figure 3).
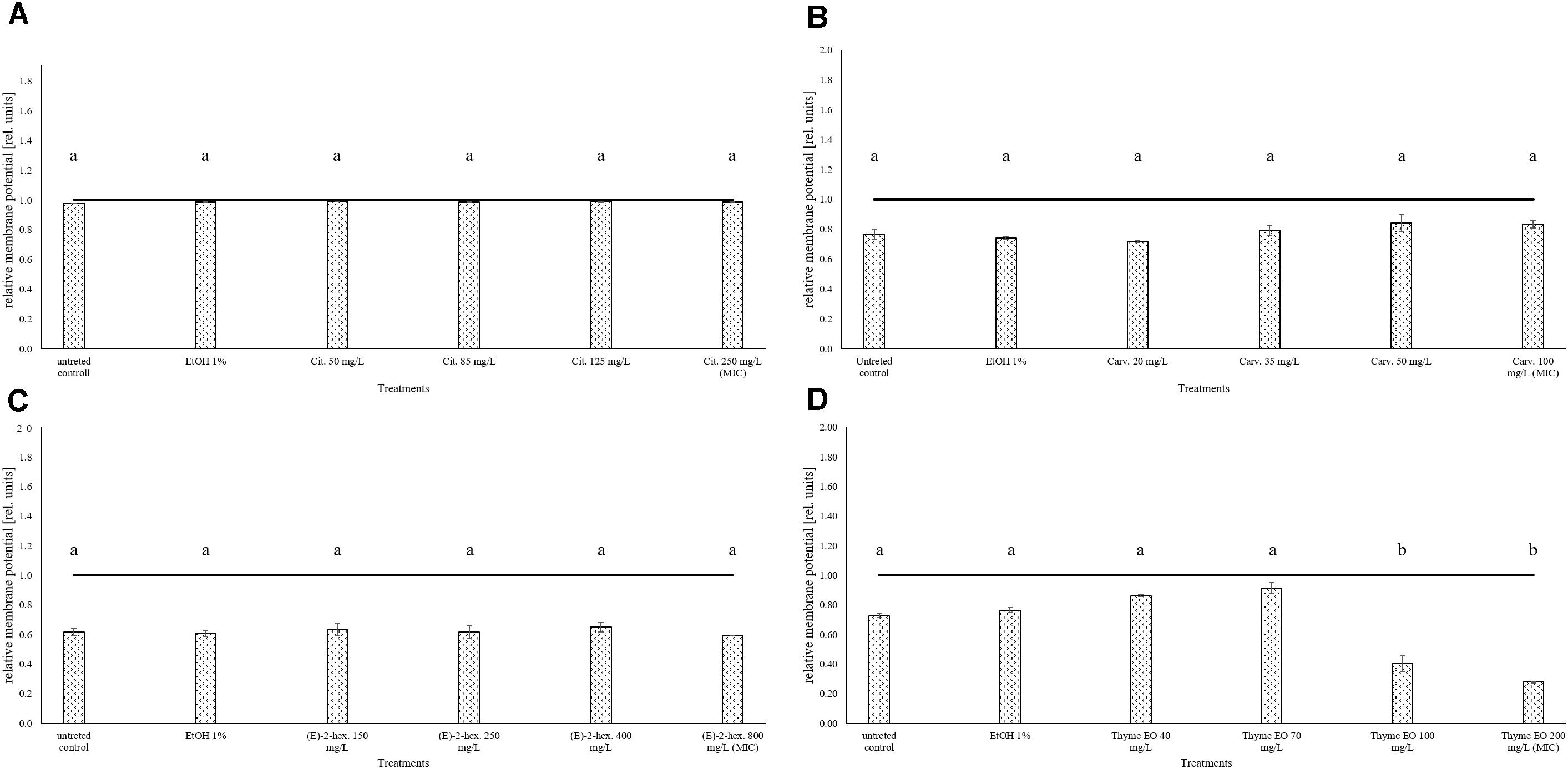
FIGURE 3. Relative membrane potential of L. monocytogenes Scott A, expressed as red/green ratio of DiOC2(3)-fluorescence intensity, after 1 h exposure to natural antimicrobials: Citral (A), Carvacrol (B), (E)-(2)-hexenal (C), and thyme EO (D). The black line represents the threshold between polarized (ratio > 1) and depolarized (ratio < 1) cells. Different letters indicate that data are significantly different (p < 0.05).
Treatment Effects on Membrane Integrity of Escherichia coli MG 1655
In all trials, the percentage of E. coli stained only with TO (cells with intact cell membrane) remained almost constant (above 80%) after the exposure to 1% ethanol (Figure 4). Citral determined a significant effect on cell membrane integrity even at sub-lethal concentrations (Figure 4A). After exposure to 200–300 mg/L citral, the percentages of cells with slightly permeabilized cell membranes were higher than 80%. No cells with intact cell membrane were found after the exposure to citral MIC and MBC values (Figure 4A). Minor impacts on E. coli cell membrane integrity were evidenced after the exposure to carvacrol and (E)-2-hexenal, independently on their sub-lethal concentrations (Figures 4B,C). Only the exposure to carvacrol inhibition and bactericidal concentrations (120–250 mg/L) triggered cell membrane permeabilization (Figure 4B). The effect of thyme EO on the cell membrane was dependent on the applied concentration. The fluorescence signal of intact cells decreased (80–20%) with an increase of the treatment concentration, while the percentage of cells with permeabilized cell membranes increased simultaneously (Figure 4D).
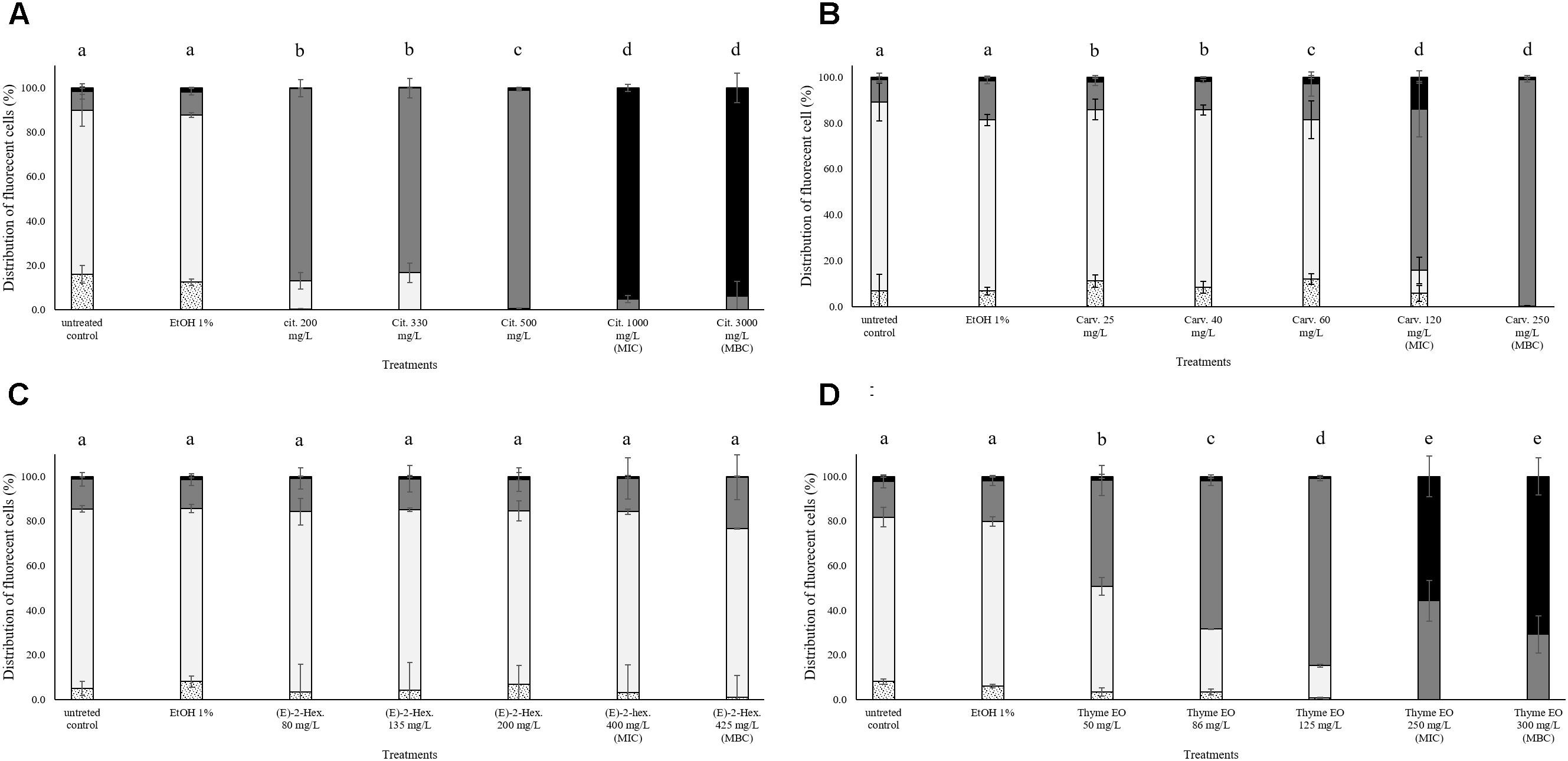
FIGURE 4. Membrane integrity of Escherichia coli MG 1655 after 1 h exposure to different concentrations of natural antimicrobials: Citral (A), Carvacrol (B), (E)-(2)-hexenal (C), and thyme EO (D). White bars with dots represent cell fragments or unstained cells; White bars represent cells with intact cell membranes; Gray bars represent cells with slightly permeabilized cell membranes; Black bars represent cells with (complete) with permeabilized cell membranes. Different letters indicate that data are significantly different (p < 0.05).
Treatment Effects on Esterase Activity and Membrane Integrity of Escherichia coli MG 1655
The exposure to citral induced cell membrane permeabilization of E. coli without a loss of esterase activity. The percentage of fluorescent cells with permeabilized cell membranes but still existing esterase activity was higher than 80% independent of the natural antimicrobial concentrations. Only the exposure to bactericidal concentrations (1000 and 3000 mg/L citral) increased (10%) the amounts of the populations with permeabilized cell membranes and without esterase activity (Figure 5A). Minor impacts on E. coli cell membrane integrity and esterase activity were evidenced after the exposure to carvacrol and (E)-2-hexenal. E. coli samples treated with the bactericidal concentrations (120 and 250 mg/L) of carvacrol showed a cell membrane permeabilization without a loss of esterase activity (Figure 5B). The exposure to (E)-2-hexenal increased the percentage of cell fragments or unstained cells, independently on the concentration used. They represented about the 15% of the whole population in all the conditions tested (Figure 5C). The effects of thyme EO on the cell membrane and esterase activity were related to the concentration tested. The fluorescence signal of the intact cells decreased (80–30%) with the exposure to the sub-lethal concentration tested while the percentage of cell populations with permeabilized cell membranes and esterase activity increased (Figure 5D). A significant loss in the cell esterase activity was only observed after the exposure to 250 and 300 mg/L thyme EO.
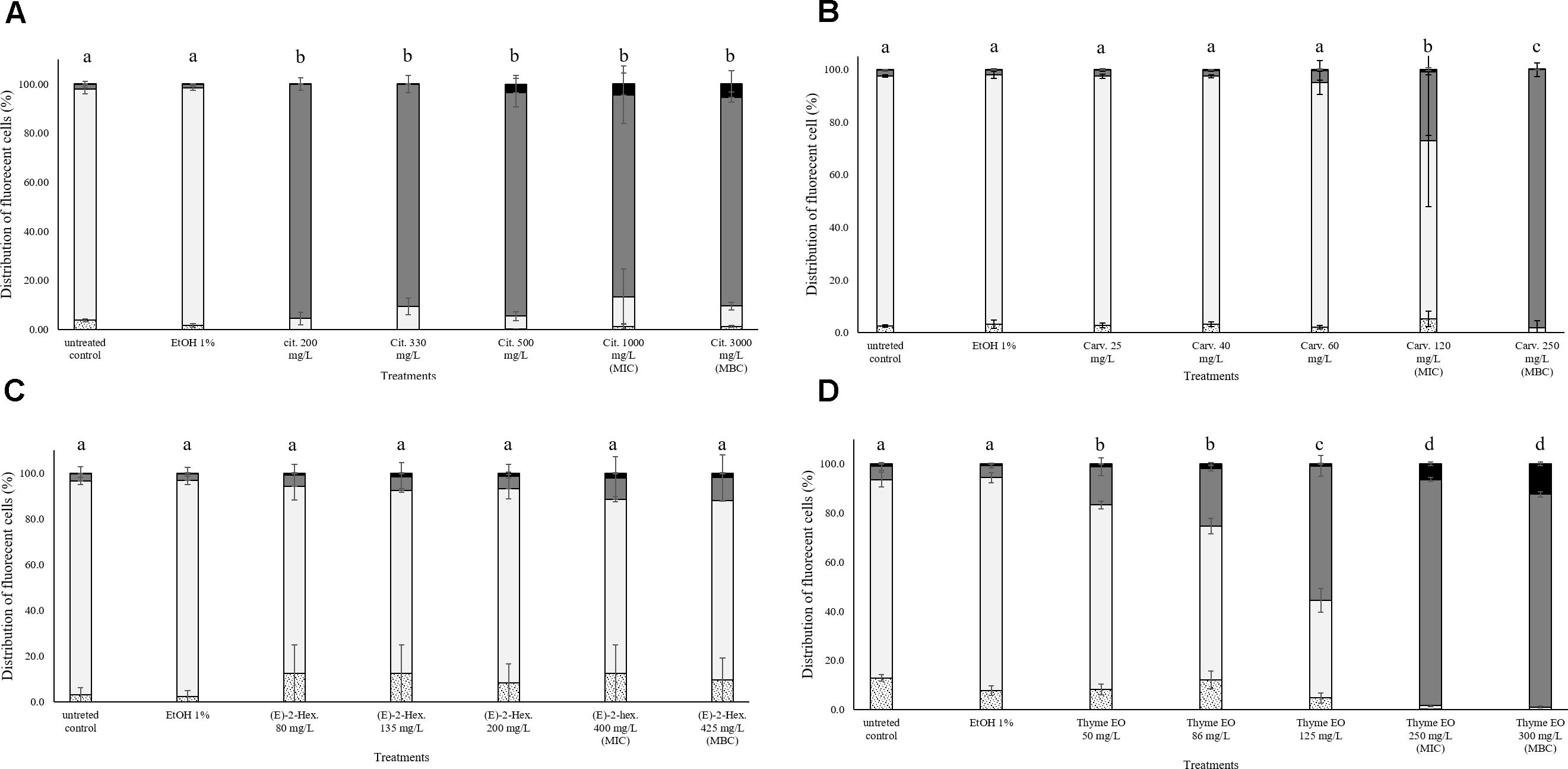
FIGURE 5. Esterase activity and membrane integrity of E. coli MG 1655 after 1 h exposure to natural antimicrobials: Citral (A), Carvacrol (B), (E)-(2)-hexenal (C), and thyme EO (D). White bars with dots represent cell fragments or unstained cells; White bars represent cells with intact cell membrane and esterase activity; Gray bars represent cell membrane permeabilization but still existing esterase activity; Black bars represent cells with cell membrane permeabilization but without esterase activity. Different letters indicate that data are significantly different (p < 0.05).
Treatment Effects on Cell Membrane Potential of Escherichia coli MG 1655
The measurement of the relative membrane potential using DiOC2(3) showed that the untreated E. coli cells had a red/green ratio of 1.69 before the exposure to citral (Figure 6A). The value was reduced below 1 independently of the concentration used, suggesting the capability of citral to depolarize the cell membrane of E. coli. A membrane depolarization was also observed for the MIC and MBC values of thyme EO (Figure 6D). No cell membrane depolarization was observed after the exposure to carvacrol and (E)-2-hexenal (Figures 6B,C) but (E)-2-hexenal induced a concentration dependent reduction of the cell membrane potential.
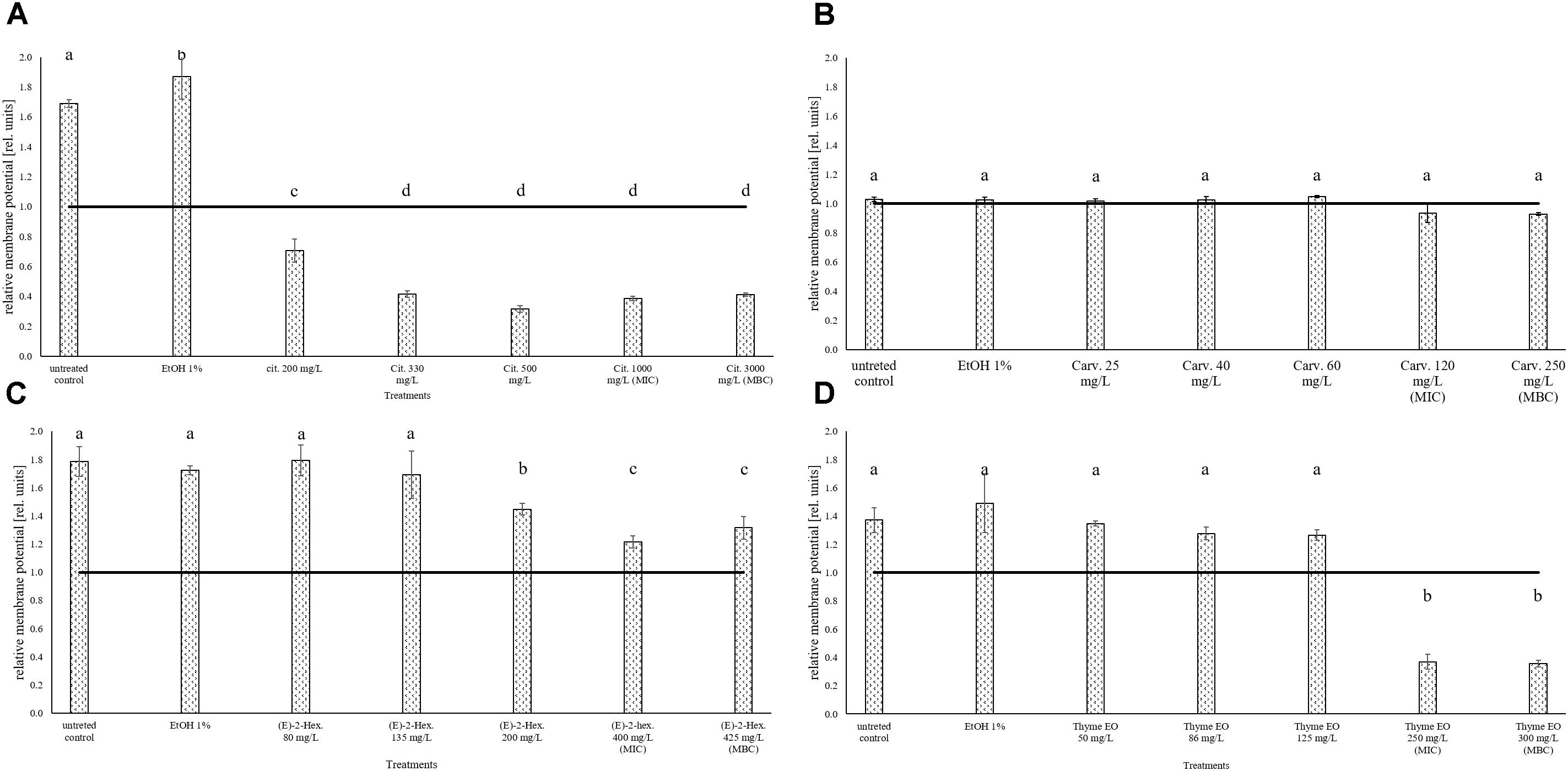
FIGURE 6. Relative membrane potential of E. coli MG 1655, expressed as red/green ratio of DiOC2(3)-fluorescence intensity, after 1 h exposure to natural antimicrobials: Citral (A), Carvacrol (B), (E)-(2)-hexenal (C), and thyme EO (D). The black line represent the threshold between polarized (ratio > 1) and depolarized (ratio < 1) cells. Different letters indicate that data are significantly different (p < 0.05).
Discussion
As described by many authors, EOs and their bioactive compounds are characterized by an antimicrobial activity both in vitro and in real food systems. Although their antimicrobial properties are well-documented, only limited information is available about their mechanisms of action on E. coli and L. monocytogenes (Lambert et al., 2001; Burt, 2004; Bakkali et al., 2008; Xu et al., 2008; Hyldgaard et al., 2012; Picone et al., 2013). Additionally, the literature on the responses of the whole cell populations of the selected species to EOs is still scarce (Xu et al., 2008). However, cell wall, membrane and energetic pathways are generally considered as the main microbial cell targets of EOs (Burt, 2004; Bakkali et al., 2008; Hyldgaard et al., 2012). In this framework multiparametric flow cytometric analyses were performed in order to assess the effects of citral, carvacrol, (E)-2-hexenal and thyme EO on the whole microbial populations of the two target microorganisms. Consequently, their effects on membrane integrity, esterase activity and cell membrane potential were investigated. Flow cytometric data of L. monocytogenes Scott A and E. coli MG 1655 populations after 1 h stress exposure revealed specific response patterns in relation to the natural antimicrobials and their concentrations. Concerning the membrane permeabilization, the percentage of permeabilized cells raised with the antimicrobial concentration applied, with the only exception for (E)-2-hexenal. The membrane integrity was analyzed using dye exclusion methods with thiazole orange (TO) and the divalent propidium iodide (PI) as dyes. In fact, TO is able to pass thought lipidic bilayers and to stain both DNA or RNA while PI, due to multiple charges, can only react with nucleic acids when membrane is disrupted or permeabilized (Kim et al., 2009; Díaz et al., 2010). Both target strains showed an increased cell membrane permeabilization with an increase of citral, carvacrol, and thyme EO concentrations. However, E. coli reacted sensitively to all the natural antimicrobials. In fact, they induced more severe membrane permeabilization and cell load reductions of E. coli in comparison to L. monocytogenes. The most effective antimicrobial against E. coli was citral. Actually, E. coli showed the highest cell load reduction and membrane permeabilization after the exposure to citral. A wide literature shows that the outer membrane of Gram-negative bacteria, which acts as a barrier against macromolecules and hydrophobic substances, increase their resistance to several antimicrobials including many EO (Nikaido and Vaara, 1985; Helander et al., 1997). However, also Somolinos et al. (2008) demonstrated that citral was more effective on E. coli J1 than on L. monocytogenes NCTC11994 under different experimental conditions, and especially at pH 7. These authors, using fluorescence microscopy and propidium iodide as dye, demonstrated that citral disrupted the outer cell envelope of E. coli, forming pores and permitting the cytoplasm entrance of molecules with a size of 660 Da. To destabilize the lipopolysaccharide layer of the outer membrane, the use of several chelating agents, such as EDTA, citric acid, and other substances, as well as high pressure homogenization have been proposed (Cutter and Siragusa, 1995; Helander et al., 1997; Vannini et al., 2004; Patrignani et al., 2010).
Different authors reported that citral and other low molecular mass ketons are sufficiently hydrophilic to pass throughout porin proteins to the deeper parts of Gram-negative bacteria without destabilization of the outer membrane (Helander et al., 1997; Lanciotti et al., 2003; Belletti et al., 2004; Belletti et al., 2008).
Carvacrol and thyme EO significantly reduced E. coli cell loads but only when used at MIC and MBC values. Only these concentrations induced a significant membrane permeabilization of E. coli cells. These data are in agreement with those of Xu et al. (2008) obtained by flow cytometry after exposure of E. coli AS1 90 to 200 mg/L of carvacrol and thymol, the major constituent of thyme EO. These authors showed cell membrane permeabilization processes associated with significant reductions of the cell loads (Xu et al., 2008). Also Gill and Holley (2006), using confocal laser scanning microscopy, showed a clear membrane disruption of E. coli O157:H7 after 10 min exposure to eugenol and carvacrol used at concentrations able to reduce the pathogen viability of about 8 log cycles.
A concentration dependent permeabilization process was also evidenced for L. monocytogenes Scott A after exposure to citral, carvacrol, and thyme EO. No literature is available on the effects of such antimicrobials on L. monocytogenes membrane permeabilization. However, Ultee et al. (1999) showed for Gram-positive Bacillus cereus that carvacrol caused an increased membrane permeability to cations such as H+ and K+. Also, no significant L. monocytogenes cell load reductions were highlighted for citral, independent of the concentration used. Somolinos et al. (2008) also showed a scarce sensitiveness of L. monocytogenes ATCC19114 serotype 4a to citral. Compared to citral, carvacrol and thyme EO were more effective on L. monocytogenes. However, significant cell load reductions were only observed using the MIC value of carvacrol and thyme EO concentrations. Friedman et al. (2002) described how carvacrol and thymol had the highest effect on the cell loads of L. monocytogenes RM2199 and RM2388 compared to other 23 EO constituents using a microplate assay. The effects of the natural antimicrobials on the esterase activity of L. monocytogenes and E. coli was measured because it is considered as reliable way to evaluate the cell damages induced after several antimicrobial treatments (Díaz et al., 2010; Surowsky et al., 2014; Fröhling and Schlüter, 2015; Hong et al., 2015; Combarros et al., 2016; Meng et al., 2016). In fact, carboxyfluorescein diacetate (cFDA), the fluorescent dye used to measure esterase activity, is a lipophilic non-fluorescent compound which is hydrolyzed in the cytoplasm to the fluorescent carboxyfluorescein (cF) by unspecific esterases. According to several literature data only cells with integer membrane and active intracellular enzymes remain fluorescent (Haugland, 1999; Hoefel et al., 2003; Fröhling and Schlüter, 2015; Reineke et al., 2015). However, the data obtained in our experimental conditions showed for both target strains that this enzymatic activity was not affected by the exposure to the antimicrobials used, independently on their concentrations. This data seems in disagreement with literature showing that membrane permeabilization is generally associated to the losses of esterase activity (Hayouni et al., 2008; Paparella et al., 2008; Xu et al., 2008). A decrease of esterase activity which was associated to membrane permeabilization was observed also in L. monocytogenes and E. coli exposed to EOs or their components (Xu et al., 2008). However the literature data evidenced losses of esterase activity only when lethal antimicrobial concentrations were used. For example, Paparella et al. (2008) showed by flow cytometric analyses that L. monocytogenes ATCC19114 serotype 4a had significant reductions of both esterase activity and cell viability after 1 h exposure to emulsified cinnamon, oregano, or thyme EOs (Paparella et al., 2008). However, these Authors tested the effects of emulsions having EO concentrations ranging between 0.02 and 0.5%. These concentrations are generally lethal concentrations for L. monocytogenes independently on the exposure conditions. However, they are not compatible with any usage in food systems due to the low sensory thresholds of the natural antimicrobials tested. In our experimental conditions, probably due to the use of concentrations significantly lower to those tested in the literature, the amount of cFDA hydrolyzed into cF after the exposure to natural antimicrobials remained constant, independently on the cell membrane permeabilization degree and the microorganism considered. In addition, the membrane potential of L. monocytogenes was not affected by the 1 h exposure to the natural antimicrobials considered. The membrane potential of cells is considered as fundamental in numerous processes of the live cell physiology and it is strongly related to bacterial viability (Novo et al., 1999). In fact, according to the literature, only living cells are able to maintain membrane potential (Díaz et al., 2010). In our experimental conditions, also at MIC values, the antimicrobials tested did not affect the membrane potential of L. monocytogenes compared to the control ones. However, also at MIC values of the tested antimicrobials, L. monocytogenes cells showed only a slight presence or even a complete absence of permeabilization of the cell membrane. By contrast, E. coli compared to L. monocytogenes, showed with a higher sensitiveness to almost all the antimicrobials considered, showed also cell membrane depolarization depending on the antimicrobial used and its concentration. A complete depolarization (red/green ratio below 1) of E. coli cells was observed for all citral concentrations tested and thyme EO MIC and MBC values. Also Kim and Kang (2017) observed that the cell membrane potential of E. coli O157:H7 was significantly reduced after exposure to citral and thymol combined with an ohmic heating treatment, both in model and real food systems.
In our experimental conditions, also (E)-2-hexenal exposure caused a reduction of relative membrane potential of the E. coli population. In contrast, no effect on the membrane potential of E. coli was evidenced after carvacrol treatments. Different studies confirmed that Gram-negative bacteria, due the outer membranes, were characterized by a higher resistance to carvacrol (Kokoska et al., 2002; Okoh et al., 2010). The reduction of membrane potential after antibacterial treatments is considered fundamental for pathogenic species, since viable but not culturable cells are still able to cause diseases (Fröhling and Schlüter, 2015).
In general, the data obtained indicated that sub-lethal treatments had minor impacts on L. monocytogenes compared to E. coli. In fact, all the antimicrobial tested induced only a slight cell membrane permeabilization (with the exception of (E)-2-hexenal) of L. monocytogenes, while only the exposure to carvacrol and thyme EO MIC values reduces the cultivability and significant cell load reductions were observed.
Escherichia coli was more sensitive to all the antimicrobials considered, not only in terms of cultivability but also in terms of membrane permeability and membrane potential. However, also for this strain, the antimicrobials used were unable to cause irreversible damages. In fact, the percentage of unstained cells of fragments remained constant and below the 3% independently on the strain, natural antimicrobial and the concentration used. As reported by Booyens and Thantsha (2014) during an antimicrobial treatment, the increase of the unstained population is related to a severe cell lysis or to a decreased staining accuracy due to formation of conglomerates. In addition, the increase of the unstained fraction is reported to be related with highly permeabilized or lysed cells unable to grow (Fröhling and Schlüter, 2015). The higher effect on membrane permeabilization of TO and PI staining compared to cF and PI dyes can be due to the fact that the effect of the last dyes was measured after a longer time span following the exposure to antimicrobials. It is presumable that the longer incubation after the stress exposure permitted the activation of microbial response mechanisms involving cell membrane repair. A wide literature shows that a precocious modification of cell membrane composition is pivotal for the microbial adaptation and survival in harsh conditions and sub-lethal stresses in order to maintain the proper fluidity, functionality, and permeability (Guerzoni et al., 2001; Gianotti et al., 2009; Mendoza, 2014; Serrazanetti et al., 2015; Siroli et al., 2015a). In fact, the immediate activation of the response mechanisms involving membrane modification was demonstrated both in fungi and bacteria to face even short time (lower that 100 min) physical-chemical stresses, including the exposure to natural antimicrobials (Guerzoni et al., 2001; Di Pasqua et al., 2006; Patrignani et al., 2008; Gianotti et al., 2009; Mendoza, 2014; Serrazanetti et al., 2015).
Conclusion
The flow cytometry approach allowed to understand the targets of sub-lethal concentrations of citral, carvacrol, (E)-2-hexenal and thyme EO to L. monocytogenes and E. coli cells. The data showed that the membrane permeabilization is a common action mechanism of the antimicrobials considered on both strains. In contrast, they showed that esterase activity was not affected independent of strain, antimicrobial and its concentration. The approach used revealed that some antimicrobials such as citral, carvacrol and thyme EO were more effective against the Gram-negative strain used. These results are particularly important since Gram-negative bacteria are more resistant to many antimicrobials. However, the multiparameter data obtained showed that the natural antimicrobials and the concentrations used also caused reversible damages on E. coli MG 1655, the most sensitive strain tested, since the percentage of cell fragments remained constant even when the MIC values were used and when the membrane was depolarized. These data suggests that the levels used of citral, carvacrol, and thyme EO can be used as preservatives to control the growth of L. monocytogenes and E. coli only in combinations with other hurdles. In fact, concentrations capable of having lethal effects are incompatible with the food sensory features due to their low sensory threshold. Consequently, the detailed knowledge of the action mechanisms of natural antimicrobials considered in relation to the others hurdles applied is mandatory for their implementation at industrial level as preservation strategies. However, the implementation processes should be optimized in relation to the food matrices and production processes.
Author Contributions
GB and FP contributed equally to the manuscript in the realization of lab trials. LS contributed to the statistical analysis. RL, OS, and AF contributed to the manuscript writing.
Conflict of Interest Statement
The authors declare that the research was conducted in the absence of any commercial or financial relationships that could be construed as a potential conflict of interest.
The handling Editor declared a past co-authorship with the authors OS and AF.
References
Aiemsaard, J., Aiumlamai, S., Aromdee, C., Taweechaisupapong, S., and Khunkitti, W. (2011). The effect of lemongrass oil and its major components on clinical isolate mastitis pathogens and their mechanisms of action on Staphylococcus aureus DMST 4745. Res. Vet. Sci. 91, e31–e37. doi: 10.1016/j.rvsc.2011.01.012
Amatiste, S., Sagrafoli, D., Giacinti, G., Rosa, G., Carfora, V., Marri, N., et al. (2014). Antimicrobial activity of essential oils against Staphylococcus aureus in fresh sheep cheese. Ital. J. Food Sci. 3, 1696. doi: 10.4081/ijfs.2014.1696
Ananta, E., Voigt, D., Zenker, M., Heinz, V., and Knorr, D. (2005). Cellular injuries upon exposure of Escherichia coli and Lactobacillus rhamnosus to high-intensity ultrasound. J. appl. Microbiol. 99, 271–278. doi: 10.1111/j.1365-2672.2005.02619.x
Bakkali, F., Averbeck, S., Averbeck, D., and Idaomar, M. (2008). Biological effects of essential oils–a review. Food Chem. Toxicol. 46, 446–475. doi: 10.1016/j.fct.2007.09.106
Barbosa, L. N., Probst, I. S., Murbach Teles Andrade, B. F., Bérgamo Alves, F. C., Albano, M., Mores Rall, V. L., et al. (2015). Essential oils from herbs against foodborne pathogens in chicken sausage. J. Oleo. Sci. 64, 117–124. doi: 10.5650/jos.ess14163
Belletti, N., Lanciotti, R., Patrignani, F., and Gardini, F. (2008). Antimicrobial efficacy of citron essential oil on spoilage and pathogenic microorganisms in fruit-based salads. J. Food Sci. 73, M331–M338. doi: 10.1111/j.1750-3841.2008.00866.x
Belletti, N., Ndagijimana, M., Sisto, C., Guerzoni, M. E., Lanciotti, R., and Gardini, F. (2004). Evaluation of the antimicrobial activity of citrus essences on Saccharomyces cerevisiae. J. Agr. Food Chem. 52, 6932–6938. doi: 10.1021/jf049444v
Ben Jemaa, M., Falleh, H., Neves, M. A., Isoda, H., Nakajima, M., and Ksouri, R. (2017). Quality preservation of deliberately contaminated milk using thyme free and nanoemulsified essential oils. Food Chem. 217, 726–734. doi: 10.1016/j.foodchem.2016.09.030
Berney, M., Hammes, F., Bosshard, F., Weilenmann, H.-U., and Egli, T. (2007). Assessment and interpretation of bacterial viability by using the LIVE/DEAD BacLight Kit in combination with flow cytometry. Appl. Environ. Microb. 73, 3283–3290. doi: 10.1128/AEM.02750-06
Booyens, J., and Thantsha, M. S. (2014). Fourier transform infra-red spectroscopy and flow cytometric assessment of the antibacterial mechanism of action of aqueous extract of garlic (Allium sativum) against selected probiotic Bifidobacterium strains. BMC Complement. Altern. Med. 14:289. doi: 10.1186/1472-6882-14-289
Boskovic, M., Zdravkovic, N., Ivanovic, J., Janjic, J., Djordjevic, J., Starcevic, M., et al. (2015). Antimicrobial activity of thyme (Thymus vulgaris) and oregano (Origanum vulgare) essential oils against some food-borne microorganisms. Proc. Food Sci. 5, 18–21. doi: 10.1016/j.profoo.2015.09.005
Burt, S. (2004). Essential oils: their antibacterial properties and potential applications in foods—a review. Int. J. Food Microbiol. 94, 223–253. doi: 10.1016/j.ijfoodmicro.2004.03.022
Caccioni, D., Gardini, F., Lanciotti, R., and Guerzoni, M. (1997). Antifungal activity of natural volatile compounds in relation to their vapour pressure. Sci. Aliment. 17, 21–34.
Chueca, B., Ramírez, N., Arvizu-Medrano, S. M., García-Gonzalo, D., and Pagán, R. (2016). Inactivation of spoiling microorganisms in apple juice by a combination of essential oils’ constituents and physical treatments. Food Sci. Technol. Int. 22, 389–398. doi: 10.1177/1082013215606832
Combarros, R., Collado, S., and Díaz, M. (2016). Toxicity of titanium dioxide nanoparticles on Pseudomonas putida. Water Res. 90, 378–386. doi: 10.1016/j.watres.2015.12.040
Cutter, C. N., and Siragusa, G. R. (1995). Population reductions of Gram-negative pathogens following treatments with nisin and chelators under various conditions. J. Food Protection 58, 977–983. doi: 10.4315/0362-028X-58.9.977
Da Silveira, M., and Abee, T. (2009). Activity of ethanol-stressed Oenococcus oeni cells: a flow cytometric approach. J. Appl. Microbiol. 106, 1690–1696. doi: 10.1111/j.1365-2672.2008.04136.x
Dhar, N., and McKinney, J. D. (2007). Microbial phenotypic heterogeneity and antibiotic tolerance. Curr. Opin. Microl. 10, 30–38. doi: 10.1016/j.mib.2006.12.007
Di Pasqua, R., Hoskins, N., Betts, G., and Mauriello, G. (2006). Changes in membrane fatty acids composition of microbial cells induced by addiction of thymol, carvacrol, limonene, cinnamaldehyde, and eugenol in the growing media. J. Agric. Food Chem. 54, 2745–2749. doi: 10.1021/jf052722l
Díaz, M., Herrero, M., García, L. A., and Quirós, C. (2010). Application of flow cytometry to industrial microbial bioprocesses. Biochem. Eng. J. 48, 385–407. doi: 10.1016/j.bej.2009.07.013
Ehsani, A., Hashemi, M., Hosseini Jazani, N., Aliakbarlu, J., Shokri, S., and Naghibi, S. S. (2016). Effect of Echinophora platyloba DC. essential oil and lycopene on the stability of pasteurized cream obtained from cow milk. Vet. Res. Forum 7, 139–148.
Fratianni, F., De Martino, L., Melone, A., De Feo, V., Coppola, R., and Nazzaro, F. (2010). Preservation of chicken breast meat treated with thyme and balm essential oils. J. Food Sci. 75, M528–M535. doi: 10.1111/j.1750-3841.2010.01791.x
Friedman, M., Henika, P. R., and Mandrell, R. E. (2002). Bactericidal activities of plant essential oils and some of their isolated constituents against Campylobacter jejuni, Escherichia coli, Listeria monocytogenes, and Salmonella enterica. J. Food Protoc. 65, 1545–1560. doi: 10.4315/0362-028X-65.10.1545
Fröhling, A., Klocke, S., Hausdorf, L., Klocke, M., and Schlüter, O. (2012). A method for viability testing of Pectobacterium carotovorum in postharvest processing by means of flow cytometry. Food Bioprocess Tech. 5, 2871–2879. doi: 10.1007/s11947-011-0749-6
Fröhling, A., and Schlüter, O. (2015). Flow cytometric evaluation of physico-chemical impact on Gram-positive and Gram-negative bacteria. Front. Microbiol. 6:939. doi: 10.3389/fmicb.2015.00939
Gianotti, A., Iucci, L., Guerzoni, M. E., and Lanciotti, R. (2009). Effect of acidic conditions on fatty acid composition and membrane fluidity of Escherichia coli strains isolated from Crescenza cheese. Ann. Microbiol. 59:603. doi: 10.1007/BF03175152
Gill, A. O., and Holley, R. A. (2006). Disruption of Escherichia coli, Listeria monocytogenes and Lactobacillus sakei cellular membranes by plant oil aromatics. Int. J. Food Microbiol. 108, 1–9. doi: 10.1016/j.ijfoodmicro.2005.10.009
Guerzoni, M. E., Lanciotti, R., and Cocconcelli, P. S. (2001). Alteration in cellular fatty acid composition as a response to salt, acid, oxidative and thermal stresses in Lactobacillus helveticus. Microbiology 147, 2255–2264. doi: 10.1099/00221287-147-8-2255
Haugland, R. P. (1994). Spectra of fluorescent dyes used in flow cytometry. Methods Cell Biol. 42, 641–663. doi: 10.1016/S0091-679X(08)61100-0
Hayouni, E. A., Bouix, M., Abedrabba, M., Leveau, J.-Y., and Hamdi, M. (2008). Mechanism of action of Melaleuca armillaris (Sol. Ex Gaertu) Sm. essential oil on six LAB strains as assessed by multiparametric flow cytometry and automated microtiter-based assay. Food Chem. 111, 707–718. doi: 10.1016/j.foodchem.2008.04.044
Helander, I. M., Alakomi, H.-L., Latva-Kala, K., Mattila-Sandholm, T., Pol, I., Smid, E. J., et al. (1998). Characterization of the action of selected essential oil components on Gram-negative bacteria. J. Agric. Food Chem. 46, 3590–3595. doi: 10.1021/jf980154m
Helander, I. M., Von Wright, A., and Mattila-Sandholm, T. (1997). Potential of lactic acid bacteria and novel antimicrobials against Gram-negative bacteria. Trends Food Sci. Technol. 8, 146–150. doi: 10.1016/S0924-2244(97)01030-3
Hewitt, C. J., and Nebe-Von-Caron, G. (2004). The application of multi-parameter flow cytometry to monitor individual microbial cell physiological state. Adv. Biochem. Eng. Biotechnol. 89, 197–223. doi: 10.1007/b93997
Hoefel, D., Grooby, W. L., Monis, P. T., Andrews, S., and Saint, C. P. (2003). A comparative study of carboxyfluorescein diacetate and carboxyfluorescein diacetate succinimidyl ester as indicators of bacterial activity. J. Microbiol. Methods 52, 379–388. doi: 10.1016/S0167-7012(02)00207-5
Hong, J., Guan, W., Jin, G., Zhao, H., Jiang, X., and Dai, J. (2015). Mechanism of tachyplesin I injury to bacterial membranes and intracellular enzymes, determined by laser confocal scanning microscopy and flow cytometry. Microbiol. Res. 170, 69–77. doi: 10.1016/j.micres.2014.08.012
Hyldgaard, M., Mygind, T., and Meyer, R. L. (2012). Essential oils in food preservation: mode of action, synergies, and interactions with food matrix components. Front. Microbiol. 3:12. doi: 10.3389/fmicb.2012.00012
Ivanovic, J., Misic, D., Zizovic, I., and Ristic, M. (2012). In vitro control of multiplication of some food-associated bacteria by thyme, rosemary and sage isolates. Food Control 25, 110–116. doi: 10.1016/j.foodcont.2011.10.019
Johnson, S., Nguyen, V., and Coder, D. (2013). Assessment of cell viability. Curr. Prot. Cytom. 64, 9.2.1–9.2.26. doi: 10.1002/0471142956.cy0902s64
Kim, H. T., Choi, H. J., and Kim, K. H. (2009). Flow cytometric analysis of Salmonella enterica serotype Typhimurium inactivated with supercritical carbon dioxide. J. Microbiol. Methods 78, 155–160. doi: 10.1016/j.mimet.2009.05.010
Kim, J., Marshall, M. R., and Wei, C.-I. (1995). Antibacterial activity of some essential oil components against five foodborne pathogens. J. Agric. Food Chem. 43, 2839–2845. doi: 10.1021/jf00059a013
Kim, S.-S., and Kang, D.-H. (2017). Combination treatment of ohmic heating with various essential oil components for inactivation of food-borne pathogens in buffered peptone water and salsa. Food Control 80, 29–36. doi: 10.1016/j.foodcont.2017.04.001
Kiskó, G., and Roller, S. (2005). Carvacrol and p-cymene inactivate Escherichia coli O157:H7 in apple juice. BMC Microbiol. 5:36. doi: 10.1186/1471-2180-5-36
Kokoska, L., Polesny, Z., Rada, V., Nepovim, A., and Vanek, T. (2002). Screening of some Siberian medicinal plants for antimicrobial activity. J. Ethnopharmacol. 82, 51–53. doi: 10.1016/S0378-8741(02)00143-5
Kubo, I., and Fujita, K. I. (2001). Naturally occurring anti-Salmonella agents. J. Agric. Food Chem. 49, 5750–5754. doi: 10.1021/jf010728e
Lambert, R. J., Skandamis, P. N., Coote, P. J., and Nychas, G. J. (2001). A study of the minimum inhibitory concentration and mode of action of oregano essential oil, thymol and carvacrol. J. Appl. Microbiol. 91, 453–462. doi: 10.1046/j.1365-2672.2001.01428.x
Lanciotti, R., Belletti, N., Patrignani, F., Gianotti, A., Gardini, F., and Guerzoni, M. E. (2003). Application of hexanal,(E)-2-hexenal, and hexyl acetate to improve the safety of fresh-sliced apples. J. Agric. Food Chem. 51, 2958–2963. doi: 10.1021/jf026143h
Lanciotti, R., Corbo, M. R., Gardini, F., Sinigaglia, M., and Guerzoni, M. E. (1999). Effect of hexanal on the shelf life of fresh apple slices. J. Agric. Food Chem. 47, 4769–4776. doi: 10.1021/jf990611e
Lanciotti, R., Gianotti, A., Patrignani, F., Belletti, N., Guerzoni, M. E., and Gardini, F. (2004). Use of natural aroma compounds to improve shelf-life and safety of minimally processed fruits. Trends Food Sci. Technol. 15, 201–208. doi: 10.1016/j.tifs.2003.10.004
Luscher, C., Balasa, A., Fröhling, A., Ananta, E., and Knorr, D. (2004). Effect of high-pressure-induced ice I-to-ice III phase transitions on inactivation of Listeria innocua in frozen suspension. Appl. Environ. Microbiol. 70, 4021–4029. doi: 10.1128/AEM.70.7.4021-4029.2004
Mathys, A., Chapman, B., Bull, M., Heinz, V., and Knorr, D. (2007). Flow cytometric assessment of Bacillus spore response to high pressure and heat. Innov. Food Sci. Emerg. 8, 519–527. doi: 10.1016/j.ifset.2007.06.010
Mendoza, D. D. (2014). Temperature sensing by membranes. Annu. Rev. Microbiol. 68, 101–116. doi: 10.1146/annurev-micro-091313-103612
Meng, J., Gong, Y., Qian, P., Yu, J.-Y., Zhang, X.-J., and Lu, R.-R. (2016). Combined effects of ultra-high hydrostatic pressure and mild heat on the inactivation of Bacillus subtilis. LWT Food Sci. Technol. 68, 59–66. doi: 10.1016/j.lwt.2015.12.010
Mols, M., Van Kranenburg, R., Van Melis, C. C. J., Moezelaar, R., and Abee, T. (2010). Analysis of acid-stressed Bacillus cereus reveals a major oxidative response and inactivation-associated radical formation. Environ. Microbiol. 12, 873–885. doi: 10.1111/j.1462-2920.2009.02132.x
Nakamura, S., and Hatanaka, A. (2002). Green-leaf-derived C6-aroma compounds with potent antibacterial action that act on both gram-negative and gram-positive bacteria. J. Agric. Food Chem. 50, 7639–7644. doi: 10.1021/jf025808c
Nevas, M., Korhonen, A.-R., Lindström, M., Turkki, P., and Korkeala, H. (2004). Antibacterial efficiency of Finnish spice essential oils against pathogenic and spoilage bacteria. J. Food Prot. 67, 199–202. doi: 10.4315/0362-028X-67.1.199
Newberne, P., Smith, R., Doull, J., and Feron, V. (2000). GRAS flavoring substances. Food Technol. 6, 66–84.
Nikaido, H., and Vaara, M. (1985). Molecular basis of bacterial outer membrane permeability. Microbiol. Rev. 49, 1–32.
Novo, D., Perlmutter, N. G., Hunt, R. H., and Shapiro, H. M. (1999). Accurate flow cytometric membrane potential measurement in bacteria using diethyloxacarbocyanine and a ratiometric technique. Cytometry Part A 35, 55–63. doi: 10.1002/(SICI)1097-0320(19990101)35:1<55::AID-CYTO8>3.0.CO;2-2
Okoh, O., Sadimenko, A., and Afolayan, A. (2010). Comparative evaluation of the antibacterial activities of the essential oils of Rosmarinus officinalis L. obtained by hydrodistillation and solvent free microwave extraction methods. Food Chem. 120, 308–312. doi: 10.1016/j.foodchem.2009.09.084
Pandey, A. K., Kumar, P., Singh, P., Tripathi, N. N., and Bajpai, V. K. (2017). Essential oils: sources of antimicrobials and food preservatives. Front. Microbiol. 7:2161. doi: 10.3389/fmicb.2016.02161
Paparella, A., Taccogna, L., Aguzzi, I., Chaves-Lopez, C., Serio, A., Marsilio, F., et al. (2008). Flow cytometric assessment of the antimicrobial activity of essential oils against Listeria monocytogenes. Food Control 19, 1174–1182. doi: 10.1016/j.foodcont.2008.01.002
Patel, S. (2015). Plant essential oils and allied volatile fractions as multifunctional additives in meat and fish-based food products: a review. Food Addit. Contam. Part A Chem. Anal. Control Expo. Risk Assess. 32, 1049–1064. doi: 10.1080/19440049.2015.1040081
Patrignani, F., Iucci, L., Belletti, N., Gardini, F., Guerzoni, M. E., and Lanciotti, R. (2008). Effects of sub-lethal concentrations of hexanal and 2-(E)-hexenal on membrane fatty acid composition and volatile compounds of Listeria monocytogenes, Staphylococcus aureus, Salmonella enteritidis and Escherichia coli. Int. J. Food Microbiol. 123, 1–8. doi: 10.1016/j.ijfoodmicro.2007.09.009
Patrignani, F., Siroli, L., Serrazanetti, D. I., Gardini, F., and Lanciotti, R. (2015). Innovative strategies based on the use of essential oils and their components to improve safety, shelf-life and quality of minimally processed fruits and vegetables. Trends Food Sci. Technol. 46, 311–319. doi: 10.1016/j.tifs.2015.03.009
Patrignani, F., Tabanelli, G., Siroli, L., Gardini, F., and Lanciotti, R. (2013). Combined effects of high pressure homogenization treatment and citral on microbiological quality of apricot juice. Int. J. Food Microbiol. 160, 273–281. doi: 10.1016/j.ijfoodmicro.2012.10.021
Patrignani, F., Vannini, L., Kamdem, S. L. S., Lanciotti, R., and Guerzoni, M. E. (2010). Potentialities of high-pressure homogenization to inactivate Zygosaccharomyces bailii in fruit juices. J. Food Sci. 75, M116–M120. doi: 10.1111/j.1750-3841.2009.01508.x
Picone, G., Laghi, L., Gardini, F., Lanciotti, R., Siroli, L., and Capozzi, F. (2013). Evaluation of the effect of carvacrol on the Escherichia coli 555 metabolome by using 1H-NMR spectroscopy. Food Chem. 141, 4367–4374. doi: 10.1016/j.foodchem.2013.07.004
R Core Development Team (2017). R: A Language and Environment for Statistical Computing. Vienna: R Foundation for Statistical Computing.
Radha Krishnan, K., Babuskin, S., Rakhavan, K. R., Tharavin, R., Azhagu Saravana Babu, P., Sivarajan, M., et al. (2015). Potential application of corn starch edible films with spice essential oils for the shelf life extension of red meat. J. Appl. Microbiol. 119, 1613–1623. doi: 10.1111/jam.12932
Reineke, K., Sevenich, R., Hertwig, C., Janßen, T., Fröhling, A., Knorr, D., et al. (2015). Comparative study on the high pressure inactivation behavior of the Shiga toxin-producing Escherichia coli O104:H4 and O157:H7 outbreak strains and a non-pathogenic surrogate. Food Microbiol. 46, 184–194. doi: 10.1016/j.fm.2014.07.017
Serrazanetti, D. I., Patrignani, F., Russo, A., Vannini, L., Siroli, L., Gardini, F., et al. (2015). Cell membrane fatty acid changes and desaturase expression of Saccharomyces bayanus exposed to high pressure homogenization in relation to the supplementation of exogenous unsaturated fatty acids. Front. Microbiol. 6:1105. doi: 10.3389/fmicb.2015.01105
Sienkiewicz, M., Łysakowska, M., Cieæwierz, J., Denys, P., and Kowalczyk, E. (2011). Antibacterial activity of thyme and lavender essential oils. Med. Chem. 7, 674–689. doi: 10.2174/157340611797928488
Silva-Angulo, A. B., Zanini, S. F., Rosenthal, A., Rodrigo, D., Klein, G., and Martínez, A. (2015). Comparative study of the effects of citral on the growth and injury of Listeria innocua and Listeria monocytogenes Cells. PLoS One 10:e0114026. doi: 10.1371/journal.pone.0114026
Siroli, L., Patrignani, F., Gardini, F., and Lanciotti, R. (2015a). Effects of sub-lethal concentrations of thyme and oregano essential oils, carvacrol, thymol, citral and trans-2-hexenal on membrane fatty acid composition and volatile molecule profile of Listeria monocytogenes, Escherichia coli and Salmonella enteritidis. Food Chem. 182, 185–192. doi: 10.1016/j.foodchem.2015.02.136
Siroli, L., Patrignani, F., Serrazanetti, D. I., Tabanelli, G., Montanari, C., Tappi, S., et al. (2015b). Potential of natural antimicrobials for the production of minimally processed fresh-cut apples. J. Food Proc. Technol. 6, 1–9. doi: 10.4172/2157-7110.1000415
Siroli, L., Patrignani, F., Serrazanetti, D. I., Tappi, S., Rocculi, P., Gardini, F., et al. (2015c). Natural antimicrobials to prolong the shelf-life of minimally processed lamb’s lettuce. Postharvest Biol. Tech. 103, 35–44. doi: 10.1016/j.postharvbio.2015.02.016
Siroli, L., Patrignani, F., Serrazanetti, D. I., Tabanelli, G., Montanari, C., Tappi, S., et al. (2014). Efficacy of natural antimicrobials to prolong the shelf-life of minimally processed apples packaged in modified atmosphere. Food Control 46, 403–411. doi: 10.1016/j.foodcont.2014.05.049
Somolinos, M., García, D., Condón, S., Mackey, B., and Pagán, R. (2010). Inactivation of Escherichia coli by citral. J. Appl. Microbiol. 108, 1928–1939. doi: 10.1111/j.1365-2672.2009.04597.x
Somolinos, M., García, D., Pagán, R., and Mackey, B. (2008). Relationship between sublethal injury and microbial inactivation by the combination of high hydrostatic pressure and citral or tert-butyl hydroquinone. Appl. Environ. Microbiol. 74, 7570–7577. doi: 10.1128/AEM.00936-08
Sunny-Roberts, E. O., and Knorr, D. (2008). Evaluation of the response of Lactobacillus rhamnosus VTT E-97800 to sucrose-induced osmotic stress. Food Microbiol. 25, 183–189. doi: 10.1016/j.fm.2007.05.003
Surowsky, B., Fröhling, A., Gottschalk, N., Schlüter, O., and Knorr, D. (2014). Impact of cold plasma on Citrobacter freundii in apple juice: inactivation kinetics and mechanisms. Int. J. Food Microbiol. 174, 63–71. doi: 10.1016/j.ijfoodmicro.2013.12.031
Swamy, M. K., Akhtar, M. S., and Sinniah, U. R. (2016). Antimicrobial properties of plant essential oils against human pathogens and their mode of action: an updated review. Evid. Based. Complement. Altern. 2016:3012462. doi: 10.1155/2016/3012462
Tamburini, S., Ballarini, A., Ferrentino, G., Moro, A., Foladori, P., Spilimbergo, S., et al. (2013). Comparison of quantitative PCR and flow cytometry as cellular viability methods to study bacterial membrane permeabilization following supercritical CO2 treatment. Microbiology 159, 1056–1066. doi: 10.1099/mic.0.063321-0
Tongnuanchan, P., and Benjakul, S. (2014). Essential oils: extraction, bioactivities, and their uses for food preservation. J. Food Sci. 79, R1231–R1249. doi: 10.1111/1750-3841.12492
Trombetta, D., Saija, A., Bisignano, G., Arena, S., Caruso, S., Mazzanti, G., et al. (2002). Study on the mechanisms of the antibacterial action of some plant α, β-unsaturated aldehydes. Lett. Appl. Microbiol. 35, 285–290. doi: 10.1046/j.1472-765X.2002.01190.x
Ultee, A., Kets, E., and Smid, E. (1999). Mechanisms of action of carvacrol on the food-borne pathogen Bacillus cereus. Appl. Environ. Microbiol. 65, 4606–4610.
Valero, M., and Francés, E. (2006). Synergistic bactericidal effect of carvacrol, cinnamaldehyde or thymol and refrigeration to inhibit Bacillus cereus in carrot broth. Food Microbiol. 23, 68–73. doi: 10.1016/j.fm.2005.01.016
Vannini, L., Lanciotti, R., Baldi, D., and Guerzoni, M. (2004). Interactions between high pressure homogenization and antimicrobial activity of lysozyme and lactoperoxidase. Int. J. Food Microbiol. 94, 123–135. doi: 10.1016/j.ijfoodmicro.2004.01.005
Xu, J., Zhou, F., Ji, B. P., Pei, R. S., and Xu, N. (2008). The antibacterial mechanism of carvacrol and thymol against Escherichia coli. Lett. Appl. Microbiol. 47, 174–179. doi: 10.1016/j.ijfoodmicro.2004.01.005
Zanini, S. F., Silva-Angulo, A. B., Rosenthal, A., Aliaga, D. R., and Martínez, A. (2014a). Influence of the treatment of Listeria monocytogenes and Salmonella enterica Serovar Typhimurium with citral on the efficacy of various antibiotics. Foodborne Pathog. Dis. 11, 265–271. doi: 10.1089/fpd.2013.1635
Zanini, S. F., Silva-Angulo, A. B., Rosenthal, A., Rodrigo, D., and Martínez, A. (2014b). Effect of citral and carvacrol on the susceptibility of Listeria monocytogenes and Listeria innocua to antibiotics. Lett. Appl. Microbiol. 58, 486–492. doi: 10.1111/lam.12218
Keywords: food safety, non-thermal treatment, pathogens, stress response, membrane permeabilization
Citation: Braschi G, Patrignani F, Siroli L, Lanciotti R, Schlueter O and Froehling A (2018) Flow Cytometric Assessment of the Morphological and Physiological Changes of Listeria monocytogenes and Escherichia coli in Response to Natural Antimicrobial Exposure. Front. Microbiol. 9:2783. doi: 10.3389/fmicb.2018.02783
Received: 17 July 2018; Accepted: 30 October 2018;
Published: 14 November 2018.
Edited by:
Henry Jaeger, Universität für Bodenkultur Wien, AustriaReviewed by:
Janet R. Donaldson, The University of Southern Mississippi, United StatesVeronica Lazar, University of Bucharest, Romania
Copyright © 2018 Braschi, Patrignani, Siroli, Lanciotti, Schlueter and Froehling. This is an open-access article distributed under the terms of the Creative Commons Attribution License (CC BY). The use, distribution or reproduction in other forums is permitted, provided the original author(s) and the copyright owner(s) are credited and that the original publication in this journal is cited, in accordance with accepted academic practice. No use, distribution or reproduction is permitted which does not comply with these terms.
*Correspondence: Rosalba Lanciotti, cm9zYWxiYS5sYW5jaW90dGlAdW5pYm8uaXQ=
†These authors have contributed equally to this work