- Centro de Referencia para Lactobacilos, Consejo Nacional de Investigaciones Científicas y Técnicas, San Miguel de Tucuman, Argentina
In order to eliminate the widespread use of antibiotics in livestock production, the research for alternatives has increased lately. This study examined the safety of 40 lactic acid bacteria (LAB) isolated from bovine feedlot environment and previously selected as potential probiotics. A high sensitivity prevalence to ampicillin (AMP, 100%), gentamicin (GEN, 96.3%), kanamycin (KAN, 96.3%), clindamycin (CLI, 85.2%), chloramphenicol (CHL, 92.6%) and streptomycin (STR, 88.9%) while moderate and high resistance against erythromycin (ERY, 48%) and tetracycline (TET, 79%) respectively, were determined. Feedlot enterococci and pediococci displayed high resistance to CLI, ERY, GEN and TET (73, 100, 54.5, and 73%, respectively). Among fifteen resistance genes investigated, seven were identified in lactobacilli; their presence not always was correlated with phenotypic resistance. STR resistance genes, aadA and ant(6) were observed in 7.4 and 3.7% of isolates, respectively; genes responsible for aminoglycosides resistance, such as bla (7.4%), and aph(3”)-III (3.7%) were also recognized. In addition, resistance cat and tetS genes (3.7 and 7.4%, respectively) were harbored by feedlot lactobacilli strains. The presence of ermB gene in 22.3% of isolates, including two of the six strains phenotypically resistant to ERY, exhibited the highest prevalence among the assessed antibiotics. None of the feedlot lactobacilli harbored virulence factors genes, while positive PCR amplification for ace, agg, fsrA, and atpA genes was found for enterococci. With the objective of producing large cell biomass for probiotic delivery, growth media without peptone but containing glucose and skim milk powder (Mgl and Mlac) were selected as optimal. Lactobacillus acidophilus CRL2074, L. amylovorus CRL2115, L. mucosae CRL2069, and L. rhamnosus CRL2084 were strains selected as free of antibiotic resistance and virulence determinants, able to reach high cell numbers in non-expensive culture media and being compatible among them.
Introduction
In the last 20 years, direct-fed-microbials (DFM) supplementation to improve the health and performance of livestock has generated a great interest. Specifically for feedlot beef cattle, main targets for probiotics are health promotion to avoid or reduce ruminal acidosis, increase weight gain and feed conversion as well as human pathogens shedding decrease (Retta, 2016). Ruminants benefit from the symbiosis between the host and the rumen microbes, which supply protein, vitamins and short chain organic acids for the animal host (Chaucheyras-Durand and Durand, 2010). Defined as “live microorganisms which when administered in adequate amounts confer a health benefit on the host” (FAO, 2002; Hill et al., 2014), probiotic bacteria represent a new and efficient alternative to traditional prophylactic therapies for animal management in artificial environments. The increased interest for DFM application constitute a driving force to reduce or eliminate the use of low-dose antibiotics in livestock production; low antibiotic concentrations found in natural environments lead to enrich resistant bacterial populations (Gullberg et al., 2011). In the European Union, the use of antibiotics for animal growth promotion was banned in 2006 and a similar ban for animal husbandry is currently discussed in United States of America (Cantas et al., 2013). Internationally, many countries have adopted mandatory restrictions on antimicrobial use, and veterinary prescription to use these drugs in food animals are obligatory (Maron et al., 2013).
According to WHO (World Health Organization) to attain a probiotic status, microorganisms have to meet some of the principles related to their safety and biological properties. Lactic acid bacteria (LAB), besides their essential role in food fermentations are also important as probiotics. For this type of use, requirements for safety assessment have increased; they should not exhibit neither pathogenic activity nor antibiotic resistance (AR) encoding genes and sustain genetic stability. The evaluation of the antibiotic susceptibility of LAB has recently grown because of their potential to spread resistance by horizontal gene transfer in which plasmids, transposons and integrons are involved; these mobile elements include AR genes mostly responsible for intra- and inter-species transfer of genetic material (van Reenen and Dicks, 2011; Gueimonde et al., 2013). The large numbers of LAB in fermented products and in the gastrointestinal tract (GIT) supports the presence of different resistance mechanisms via mutation; once a bacteria becomes resistant, the element is amplified and may be transmitted to another host. Enterococcus species have been described as a major source of nosocomial infections in human and veterinary medicine and a correlation of AR and infective determinants was established (Garcia-Migura et al., 2014). Enterococci factors that contribute to pathogenesis include cytolysin, aggregation substance, adhesins and hydrolytic enzymes (Franz et al., 2011). Remarkably, food isolated enterococci were shown to harbor either single or multiple virulence factors, however, their incidences among probiotic enterococci strains were noticeably lower (Franz et al., 2011; Beukers et al., 2015; Imperial and Ibana, 2016). Although lower occurrence, AR for non-enterococcal LAB emerged from medical, veterinary and food sources; the presence of potentially transferable resistance genes has been established, which appear to be intrinsic as well as transferable (Ammor et al., 2007; Devirgiliis et al., 2013; Abriouel et al., 2015). Among the microorganisms used in animal feed, mainly Gram-positive bacterial strains that act as bioregulators of the intestinal microbiota and enhancers of host’s natural defenses, were applied (Hill et al., 2014). As normal components of animal GIT microbiota, different genera and species of LAB were used to potentially modulate GI microbial health, nutrient use and animal productivity (Retta, 2016).
On the other hand, as first step for probiotic delivery to feedlot cattle, optimization of an effective and low cost growth medium for culturing LAB must be achieved. Because LAB are fastidious microorganisms and many elements like carbohydrates, amino acids, peptides, vitamins and Mg/Mn salts are required for growth, commercial media are generally optimal, but due to the high cost they result inappropriate for large-scale biomass production. In addition, LAB growth activity is affected by culture conditions such as pH, temperature, medium formulation, and others. Among ingredients, yeast extract was found as highly significant for enhanced biomass production in low cost cultivation conditions (Chiang et al., 2015; Manzoor et al., 2017). In this study, in view to design a probiotic formula for its administration to feedlot cattle, AR and virulence factors incidence for 40 LAB strains isolated from cattle environment, were investigated. In addition, optimal growth conditions of selected probiotic LAB strains were preliminary investigated for high cell mass production.
Materials and Methods
Microorganisms and Growth Conditions
Forty LAB previously isolated from feedlot cattle environment and selected for their beneficial characteristics (Maldonado et al., 2018) were used, including Lactobacillus (L.) acidophilus (3), L. amylovorus (4), L. casei (1), L. fermentum (1), L. mucosae (14), L. plantarum (3), L. rhamnosus (1), Pediococcus (P.) acidilactici (2), Enterococcus (E.) durans (3), E. faecium (2) and E. hirae (6). Inoculum of strains were prepared by transferring glycerol stock culture to MRS broth (Merck, Darmstadt, Germany) and sub-cultured twice in the same media at 37°C for 16 h.
Safety Evaluation
Phenotypic Antibiotic Resistance and MIC Determination
Minimum inhibitory concentrations (MIC, μg/ml) of eight antibiotics: ampicillin (AMP), clindamycin (CLI), chloramphenicol (CHL), erythromycin (ERY), gentamicin (GEN), kanamycin (KAN), tetracycline (TET), and streptomycin (STR) were determined for LAB strains (40) according to ISO 10932:2010 standard. Epidemiological cut-off values based on the recommendation of the European Committee on Antimicrobial Susceptibility Testing (EUCAST) and EFSA-FEEDAP (2012) Panel on Additives and Products or Substance used in Animal Feeding (FEEDAP) were applied. All antibiotics were purchased from Sigma–Aldrich (St. Louis, MO, United States and ICN Biomedicals, Santa Ana, CA, United States). In parallel, accuracy of susceptibility testing was monitored by the use of quality control strains (Lactobacillus plantarum ATCC14917, Enterococcus faecalis ATCC29212).
Hemolysin and Gelatinase Activity
Hemolysin activity was determined on Columbia Blood Agar (Oxoid) containing 5% defibrinized horse blood after 48 h of incubation at 37°C, both under aerobic and anaerobic conditions. Zones of clearing around colonies indicated β-haemolysin production. Gelatinase production was detected by inoculating LAB onto freshly prepared peptone-yeast extract agar containing gelatin (30 g/L; Difco). Plates were incubated overnight at 37°C and cooled at ambient temperature for 2 h. The appearance of a turbid zone around the colonies was considered as positive result for gelatinase production.
PCR Detection of Antimicrobial Resistance Genes and Potential Virulence Factors
The presence of genes coding for AR in LAB strains phenotypically susceptible to antibiotics (described above) and virulence factors were evaluated through PCR reactions. Specific primers used and their target genes, amplicon sizes and PCR protocol references used for genes detection are shown in Table 1. PCR-amplifications were performed from total bacteria DNA obtained according to Pospiech and Neumann (1995) in 25 μl reaction mixture containing 1 μl of purified DNA, 1 μM of each primers, 0.1 mM of each dNTP (2.5 Mm), buffer 1x, 1.5 mM MgCl2 (25 Mm) and 2.5 U/100 μl of Taq polymerase (Inbio Highway, Argentina). Samples were subjected to an initial cycle of denaturation (94°C for 5 min), followed by 28 cycles of denaturation (94°C for 1 min), annealing for 1 min at the temperature of the primer pairs and elongation (72°C for 1 min 30 seg), ending with one cycle of final extension (72°C for 5 min) in a MyCyclerTM (BioRad, Richmond, CA, United States) thermocycler. PCR-products were separated by electrophoresis on 1% (w/v) agarose at 80 V for 45 min. Gels were stained with GelRedTM (Biotium Inc., Hayward, CA, United States) and visualized with a UV light transilluminator (320 nm). The molecular size marker used was 1 kb Plus DNA ladder (Invitrogen, Buenos Aires, Argentina).
Optimization of Growth Medium for Probiotic Strains Production
Based on safety results, L. acidophilus CRL2074, L. amylovorus CRL2116, L. mucosae CRL2069, and L. rhamnosus CRL2084 were selected and the impact of different culture media formulated with various ingredients on biomass production was investigated. Five combinations of different components, MRS and MRSc (pH was controlled by adding NaOH 5N at 6, 18, and 24 h) were evaluated, their compositions being shown in Table 2. Before the trial, selected LAB strains were inoculated (2%) in 5 ml of each prepared media and sub-cultured twice during 12 h at 37°C. For each medium and LAB strain, viable LAB were quantified after dilutions and plating on MRS agar. Maximum growth rate (μ h-1) and growth potential (CFU/mL at 24 h – CFU/mL at 0 h) were determined.
Compatibility of Selected LAB Strains
Beneficial LAB strains previously selected were tested for interactions among them. MRS (15 ml) melted and tempered at 45°C were vigorously mixed with 200 μL of an overnight culture of each LAB and poured into Petri dishes. Wells of 10 mm in diameter were cut in the agar and 30 μL of the cell-free supernatant of each strain was placed into each well. The plates were incubated aerobically overnight at 37°C, and inhibition halos observed.
Statistics
Agar assays were performed by duplicate and growth curves by triplicate. In the case of AR, media values were compared with cut-off points. The media and SD were calculated for growth curves, results (means OD ± SD) being evaluated by the application of ANOVA to define differences and statistical significances were determined by the Tukey test.
Results and Discussion
Antimicrobial Susceptibility Testing
The use of probiotics instead of antibiotic therapy is gaining acceptance worldwide to alleviate antibiotic-mediated complications and enhance livestock health conditions. However, safety concerns have been raised by the use of LAB strains carrying AR genes themselves, as they can potentially transfer them to other commensal and/or pathogenic bacteria through horizontal gene mechanisms (Imperial and Ibana, 2016). Thus, to use as probiotics, safety traits of forty LAB strains previously isolated and identified from feedlot cattle environment (Maldonado et al., 2018), were investigated. The MIC of eight antimicrobial agents for 40 LAB strains involving Lactobacillus (27), Pediococcus (2), Enterococcus (11) strains, was determined. Results showed that the obtained MICs were in the range (μg/ml) of 0.063-16 (CLI); 0.125-64 (CHL); 0.25-16 (ERY); 0.5-64 (GEN); 1-128 (STR) and 0.5-128 (TET); ≤ 0.032 (AMP) and ≤ 1024 (KAN), as shown in Table 3. Feedlot lactobacilli were found resistant to the glycopeptide VAN (data not shown), this phenotype being characterized as an intrinsic resistance in LAB (Gueimonde et al., 2013). Similarly, all strains were sensitive toward the β-lactam AMP in coincidence with that reported for probiotics L. acidophilus, L. rhamnosus, and L. casei, commercial starter L. plantarum and L. mucosae strains (Hummel et al., 2007; Klose et al., 2014); however resistance toward AMP was described for chicken lactobacilli (Dec et al., 2017). Although resistance to aminoglycosides has been often observed for probiotic and starter lactobacilli (Hummel et al., 2007; Nawaz et al., 2011; Abriouel et al., 2015), GEN, KAN, and STR sensitivity was repeatedly described in feedlot lactobacilli (>92%) (Table 3). Only Lactobacillus CRL2158 was resistant to GEN, L. plantarum CRL2103 exhibited resistance to KAN and L. acidophilus CRL2074, L. amylovorus CRL2065 as well as L. mucosae CRL2155 were resistant to STR. In coincidence, low lactobacilli resistance to aminoglycosides was reported for chicken intestinal LAB strains (Dec et al., 2017). In this study, the low MICs found for feedlot L. mucosae strains agrees with that described for wild boars fecal strains (Klose et al., 2014). In addition, resistance to STR of L. acidophilus from human origin and L. amylovorus from broilers were reported (Cauwerts et al., 2006; Klare et al., 2007). On the other hand, high prevalence of KAN resistance was described for L. acidophilus, L. rhamnosus, and L. casei from probiotic products (Temmerman et al., 2003). Resistance/sensitivity of L. plantarum to KAN were found to be controversial, strains isolated from probiotic products and fermented foods were reported as susceptible (Temmerman et al., 2003), while resistance was described by Nawaz et al. (2011). Discrepancies might be assigned to differences in the evaluated species, applied methods or strains source.
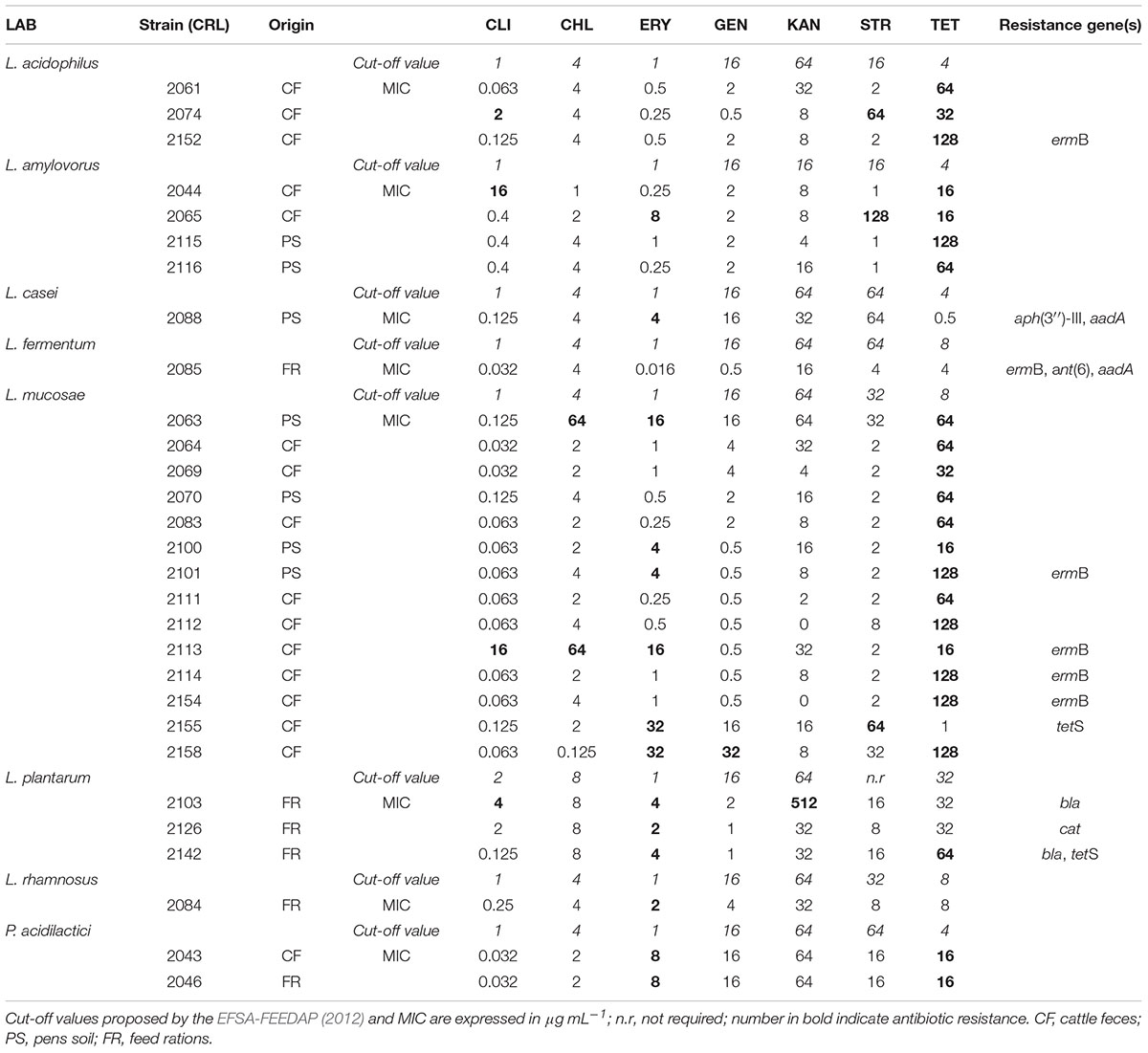
TABLE 3. Distribution of MICs and antibiotic resistance genes among lactobacilli and pediococci isolated from feedlot environment.
Generally, lactobacilli were sensitive to antibiotics inhibiting protein synthesis, such as CLI, CHL, ERY, and TET (Ammor et al., 2007; Klare et al., 2007). In agreement, high susceptibility (MICs below the cut-off value) to CLI and CHL was described among feedlot lactobacilli involving 85.2 and 92.6% of strains, respectively. Similar results were reported for lactobacilli isolated from chickens and wild boar feces (Klose et al., 2014; Dec et al., 2017), however, high prevalence of lincosamides (CLI) resistance was published for broilers cloacal lactobacilli (Cauwerts et al., 2006). With the exception of L. acidophilus and L. fermentum, all other lactobacilli (44.5%) showed to be resistant to ERY. These results are in line with those reported for human and animal L. rhamnosus, L. amylovorus, probiotic L. casei and meat starter L. plantarum strains (Cauwerts et al., 2006; Hummel et al., 2007; Gueimonde et al., 2013) while feedlot L. mucosae resistance (43%) toward ERY resulted higher to that reported for wild boars intestinal strains (Klose et al., 2014). Moreover, an unexpected high prevalence of TET resistance was observed among feedlot Lactobacillus (78% of the strains) with MICs values far beyond the cut-off value (128 μg/ml). L. acidophilus CRL2152, L. amylovorus CRL2115 and 4 strains of L. mucosae exhibited the highest TET resistance level in agreement with those reported for food and animal feces lactobacilli (Klose et al., 2014; Sornplang et al., 2016).
When feedlot pediococci were analyzed, resistance toward ERY and TET, while sensitivity to the other antimicrobials were obtained (Table 3). Susceptibility to AMP, CHL, GEN, and STR is in accordance to previous results (Danielsen et al., 2007; Hummel et al., 2007). P. acidilactici (two strains) resistances to ERY and TET agree to that described for food and animal strains (Ammor et al., 2007; Danielsen et al., 2007; Hummel et al., 2007) starter strains (Hummel et al., 2007), respectively. Nonetheless, as reported by Danielsen et al. (2007), pediococci are intrinsically resistant to TET in addition to VAN. On the other hand, enterococci as commensal inhabitants of the GIT of warm-blooded animals were dominant in feedlot environment (Maldonado et al., 2018). Since this genus emerged as important human and veterinary pathogen/opportunist, the incidence of antimicrobial resistance and virulence determinants were also investigated. Feedlot enterococci, mostly isolated form cattle feces, showed sensitivity to AMP, CHL, KAN and STR while resistance to CLI, ERY, GEN and TET (73, 100, 54.5, and 73%, respectively) was displayed (Table 4). Susceptibility of enterococci to AMP and CHL is in accordance to that previously reported (Anderson et al., 2008), and similar sensitivity to STR for enterococci isolated from feedlot steers was described by Beukers et al. (2015). In agreement, low incidence (<10%) of KAN and CHL resistance was reported for wild game Spanish meat enterococci (Guerrero-Ramos et al., 2016). Resistance to GEN found in 56% of Enterococcus agrees with that reported by Iseppi et al. (2015) for pet animal’s enterococci. The unexpected high percentage of feedlot enterococci resistant to CLI (82%), ERY (100%) and TET (73%) are concordant with that reported for dairy/bison cattle and pet feces (Anderson et al., 2008; Jackson et al., 2010; Iseppi et al., 2015; Beukers et al., 2015). Among the recovered enterococci from feedlot steers feces, E. hirae was revealed to predominate (Maldonado et al., 2018) and was also described among the highest antibiotic resistant enterococci species (Beukers et al., 2015). In addition, multi-resistance to at least three antimicrobial agents were found for 30% of feedlot strains in which CLI was mostly involved for enterococci strains (Table 4). Fifteen lactobacilli isolates (37.5%) were resistant to only one antibiotic, L. casei, L. plantarum, and L. rhamnosus strains showing ERY with MICs 1 ≥ μg/ml, while L. acidophilus, L. amylovorus, and L. mucosae exhibited TET MICs ≥ 4 μg/ml. Similarly, Klose et al. (2014) reported multi-resistance to CHL/KAN/STR/TET for L. mucosae strains isolated from wild boars feces. Specifically for enterococci, multidrug resistance patterns found are in agreement to that reported for E. hirae, E. faecium, and E. durans from dairy cows feces, E. hirae being resistant up to seven antimicrobials (Jackson et al., 2010). Nevertheless, L. fermentum CRL2085 from feedlot cattle was phenotypically sensitive to all assayed antibiotics in this study, in disagreement to that reported for fermented food and animal/human feces strains which were resistant to ERY and TET (Ammor et al., 2007; Nawaz et al., 2011; Sornplang et al., 2016).
Identification of Antibiotic Resistant Genes in Feedlot LAB Strains
Given the high prevalence of CLI, ERY, GEN and TET resistances found for enterococci, only lactobacilli sensitive strains were subjected to PCR amplification for the detection of resistance genes. Antibiotic sensitive LAB strains in which resistance genes have been detected are shown in Table 3. Seven of the 15 investigated genes were evidenced in feedlot lactobacilli. Although the presence of these genes were not always phenotypically correlated, molecular determinants for 27.5% of lactobacilli strains were found. While none of the feedlot strains was phenotypically resistant to AMP, PCR analysis showed L. plantarum CRL2103/CRL214 strains harboring bla genes. Similar results were reported for this lactobacilli species from swine and poultry meat, that even phenotypically negative, were found to carry blaZ gene (Aquilanti et al., 2007). In contrast, although phenotypically resistant, Hummel et al. (2007) observed a lack of molecular detection of bla gene for L. plantarum starter strains. In addition, none of the feedlot lactobacilli was found to host GEN resistance gene, although L. mucosae CRL2158 was phenotypically resistant. Nevertheless, the presence of the aac(6′)aph(2′′) gene encoding for GEN resistance in lactobacilli isolated from chicken, pigs, pet and wild boars feces, was reported (Ammor et al., 2007; Klose et al., 2014; Dec et al., 2017). Although phenotipically sensitive, the aph(3′′)-III gene conferring KAN resistance was present in L. casei CRL2088 in coincidence to that described for probiotic strain by Ouoba et al. (2008). Conversely, even when L. plantarum CRL2103 exhibited high phenotypic resistance (MIC ≥ 512 μg/ml), KAN resistance gene was absent. Likewise, from the genomic DNA of L. mucosae CRL2063/CRL2113 with a resistant phenotype to CHL, cat gene could not be amplified. Similarly, the occurrence of this gene was reported in L. plantarum CRL2126 with MIC ≥ 8 μg/ml (cut-off value) in coincidence with that reported for strains isolated from probiotic products (Temmerman et al., 2003). When STR resistance genes, aadA and ant(6) were evaluated, their presence in L. casei CRL2088 and L. fermentum CRL2085 strains were detected, MICs values were ≤ to cut-off value. The aadA gene was present in both lactobacilli strains, whereas ant(6) gene only occurred in the STR sensitive L. fermentum strain (MIC ≥ 4 μg/ml). Although phenotypic sensitivity to all assayed antibiotics, L. fermentum CRL2085 exhibited the co-occurrence of both STR resistance genes. Positive PCR for aadA gene in L. casei CRL2018 obtained in this study agrees with that reported for food and human strains, but none of the phenotypically resistant or sensitive food L. fermentum strains were positive for the investigated STR resistance genes (Ouoba et al., 2008). Resistance to aminoglycosides may result from various mechanisms, such as the lack of cytochrome electron transport responsible for antibiotic uptake, changes in cellular permeability and enzymatic antibiotic modification by acetyl-, adenyl-, and phospho-transferases, whose encoding genes are mostly found on plasmids and transposons (Abriouel et al., 2015).
Furthermore, a prevalence of erm and tet genes among feedlot lactobacilli was found (Table 3). ERY resistance genes were identified as ermB, while TET resistance genes belonged to the tet(S) class. Six of 27 feedlot lactobacilli harbored ermB gene; L. mucosae CRL2101/CRL2113/CRL2114/CRL2154 phenotypically resistant to ERY (MICs ≥ 1 μg/ml) as well as L. acidophilus CRL2152 and L. fermentum CRL2085 susceptible to ERY (MICs ≤ 0.5 μg/ml) displayed positive PCR for ermB gene. In contrast to these results, L. mucosae strains from wild boars feces did not harbor ERY resistant genes (Klose et al., 2014). The detection of ermB as a major resistant gene for this class of antibiotic in bovine cattle LAB is consistent with that previously reported for lactobacilli from various sources (Ammor et al., 2007; Hummel et al., 2007; Klare et al., 2007; Anderson et al., 2008; Nawaz et al., 2011). When TET resistance genes was analyzed, despite its high phenotypic prevalence with MICs far beyond the break point, a low occurrence of tetS gene was detected. This gene conferring resistance to TET was only present in the phenotypically resistant L. plantarum CRL2142, this being in coincidence with that found from food and human strains (Ammor et al., 2007; Zonenschain et al., 2009; Nawaz et al., 2011), whereas L. mucosae CRL2155 with a sensitive phenotype (MIC = 1 μg/ml), was PCR positive for tetS gene. Similarly, Klose et al. (2014) reported sensitive L. mucosae isolates from wild boars as harboring tetS gene. Resistance tetS and ermB genes were identified on both, plasmids and the chromosome for Lactobacillus species from different fermented foods (Nawaz et al., 2011; Abriouel et al., 2015). The high level of resistance to ERY and TET in lactobacilli from feedlot environment is in agreement with the use of these antibiotics in veterinary therapy and for growth promotion in domestic and meat animals (Anderson et al., 2008). Of the resistant lactobacilli and pediococci, eleven strains (28%) carried resistance genes, which was higher than that reported for LAB from dairy, pharmaceutical and probiotic products, in which only 12% of strains were PCR positive. From strains carrying resistance genes, only three correlated with phenotypic results (L. plantarum CRL2142 and L. mucosae CRL2101/2113 for TET and ERY, respectively). As recently reported by Hughes and Andersson (2017), the lack of correlation between phenotype and genotype may be explained by the intrinsic resistance to the tested antibiotics and the resistance emergence through evolutionary events such as mutations or defective expression of the resistance gene due to environmental and genetic modulation of the phenotypic expression of AR.
Identification of Virulence Factors
Enterococci and lactobacilli are commensal bacteria of the human and bovine GIT, but are also associated with clinical and community-acquired infections in humans (Franz et al., 2011). Genes encoding virulence factors were studied in feedlot LAB strains and results are shown in Table 4. When enzymatic activities were examined, neither gelatinase nor β-hemolytic activities were exhibited by the analyzed LAB strains; all enterococci showed α-hemolysis, while lactobacilli (four L. mucosae strains) were also α-hemolytic, the remaining feedlot strains (85%) were γ-hemolytic or non-hemolytic (data not shown). In coincidence, none of the enterococci from pet animal’s feces, food and water were β-hemolytic (Abriouel et al., 2008; Iseppi et al., 2015) although gelatinase activity was described for dairy and pet feces enterococci (Lopez et al., 2006; Iseppi et al., 2015). In addition, the absence of β-hemolysis in feedlot enterococci that correlated with the lack of amplification of cylA gene is in line with that reported for environmental enterococci (Pangallo et al., 2008). None of the feedlot lactobacilli harbored virulence factors genes (data not shown); these are generally regarded as safe due to their long history of presence in the normal GIT of humans and animals and safe use in fermented foods. However, lactobacilli have been associated with several cases of infections such as bacteremia, endocarditis but also with localized infections, L. casei and L. rhamnosus being common causative agents (Lara-Villoslada et al., 2010).
The frequency of genes encoding virulence factors among the feedlot enterococci strains is shown in Table 4. Positive PCR amplification for accessory colonization factor (ace), aggregation substance (agg), quorum sensing (fsrA) and ATP synthase subunit alpha (atpA) genes were found for E. durans and E. hirae strains. In agreement with this result, the absence of genes coding for virulence factors in E. faecium from sheep feces was reported (Mannu et al., 2003). In contrast, positive PCR for the other genes here evaluated was described for E. faecium from food, clinical and pet feces samples (Abriouel et al., 2008; Iseppi et al., 2015). The presence of ace gene was detected in feedlot E. durans and E. hirae strains, while agg gene was found in 2 out of 3 E. durans strains, fsrA and atpA genes being also PCR positive for E. hirae feedlot strains. On the contrary, a lack of amplification of the virulence genes here assayed was reported for E. durans and E. hirae from fermented sausages and pet feces (Martin et al., 2005; Fontana et al., 2009; Iseppi et al., 2015). In particular, fsrA gene coding for quorum sensing regulatory mechanism was present in feedlot E. hirae CRL2068 in coincidence with that recently described for dairy strains by Popović et al. (2018). The lack of amplification of fsrA gene in E. durans feedlot strains agrees with that reported for this species by Golińska et al. (2013). In addition, atpA gene encoding for the alpha subunit of ATP synthase was present in feedlot E. hirae CRL2089 in correlation with the use of this virulence determinant as identification probe for poultry E. hirae strains (Champagne et al., 2011). Nevertheless, the absence of gelE gene in feedlot enterococci, in coincidence with the lack of gelatinase activity, agrees with that reported by Diarra et al. (2010) for Enterococcus species isolated from broilers chicken. Although the low incidence of virulence genes among feedlot enterococci, positive PCR genes were related to adhesion, colonization, biofilm formation and energy metabolism which may facilitate gene transfer in the GIT of meat animals and problematic pathogen lineages might arise.
Optimization of Growth Conditions for Selected Feedlot Probiotic LAB. Strains Compatibility
Preliminary experiments to optimize the large-scale production required to deliver high numbers of probiotic live bacteria to feedlot cattle were performed; large-scale and low cost production of these bacteria is becoming an important issue. Therefore, the ability to produce a large number of cells, growth parameters and the use of low cost media ingredients should be considered for growth medium optimization. The selection of feedlot strains to be applied as probiotics previously carried out (Maldonado et al., 2018) together with safety traits (this study) allows the selection of L. acidophilus CRL2074, L. amylovorus CRL2116, L. mucosae CRL2069 and L. rhamnosus CRL2084 for a preliminary screening of optimal culture conditions to produce high cell mass. For this purpose, five different media involving several nitrogen (skim milk, soluble protein concentrate from whey, peptone casein, yeast extract) and carbon sources (glucose, lactose) were assayed, their composition being shown in Table 2. Results showed a high dependence of lactobacilli growth on the composition of the different evaluated culture media (Figure 1 and Table 5). Different kinetics were displayed both measuring OD and CFU/mL counts. When OD560 max was determined in clear media (Mpep, Mgl, MRSc and MRS), L. acidophilus, L. amylovorus and L. mucosae were not able to grow in Mpep medium containing peptone, while L. rhamnosus exhibited a slight growth (Figure 1). A better growth was found for all four lactobacilli in Mgl medium (containing glucose) and higher OD560max were exhibited by L. mucosae and L. rhamnosus at 24 h. Nevertheless, maximal OD values were also observed for lactobacilli when MRSc was used; MRS (free pH) values were somewhat lower. When growth parameters were calculated from counts (CFU/mL) obtained by plate-dilution method, lactobacilli yielded the highest growth (>109 CFU/mL) when inoculated in Mgl, Mlac, MRS, and MRSc media; lower growth was obtained in Mw and Msm media while mostly poor growth was produced in Mpep medium. Highest cell numbers were reached in Mgl (L. acidophilus and L. mucosae), Mlac (L. acidophilus, L. amylovorus, and L. rhamnosus) and MRS (L. acidophilus and L. mucosae). As reported by Manzoor et al. (2017), even when MRS medium represents a rich and suitable condition to support optimal lactobacilli growth, its high formulation cost and potential environmental hazards make it unviable for large-scale commercial applications. From our results, Mlac (g/l: skim milk, 10; yeast extract, 10; lactose, 30 pH: 6.5) and Mgl (g/l: yeast extract, 20; glucose, 10 pH: 5.9) showed the best conditions for the semi-industrial production of selected feedlot probiotic lactobacilli (Table 5). The presence of sodium acetate in Mgl medium, a component of commercial MRS medium, was reported as energy source and selective agent for lactobacilli (Stiles et al., 2002). On the other hand, whey protein concentrate (protein, 78%; carbohydrates, 4.5%) medium (Mw) as well as skim milk containing medium (Msm), a nitrogen (casein, ∼35%) and carbon (lactose, ∼50%) source respectively, were not able to produce high lactobacilli biomass. This result disagrees with economic and growth advantages of skim milk-based media used for LAB biomass production (Kusnadi and Afriyan, 2012). In coincidence to that found by measuring OD (Figure 1), the lowest biomass production by the selected lactobacilli was obtained in peptone casein containing medium (Mpep), L. acidophilus, L. amylovorus, and L. rhamnosus displaying the lower CFU/mL max values (Table 5). Particularly for L. acidophilus, Olson and Aryana (2012) reported a growth decrease in the presence of peptone compared with skim milk in coincidence with results from this study. Modified media composed by yeast extract, glucose and sodium acetate/sodium glutamate as major ingredients omitting peptone (expensive nitrogen source) were used for biomass production by fecal L. plantarum strains intended to be used as probiotic (Hwang et al., 2011). Optimized media containing agro-industrial residues such as cheese whey, industrial yeast extract, corn steep liquor, soybean meal and molasses among others, were assayed for lactobacilli biomass production (Hwang et al., 2011; Chiang et al., 2015; Manzoor et al., 2017). In view to be used as probiotic mixture, a final Lactobacillus strains compatibility was carried out. Results indicated that there was not inhibition of one strain on the growth of another.
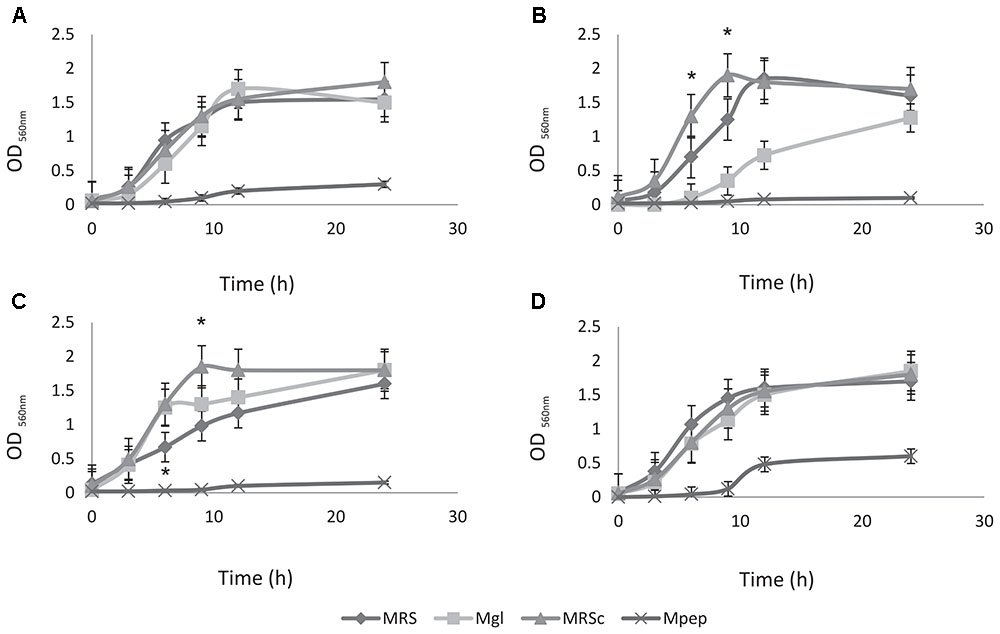
FIGURE 1. Growth kinetics of probiotic lactobacilli strains in different culture media. (A) Lactobacillus acidophilus CRL 2074, (B) L. amylovorus CRL 2116, (C) L. mucosae CRL 2069, and (D) L. rhamnosus CRL 2084.
Conclusion
From this study, LAB isolated from steers feces, soil pens and feed rations were found as a reservoir of AR and virulence genes. However, L. acidophilus CRL2074, L. amylovorus CRL2116, L. mucosae CRL2069 and L. rhamnosus CRL2084 were able to be selected as probiotic candidates being free of AR and virulence factors, reaching high cell numbers in optimal culture media and compatible among them. These strains, alone or in combination, are being administered to feedlot steers for in vivo studies to elucidate their health and productivity benefits.
Author Contributions
FM and NM performed the laboratory phenotypic antibiotic test. CA carried out molecular work for antibiotic and virulence genes investigation. HM was responsible for culture conditions optimization. MN-M and GV organized experimental protocols, analyzed the data, discussed and wrote the manuscript. All the authors reviewed the manuscript.
Funding
This work was supported by grants from CONICET (PIP 545 and 774) and ANPCyT (PICT 1187) as well as by a PDTS and CONICET-Molino Trigotuc S.A. agreement.
Conflict of Interest Statement
The authors declare that the research was conducted in the absence of any commercial or financial relationships that could be construed as a potential conflict of interest.
References
Aarestrup, F. N., Agerso, Y., Gerner-Smidt, P., Madsen, M., and Jensen, L. B. (2000). Comparison of antimicrobial resistance phenotypes and resistance genes in Enterococcus faecalis and Enterococcus faecium from humans in the community, broilers, and pigs in Denmark. Diagn. Microbiol. Infect. Dis. 37, 127–137.
Abriouel, H., Ben Omar, N., Cobo Molinos, A., Lucas López, R., Grande, M. J., Martínez-Viedma, P., et al. (2008). Comparative analysis of genetic diversity and incidence of virulence factors and antibiotic resistance among enterococcal populations from raw fruit and vegetable foods, water and soil, and clinical samples. Int. J. Food Microbiol. 123, 38–49. doi: 10.1016/j.ijfoodmicro.2007.11.067
Abriouel, H., Casado Muñoz, M. C., Lavilla Lerma, L., Pérez Montoro, B., Bockelmann, W., Pichner, R., et al. (2015). New insights in antibiotic resistance of Lactobacillus species from fermented foods. Food Res. Int. 78, 465–481. doi: 10.1016/j.foodres.2015.09.016
Al-Talib, H., Zuraina, N., Kamarudin, B., and Yean, C. Y. (2015). Genotypic variations of virulent genes in Enterococcus faecium and Enterococcus faecalis isolated from three hospitals in Malaysia. Adv. Clin. Exp. Med. 24, 121–127. doi: 10.17219/acem/38162
Ammor, M. S., Flórez, A. B., and Mayo, B. (2007). Antibiotic resistance in non-enterococcal lactic acid bacteria and bifidobacteria. Food Microbiol. 24, 559–570. doi: 10.1016/j.fm.2006.11.001
Anderson, J. F., Parrish, T. D., Akhtar, M., Zurek, L., and Hirt, H. (2008). Antibiotic resistance of Enterococci in American Bison (Bison bison) from a nature preserve compared to that of Enterococci in pastured cattle. Appl. Environ. Microbiol. 74, 1726–1730. doi: 10.1128/AEM.02164-07
Aquilanti, L., Garofalo, C., Osimani, A., Silvestri, G., Vignaroli, C., and Clementi, F. (2007). Isolation and molecular characterization of antibiotic-resistant Lactic Acid Bacteria from poultry and swine meat products. J. Food Prot. 70, 557–565.
Beukers, A. G., Zaheer, R., Cook, S. R., Stanford, K., Chaves, A. V., Ward, M. P., et al. (2015). Effect of in-feed administration and withdrawal of tylosin phosphate on antibiotic resistance in enterococci isolated from feedlot steers. Front. Microbiol. 6:483. doi: 10.3389/fmicb.2015.00483
Cantas, L., Shah, S. Q. A., Cavaco, L. M., Manaia, C. M., Walsh, F., Popowska, M., et al. (2013). A brief multi-disciplinary review on antimicrobial resistance in medicine and its linkage to the global environmental microbiota. Front. Microbiol. 4:96. doi: 10.3389/fmicb.2013.00096
Cauwerts, K., Pasmans, F., Devriese, L. A., Martel, A., Haesebrouck, F., and Decostere, A. (2006). Cloacal Lactobacillus isolates from broilers show high prevalence of resistance towards macrolide and lincosamide antibiotics. Avian Pathol. 35, 160–164. doi: 10.1080/03079450600598137
Champagne, J., Diarra, M. S., Rempel, H., Topp, E., Greer, C. W., Harel, J., et al. (2011). Development of a DNA microarray for Enterococcal species, virulence, and antibiotic resistance gene determinations among isolates from poultry. Appl. Environ. Microbiol. 77, 2625–2633. doi: 10.1128/AEM.00263-11
Chaucheyras-Durand, F., and Durand, H. (2010). Probiotics in animal nutrition and health. Benef. Microbes 1, 3–9. doi: 10.3920/BM2008.1002
Chiang, M.-L., Chen, H.-C., Chen, K.-N., Lin, Y.-C., Lin, Y.-T., and Chen, M.-J. (2015). Optimizing production of two potential probiotic Lactobacilli strains Isolated from piglet feces as feed additives for weaned piglets. Asian Australas. J. Anim. Sci. 28, 1163–1170. doi: 10.5713/ajas.14.0780
Clark, N. C., Olsvik, O., Swenson, J. M., Spiegel, C. A., and Tenover, F. C. (1999). Detection of a streptomycin/spectinomycin adenylyltransferase gene (aadA) in Enterococcus faecalis. Antimicrob. Agents Chemother. 43, 157–160.
Danielsen, M., Simpson, P. J., O’Connor, E. B., Ross, R. P., and Stantonet, C. (2007). Susceptibility of Pediococcus spp. to antimicrobial agents. J. Appl. Microbiol. 102, 384–389. doi: 10.1111/j.1365-2672.2006.03097.x
Dec, M., Urban-Chmiel, R., Stêpień-Pyśniak, D., and Wernicki, A. (2017). Assessment of antibiotic susceptibility in Lactobacillus isolates from chickens. Gut Pathog. 9, 54–69. doi: 10.1186/s13099-017-0203-z
Devirgiliis, C., Zinno, P., and Perozzi, G. (2013). Update on antibiotic resistance in foodborne Lactobacillus Lactococcus species. Front. Microbiol. 4:301. doi: 10.3389/fmicb.2013.00301
Diarra, M. S., Rempel, H., Champagne, J., Masson, L., Pritchard, J., and Topp, E. (2010). Distribution of antimicrobial resistance and virulence genes in Enterococcus spp. and characterization of isolates from broiler chickens. Appl. Environ. Microbiol. 76, 8033–8043. doi: 10.1128/AEM.01545-10
EFSA-FEEDAP (2012). Guidance on the assessment of bacterial susceptibility to antimicrobials of human and veterinary importance. EFSA J. 10, 2740–2749. doi: 10.2903/j.efsa.2012.2740
Espeche, M. C., Pellegrino, M., Frola, I., Larriestra, A., Bogni, C., and Nader-Macías, M. E. F. (2012). Lactic acid bacteria from raw milk as potentially beneficial strains to prevent bovine mastitis. Anaerobe 18, 103–109. doi: 10.1016/j.anaerobe.2012.01.002
FAO. (2002). Guidelines for the Evaluation of Probiotics in Food. Available at: http://www.who.int/foodsafety/fs_management/en/probiotic_guidelines.pdf
Fontana, C., Gazzola, S., Cocconcelli, P. S., and Vignolo, G. (2009). Population structure and safety aspects of Enterococcus strains isolated from artisanal dry fermented sausages produced in Argentina. Lett. Appl. Microbiol. 49, 411–414. doi: 10.1111/j.1472-765X.2009.02675.x
Franz, C. M., Huch, M., Abriouel, H., Holzapfel, W., and Gálvez, A. (2011). Enterococci as probiotics and their implications in food safety. Int. J. Food Microbiol. 151, 125–140. doi: 10.1016/j.ijfoodmicro.2011.08.014
Garcia-Migura, L., Hendriksen, R. S., Fraile, L., and Aarestrup, F. M. (2014). Antimicrobial resistance of zoonotic and commensal bacteria in Europe: the missing link between consumption and resistance in veterinary medicine. Vet. Microbiol. 170, 1–9. doi: 10.1016/j.vetmic.2014.01.013
Golińska, E., Tomusiak, A., Gosiewski, T., Więcek, G., Machul, A., Mikołajczyk, D., et al. (2013). Virulence factors of Enterococcus strains isolated from patients with inflammatory bowel disease. World J. Gastroenterol. 19, 3562–3572. doi: 10.3748/wjg.v19.i23.3562
Gueimonde, M., Sánchez, B., de los Reyes-Gavilán, C. G., and Margolles, A. (2013). Antibiotic resistance in probiotic bacteria. Front. Microbiol. 4:202. doi: 10.3389/fmicb.2013.00202
Guerrero-Ramos, E., Cordero, J., Molina-González, D., Poeta, P., Igrejas, G., Alonso-Calleja, C., et al. (2016). Antimicrobial resistance and virulence genes in enterococci from wild game meat in Spain. Food Microbiol. 53, 156–164. doi: 10.1016/j.fm.2015.09.007
Gullberg, E., Cao, S., Berg, O. G., Ilbäck, C., Sandegren, L., Hughes, D., et al. (2011). Selection of resistant bacteria at very low antibiotic concentrations. PLoS Pathog. 7:e1002158. doi: 10.1371/journal.ppat.1002158
Hill, C., Guarner, F., Reid, G., Gibson, G. R., Merenstein, D. J., Pot, B., et al. (2014). The international scientific association for probiotics and prebiotics consensus statement on the scope and appropriate use of the term probiotic. Nat. Rev. Gastroenterol. Hepatol. 11, 506–514. doi: 10.1038/nrgastro.2014.66
Hughes, D., and Andersson, D. I. (2017). Environmental and genetic modulation of the phenotypic expression of antibiotic resistance. FEMS Microbiol. Rev. 41, 374–391. doi: 10.1093/femsre/fux004
Hummel, A., Hertel, C., Holzapfel, W. H., and Franz, C. M. A. P. (2007). Antibiotic resistances of starter and probiotic strains of Lactic Acid Bacteria. Appl. Environ. Microbiol. 73, 730–739. doi: 10.1128/AEM.02105-06
Hwang, C.-F., Chen, J.-N., Huang, Y.-T., and Mao, Z.-Y. (2011). Biomass production of Lactobacillus plantarum LP02 isolated from infant feces with potential cholesterol lowering ability. Afr. J. Biotechnol. 10, 7010–7020. doi: 10.5897/AJB11.507
Imperial, I. C. V. J., and Ibana, J. A. (2016). Addressing the antibiotic resistance problem with probiotics: reducing the risk of its double-edged sword effect. Front. Microbiol. 7:1983. doi: 10.3389/fmicb.2016.01983
Iseppi, R., Messi, P., Anacarso, I., Bondi, M., Sabia, C., Condò, C., et al. (2015). Antimicrobial resistance and virulence traits in Enterococcus strains isolated from dogs and cats. New Microbiol. 38, 369–378.
Jackson, C. R., Lombard, J. E., Dargatz, D. A., and Fedorka-Cray, P. J. (2010). Prevalence, species distribution and antimicrobial resistance of enterococci isolated from US dairy cattle. Lett. Appl. Microbiol. 52, 41–48. doi: 10.1111/j.1472-765X.2010.02964.x
Klare, I., Konstabel, C., Werner, G., Huys, G., Vankerckhoven, V., Kahlmeter, G., et al. (2007). Antimicrobial susceptibilities of Lactobacillus, Pediococcus and Lactococcus human isolates and cultures intended for probiotic or nutritional use. J. Antimicrob. Chemother. 59, 900–912. doi: 10.1093/jac/dkm035
Klose, V., Bayer, K., Kern, C., Goelß, F., Fibi, S., and Wegl, G. (2014). Antibiotic resistances of intestinal lactobacilli isolated from wild boars. Vet. Microbiol. 168, 240–244. doi: 10.1016/j.vetmic.2013.11.014
Kusnadi, J., and Afriyan, T. (2012). “The growth of probiotic bacteria Lactobacillus plantarum and Lactobacillus acidophilus in skim milk and Taro (Colocasia esculenta L. Schott Var. Boring) flour composite medium,” in International Conference on Environmental and Biological Sciences 2012, Bangkok.
Lara-Villoslada, F., Olivares, M., and Xaus, J. (2010). “Safety of probiotic bacteria,” in Bioactive Foods in Promoting Health: Probiotics and Prebiotics, ed. V. R. Preedy (Cambridge, MA: Academic Press), 47–58. doi: 10.1016/B978-0-12-374938-3.00004-9
Lopez, M. F. S., Simões, A. P., Tenreiro, R., Figueiredo Marques, J. J., and Barreto Crespo, M. T. (2006). Activity and expression of a virulence factor, gelatinase, in dairy enterococci. Int. J. Food Microbiol. 112, 208–214. doi: 10.1016/j.ijfoodmicro.2006.09.004
Maldonado, N., Aristimuño Ficoseco, C., Mansilla, F. I., Melián, C., Hébert, E. M., Vignolo, G., et al. (2018). Identification, characterization and selection of autochthonous lactic acid bacteria as probiotics for feedlot cattle. Livestock Sci. 212, 99–110. doi: 10.1016/j.livsci.2018.04.003
Mannu, L., Paba, A., Daga, E., Comunian, R., Zanetti, S., Dupré, I., et al. (2003). Comparison of the incidence of virulence determinants and antibiotic resistance between Enterococcus faecium strains of dairy, animal and clinical origin. Int. J. Food Microbiol. 88, 291–304.
Manzoor, A., Qazi, J. I., ul Haq, I., Mukhtar, H., and Rasool, A. (2017). Significantly enhanced biomass production of a novel bio-therapeutic strain Lactobacillus plantarum (AS-14) by developing low cost media cultivation strategy. J. Biol. Eng. 11, 17–26. doi: 10.1186/s13036-017-0059-2
Maron, D. F., Smith, T. J. S., and Nachman, K. E. (2013). Restrictions on antimicrobial use in food animal production: an international regulatory and economic survey. Glob. Health 9, 48–58. doi: 10.1186/1744-8603-9-48
Martin, B., Garriga, M., Hugas, M., and Aymerich, T. (2005). Genetic diversity and safety aspects of enterococci from slightly fermented sausages. J. Appl. Microbiol. 98, 1177–1190. doi: 10.1111/j.1365-2672.2005.02555.x
Nawaz, M., Wang, J., Zhou, A., Ma, C., Wu, X., Moore, J.-E., et al. (2011). Characterization and transfer of antibiotic resistance in lactic acid bacteria from fermented food products. Curr. Microbiol. 62, 1081–1089. doi: 10.1007/s00284-010-9856-2
Ng, L. K., Martin, I., Alfa, M., and Mulvey, M. (2001). Multiplex PCR for the detection of tetracycline resistant genes. Mol. Cell. Probes 15, 209–215. doi: 10.1006/mcpr.2001.0363
Olson, D. W., and Aryana, K. J. (2012). Effect of prebiotics on Lactobacillus acidophilus growth and resulting pH changes in skim milk and a model peptone system. J. Microb. Biochem. Technol. 4, 121–125. doi: 10.4172/1948-5948.1000081
Ouoba, L. I. I., Lei, V., and Jensen, L. B. (2008). Resistance of potential probiotic lactic acid bacteria and bifidobacteria of African and European origin to antimicrobials: determination and transferability of the resistance genes to other bacteria. Int. J. Food Microbiol. 121, 217–224. doi: 10.1016/j.ijfoodmicro.2007.11.018
Pangallo, D., Drahovská, H., Harichová, J., Karelová, E., Chovanová, K., Ferianc, P., et al. (2008). Assessment of environmental enterococci: bacterial antagonism, pathogenic capacity and antibiotic resistance. Antonie Van Leeuwenhoek 94, 555–562. doi: 10.1007/s10482-008-9272-9
Popović, N., Dinić, M., Tolinač, M., Mihajlović, S., Terzić-Vidojević, A., Bojić, J., et al. (2018). New insight into biofilm formation ability, the presence of virulence genes and probiotic potential of Enterococcus sp. dairy isolates. Front. Microbiol. 9:78. doi: 10.3389/fmicb.2018.00078
Pospiech, A., and Neumann, B. (1995). A versatile quick-prep of genomic DNA from gram-positive bacteria. Trends Genet. 11, 217–218. doi: 10.1016/S0168-9525(00)89052-6
Qin, Y., Luo, Z. Q., Smyth, A. J., Gao, P., Beck von Bodman, S., and Farrand, S. K. (2000). Quorum-sensing signal binding results in dimerization of TraR and its release from membranes into the cytoplasm. EMBO J. 19, 5212–5221. doi: 10.1093/emboj/19.19.5212
Retta, K. S. (2016). Role of probiotics in rumen fermentation and animal performance: a review. Int. J. Livestock Prod. 7, 24–32. doi: 10.5897/IJLP2016.0285
Šeme, H., Gjuračić, K., Kos, B., Fujs, Š,Štempelj, M., Petković, H., et al. (2015). Acid resistance and response to pH-induced stress in two Lactobacillus plantarum strains with probiotic potential. Benef. Microbes 6, 369–379. doi: 10.3920/BM2014.0069
Sillanpää, J., Chang, C., Singh, K. V., Montealegre, M. C., Nallapareddy, S. R., Harvey, B. R., et al. (2013). Contribution of individual Ebp pilus subunits of Enterococcus faecalis OG1RF to pilus biogenesis, biofilm formation and urinary tract infection. PLoS One 8:e68813. doi: 10.1371/journal.pone.0068813
Sornplang, P., Sakulsawasdiphan, K., Piyadeatsoontorn, S., and Surasorn, B. (2016). Antimicrobial susceptibility of lactic acid bacteria isolated from human and food-producing animal feces in Khon Kaen Province. Thailand. Trop. Anim. Health Prod. 48, 1739–1745. doi: 10.1007/s11250-016-1116-4
Stiles, J. S., Penkar, Plocková, M., Chumchalová, J., and Bullerman, L. B. (2002). Antifungal activity of sodium acetate and Lactobacillus rhamnosus. J. Food Prot. 65, 1188–1191. doi: 10.4315/0362-028X-65.7.1188
Temmerman, R., Pot, B., Huys, G., and Swingset, J. (2003). Identification and antibiotic susceptibility of bacterial isolates from probiotic products. Int. J. Food Microbiol. 81, 1–10. doi: 10.1016/S0168-1605(02)00162-9
van Reenen, C. A., and Dicks, L. N. (2011). Horizontal gene transfer amongst probiotic lactic acid bacteria and other intestinal microbiota: what are the possibilities? A review. Arch. Microbiol. 193, 157–168. doi: 10.1007/s00203-010-0668-3
Vankerckhoven, V., Van Autgaerden, T., Vael, C., Lammens, C., Chapelle, S., Rossi, R., et al. (2004). Development of a Multiplex PCR for the detection of asa1, gelE, cylA, esp, and hyl genes in Enterococci and survey for virulence determinants among European hospital isolates of Enterococcus faecium. J. Clin. Microbiol. 42, 4473–4479. doi: 10.1016/j.tifs.2007.07.013
Keywords: lactic acid bacteria, antibiotic resistance, virulence determinants, growth conditions, feedlot cattle
Citation: Aristimuño Ficoseco C, Mansilla FI, Maldonado NC, Miranda H, Nader-Macias MEF and Vignolo GM (2018) Safety and Growth Optimization of Lactic Acid Bacteria Isolated From Feedlot Cattle for Probiotic Formula Design. Front. Microbiol. 9:2220. doi: 10.3389/fmicb.2018.02220
Received: 09 May 2018; Accepted: 30 August 2018;
Published: 28 September 2018.
Edited by:
Charles W. Knapp, University of Strathclyde, United KingdomReviewed by:
Patricia Burns, CONICET Instituto de Lactología Industrial (INLAIN), ArgentinaLucilla Iacumin, Università degli Studi di Udine, Italy
Copyright © 2018 Aristimuño Ficoseco, Mansilla, Maldonado, Miranda, Nader-Macias and Vignolo. This is an open-access article distributed under the terms of the Creative Commons Attribution License (CC BY). The use, distribution or reproduction in other forums is permitted, provided the original author(s) and the copyright owner(s) are credited and that the original publication in this journal is cited, in accordance with accepted academic practice. No use, distribution or reproduction is permitted which does not comply with these terms.
*Correspondence: María E. Fátima Nader-Macias, Zm5hZGVyQGNlcmVsYS5vcmcuYXI=