- 1State Key Laboratory of Veterinary Etiological Biology, Key Laboratory of Veterinary Parasitology of Gansu Province, Key Laboratory of Veterinary Public Health of Agriculture Ministry, Lanzhou Veterinary Research Institute, Chinese Academy of Agricultural Sciences, Lanzhou, China
- 2Jiangsu Co-innovation Center for Prevention and Control of Important Animal Infectious Disease, Yangzhou, China
The Qinghai-Tibet Plateau is a highly endemic area of alveolar echinococcosis where a series of intermediate hosts, especially voles and pikas, are infected with Echinococcus multilocularis. The metacestodes of E. multilocularis are fluid-filled, asexually proliferating cysts, and they are mainly found in the host's liver in the form of tumor-like growths. In this study, we investigated the genetic variations of E. multilocularis in four mitochondrial (mt) genes, namely, NADH dehydrogenase subunit 5 (nad5), adenosine triphosphate subunit 6 (atp6), cytochrome c oxidase subunit 1 (cox1), and NADH dehydrogenase subunit 1 (nad1). The complete nad5, atp6, cox1, and nad1 genes were amplified separately from each hydatid cyst isolate using polymerase chain reaction (PCR) and then sequenced. Phylogenetic trees were then generated based on the combined mt genes using MrBayes 3.1.2 and PAUP version 4.0b10. The results showed that thirty of 102 voles and two of 49 pikas were infected with E. multilocularis. The genetic variation distances among all E. multilocularis samples were 0.1–0.4%, 0.2–0.4%, 0.1–0.6%, and 0.1–0.4% for nad5, atp6, nad1, and cox1, respectively. Compared to previous studies of the genetic diversity of E. multilocularis based on the cox1 gene, the genetic distances within the same group were 1.3–1.7% (Mongolia strain), 0.6–0.8% (North American strain), 0.3–0.6% (European strain), and 0.1–0.4% (Asian strain). Based on concatenated sequences of the nad5, atp6, cox1, and nad1 genes all haplotypes were divided into two clusters. In conclusion, the genetic diversity of E. multilocularis based on mt genes on a small local area is at low level but between different regions with long distance and different ecological environment each other, the genetic diversity is at relatively high level; genetic variation is higher in the nad1 gene than that in the other three mt genes. The results on a local scale provide basic information for further study of the molecular epidemiology, genetic differences and control of E. multilocularis in Qinghai Province, China.
Introduction
Echinococcus multilocularis is a small cestode that cause the parasitic zoonosis alveolar echinococcosis (AE), which was one of the 17 neglected tropical diseases (NTDs) prioritized by the World Health Organization (WHO) in 2012 (Agudelo Higuita et al., 2016). AE was discovered in the Nineteenth century; it has a considerable socioeconomic impact and until now has been sporadically found in humans (Nakao et al., 2007; Spahn et al., 2016). E. multilocularis is mainly distributed in holarctic regions, including North America (also found in southwestern Ontario), Europe, and Asia (Massolo et al., 2014; Oksanen et al., 2016; Deplazes et al., 2017; Trotz-Williams et al., 2017). Asia, especially China, has many highly endemic areas, such as Qinghai, Gansu, and Sichuan. E. multilocularis infects two kinds of hosts: the typical intermediate host (IH), a wide spectrum of mammalian species including small herbivorous, rodents (predominantly) and pikas, and the typical definitive hosts (DH), which are canids and mammalian species, including foxes, wolves, dogs, and cats (Vuitton et al., 2003; Deplazes et al., 2011; Hegglin and Deplazes, 2013; Conraths and Deplazes, 2015; Raoul et al., 2015; Knapp et al., 2016; Eckert and Thompson, 2017). Humans play the role of an aberrant IH for E. multilocularis and can be infected when ingesting eggs released into vegetables and food by adult worms. Then, the parasite larvae travel to internal organs, mainly the liver (Torgerson et al., 2010; Conraths et al., 2017).
Mitochondrial DNA (mtDNA) has an important role in the taxonomy of Echinococcus species. According to mtDNAs, E. granulosus species include 10 genotypes (G1-G10) and genetic variation in mtDNAs has also been found within E. multilocularis (Bowles et al., 1992; Nakao et al., 2007; McManus, 2013). From early studies, E. multilocularis has been divided into two geographical genotypes, M1 (Europe) and M2 (China, Alaska and North America), based on four nucleotide substitutions in the nad1 gene (Bowles and McManus, 1993; Okamoto et al., 1995). Nakao and his co-workers found 17 regional haplotypes based on three mt protein-coding genes: cytochrome c oxidase subunit 1 (cox1), cytochrome b (cytb), and NADH dehydrogenase subunit 2 (nad2) (Nakao et al., 2009). All of those studies show a relatively low mt nucleotide diversity within E. multilocularis. To date, more genes have been used for investigating genetic variation in E. multilocularis. Although the nuclear genome and the microsatellite targets are also used for molecular markers (Knapp et al., 2008; Umhang et al., 2014; Laurimaa et al., 2015; Karamon et al., 2017), mt genes are more commonly used in the analysis of genetic variation in E. multilocularis, because they have a great copy number and evolve at a high rate (Brown et al., 1979; Ciesielski et al., 2016).
In our study, we chose mtDNAs as molecular markers to assess the diversity of E. multilocularis in plateau voles (Neodon/ Microtus fuscus) and plateau pikas (Ochotona curzoniae). In addition to the common mt cox1 and nad1 genes, NADH dehydrogenase subunit 5 (nad5) and adenosine triphosphate subunit 6 (atp6) were also included for genetic variation analysis, which provide basic information on molecular epidemiology, genetic variations or differences in E. multilocularis in China.
Materials and Methods
Ethics Statement
All animals were handled in strict accordance with good animal practice according to the Animal Ethics Procedures and Guidelines of the People's Republic of China, and the study was approved by the Animal Ethics Committee of Lanzhou Veterinary Research Institute, Chinese Academy of Agricultural Sciences (No. LVRIAEC2012-007). In addition, all mice were handled in strict accordance with the animal protection laws of the People's Republic of China (A Draft of an Animal Protection Law in China released on September 18, 2009).
Biosecurity Statement
The biohazards, biological select agents, toxins, restricted materials or reagents involved in the research have been carried out according to the “Measures for the prevention and control of environmental pollution of hazardous chemicals” issued by the state environmental protection administration (No. 27_2005-03-30) and the “Regulations on the management of medical waste” promulgated by the state council of the People's Republic of China (2003-06-16).
Sample Collection
The specimens of E. multilocularis were collected from Jiuzhi County, Golog Autonomous Prefecture, Qinghai Province (33.32° N, 100.53° E) at an average elevation of 4,100 m above sea level. All samples were collected at random in the field amid a “Rodent control program” that was carried out by the local Center for Animal Disease Control and Prevention. Cystic lesions were collected from two intermediate host species: plateau voles and pikas. They were isolated and placed in 70% (v/v) ethanol before being sent to the laboratory at Lanzhou Veterinary Research Institute (LRVI). The lesions were used for DNA extraction, PCR amplification and sequencing to confirm the parasite species and analyze genetic variations. Additionally, one E. multilocularis metacestode sample from the Xinjiang Uygur Autonomous Region was preserved by our laboratory.
DNA Extraction
The cystic lesions were rinsed repeatedly using phosphate buffered saline (PBS) to remove the ethanol, and then, the lesions were disrupted using TissueLysers before DNA extraction. With the use of the TIANamp Genomic DNA Kit (TianGen Biotech, Beijing, China), the total DNA was extracted according to the manufacturer's protocols.
PCR Amplification and Sequencing of MT Genes
Four pairs of primers were designed for nad5, atp6, nad1, and cox1 genes (Table 1). A PCR reaction mix included 25 μl Premix Ex Taq version 2.0 (Takara Biomedicals, Shiga, Japan), 1 μl DNA template, 1 μl primer F (25 μM), 1 μl primer R (25 μM), and 22 μl ddH2O. The PCR was carried out using a standard 3-step cycle: 94°C, 4 min; 35 cycles of 94°C, 30 s; 50–57°C, 30 s' 72°C, 1–2 min; 72°C, 10 min. Each PCR product purified for sequencing was gel-cut and DNA was recovered through a column (AxyPrep DNA Gel Extraction Kit by AxyGen). The purified products were sent to GENEWIZ, Inc. (Beijing, China) to be sequenced using Sanger dideoxy chain termination in an ABI3730 DNA Analyzer.
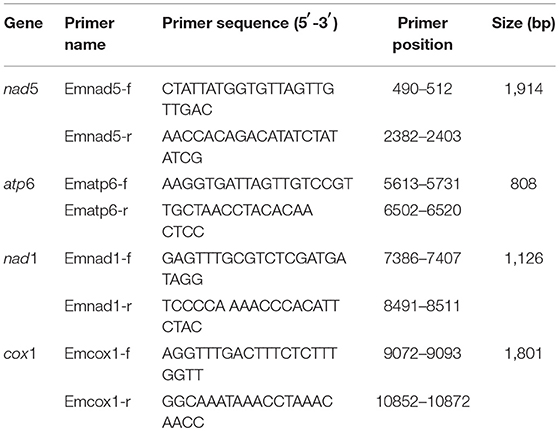
Table 1. Primers for PCR amplification for mt genes of E. multilocularis with positions based on a reference sequence (AB018440 in GenBank).
Sequence Alignment and Analysis
The open reading frames of all raw nucleotide sequences of the nad5, atp6, nad1, and cox1genes of each isolate were assembled, edited and concatenated into a total sequence using the software package SeqMan (DNAStar, Inc., Madison, WI, www.dnastar.com/t-megalign.aspx) and Clustal W2 (online software, http://www.ebi.ac.uk/Tools/msa/clustalw2/, serviced by EBI, the European Bioformatics Institute). The total sequences (one of the sequences having 100% similarity with the others was chosen) were aligned using BioEdit v7.2.3 and ClustalX 1.83 (Thompson et al., 1997; Hall, 1999). The Megalign software (DNAStar, Inc., Madison, WI) was used to calculate the genetic divergence between different haplotypes. In phylogenetic reconstruction, E. shiquicus (GenBank accession no. AB159136) was used as an out group, because it was considered the sister species of E. multilocularis (Nakao et al., 2007). Bayesian analysis was performed to combine four datasets by using MrBayes 3.1.2 with a general time reversible (GTR) model of DNA substitution and a gamma distribution rate variation across sites (Ronquist and Huelsenbeck, 2003). In this model, a Metropolis-coupled Markov chain Monte Carlo analysis was run for 1 million generations, and trees were sampled every 100 generations. The first one-fourth of 10,000 trees were treated as burn-in. The command was executed until the average standard deviation of the split frequencies was lower than 0.01 (Nakao et al., 2009; Zhao et al., 2013).
Phylogenetic reconstruction was completed using PAUP (phylogenetic analysis using parsimony) version 4.0b10 (Cummings, 2014) by the neighbor-joining (NJ) and maximum parsimony (MP) methods. The NJ tree was performed from HKY85 distances with inverse-squared weighting (power = 2). The MP tree was executed using a heuristic search with TBR branch swapping options and 1,000 random sequence additions. The clade of trees was estimated with 1,000 replicates of bootstrap (BT) analysis. The descriptive tree statistics, tree length (TL), consistency index (CI), retention index (RI), rescaled consistency index (RC), and homoplasy index (HI) for Maximum Parsimonious Tree were engendered (Zhao et al., 2013).
Then, we used MEGA6 to construct a phylogenetic tree based on the cox1 gene by maximum likelihood approaches (ML) (GTR+G+I model; 1,000 bootstraps) with the sequences (GenBank accession no. AB477010-AB477012, AB461412- AB461420, AB688125-AB688135, AB777915-AB777921, AB813186-AB813188, KT001423, KT001424, and KY205677-KY205691) downloaded from NCBI (Tamura et al., 2013). The network of E. multilocularis based on mt concatenated sequences was calculated using TCS 1.21 software (Clement et al., 2000).
Results
E. multilocularis cysts
A total of 102 voles (N. fuscus) and 49 pikas (O. curzoniae) were captured in Jiuzhi County. Of these animals, 32 (30 voles, infection rate: 29.41%; 2 pikas, infection rate: 4.08%) had cystic lesions in their viscera. From these 32 individuals, 39 cysts were isolated. Twenty-seven cysts were found only in the liver, and the others were found not only in the liver but also in the lungs, the intestinal wall and the muscles (Table 2).
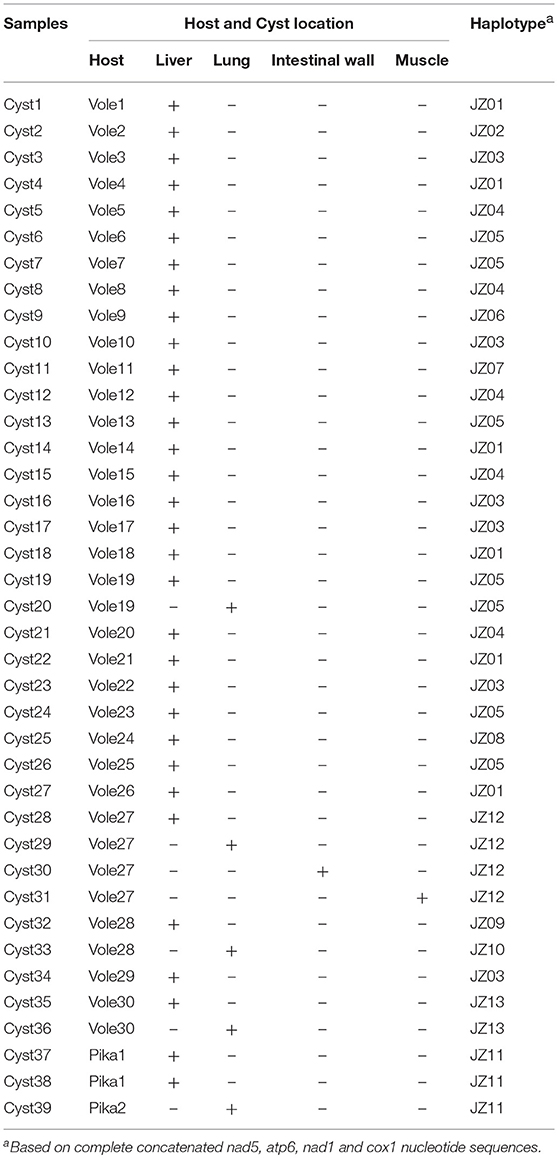
Table 2. Haplotypes of E. multilocularis isolates, their species, and their organ location in the 32 samples.
Characterization of Haplotypes and Phylogenetic Trees Analysis
The combined sequences of nad5 (1,575 bp), atp6 (516 bp), nad1 (894 bp) and cox1 (1,608 bp) genes were distributed into 15 haplotypes including one from Xinjiang Uygur Autonomous Region and one from Yushu Tibetan Autonomous Prefecture (Table 2), which were designated as JZ01 to JZ13 (haplotypes from Jiuzhi), XJ (haplotype from Xinjiang), and YS (haplotype from Yushu), respectively. When coming from a single gene, the number of haplotypes decreasedto 7 in nad5, 2 in atp6, 4 in nad1, and 11 in cox1 (Table 3). Phylogenetic analyse showed that the genetic diversity of individual gene changes was 0.1–0.4% (nad5), 0.2–0.4% (atp6), 0.1–0.6% (nad1), and 0.1–0.4% (cox1). Within the four genes, cox1 had the most mutation sites (with 9 sites), followed by 7 sites in nad5, 2 sites in atp6 and 2 sites in nad1 (Table 4). When we compared the sequences with a reference sequence for partitioned nad5, atp6, nad1, and cox1 genes, there were only two haplotypes in the four genes. The genetic distance for JZ01 wa 99.7, 99.8, 99.9, and 99.8%, and for JZ03, it was 99.7, 99.6, 100, and 99.9%. The sequence analysis with the reference sequence in this study further predicted that all of the samples or specimens isolated from plateau voles and pikas belonged to E. multilocularis.
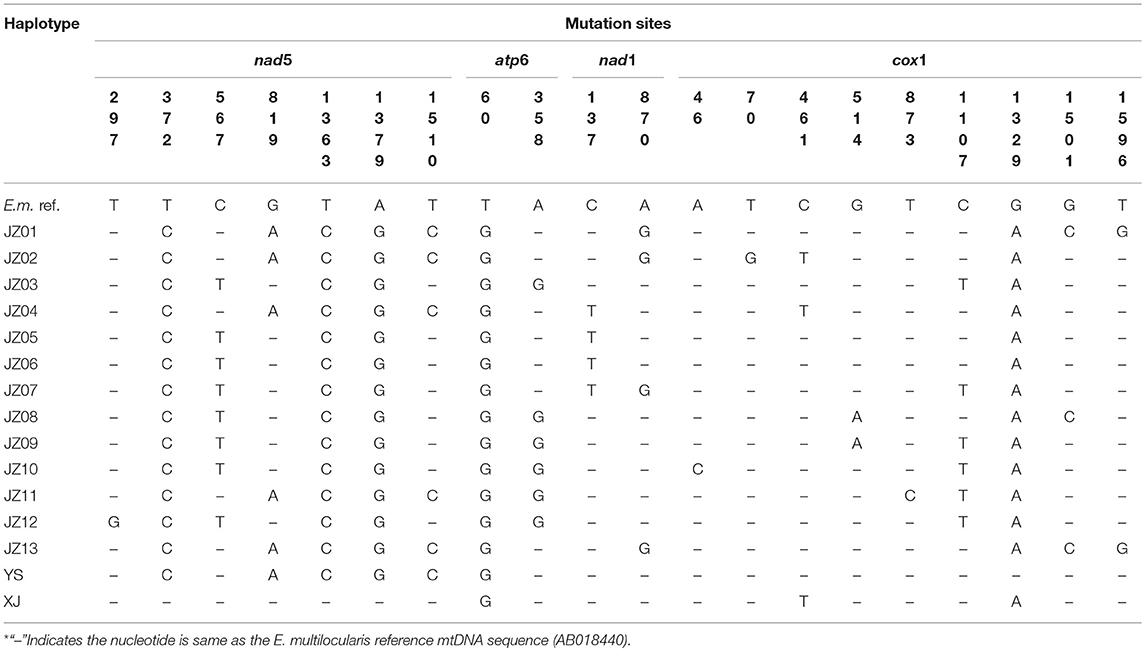
Table 4. Mutation sites in the complete nad5, atp6, nad1 and cox1 gene sequences among different E. multilocularis (E.m.) haplotypes.
There were a number of changes in amino acids, with synonymous substitutions in 7 sites, from 460S to 460N (nad5, haplotype XJ); from 120R to 120G (atp6, haplotypes JZ03, JZ08-JZ12); from 46A to 46V (nad1, haplotypes JZ04-JZ07); from 16K to 16Q (cox1, haplotype JZ10); from 24L to 24V (cox1, haplotype JZ02); from 46E to 46Q (cox1, haplotype JZ06); from 153A to 153V (cox1, haplotypes JZ02, JZ04, XJ); from 172V to 172I (cox1, haplotypes JZ09, JZ10); from 443I to 443M (cox1, haplotypes YS); from 501A to 501P (cox1, haplotypes JZ01, JZ13) and from 532F to 532L (cox1, haplotypes JZ01, JZ13). There was a heterogeneous substitution in 504 (cox1), from W to R (haplotypes JZ01, JZ02, JZ04, JZ11, JZ13, YS).
The phylogram based on the maximum parsimony method precisely revealed that the 14 haplotypes were divided into two large haplogroups: the JZ03, JZ05-JZ10, and JZ12 haplotypes had a close relationship with each other (classified as C1 haplogroup) and the rest of the haplotypes gathered together (classified as C2 haplogroup) (Figure 1). The haplotype XJ from Xinjiang Province is at the base of the phylogenetic tree. In this phylogenetic tree, the bootstrap value of three nodes was almost the same and was low. The maximum genetic diversity between the XJ haplotype and C1 haplogroup reached 0.2%, and the divergence between haplotype XJ and C2 was 0.2%. When compared haplogroup C1 with C2, the rate of pairwise divergence was the same. Haplotype cladograms of E. multilocularis were carried by Bayesian and NJ methods using the combined nucleotide sequences of the cox1, nad1, nad5, and atp6 genes. The two methods both showed a similar result with two clades (Figure 2). Furthermore, the network of those haplotypes also divided into the two same groups (Figure 3). Compared to previous studies of the genetic diversity of E. multilocularis based on the cox1 gene, we found that the sequences could be divided into 4 groups; Mongolia strain, North American strain, European strain and Asian strain. The highest genetic divergence is 1.3–1.7% (samples collected from Inner Mongolia, Mongolia and Russia), followed by 0.6–0.8% (samples collected from the USA: Alaska and Indiana), 0.3–0.6% (samples collected from Austria, Estonia, France, Poland, Slovakia and Russia) and 0.1–0.4% (samples collected from China: Sichuan, Kazakhstan, Japan: Hokkaido). The genetic distance within the same group is 0.1–0.3% (Mongolia strain), 0.4% (North American strain), 0.1–0.3% (European strain), and 0.1–0.4% (Asian strain) (Nakao et al., 2009; Ito et al., 2010; Konyaev et al., 2012; Laurimaa et al., 2015; Karamon et al., 2017). Similarly, according to the phylogenetic tree based on the cox1 gene constructed by MEGA6, all haplotypes clustered together with the haplotypes downloaded from NCBI (samples collected from China: Sichuan, Kazakhstan, Japan: Hokkaido), belonging to the Asian clade (not shown).
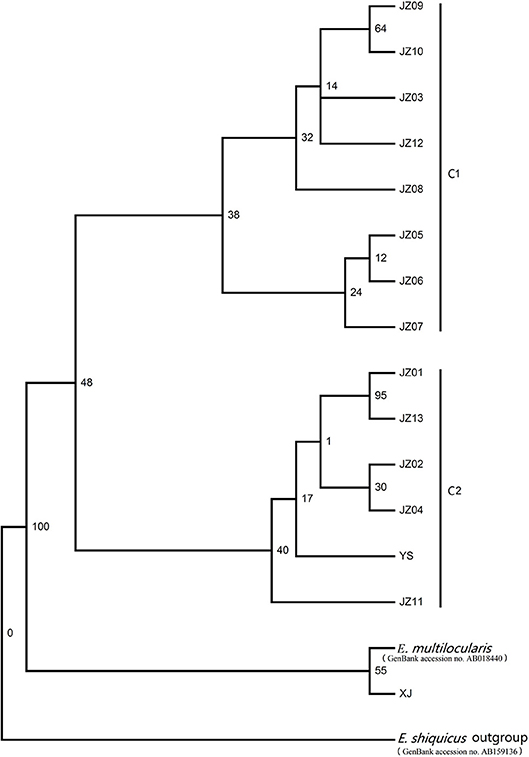
Figure 1. Maximum parsimony (MP) tree constructed with the PAUP version 4.0b10 based on the concatenated nucleotide sequences of the nad5, atp6, nad1, and cox1 genes.
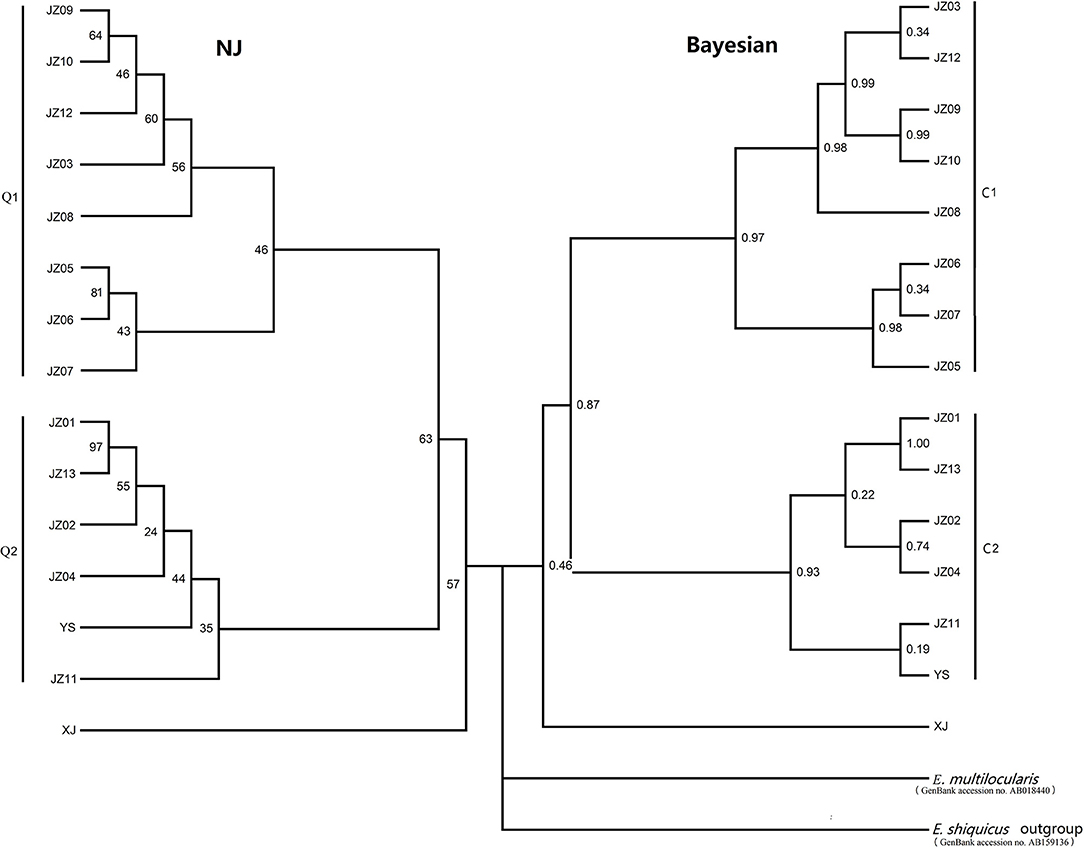
Figure 2. Haplotype cladograms of E. multilocularis inferred by the neighbor-joining (NJ) and Bayesian methods using the concatenated nucleotide sequences of the nad5, atp6, nad1, and cox1 genes.
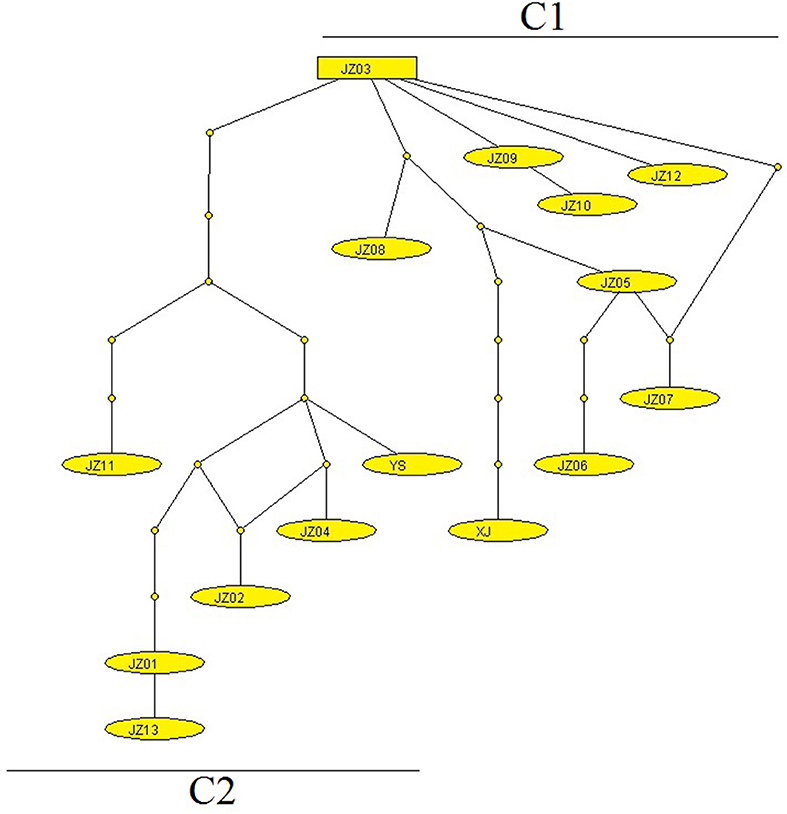
Figure 3. The network of E. multilocularis generated using mt concatenated genes collected from voles and pikas. Each link between haplotypes indicates one mutational difference and the unlabeled nodes point out that inferred steps not found in the samples.
Discussion
The genus Echinococcus has a unique reproductive cycle, including a sexual reproduction in the adult stage and asexual proliferation in the larval phase. Because of this reproductive model, Echinococcus has genetic monomorphism in local populations (Lymbery and Thompson, 1996; Haag et al., 1999; Nakao et al., 2007). In early studies, E. multilocularis was recognized as a subspecies of E. granulosus, but Rausch and Nelson thought that E. multilocularis had unusual morphological and biological peculiarities and should be a separate genus. Then, phylogenetic analyses based on the mt genes also indicated that E. multilocularis was different compared with E. granulosus (Lukashenko and Zorikhina, 1961; Rausch and Nelson, 1963; Tappe et al., 2010). Now the genus Echinococcus includes at least 10 species: E. granulosus, E. equinus, E. ortleppi, E. Canadensis, E. intermedius, E. felidis, E. multilocularis, E. vogeli, E. oligarthra, and E. shiquicus (Thompson, 2008, 2017; Nakao et al., 2013; Thompson and Jenkins, 2014).
Until now, a majority of published data on the gene diversity and phylogenetic analysis of the genus Echinococcus have been based on short mt genes, especially the cox1 and nad1 genes. From early studies, nine genotypes were classified in E. granulosus s. l. based on the diversity of the mt genes cox1 and nad1, and these two genes were applied in E. multilocularis genetic variation analysis (Bowles et al., 1995; Tappe et al., 2010; Romig et al., 2017). In our study, we used the 4 full-length sequences of mt genes to analyze the diversity of E. multilocularis on the local scale. Herein, the sequence divergence within E. multilocularis wase 0.1–0.4% for nad5, 0.2–0.4% for atp6, 0.1–0.6% for nad1, and 0.1–0.4% for cox1. The result showed that the nad1 gene had more diversity than those of the other genes, which is in contrast with previous studies showing that atp6 had a comparatively more diversity within the Echinococcus spp. and E. multilocularis (Yang et al., 2005; Nakao et al., 2007). However, our results were in keeping with a previous study based on the cox1 gene. The variation in the nad5 gene showed a consistent results with the published data of the other parasites (Zhao et al., 2014; Lou et al., 2015). Regarding amino acid, cox1 exhibited the highest frequency of substitution (0.7476%, 4 substitution sites/535 sites), followed by apt6 (0.5814%, 1/172), nad5 (0.3817%, 2/524), and nad1 (0.3367%, 1/297). According to the results, even though the analysis of the cox1 gene was more meaningful from an evolutionary standpoint, the phylogenetic tree based on only one gene was not accurate. The phylogenetic trees analysis revealed that all 15 haplotypes divided into 3 groups. The 14 samples isolated from Qinghai-Tibet plateau divided into two clades, and the haplotype isolated from Xinjiang province was in a separate group. In C1, the JZ09 haplotype was isolated from the lungs of voles, the JZ12 haplotype was isolated from the intestines of voles, and the remaining haplotypes were found in the liver of voles. In C2, the JZ13 haplotype was isolated from the lungs in voles, the JZ11 haplotype was isolated from the lungs of pikas, and the others were found in the liver of voles. The phylogram indicted that the different intermediate hosts and parasitic sites had no effect on the genetic diversity of E. multilocularis on a local scale. From the results of the genetic divergence within haplotypes based on the cox1 gene, we observed that the European strain has the lowest genetic diversity, and the Asian strain and Mongolia strain have the highest diversity. This also implied that the relationship maybe not important between geographical distances and genetic distances.
In conclusion, our study, based on the mitochondrial complete coding genes nad5, atp6, nad1, and cox1, shows a relatively low genetic variation among the samples from voles and pikas on a local scale. The genetic variation is higher in nad1 than that in the other three mt genes, and the concatenated mt sequences of the cox1, nad1, nad5, and atp6 genes are useful in phylogenetic reconstruction within E. multilocularis isolates.
Author Contributions
JL, LL, HY, and WJ conceived and designed the experiments. JL, LL, and YF performed the experiments and the data analyses. JL prepared the figures and wrote the manuscript, WJ and HY provided improving paragraphs and BF and XZ provided very constructive suggestions for revisions.
Funding
This study was supported by the National Key Basic Research Program (973 Program) of China (2015CB150300), the National Key Research and Development Program of China (2017YFD0501300), and the National Natural Science Foundation of China (31402191, 31401148).
Conflict of Interest Statement
The authors declare that the research was conducted in the absence of any commercial or financial relationships that could be construed as a potential conflict of interest.
The handling editor declared a past co-authorship with one of the authors DB.
Acknowledgments
We would like to thank David Blair from the School of Marine and Tropical Biology, James Cook University for his constructive suggestions for the manuscript, Guang-Wei Hu from the Qinghai Provincial Animal Disease Prevention and Control Center, and veterinarians from the Jiuzhi Animal Disease Prevention and Control Center, Qinghai Province for their support in the collection of cyst samples.
References
Agudelo Higuita, N. I., Brunetti, E., and Mccloskey, C. (2016). Cystic echinococcosis. J. Clin. Microbiol. 54, 518–523. doi: 10.1128/JCM.02420-15
Bowles, J., Blair, D., and McManus, D. (1995). A molecular phylogeny of the genus Echinococcus. Parasitology 110, 317–328. doi: 10.1017/S0031182000080902
Bowles, J., Blair, D., and McManus, D. P. (1992). Genetic variants within the genus Echinococcus identified by mitochondrial DNA sequencing. Mol. Biochem. Parasitol. 54, 165–173. doi: 10.1016/0166-6851(92)90109-W
Bowles, J., and McManus, D. P. (1993). NADH dehydrogenase 1 gene sequences compared for species and strains of the genus Echinococcus. Int. J. Parasitol. 23, 969–972. doi: 10.1016/0020-7519(93)90065-7
Brown, W. M., George, M., and Wilson, A. C. (1979). Rapid evolution of animal mitochondrial DNA. Proc. Natl. Acad. Sci. U.S.A. 76, 1967–1971. doi: 10.1073/pnas.76.4.1967
Ciesielski, G. L., Oliveira, M. T., and Kaguni, L. S. (2016). Animal mitochondrial DNA replication. Enzymes 39, 255–292. doi: 10.1016/bs.enz.2016.03.006
Clement, M., Posada, D., and Crandall, K. A. (2000). TCS: a computer program to estimate gene genealogies. Mol. Ecol. 9, 1657–1659. doi: 10.1046/j.1365-294x.2000.01020.x
Conraths, F. J., and Deplazes, P. (2015). Echinococcus multilocularis: epidemiology, surveillance and state-of-the-art diagnostics from a veterinary public health perspective. Vet. Parasitol. 213, 149–161. doi: 10.1016/j.vetpar.2015.07.027
Conraths, F. J., Probst, C., Possenti, A., Boufana, B., Saulle, R., Torre, G. L., et al. (2017). Potential risk factors associated with human alveolar echinococcosis: systematic review and meta-analysis. PLoS Negl. Trop. Dis. 11:e0005801. doi: 10.1371/journal.pntd.0005801
Cummings, M. P. (2014). PAUP* [Phylogenetic Analysis Using Parsimony (and Other Methods)]. 4th Edn. Sunderland: Sinauer Associates.
Deplazes, P., Rinaldi, L., Alvarez Rojas, C. A., Torgerson, P. R., Harandi, M. F., Romig, T., et al. (2017). Global distribution of alveolar and cystic echinococcosis. Adv. Parasitol. 95, 315–493. doi: 10.1016/bs.apar.2016.11.001
Deplazes, P., van Knapen, F., Schweiger, A., and Overgaauw, P. A. (2011). Role of pet dogs and cats in the transmission of helminthic zoonoses in Europe, with a focus on echinococcosis and toxocarosis. Vet. Parasitol. 182, 41–53. doi: 10.1016/j.vetpar.2011.07.014
Eckert, J., and Thompson, R. C. (2017). Historical aspects of echinococcosis. Adv. Parasitol. 95, 1–64. doi: 10.1016/bs.apar.2016.07.003
Haag, K. L., Araujo, A. M., Gottstein, B., Siles-Lucas, M., Thompson, R., and Zaha, A. (1999). Breeding systems in Echinococcus granulosus (Cestoda; Taeniidae): selfing or outcrossing? Parasitology 118, 63–71.
Hall, T. A. (1999). BioEdit: A User-Friendly Biological Sequence Alignment Editor and Analysis Program for Windows 95/98/NT, Nucleic Acids Symposium Series. London: Information Retrieval Ltd., c1979-c(2000), 95–98.
Hegglin, D., and Deplazes, P. (2013). Control of Echinococcus multilocularis: strategies, feasibility and cost-benefit analyses. Int. J. Parasitol. 43, 327–337. doi: 10.1016/j.ijpara.2012.11.013
Ito, A., Agvaandaram, G., Bat-Ochir, O. E., Chuluunbaatar, B., Gonchigsenghe, N., Yanagida, T., et al. (2010). Histopathological, serological, and molecular confirmation of indigenous alveolar echinococcosis cases in Mongolia. Am. J. Trop. Med. Hygiene 82, 266–269. doi: 10.4269/ajtmh.2010.09-0520
Karamon, J., Stojecki, K., Samorek-Pierog, M., Bilska-Zajac, E., Rozycki, M., Chmurzynska, E., et al. (2017). Genetic diversity of Echinococcus multilocularis in red foxes in Poland: the first report of a haplotype of probable Asian origin. Folia Parasitol. 64:007. doi: 10.14411/fp.2017.007
Knapp, J., Combes, B., Umhang, G., Aknouche, S., and Millon, L. (2016). Could the domestic cat play a significant role in the transmission of Echinococcus multilocularis? A study based on qPCR analysis of cat feces in a rural area in France. Parasite 23:42. doi: 10.1051/parasite/2016052
Knapp, J., Guislain, M. H., Bart, J. M., Raoul, F., Gottstein, B., Giraudoux, P., and Piarroux, R. (2008). Genetic diversity of Echinococcus multilocularis on a local scale. Infect. Genet. Evol. J. Mol. Epidemiol. Evol. Genetics Infect. Dis. 8, 367–373. doi: 10.1016/j.meegid.2008.02.010
Konyaev, S. V., Yanagida, T., Ingovatova, G. M., Shoikhet, Y. N., Nakao, M., Sako, Y., et al. (2012). Molecular identification of human echinococcosis in the Altai region of Russia. Parasitol. Int. 61, 711–714. doi: 10.1016/j.parint.2012.05.009
Laurimaa, L., Suld, K., Moks, E., Valdmann, H., Umhang, G., Knapp, J., et al. (2015). First report of the zoonotic tapeworm Echinococcus multilocularis in raccoon dogs in Estonia, and comparisons with other countries in Europe. Vet. Parasitol. 212, 200–205. doi: 10.1016/j.vetpar.2015.06.004
Lou, Y., Zhang, Y., Qiu, J.-H., Gao, J.-F., Wang, W.-T., Xiao, J.-Y., et al. (2015). Sequence variability in four mitochondrial genes among pinworm Aspicularis tetraptera isolates from laboratory mice in four provinces, China. Mitochondrial DNA 26, 431–434. doi: 10.3109/19401736.2013.855736
Lukashenko, N., and Zorikhina, V. (1961). Epidemiology of alveolar echinococcosis in central areas of the Barabinsk forest-steppe in the Novosibirsk region. Meditsinskaya Parazitologiya i Parazitarnye Bolezni 30, 159–168.
Lymbery, A., and Thompson, R. (1996). Species of Echinococcus: pattern and process. Parasitol. Today 12, 486–491. doi: 10.1016/S0169-4758(96)10071-5
Massolo, A., Liccioli, S., Budke, C., and Klein, C. (2014). Echinococcus multilocularis in North America: the great unknown. Parasite 21:73. doi: 10.1051/parasite/2014069
McManus, D. P. (2013). Current status of the genetics and molecular taxonomy of Echinococcus species. Parasitology 140, 1617–1623. doi: 10.1017/S0031182013000802
Nakao, M., Lavikainen, A., Yanagida, T., and Ito, A. (2013). Phylogenetic systematics of the genus Echinococcus (Cestoda: Taeniidae). Int. J. Parasitol. 43, 1017–1029. doi: 10.1016/j.ijpara.2013.06.002
Nakao, M., McManus, D., Schantz, P. M., Craig, P. S., and Ito, A. (2007). A molecular phylogeny of the genus Echinococcus inferred from complete mitochondrial genomes. Parasitology 134, 713–722. doi: 10.1017/S0031182006001934
Nakao, M., Xiao, N., Okamoto, M., Yanagida, T., Sako, Y., and Ito, A. (2009). Geographic pattern of genetic variation in the fox tapeworm Echinococcus multilocularis. Parasitol. Int. 58, 384–389. doi: 10.1016/j.parint.2009.07.010
Okamoto, M., Bessho, Y., Kamiya, M., Kurosawa, T., and Horii, T. (1995). Phylogenetic relationships within Taenia taeniaeformis variants and other taeniid cestodes inferred from the nucleotide sequence of the cytochrome c oxidase subunit I gene. Parasitol. Res. 81, 451–458. doi: 10.1007/BF00931785
Oksanen, A., Sileslucas, M., Karamon, J., Possenti, A., Conraths, F. J., Romig, T., et al. (2016). The geographical distribution and prevalence of Echinococcus multilocularis in animals in the European Union and adjacent countries: a systematic review and meta-analysis. Parasit. Vectors 9:519. doi: 10.1186/s13071-016-1746-4
Raoul, F., Hegglin, D., and Giraudoux, P. (2015). Trophic ecology, behaviour and host population dynamics in Echinococcus multilocularis transmission. Vet. Parasitol. 213, 162–171. doi: 10.1016/j.vetpar.2015.07.034
Rausch, R. L., and Nelson, G. S. (1963). A review of the genus Echinococcus Rudolphi, (1801). Ann. Trop. Med. Parasitol. 57, 127–135. doi: 10.1080/00034983.1963.11686168
Romig, T., Deplazes, P., Jenkins, D., Giraudoux, P., Massolo, A., Craig, P. S., et al. (2017). Ecology and life cycle patterns of Echinococcus species. Adv. Parasitol. 95, 213–314. doi: 10.1016/bs.apar.2016.11.002
Ronquist, F. H., and Huelsenbeck, J. P. (2003). MrBayes3: Bayesian phylogenetic inference under mixed models. Bioinformatics 19, 1572–1574.
Spahn, S., Helmchen, B., and Zingg, U. (2016). Alveolar echinococcosis of the right adrenal gland: a case report and review of the literature. J. Med. Case Rep. 10:325. doi: 10.1186/s13256-016-1115-0
Tamura, K., Stecher, G., Peterson, D., Filipski, A., and Kumar, S. (2013). MEGA6: Molecular evolutionary genetics analysis version 6.0. Mol. Biol. Evol. 30, 2725–2729. doi: 10.1093/molbev/mst197
Tappe, D., Kern, P., Frosch, M., and Kern, P. (2010). A hundred years of controversy about the taxonomic status of Echinococcus species. Acta Trop. 115, 167–174. doi: 10.1016/j.actatropica.2010.03.001
Thompson, J. D., Gibson, T. J., Plewniak, F., Jeanmougin, F., and Higgins, D. G. (1997). The CLUSTAL_X windows interface: flexible strategies for multiple sequence alignment aided by quality analysis tools. Nucleic Acids Res. 25, 4876–4882. doi: 10.1093/nar/25.24.4876
Thompson, R. C. (2008). The taxonomy, phylogeny and transmission of Echinococcus. Exp. Parasitol. 119, 439–446. doi: 10.1016/j.exppara.2008.04.016
Thompson, R. C. (2017). Biology and systematics of echinococcus. Adv. Parasitol. 95, 65–109. doi: 10.1016/bs.apar.2016.07.001
Thompson, R. C., and Jenkins, D. J. (2014). Echinococcus as a model system: biology and epidemiology. Int. J. Parasitol. 44, 865–877. doi: 10.1016/j.ijpara.2014.07.005
Torgerson, P. R., Keller, K., Magnotta, M., and Ragland, N. (2010). The global burden of alveolar echinococcosis. PLoS Negl. Trop. Dis. 4:e722. doi: 10.1371/journal.pntd.0000722
Trotz-Williams, L. A., Mercer, N. J., Walters, J. M., Wallace, D., Gottstein, B., Osterman-Lind, E., et al. (2017). public health follow-up of suspected exposure to Echinococcus multilocularis in Southwestern Ontario. Zoonoses Public Health. 64, 460–467. doi: 10.1111/zph.12326
Umhang, G., Knapp, J., Hormaz, V., Raoul, F., and Boue, F. (2014). Using the genetics of Echinococcus multilocularis to trace the history of expansion from an endemic area. Infect. Genet. Evol. 22, 142–149. doi: 10.1016/j.meegid.2014.01.018
Vuitton, D. A., Zhou, H., Bresson-Hadni, S., Wang, Q., Piarroux, M., Raoul, F., et al. (2003). Epidemiology of alveolar echinococcosis with particular reference to China and Europe. Parasitology 127(Suppl), S87–S107. doi: 10.1017/S0031182003004153
Yang, Y. R., Rosenzvit, M., Zhang, L., Zhang, J., and McManus, D. (2005). Molecular study of Echinococcus in west-central China. Parasitology 131, 547–555. doi: 10.1017/S0031182005007973
Zhao, C. L., Cui, B. K., and Dai, Y. C. (2013). New species and phylogeny of Perenniporia based on morphological and molecular characters. Fungal Divers. 58, 47–60. doi: 10.1007/s13225-012-0177-6
Zhao, Z. H., Bian, Q. Q., Ren, W. X., Cheng, W. Y., Jia, Y. Q., Fang, Y. Q., et al. (2014). Genetic variability of Baylisascaris schroederi from the Qinling subspecies of the giant panda in China revealed by sequences of three mitochondrial genes. Mitochondrial DNA 25, 212–217. doi: 10.3109/19401736.2013.792074
Keywords: E. multilocularis, mitochondrial gene, genetic variation, haplotypes, plateau vole, plateau pika
Citation: Li J, Li L, Fan Y, Fu B, Zhu X, Yan H and Jia W (2018) Genetic Diversity in Echinococcus multilocularis From the Plateau Vole and Plateau Pika in Jiuzhi County, Qinghai Province, China. Front. Microbiol. 9:2632. doi: 10.3389/fmicb.2018.02632
Received: 30 November 2017; Accepted: 16 October 2018;
Published: 05 November 2018.
Edited by:
Wei Hu, Fudan University, ChinaReviewed by:
Ning Xiao, Chinese Center For Disease Control and Prevention, ChinaAdriano Casulli, Istituto Superiore di Sanità (ISS), Italy
Da-Bing Lu, Soochow University, China
Copyright © 2018 Li, Li, Fan, Fu, Zhu, Yan and Jia. This is an open-access article distributed under the terms of the Creative Commons Attribution License (CC BY). The use, distribution or reproduction in other forums is permitted, provided the original author(s) and the copyright owner(s) are credited and that the original publication in this journal is cited, in accordance with accepted academic practice. No use, distribution or reproduction is permitted which does not comply with these terms.
*Correspondence: Hong-bin Yan, eWFuaG9uZ2JpbkBjYWFzLmNu
Wan-zhong Jia, amlhd2FuemhvbmdAY2Fhcy5jbg==
†These authors have contributed equally to this work