- 1Microbial Ecology Group, Department of Biology, Lund University, Lund, Sweden
- 2Department of Microbial Ecology, Netherlands Institute of Ecology (NIOO-KNAW), Wageningen, Netherlands
- 3Department of Soil Quality, Wageningen University and Research Centre, Wageningen, Netherlands
In many parts of the world, agricultural ecosystems are increasingly exposed to severe drought, and rainfall events due to climate changes. This coincides with a higher vulnerability of crops to soil-borne diseases, which is mostly ascribed to decreased resistance to pathogen attacks. However, loss of the natural capacity of soil microbes to suppress soil-borne plant pathogens may also contribute to increased disease outbreaks. In this perspectives paper, we will discuss the effect of extreme weather events on pathogen-antagonist interactions during drought and rainfall events and upon recovery. We will focus on diseases caused by root-infecting fungi and oomycetes. In addition, we will explore factors that affect restoration of the balance between pathogens and other soil microbes. Finally, we will indicate potential future avenues to improve the resistance and/or recovery of natural biocontrol during, and after water stresses. As such, our perspective paper will highlight a knowledge gap that needs to be bridged to adapt agricultural ecosystems to changing climate scenarios.
Introduction
Climate change is expected to increase the exposure of agricultural ecosystems to extreme drought and rainfall events (IPCC, 2012; Fischer and Knutti, 2016), which can result in severe decreases in crop yields (Challinor et al., 2014; Obidiegwu et al., 2015; Challinor et al., 2016; Eurostats, 2016). It will, therefore, be a great challenge to maintain sufficient food production for the growing human population. Next to direct decreases in crop yields due to unfavorable growth conditions, additional problems may be caused by a reduced resistance of agricultural crops to soil-borne plant pathogen attacks after drought and rainfall events (Ramegowda and Senthil-Kumar, 2015; Dikilitas et al., 2016). The coincidence of extreme weather events and higher vulnerability of crops to pathogen attacks can be due to a decrease in the plant immune response (for a detailed review on this topic see Ramegowda and Senthil-Kumar, 2015) and/or an altered pathogen pressure.
Root-infecting fungi and oomycetes are two major groups of pathogens causing problems in agricultural crops at a broad range of moisture levels (Duncan and Kennedy, 1989; Dixon and Tilston, 2010; Thompson et al., 2013). For example, high water content increases the ability of motile zoospores of plant pathogenic oomycetes to reach roots (Malajczuk and Theodorou, 1979; Judelson and Blanco, 2005). In contrast, drought increases the amount of drought resistant microorganisms. Fungi are often more resistant to drought than bacteria (Barnard et al., 2013; Meisner et al., 2013; de Vries et al., 2018) and many fungal pathogens, such as species belonging to Fusarium or Verticillium genera, are often involved in increased pathogen pressure during drought, (Dikilitas et al., 2016). Hence, the types of pathogens that thrive under drought and wet conditions will differ.
A largely ignored potential mechanism of increased pathogen pressure after an extreme drought or rainfall event is the reduction of the natural capacity of soil to suppress pathogens. The legacy of an environmental stress, including water stress, can decrease the biological suppression of crop pathogens and therewith increase the vulnerability of crops for pathogen attacks (Ho and Ko, 1985; Lootsma and Scholte, 1997; van Agtmaal et al., 2015). Most soils show a certain level of suppression against pathogenic fungi and oomycetes, often referred to as general soil suppression (Garbeva et al., 2011). Competitive interactions in soil microbial communities are thought to be the major causal factor of general soil suppression (Garbeva et al., 2011). In addition, some soils show so-called specific suppression against one pathogenic species (Raaijmakers and Mazzola, 2016). The plant’s response to increased pathogen abundance depends on the microbial community colonizing the roots and the plant’s ability to tolerate water stress. The colonization of plant roots by soil microorganisms is influenced by the amount and composition of rhizodeposits (Philippot et al., 2013). Several root-colonizing microorganisms are known to improve the plants response to pathogens (Berendsen et al., 2012). In addition, several rhizosphere microorganisms can increase drought tolerance in plants (Ngumbi and Kloepper, 2016). However, there is limited information about interactions of plant-growth promoting microbes with pathogens during drought stress and upon recovery. In this perspectives paper, we propose that improvements to the maintenance and recovery of suppression of plant pathogens during and after drought and rainfall may prevent severe losses due to soil-borne pathogens. In addition, we will suggest areas for future research that improve our understanding of how extreme drought and rainfall events will affect interactions between pathogen suppressive microorganisms and crop pathogens.
Antagonistic Interactions Between Pathogens and Heterotrophic Microbes
The suppression of pathogen infection on roots is caused by interactions with other soil microorganisms (van Os et al., 1999; Duran et al., 2017) and often occurs via the production of inhibitory secondary metabolites (Garbeva et al., 2011). Chemical compounds, such as antibiotics, that are produced during antagonistic interactions between competing heterotrophic microbes may also affect other biota in soils, including pathogens (Garbeva et al., 2011; Raaijmakers and Mazzola, 2012; Schulz-Bohm et al., 2017). Most secondary chemicals exuded by microorganisms can diffuse through the water-filled area of soil pores and, therefore, only interact with microbes that live in the water phase (Tyc et al., 2017). However, one group of secondary compounds, volatiles, is of special interest, as volatiles can diffuse through both the water-filled and air-filled soil pores thereby widening the spatial range of inhibition of pathogens (Schmidt et al., 2015; Tyc et al., 2017). As such, the impact of fluctuations of soil water content on the role of volatiles in pathogen suppression is of special interest (Peñuelas et al., 2014). Differences in moisture content will affect the composition of chemical compounds produced by soil microbes (Bastos and Magan, 2007; Hiltpold and Turlings, 2008). Waterlogged conditions after heavy rainfall will expel gasses from soil and reduce the movement of gasses in soil (Moyano et al., 2013). Volatiles will be especially involved in competitive interactions in the air-filled area of the pores in unsaturated soils (Figure 1A), whereas water soluble secondary metabolites will be the main compounds in antagonistic interactions during waterlogged conditions (Figure 1C). Therefore, the chemical and physical characteristics of secondary metabolites that are effective in suppressing interactions will be determined by soil moisture conditions (Figure 1).
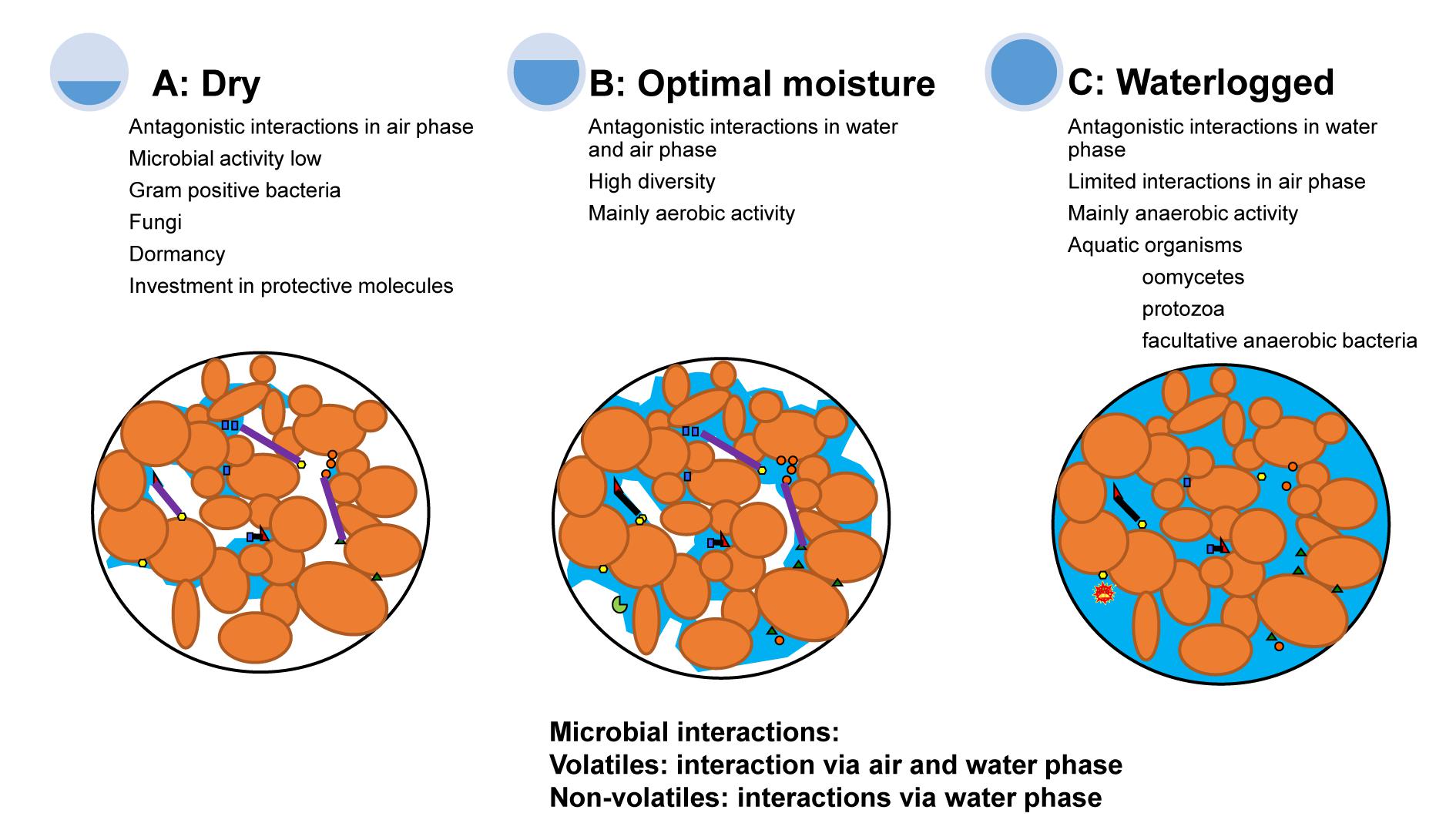
FIGURE 1. The types of antagonistic interactions between pathogens and other soil microorganisms are influenced by water availability. Under dry conditions (A), there is a big air phase and the interactions between microorganisms may occur mainly via volatile organic compounds in the air phase. However, the microbial activity of both resident and plant pathogens is low when moisture is limiting. Microorganisms that survive drought may invest in protective molecules or formation of dormancy structures. During optimal moisture conditions (B), most microorganisms grow aerobically and interact via secondary chemicals, enzymes and volatiles in both the air and water phase. During waterlogged conditions (C), interactions between microorganisms occur in the water phase of soils. Microorganisms that survive waterlogged conditions include organisms that can cope with anoxic conditions. Small triangles, squares, and circles reflect different soil microorganisms. The purple lines reflect microbial interactions that occur in the air phase and the black lines reflect interactions that occur in the water phase of soil. The blue areas indicate the water phase and the white areas the air phase. Figure adapted from Moyano et al. (2013).
There is increasing evidence that volatiles produced by soil microorganisms play an important role in the natural suppression of pathogens. For example, growth of three common plant pathogens was inhibited by volatiles emitted from 50 agricultural soils (van Agtmaal et al., 2018). Production of pathogen-inhibiting volatiles by bacteria has received particular attention in research (Garbeva et al., 2011; Schmidt et al., 2015; Schulz-Bohm et al., 2017). Research on suppression of fungal pathogens by bacterial volatiles has also indicated that composition of bacterial communities is an important determinant of the spectrum of volatiles produced. For example, loss of rare soil bacteria decreased volatiles that suppressed in vitro growth of the plant pathogen Fusarium oxysporum (Hol et al., 2015). In addition, the legacy of anaerobic disinfection, which is the anaerobic treatment of soil in between crop cover, reduced volatiles and pathogen suppression three months after recovery, via effects on the bacterial community composition (van Agtmaal et al., 2015). Differences in soil moisture can affect the composition of the microbial community (Barnard et al., 2015; Hartmann et al., 2017; Meisner et al., 2018) and, consequently, also the spectrum of inhibiting compounds. The question remains if these changes coincide with altered pathogen suppression.
Balance Between Soil Pathogens and Heterotrophic Soil Microbes
Pathogen suppression will be influenced by the response of both heterotrophic microorganisms and pathogens to drought and waterlogged conditions as well as their ability to recover (Figure 2A). First, both pathogens and heterotrophic microorganisms have to survive the extreme conditions. This will likely depend upon the niche space for water availability as microbial species, including pathogens, differ in their potential to maintain activity along a range of matric potentials (Whiting et al., 2001; Lennon et al., 2012). A wider niche space for a microorganism results in a higher chance of surviving the extreme conditions and, consequently, a higher chance to be present in the recovery phase. Soil microorganisms often experience anoxic conditions when exposed to waterlogged conditions. This can have an impact on the composition of microbes in the recovery phase (van Agtmaal et al., 2015). Microbes may also survive unfavorable conditions by going into dormancy (Manzoni et al., 2014; Shoemaker and Lennon, 2018), by producing protective molecules, such as osmolytes (Warren, 2014) or extracellular peptides (Or et al., 2007). Another strategy to survive is to have a thicker cell wall such as the thick peptidoglycan layer of Gram positive bacteria (Potts, 1994; Schimel et al., 2007).
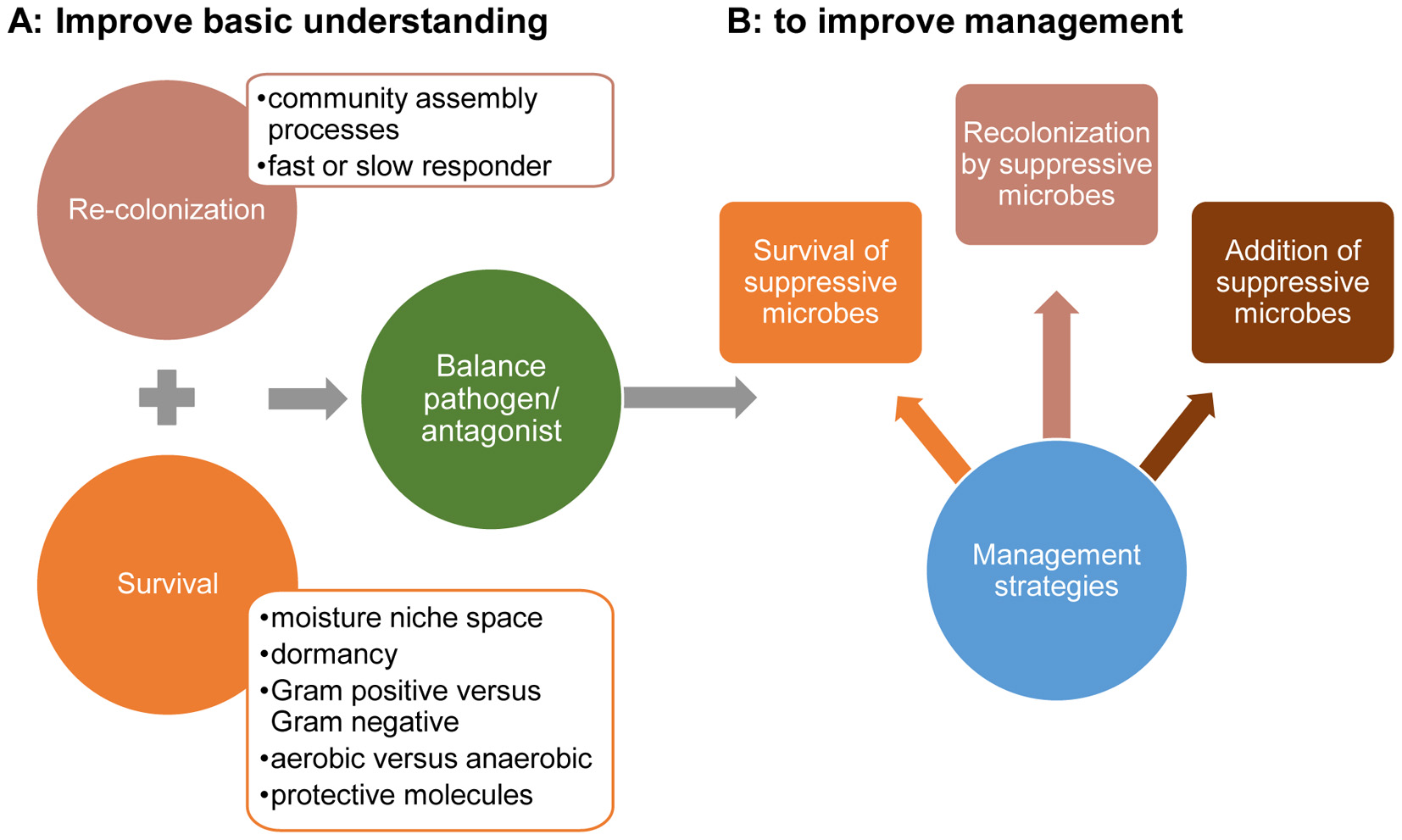
FIGURE 2. Future research priorities are to improve basic understanding of microbial interactions that affect the balance between pathogens and antagonists upon their survival during exposure to extreme water stress and recolonization strategies during moisture stress and upon recovery (A) and use this basic understanding to improve management strategies that improve the pathogen suppression (B).
Although there are many survival strategies to cope with drought and waterlogged conditions, cells of many soil microorganisms are irreversibly damaged (Nocker et al., 2012). For example, drying increases damage to DNA and enzymes (Dose et al., 1991; Potts, 1994). As a result, the active microbial biomass size is reduced upon recovery (Kieft et al., 1987; Lennon et al., 2012; Meisner et al., 2017). The partial elimination of microbes does result in an increase in the number of empty niches available upon recovery that both pathogens and other microbes can colonize. The success of colonization of empty niches by microbial species is determined by community assembly rules, such as priority effects. Priority effects describe the inhibitory or facilitative effects of early arriving species on next arriving ones (Fukami, 2015). Species that will recover faster from an extreme weather event will likely have a priority to become abundant first (Placella et al., 2012). In addition, dispersal due to movement of spores via wind or mixing of the content of soil pores during heavy rainfall and rewetting events can affect the composition of microbial species developing during recovery (Szekely and Langenheder, 2017).
The increased availability of easily available substrates upon recovery (Williams and Xia, 2009) due to increased necromass will act as a surplus of food sources for both pathogens and other microorganisms. This implies that the competitive pressure for energy resources is temporarily relieved. This is expected to coincide with a decrease in intensity of antagonistic interactions between microorganisms, including antagonistic interactions that suppress soil-borne plant pathogens. A similar condition can be created by adding easily available substrates to soils. For example non-mature compost can result in an increased infection by soil-borne pathogens (Hoitink and Grebus, 1994). Several factors can contribute to an increased risk for outbreaks of soil-borne pathogens during nutrient excess, namely (1) lower colonization of microorganisms that suppress pathogens (Hoitink et al., 1997); (2) decreased production of secondary metabolites due to investment of nutrients in growth and not in defense strategies (Coley et al., 1985; de Boer et al., 2003; Ghoul and Mitri, 2016); (3) reduced sensitivity of microorganisms, which are well fed, to inhibitory compounds, because they invest more in defense strategies (Garbeva et al., 2011). Thus, community assembly processes, the availability of labile nutrients and empty niches will influence the composition of the microbial communities during the recovery phase. Indeed, composition of microbial communities has often been observed to differ with different moisture treatments (Fierer et al., 2003; Drigo et al., 2017; Hartmann et al., 2017; Naylor and Coleman-Derr, 2017). In summary, water-related stress due to drought and rainfall events will change the interactions between microorganisms, which will affect the opportunities of pathogens to infect roots.
Future Research to Improve Agricultural Adaptation to Climate Change
Future research should take into account knowledge about microbial interactions, survival, and recovery of pathogens and antagonistic microorganisms during or after extreme water stress events to find strategies for increasing pathogen suppressive activities of microbes (Hawkes and Connor, 2017). Most important is to have insight in the key factors that affect the balance between heterotrophic soil microbes and pathogens. In this section, we will indicate knowledge gaps and management strategies that could be explored for the improvement of pathogen suppression upon the recovery of agricultural soil after drought or rainfall events.
Knowledge Needed to Improve Survival of Pathogen Suppressive Microorganisms
Survival of microorganisms is dependent on the moisture niche space and microbial traits (See “Balance Between Soil Pathogens and Heterotrophic Soil Microbes”). There are indications that drought is a natural selector for the microbial community, as microbial communities differ in soil with a legacy of drought, weeks to months after recovery (Bouskill et al., 2013; Meisner et al., 2018). Changes in the microbial community composition after a stress can affect the response of the microbial community to an additional drought stress. For example, microbial communities with a drought legacy seem to have a better ability to cope with an additional drought than microorganisms previously exposed to ambient conditions (Evans and Wallenstein, 2014). In addition, drought adapted microbes can improve fitness of plant species exposed to dry conditions (Lau and Lennon, 2012; Ngumbi and Kloepper, 2016). Drought-adapted microbes do not only improve the drought tolerance of their host plant, but also of other plants (Rodriguez et al., 2008; Marulanda et al., 2009). Drought exposed microorganisms can also recover faster to other stresses (van Kruistum et al., 2018). However, the question remains if drought-tolerant microorganisms suppress pathogens.
Microorganisms that survive waterlogged conditions need to cope with a wide range of oxygen concentrations (Neira et al., 2015). For example, Enterobacteriaceae have been observed to maintain metabolic activity when going from oxic to anoxic conditions after a rainfall event (Degelmann et al., 2009). In addition, a legacy of waterlogged conditions, such as flooding can result in a reduced suppression of bulb-rot causing Pythium spp. (van Os et al., 1999). The anaerobic activity of microbes is releasing compounds like organic acids, organic sulfides, and ammonia that can be toxic to aerobic microbes. This is the reason why stimulation of anaerobic decomposition of incorporated organic material into agricultural soils is used as a method to kill aerobic pathogens (Strauss and Kluepfel, 2015). However, changes in microbial community composition due to anaerobic disinfestation can cause a drastic reduction of the pathogen suppressive capacity of soils that remains present months after recovery (van Agtmaal et al., 2015). This implies that pathogens that will survive waterlogged conditions can remain abundant in the recovery phase. However, it is unknown if microorganisms that survive anaerobic conditions can improve pathogen suppression upon a second rainfall event.
Strategies to Improve Re-colonization of Pathogen Suppressive Microbes
Management strategies should focus on ways to improve re-colonization of empty niches by microbes that suppress pathogens, as this would allow for an earlier recovery of pathogen suppression. One way of improving recovery is the addition or manipulation of organic material, as the ‘carrying capacity of substrate’ has been suggested to regulate species composition, their abundance, and activity and therewith regulates the suppression of pathogens (Hoitink et al., 1997). Soil with higher carbon content can maintain higher moisture levels during droughts (Ng et al., 2015) and higher microbial biomass (Hueso et al., 2012). Accordingly, the addition of organic material may improve survival and create patches of microbes that can colonize empty niches upon recovery. However, difference in decomposition stage of the organic material can be important to consider. Early stages of the breakdown of organic material have many easily available substrates and are low in supporting pathogen suppression. In contrast, later stages with more recalcitrant substrates may have higher pathogen suppression (Hoitink et al., 1997; Bonanomi et al., 2010; Berg and McClaugherty, 2014). Differences in decomposition stage may explain why organic amendments can have different effects on the microbial biomass after recovery (Bapiri et al., 2010; Lado-Monserrat et al., 2014; Ng et al., 2015). As such, there are many avenues for future studies to identify if and how patches of organic material affect pathogen suppression during the recovery phase.
Pathogen suppression could also be managed by the addition of specific microorganisms or complete microbial communities (O’Hanlon et al., 2012). For example, the addition of a forest fungus (Penicillium WPTIIIA3) can increase yields of winter wheat when this species is exposed to drought and Fusarium pathogens (Ridout and Newcombe, 2016). This strategy would be beneficial when knowledge of the specific pathogen and pathogen suppressive microorganism is available (Borneman and Becker, 2007). However, added single strains need to establish and overcome the colonization resistance of the soil microbiome (van Veen et al., 1997; de Boer, 2017), which can be difficult due to the high diversity of soil microbial communities (van Elsas et al., 2012; Bashan et al., 2014). Thus, it can be difficult to overcome the colonization resistance of the resident community when all niches are filled with other microbes. These difficulties can change when extreme weather events result in empty niches for the introduced microorganism to establish. Therefore, the addition of beneficial microorganisms in the recovery phase may be successful as they can colonize empty niches and can be worthwhile to be investigated (Adam et al., 2016). The addition of beneficial microbes could potentially be combined by rewetting with water spraying systems during the recovery from drought conditions. An alternate strategy could be to engineer microbial communities that benefit host plants under climate change, suppress pathogens and are able to colonize, and survive in the soil environment (Oyserman et al., 2018). These beneficial microorganisms could belong to the group of plant growth promoting microorganisms as they have the ability to both improve the plants physiological response to drought in sterile soils (Mayak et al., 2004; Timmusk et al., 2014) and can act as disease control agent (Kloepper et al., 2004). However, future studies should identify plant growth promoting microorganisms that can both improve drought resistant and disease resistance in crops (Coleman-Derr and Tringe, 2014; Ngumbi and Kloepper, 2016).
Conclusion
We conclude that the higher sensitivity of crops to infections by soil-borne pathogens during and after extreme weather events is in part due to loss of the pathogen suppressive capacity of soils. Therefore, adaptation of agricultural ecosystems to changing climate scenarios should include improvements of pathogen suppression of soil during and after extreme drought and rainfall events. However, basic knowledge about effects of extreme weather events on microbial interactions, survival of microorganisms that induce pathogen suppression as well as recovery of the pathogen suppression appears not to be addressed in literature. This knowledge is needed to develop management strategies that improve pathogen suppressive soils (Figure 2). Management strategies should focus on improving survival and early recolonization of pathogen-suppressing microorganisms during the recovery phase after extreme weather events. Improved survival may be achieved via the natural selection of soil microorganisms to cope with drought or waterlogged conditions (selection by repeated stress) or via the addition of organic materials (survival spots). The challenge will be to find a strategy that allows to manage both drought and waterlogged conditions as the microorganism that respond to drought will differ from the ones that survive waterlogged conditions. In addition, improved and faster recovery of pathogen suppressive microorganisms can be managed by the addition of pathogen suppressive microorganisms. As such, there are many research directions to improve our understanding of pathogen suppression during and upon recovery to the drought and rainfall events. This understanding is needed to adapt agricultural ecosystems to changing climate scenarios.
Author Contributions
AM and WB conceived and designed the ideas for the article and wrote the manuscript.
Funding
AM was supported by an international career grant from the Swedish Research Council (VR, Grant No. 330-2014-6430) and Marie Sklodowska-Curie Actions (Cofund Project INCA600398).
Conflict of Interest Statement
The authors declare that the research was conducted in the absence of any commercial or financial relationships that could be construed as a potential conflict of interest.
Acknowledgments
We thank Paolina Garbeva for comments on a previous version of the manuscript. This is NIOO publication 6597.
References
Adam, E., Groenenboom, A. E., Kurm, V., Rajewska, M., Schmidt, R., Tyc, O., et al. (2016). Controlling the microbiome: microhabitat adjustments for successful biocontrol strategies in soil and human gut. Front. Microbiol. 7:1079. doi: 10.3389/fmicb.2016.01079
Bapiri, A., Bååth, E., and Rousk, J. (2010). Drying-rewetting cycles affect fungal and bacterial growth differently in an arable soil. Microb. Ecol. 60, 419–428. doi: 10.1007/s00248-010-9723-5
Barnard, R. L., Osborne, C. A., and Firestone, M. K. (2013). Responses of soil bacterial and fungal communities to extreme desiccation and rewetting. ISME J. 7, 2229–2241. doi: 10.1038/ismej.2013.104
Barnard, R. L., Osborne, C. A., and Firestone, M. K. (2015). Changing precipitation pattern alters soil microbial community response to wet-up under a Mediterranean-type climate. ISME J. 9, 946–957. doi: 10.1038/ismej.2014.192
Bashan, Y., de-Bashan, L. E., Prabhu, S. R., and Hernandez, J.-P. (2014). Advances in plant growth-promoting bacterial inoculant technology: formulations and practical perspectives (1998–2013). Plant Soil 378, 1–33. doi: 10.1007/s11104-013-1956-x
Bastos, A. C., and Magan, N. (2007). Soil volatile fingerprints: use for discrimination between soil types under different environmental conditions. Sens. Actuators B Chem. 125, 556–562. doi: 10.1016/j.snb.2007.03.001
Berendsen, R. L., Pieterse, C. M., and Bakker, P. A. (2012). The rhizosphere microbiome and plant health. Trends Plant Sci. 17, 478–486. doi: 10.1016/j.tplants.2012.04.001
Berg, B., and McClaugherty, C. (2014). Plant Litter: Decomposition, Humus Formation, Carbon Sequestration. Berlin: Springer.
Bonanomi, G., Antignani, V., Capodilupo, M., and Scala, F. (2010). Identifying the characteristics of organic soil amendments that suppress soilborne plant diseases. Soil Biol. Biochem. 42, 136–144. doi: 10.1016/j.soilbio.2009.10.012
Borneman, J., and Becker, J. O. (2007). Identifying microorganisms involved in specific pathogen suppression in soil. Annu. Rev. Phytopathol. 45, 153–172. doi: 10.1146/annurev.phyto.45.062806.094354
Bouskill, N. J., Lim, H. C., Borglin, S., Salve, R., Wood, T. E., Silver, W. L., et al. (2013). Pre-exposure to drought increases the resistance of tropical forest soil bacterial communities to extended drought. ISME J. 7, 384–394. doi: 10.1038/ismej.2012.113
Challinor, A. J., Koehler, A. K., Ramirez-Villegas, J., Whitfield, S., and Das, B. (2016). Current warming will reduce yields unless maize breeding and seed systems adapt immediately. Nat. Clim. Chang. 6, 954–958. doi: 10.1038/nclimate3061
Challinor, A. J., Watson, J., Lobell, D. B., Howden, S. M., Smith, D. R., and Chhetri, N. (2014). A meta-analysis of crop yield under climate change and adaptation. Nat. Clim. Chang. 4, 287–291. doi: 10.1038/nclimate2153
Coleman-Derr, D., and Tringe, S. G. (2014). Building the crops of tomorrow: advantages of symbiont-based approaches to improving abiotic stress tolerance. Front. Microbiol. 5:283. doi: 10.3389/fmicb.2014.00283
Coley, P. D., Bryant, J. P., and Chapin, F. S. (1985). Resource availability and plant antiherbivore defense. Science 230, 895–899. doi: 10.1126/science.230.4728.895
de Boer, W. (2017). Upscaling of fungal–bacterial interactions: from the lab to the field. Curr. Opin. Microbiol. 37, 35–41. doi: 10.1016/j.mib.2017.03.007
de Boer, W., Verheggen, P., Klein Gunnewiek, P. J. A., Kowalchuk, G. A., and van Veen, J. A. (2003). Microbial community composition affects soil fungistasis. Appl. Environ. Microbiol. 69, 835–844. doi: 10.1128/AEM.69.2.835-844.2003
de Vries, F. T., Griffiths, R. I., Bailey, M., Craig, H., Girlanda, M., Gweon, H. S., et al. (2018). Soil bacterial networks are less stable under drought than fungal networks. Nat. Commun. 9:3033. doi: 10.1038/s41467-018-05516-7
Degelmann, D. M., Kolb, S., Dumont, M., Murrell, J. C., and Drake, H. L. (2009). Enterobacteriaceae facilitate the anaerobic degradation of glucose by a forest soil. FEMS Microbiol. Ecol. 68, 312–319. doi: 10.1111/j.1574-6941.2009.00681.x
Dikilitas, M., Karakas, S., Hashem, A., Abd Allah, E. F., and Ahmad, P. (2016). “Oxidative stress and plant responses to pathogens under drought conditions,” in Water Stress and Crop Plants: a Sustainable Approach, ed. P. Ahmad (Hoboken, NJ: John Wiley & Sons), 102–123.
Dixon, G. R., and Tilston, E. L. (2010). “Soil-borne pathogens and their interactions with the soil environment,” in Soil Microbiology and Sustainable Crop Production, eds G. R. Dixon and E. L. Tilston (Dordrecht: Springer Netherlands), 197–271.
Dose, K., Biegerdose, A., Kerz, O., and Gill, M. (1991). DNA-strand breaks limit survival in extreme dryness. Orig. Life Evol. Biosph. 21, 177–187. doi: 10.1007/bf01809446
Drigo, B., Nielsen, U. N., Jeffries, T. C., Curlevski, N. J. A., Singh, B. K., Duursma, R. A., et al. (2017). Interactive effects of seasonal drought and elevated atmospheric carbon dioxide concentration on prokaryotic rhizosphere communities. Environ. Microbiol. 19, 3175–3185. doi: 10.1111/1462-2920.13802
Duncan, J. M., and Kennedy, D. M. (1989). The effect of waterlogging on Phytophthora root rot of red raspberry. Plant Pathol. 38, 161–168. doi: 10.1111/j.1365-3059.1989.tb02129.x
Duran, P., Jorquera, M., Viscardi, S., Carrion, V. J., de la Luz Mora, M., and Pozo, M. J. (2017). Screening and characterization of potentially suppressive soils against Gaeumannomyces graminis under extensive wheat cropping by Chilean indigenous communities. Front. Microbiol. 8:1552. doi: 10.3389/fmicb.2017.01552
Eurostats (2016). Agriculture, Forestry and Fishery Statistics. Luxembourg: Publications Office of the European Union.
Evans, S. E., and Wallenstein, M. D. (2014). Climate change alters ecological strategies of soil bacteria. Ecol. Lett. 17, 155–164. doi: 10.1111/ele.12206
Fierer, N., Schimel, J. P., and Holden, P. A. (2003). Influence of drying-rewetting frequency on soil bacterial community structure. Microb. Ecol. 45, 63–71. doi: 10.1007/s00248-002-1007-2
Fischer, E. M., and Knutti, R. (2016). Observed heavy precipitation increase confirms theory and early models. Nat. Clim. Chang. 6, 986–991. doi: 10.1038/nclimate3110
Fukami, T. (2015). Historical contingency in community assembly: integrating niches, species pools, and priority effects. Annu. Rev. Ecol. Evol. Syst. 46, 1–23. doi: 10.1146/annurev-ecolsys-110411-160340
Garbeva, P., Hol, W. H. G., Termorshuizen, A. J., Kowalchuk, G. A., and de Boer, W. (2011). Fungistasis and general soil biostasis – A new synthesis. Soil Biol. Biochem. 43, 469–477. doi: 10.1016/j.soilbio.2010.11.020
Ghoul, M., and Mitri, S. (2016). The ecology and evolution of microbial competition. Trends Microbiol. 24, 833–845. doi: 10.1016/j.tim.2016.06.011
Hartmann, M., Brunner, I., Hagedorn, F., Bardgett, R. D., Stierli, B., Herzog, C., et al. (2017). A decade of irrigation transforms the soil microbiome of a semi-arid pine forest. Mol. Ecol. 26, 1190–1206. doi: 10.1111/mec.13995
Hawkes, C. V., and Connor, E. W. (2017). Translating phytobiomes from theory to practice: ecological and evolutionary considerations. Phytobiomes 1, 57–69. doi: 10.1094/PBIOMES-05-17-0019-RVW
Hiltpold, I., and Turlings, T. C. J. (2008). Belowground chemical signaling in maize: when simplicity rhymes with efficiency. J. Chem. Ecol. 34, 628–635. doi: 10.1007/s10886-008-9467-6
Ho, W. C., and Ko, W. H. (1985). Soil microbiostasis: effects of environmental and edaphic factors. Soil Biol. Biochem. 17, 167–170. doi: 10.1016/0038-0717(85)90110-5
Hoitink, H. A. J., and Grebus, M. E. (1994). Status of biological control of plant diseases with composts. Compost Sci. Util. 2, 6–12. doi: 10.1080/1065657X.1994.10771134
Hoitink, H. A. J., Stone, A. G., and Han, D. Y. (1997). Suppression of plant diseases by composts. Hortscience 32, 184–187.
Hol, W. H. G., Garbeva, P., Hordijk, C., Hundscheid, M. P. J., Gunnewiek, P. J. A. K., van Agtmaal, M., et al. (2015). Non-random species loss in bacterial communities reduces antifungal volatile production. Ecology 96, 2042–2048. doi: 10.1890/14-2359.1
Hueso, S., García, C., and Hernández, T. (2012). Severe drought conditions modify the microbial community structure, size and activity in amended and unamended soils. Soil Biol. Biochem. 50, 167–173. doi: 10.1016/j.soilbio.2012.03.026
IPCC (2012). Managing the Risks of Extreme Events and Disasters to Advance Climate Change Adaptation. A Special Report of Working Groups I and II of the Intergovernmental Panel on Climate Change, eds C. B. Field, V. Barros, T. F. Stocker, D. Qin, D. J. Dokken, K. L. Ebi, et al. (Cambridge: Cambridge University Press).
Judelson, H. S., and Blanco, F. A. (2005). The spores of Phytophthora: weapons of the plant destroyer. Nat. Rev. Microbiol. 3, 47–58. doi: 10.1038/nrmicro1064
Kieft, T. L., Soroker, E., and Firestone, M. K. (1987). Microbial biomass response to a rapid increase in water potential when dry soil is wetted. Soil Biol. Biochem. 19, 119–126. doi: 10.1016/0038-0717(87)90070-8
Kloepper, J. W., Ryu, C.-M., and Zhang, S. (2004). Induced Systemic Resistance and Promotion of Plant Growth by Bacillus spp. Phytopathology 94, 1259–1266. doi: 10.1094/PHYTO.2004.94.11.1259
Lado-Monserrat, L., Lull, C., Bautista, I., Lidón, A., and Herrera, R. (2014). Soil moisture increment as a controlling variable of the “Birch effect”. Interactions with the pre-wetting soil moisture and litter addition. Plant Soil 379, 21–34. doi: 10.1007/s11104-014-2037-5
Lau, J. A., and Lennon, J. T. (2012). Rapid responses of soil microorganisms improve plant fitness in novel environments. Proc. Natl. Acad. Sci. U.S.A. 109, 14058–14062. doi: 10.1073/pnas.1202319109
Lennon, J. T., Aanderud, Z. T., Lehmkuhl, B. K., and Schoolmaster, D. R. (2012). Mapping the niche space of soil microorganisms using taxonomy and traits. Ecology 93, 1867–1879. doi: 10.1890/11-1745.1
Lootsma, M., and Scholte, K. (1997). Effect of soil moisture content on the suppression of Rhizoctonia stem canker on potato by the nematode Aphelenchus avenae and the springtail Folsomia fimetaria. Plant Pathol. 46, 209–215. doi: 10.1046/j.1365-3059.1997.d01-229.x
Malajczuk, N., and Theodorou, C. (1979). Influence of water potential on growth and cultural-characteristics of phytophthora-cinnamomi. Trans. Br. Mycol. Soc. 72, 15–18. doi: 10.1016/s0007-1536(79)80002-9
Manzoni, S., Schaeffer, S. M., Katul, G., Porporato, A., and Schimel, J. P. (2014). A theoretical analysis of microbial eco-physiological and diffusion limitations to carbon cycling in drying soils. Soil Biol. Biochem. 73, 69–83. doi: 10.1016/j.soilbio.2014.02.008
Marulanda, A., Barea, J.-M., and Azcón, R. (2009). Stimulation of plant growth and drought tolerance by native microorganisms (AM Fungi and Bacteria) from dry environments: mechanisms related to bacterial effectiveness. J. Plant Growth Regul. 28, 115–124. doi: 10.1007/s00344-009-9079-6
Mayak, S., Tirosh, T., and Glick, B. R. (2004). Plant growth-promoting bacteria that confer resistance to water stress in tomatoes and peppers. Plant Sci. 166, 525–530. doi: 10.1016/j.plantsci.2003.10.025
Meisner, A., Bååth, E., and Rousk, J. (2013). Microbial growth responses upon rewetting soil dried for four days or one year. Soil Biol. Biochem. 66, 188–192. doi: 10.1016/j.soilbio.2013.07.014
Meisner, A., Jacquiod, S., Snoek, B. L., ten Hooven, F. C., and van der Putten, W. H. (2018). Drought legacy effects on the composition of soil fungal and prokaryote communities. Front. Microbiol. 9:294. doi: 10.3389/fmicb.2018.00294
Meisner, A., Leizeaga, A., Rousk, J., and Bååth, E. (2017). Partial drying accelerates bacterial growth recovery to rewetting. Soil Biol. Biochem. 112, 269–276. doi: 10.1016/j.soilbio.2017.05.016
Moyano, F. E., Manzoni, S., and Chenu, C. (2013). Responses of soil heterotrophic respiration to moisture availability: an exploration of processes and models. Soil Biol. Biochem. 59, 72–85. doi: 10.1016/j.soilbio.2013.01.002
Naylor, D., and Coleman-Derr, D. (2017). Drought stress and root-associated bacterial communities. Front. Plant Sci. 8:2223. doi: 10.3389/fpls.2017.02223
Neira, J., Ortiz, M., Morales, L., and Acevedo, E. (2015). Oxygen diffusion in soils: understanding the factors and processes needed for modeling. Chil. J. Agric. Res. 75, 35–44. doi: 10.4067/s0718-58392015000300005
Ng, E. L., Patti, A. F., Rose, M. T., Schefe, C. R., Smernik, R. J., and Cavagnaro, T. R. (2015). Do organic inputs alter resistance and resilience of soil microbial community to drying? Soil Biol. Biochem. 81, 58–66. doi: 10.1016/j.soilbio.2014.10.028
Ngumbi, E., and Kloepper, J. (2016). Bacterial-mediated drought tolerance: current and future prospects. Appl. Soil Ecol. 105, 109–125. doi: 10.1016/j.apsoil.2016.04.009
Nocker, A., Fernandez, P. S., Montijn, R., and Schuren, F. (2012). Effect of air drying on bacterial viability: a multiparameter viability assessment. J. Microbiol. Methods 90, 86–95. doi: 10.1016/j.mimet.2012.04.015
Obidiegwu, J. E., Bryan, G. J., Jones, H. G., and Prashar, A. (2015). Coping with drought: stress and adaptive responses in potato and perspectives for improvement. Front. Plant Sci. 6:542. doi: 10.3389/fpls.2015.00542
O’Hanlon, K. A., Knorr, K., Jorgensen, L. N., Nicolaisen, M., and Boelt, B. (2012). Exploring the potential of symbiotic fungal endophytes in cereal disease suppression. Biol. Control 63, 69–78. doi: 10.1016/j.biocontrol.2012.08.007
Or, D., Smets, B. F., Wraith, J. M., Dechesne, A., and Friedman, S. P. (2007). Physical constraints affecting bacterial habitats and activity in unsaturated porous media – a review. Adv. Water Resour. 30, 1505–1527. doi: 10.1016/j.advwatres.2006.05.025
Oyserman, B. O., Medema, M. H., and Raaijmakers, J. M. (2018). Road MAPs to engineer host microbiomes. Curr. Opin. Microbiol. 43, 46–54. doi: 10.1016/j.mib.2017.11.023
Peñuelas, J., Asensio, D., Tholl, D., Wenke, K., Rosenkranz, M., Piechulla, B., et al. (2014). Biogenic volatile emissions from the soil. Plant Cell Environ. 37, 1866–1891. doi: 10.1111/pce.12340
Philippot, L., Raaijmakers, J. M., Lemanceau, P., and van der Putten, W. H. (2013). Going back to the roots: the microbial ecology of the rhizosphere. Nat. Rev. Microbiol. 11, 789–799. doi: 10.1038/nrmicro3109
Placella, S. A., Brodie, E. L., and Firestone, M. K. (2012). Rainfall-induced carbon dioxide pulses result from sequential resuscitation of phylogenetically clustered microbial groups. Proc. Natl. Acad. Sci. U.S.A. 109, 10931–10936. doi: 10.1073/pnas.1204306109
Raaijmakers, J. M., and Mazzola, M. (2012). Diversity and natural functions of antibiotics produced by beneficial and plant pathogenic bacteria. Annu. Rev. Phytopathol. 50, 403–424. doi: 10.1146/annurev-phyto-081211-172908
Raaijmakers, J. M., and Mazzola, M. (2016). Soil immune responses. Science 352, 1392–1393. doi: 10.1126/science.aaf3252
Ramegowda, V., and Senthil-Kumar, M. (2015). The interactive effects of simultaneous biotic and abiotic stresses on plants: mechanistic understanding from drought and pathogen combination. J. Plant Physiol. 176, 47–54. doi: 10.1016/j.jplph.2014.11.008
Ridout, M., and Newcombe, G. (2016). Disease suppression in winter wheat from novel symbiosis with forest fungi. Fungal Ecol. 20, 40–48. doi: 10.1016/j.funeco.2015.10.005
Rodriguez, R. J., Henson, J., Van Volkenburgh, E., Hoy, M., Wright, L., Beckwith, F., et al. (2008). Stress tolerance in plants via habitat-adapted symbiosis. ISME J. 2, 404–416. doi: 10.1038/ismej.2007.106
Schimel, J., Balser, T. C., and Wallenstein, M. (2007). Microbial stress-response physiology and its implications for ecosystem function. Ecology 88, 1386–1394. doi: 10.1890/06-0219
Schmidt, R., Cordovez, V., de Boer, W., Raaijmakers, J., and Garbeva, P. (2015). Volatile affairs in microbial interactions. ISME J. 9, 2329–2335. doi: 10.1038/ismej.2015.42
Schulz-Bohm, K., Martín-Sánchez, L., and Garbeva, P. (2017). Microbial volatiles: small molecules with an important role in intra- and inter-kingdom interactions. Front. Microbiol. 8:2484. doi: 10.3389/fmicb.2017.02484
Shoemaker, W. R., and Lennon, J. T. (2018). Evolution with a seed bank: the population genetic consequences of microbial dormancy. Evol. Appl. 11, 60–75. doi: 10.1111/eva.12557
Strauss, S. L., and Kluepfel, D. A. (2015). Anaerobic soil disinfestation: a chemical-independent approach to pre-plant control of plant pathogens. J. Integr. Agric. 14, 2309–2318. doi: 10.1016/s2095-3119(15)61118-2
Szekely, A. J., and Langenheder, S. (2017). Dispersal timing and drought history influence the response of bacterioplankton to drying-rewetting stress. ISME J. 11, 1764–1776. doi: 10.1038/ismej.2017.55
Thompson, S., Levin, S., and Rodriguez-Iturbe, I. (2013). Linking plant disease risk and precipitation drivers: a dynamical systems framework. Am. Nat. 181, E1–E16. doi: 10.1086/668572
Timmusk, S., Abd El-Daim, I. A., Copolovici, L., Tanilas, T., Kännaste, A., Behers, L., et al. (2014). Drought-tolerance of wheat improved by rhizosphere bacteria from harsh environments: enhanced biomass production and reduced emissions of stress volatiles. PLoS One 9:e96086. doi: 10.1371/journal.pone.0096086
Tyc, O., Song, C., Dickschat, J. S., Vos, M., and Garbeva, P. (2017). The ecological role of volatile and soluble secondary metabolites produced by soil bacteria. Trends Microbiol. 25, 280–292. doi: 10.1016/j.tim.2016.12.002
van Agtmaal, M., Straathof, A. L., Termorshuizen, A., Lievens, B., Hoffland, E., and de Boer, W. (2018). Volatile-mediated suppression of plant pathogens is related to soil properties and microbial community composition. Soil Biol. Biochem. 117, 164–174. doi: 10.1016/j.soilbio.2017.11.015
van Agtmaal, M., van Os, G., Hol, G., Hundscheid, M., Runia, W., Hordijk, C., et al. (2015). Legacy effects of anaerobic soil disinfestation on soil bacterial community composition and production of pathogen-suppressing volatiles. Front. Microbiol. 6:701. doi: 10.3389/fmicb.2015.00701
van Elsas, J. D., Chiurazzi, M., Mallon, C. A., Elhottovă, D., Krištùfek, V., and Salles, J. F. (2012). Microbial diversity determines the invasion of soil by a bacterial pathogen. Proc. Natl. Acad. Sci. U.S.A. 109, 1159–1164. doi: 10.1073/pnas.1109326109
van Kruistum, H., Bodelier, P. L. E., Ho, A., Meima-Franke, M., and Veraart, A. J. (2018). Resistance and recovery of methane-oxidizing communities depends on stress regime and history; a microcosm study. Front. Microbiol. 9:1714. doi: 10.3389/fmicb.2018.01714
van Os, G. J., Wijnker, J. P. M., and van Gulik, W. J. M. (1999). Effects of soil fumigation and flooding on suppression of Pythium root rot in ornamental bulb culture. Eur. J. Plant Pathol. 105, 791–800. doi: 10.1023/a:1008722517813
van Veen, J. A., van Overbeek, L. S., and van Elsas, J. D. (1997). Fate and activity of microorganisms introduced into soil. Microbiol. Mol. Biol. Rev. 61, 121–135.
Warren, C. R. (2014). Response of osmolytes in soil to drying and rewetting. Soil Biol. Biochem. 70, 22–32. doi: 10.1016/j.soilbio.2013.12.008
Whiting, E. C., Khan, A., and Gubler, W. D. (2001). Effect of temperature and water potential on survival and mycelial growth of Phaeomoniella chlamydospora and Phaeoacremonium spp. Plant Dis. 85, 195–201. doi: 10.1094/PDIS.2001.85.2.195
Keywords: extreme weather events, climate change, crop, pathogen, disease suppression, soil microorganisms, antagonistic interactions
Citation: Meisner A and de Boer W (2018) Strategies to Maintain Natural Biocontrol of Soil-Borne Crop Diseases During Severe Drought and Rainfall Events. Front. Microbiol. 9:2279. doi: 10.3389/fmicb.2018.02279
Received: 04 June 2018; Accepted: 06 September 2018;
Published: 02 November 2018.
Edited by:
Adrian Ho, Leibniz Universität Hannover, GermanyReviewed by:
Katharina Kujala, University of Oulu, FinlandHamed Azarbad, Institute Armand Frappier (INRS) and University of Québec, Canada
Copyright © 2018 Meisner and de Boer. This is an open-access article distributed under the terms of the Creative Commons Attribution License (CC BY). The use, distribution or reproduction in other forums is permitted, provided the original author(s) and the copyright owner(s) are credited and that the original publication in this journal is cited, in accordance with accepted academic practice. No use, distribution or reproduction is permitted which does not comply with these terms.
*Correspondence: Annelein Meisner, QW5uZWxlaW4uTWVpc25lckBiaW9sLmx1LnNl