- GEOMAR Helmholtz Centre for Ocean Research Kiel, Kiel, Germany
Fixed nitrogen (N) limits productivity across much of the low-latitude ocean. The magnitude of its inventory results from the balance of N input and N loss, the latter largely occurring in regionally well-defined low-oxygen waters and sediments (denitrification and anammox). The rate and distribution of N input by biotic N2 fixation, the dominant N source, is not well known. Here we compile N2 fixation estimates from experimental measurements, tracer-based geochemical and modeling approaches, and discuss their limitations and uncertainties. The lack of adequate experimental data coverage and the insufficient understanding of the controls of marine N2 fixation result in high uncertainties, which make the assessment of the current N-balance a challenge. We suggest that a more comprehensive understanding of the environmental and ecological interaction of marine N2 fixers is required to advance the field toward robust N2 fixation rates estimates and predictions.
Introduction
Marine N2 fixation, the largest source of fixed N to the ocean, maintains ocean fertility by compensating for N-losses via denitrification. Global warming, likely inducing ocean deoxygenation (Keeling et al., 2010), and increasing N load from the atmosphere and/or rivers, are perturbing the N cycle leading to increasing N loss via denitrification on the one hand (Codispoti et al., 2001; Codispoti, 2007) and to alterations of the niche of marine N2 fixers on the other (Landolfi et al., 2017), with an unknown net effect on the N inventory. A prolonged mismatch between N inputs and losses would cause changes to the oceanic fixed N inventory, potentially affecting ocean productivity and ocean carbon (C) storage. Narrowing down the uncertainties in current N2 fixation estimates is key to diagnosing any imbalance in the marine N budget and its effect on the marine C budget.
N2 fixation can be inferred experimentally from incubation assays (Capone, 1993; Montoya et al., 1996), and from its geochemical imprint on nutrient (Gruber and Sarmiento, 1997) and stable N isotope distributions (Altabet, 2007). Geochemical estimates use the integrated N2 fixation signature over large scales of space and time, smoothing any small-scale variability inherent in experimental N2 fixation measurements. Traditionally, geochemical (Gruber, 2004) and experimental (Capone et al., 2005) estimates suggested highest N2 fixation rates in the Tropical North Atlantic, remote from the region of largest pelagic N-loss in the Eastern Tropical Pacific. Although such spatial separation was difficult to reconcile with paleo-oceanographic evidence of a closely balanced N-cycle, considered to require tight stabilizing feedbacks between N-loss and N2 fixation (Altabet, 2007), it was in line with the well-documented high energy and iron requirements of diazotrophs (Kustka et al., 2003) that would restrict them to warm and iron-rich waters. This view has been challenged by a model-based geochemical N2 fixation estimate suggesting prevailing control by N deficits and close spatial coupling of N2 fixation and N loss (Deutsch et al., 2007), and by new evidence from molecular techniques demonstrating a wider diversity of N2 fixers and N2 fixation strategies. This includes free-living unicellular cyanobacteria (Zehr et al., 1998; Montoya et al., 2004), cyanobacterial symbionts and heterotrophic phylotypes (see Foster et al., 2011; Bombar et al., 2016; Caputo et al., 2018), covering novel habitats such as aphotic waters (Bonnet et al., 2013; Benavides et al., 2015, 2016, 2018) and oxygen deficient zones (ODZs) (Fernandez et al., 2011; Jayakumar et al., 2012; Bonnet et al., 2013; Löscher et al., 2014, 2016; Turk-Kubo et al., 2014; Knapp et al., 2016). Recently, severe problems in applying the N2 fixation assays came to light (Mohr et al., 2010). Considering this new evidence, using a constant correction factor of historical data, a doubling of N2 fixation has been suggested (177 ± 8 Tg N y−1, Großkopf et al., 2012). Despite the pace of new discoveries, global N2 fixation estimates remain highly uncertain (Gruber, 2016). The incomplete knowledge of the organisms involved and their physiological and ecological controls prevent any robust prediction of their distribution and activity. Here we present a brief overview of recent work covering experimental, geochemical, and model-based N2 fixation estimates and discuss knowledge gaps. We suggest future research strategies to reduce the current uncertainty.
Experimental Estimates
The compilation of surface marine N2 fixation rate measurements (MARine Ecosystem DATa) by Luo et al. (2012), with more recent data from the North Pacific (Shiozaki et al., 2015a,b, 2017), western Pacific (Bonnet et al., 2009, 2015, 2017, 2018; Shiozaki et al., 2013, 2014b; Berthelot et al., 2017), eastern tropical South Pacific (Löscher et al., 2014, 2016; Knapp et al., 2016), Indian Ocean (Shiozaki et al., 2014a), and the tropical Atlantic (Großkopf et al., 2012; Singh et al., 2017), is presented in Figure 1. The measurements reported are mostly from bulk unfiltered upper ocean samples, including cyanobacteria and potentially other diazotrophs. Although highly variable, the highest depth-integrated N2 fixation rates are found in the western tropical South Pacific (638 ± 1689 μmol N m−2 d−1, 201 profiles). These are larger than the traditionally high rates in the subtropical North Atlantic (182 ± 479 μmol N m−2 d−1, 636 profiles) and North Pacific (118 ± 101 μmol N m−2 d−1, 272 profiles). In the phosphate-rich waters of the eastern South Pacific average N2 fixation is 86 ± 99 μmol N m−2 d−1 (213 profiles). Low rates are found in the southern Indian Ocean (<20 μmol N m−2 d−1, Shiozaki et al., 2014a), and in cold Bering Sea waters (10 μmol N m−2 d−1, Shiozaki et al., 2017). Based on the MAREDAT database, Luo et al. (2012) estimated a global N2 fixation rate of 137 ± 9.2 Tg N y−1 (Table 1). Global extrapolations are difficult as observations remain sparse and highly variable in space and time (Figure 1). Only few ocean regions have sufficient data coverage to assess spatial, seasonal, or inter-annual variability (SD, Figure 1B). The large standard deviation in the western tropical South Pacific suggests a large spatio-temporal variability. The North Atlantic variability appears related to the strong spatial and temporal gradients of physical forcing and associated environmental factors (e.g., Mouriño-Carballido et al., 2011; Landolfi et al., 2016). The variability in the North Pacific is mostly associated with seasonal changes (Böttjer et al., 2016) and variable mesoscale activity (e.g., Church et al., 2009).
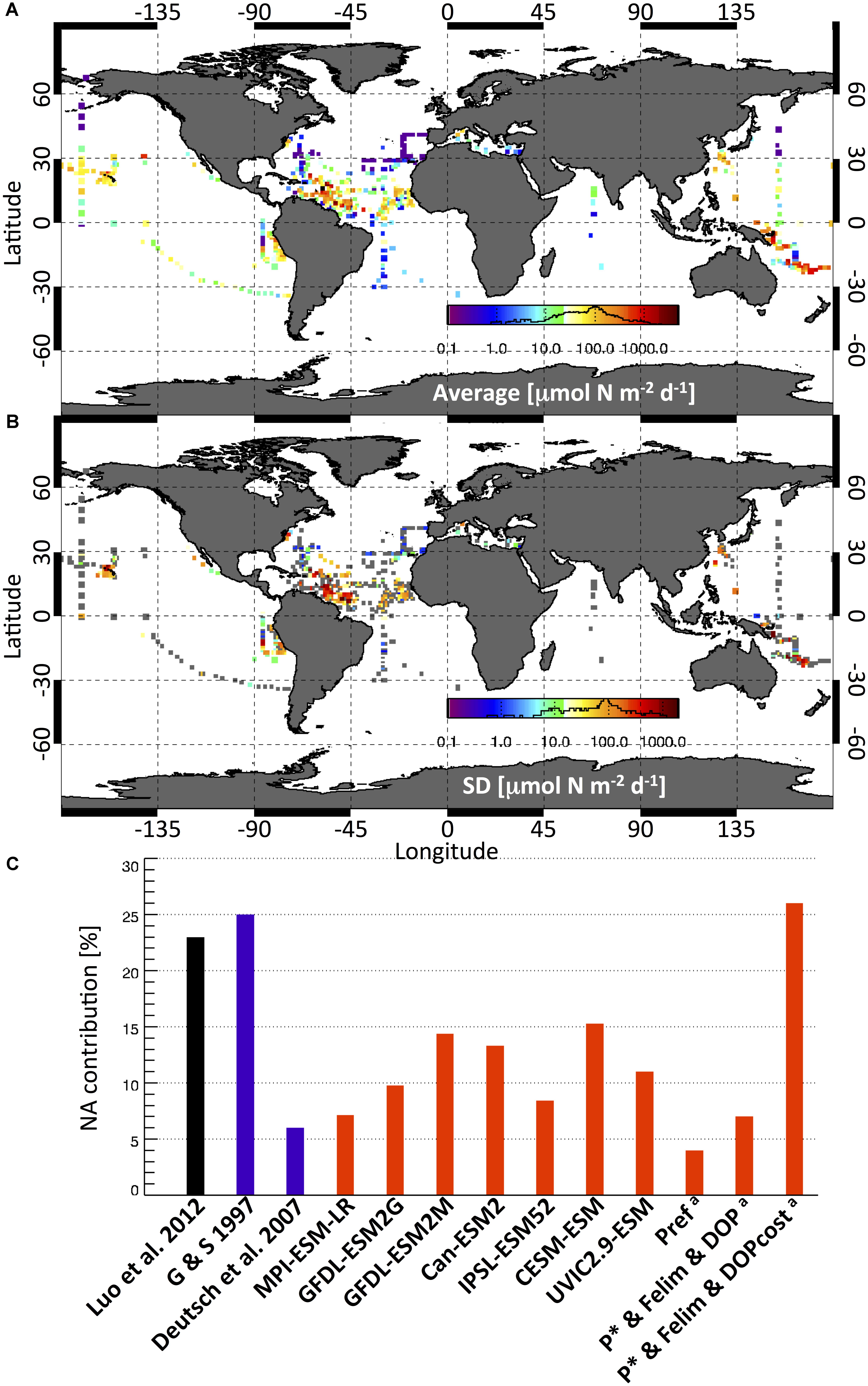
FIGURE 1. 1 × 1 degree grid (A) average and (B) standard deviation (SD) of depth integrated N2 fixation rate (μmol N m−2 d−1) experimental measurements from the MAREDAT database (Luo et al., 2012) and 800 additional data points (see text for details). Gray squares in SD plot indicate one-only data point. Data distribution histogram is shown in the color bar. (C) Experimental (black)-, geochemical (blue)-, and model (red) -based estimated contribution (%) of the North Atlantic to global N2 fixation rates. Models include those of the Coupled Model Intercomparison Project (CMIP5, see Table 1), a model with additional energetic costs of DOP uptake (UVIC2.9-ESM, Landolfi et al., 2017) and amodel experiments of Landolfi et al. (2015) accounting for preferential phosphorus remineralization (PREF), P∗ and iron limitation and DOP uptake without (P∗ and Fe and DOP), and with additional energetic and also N costs (P∗ and Fe and DOPcost).
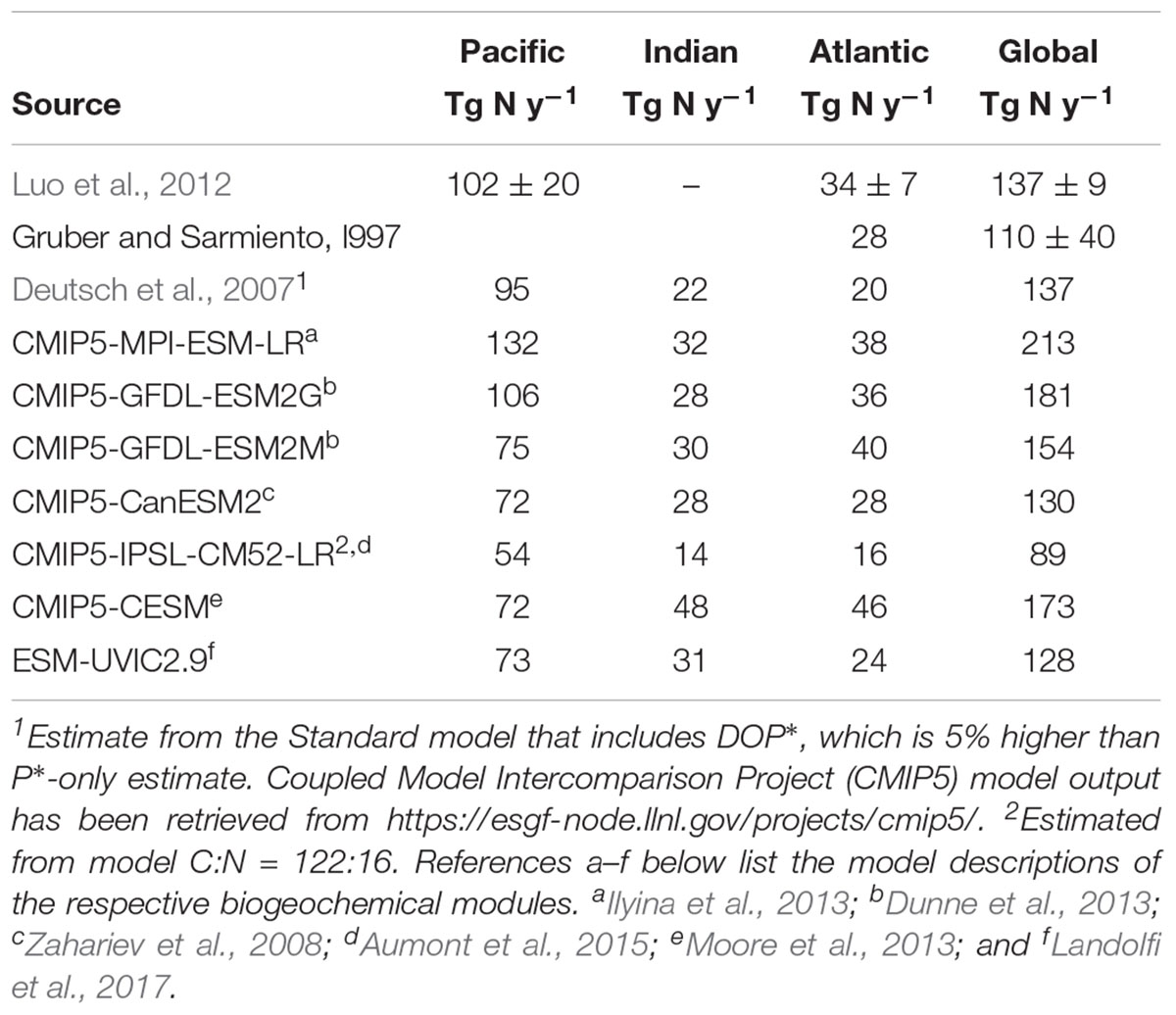
TABLE 1. Experimental, geochemical, and ESM-based (1996–2005 average) regional and global annual N2 fixation estimates (Tg N y−1).
Experimental measurements have technical drawbacks. The acetylene reduction assay (Capone, 1993), a proxy measurement of both incorporated and exuded (gross) fixed N2, measures the rate of ethylene production from added acetylene. Microbial activity repression (Fulweiler et al., 2015), low sensitivity and highly variable conversion factors for C2H4:N2 (Wilson et al., 2012), limit the robustness of this technique. The more widely (∼75% of the measurements) applied method involves adding 15N2 gas to a water sample and measuring the net incorporation of 15N2 into plankton biomass after filtration (Montoya et al., 1996). This method may result in significant underestimation of N2 fixation rates if the added 15N2 gas is not equilibrated before the start of the incubation (Mohr et al., 2010; Wilson et al., 2012; Wannicke et al., 2018). The degree of underestimation is variable and dependent on the experimental conditions (incubation time, size of bottle, shaking vigor), making the correction of historical data with a constant factor arbitrary. The 15N2 gas stock contamination by 15NH4+ or 15NOx, may result in N2 fixation overestimate (Dabundo et al., 2014). Fixation rates may be underestimated if small cells (<2 μm) are lost during filtration, particularly affecting rates where small diazotrophs may dominate the N2-fixing community (Bombar et al., 2018). Robust quantification of low rates is currently limited by the low sensitivity and high potential errors of present methods (Gradoville et al., 2017; Moisander et al., 2017). Inadequate consideration of experimental biases must be warned against. Community efforts are being made toward a consensus on methods and protocols refinement1 for yielding more accurate and comparable fixation rates.
Geochemical Estimates
Geochemical estimates of N2 fixation have been inferred from excess NO3 with respect to the Redfield-equivalent PO4 (the N∗ method), constructed from the high-quality global nutrient surveys (e.g., JGOFS/WOCE; Conkright et al., 2002), combined with information on ocean ventilation ages derived from measurements of abiotic transient tracers such as chlorofluorocarbons (CFCs). This method rests on the assumption that nutrients’ departure from the global average ratio of NO3:PO4 = 16:1 (RR, the Redfield ratio) is due to N2 fixation adding (N:P > RR), and denitrification removing (N:P < RR), nitrogen. This approach has been used to compute North Atlantic N2 fixation rates (Michaels et al., 1996; Gruber and Sarmiento, 1997; Hansell et al., 2004, 2007; Kähler et al., 2010), and derive a global estimate of 110 ± 40 Tg N y−1 (Gruber and Sarmiento, 1997; Table 1). While this method has the advantage of integrating over large scales of space and time and implicitly includes potential contributions of non-cyanobacterial N2 fixation, several potential shortcomings have been discussed: Impacts of non-Redfield remineralization of organic matter were addressed in Landolfi et al. (2008), where we extended the inorganic N∗ concept to total nitrogen excess, TNxs, to include organic nutrients. We found that for the subtropical North Atlantic, N2 fixation rates estimated via TNxs were approximately twice as high as those estimated by the N∗ method, but cautioned that other processes such as atmospheric N deposition (Zamora et al., 2010) and non-Redfield uptake of nutrients by phytoplankton (Mills and Arrigo, 2010; Weber and Deutsch, 2012), can also affect regional patterns of oceanic N∗ and TNxs and thereby influence N2 fixation rate estimates. These caveats also apply to the Pt∗ method introduced by Deutsch et al. (2007) that estimates N2 fixation from the consumption of Pt∗, which is defined as total excess P relative to the Redfield N equivalent. In contrast to the classical N∗ tracer, but similar to TNxs, Pt∗ also accounts for organic nutrients. Deutsch et al. (2007) estimated a global N2 fixation rate of 137 Tg N y−1 (i.e., about 25% higher than the N∗-based estimate), with a very large contribution from surface waters in close proximity to the largest ODZ in the eastern South Pacific and only a small contribution from the Pt∗-poor North Atlantic (Table 1). The Pt∗-based estimate contrasts with the prevailing view of elevated N2 fixation rates in the North Atlantic, as indicated both from N∗ and experimental evidence (Figure 1). Besides the above mentioned non-Redfield processes other than N2 fixation errors in the rate estimates also arise from the assumed ocean circulation that generally is not fully consistent with the observed nutrient fields to which it is applied. While current-generation global circulation models represent global-scale patterns reasonably well, they have particular deficiencies in reproducing low-oxygen regions (Cabré et al., 2015) and tracer distributions in the tropical regions (Dietze and Löptien, 2013). The refinement of global ocean GCMs is an on-going process. However, improvements to the geochemical methods may be limited given that the available nutrient distributions represent composites of data from decad of observations from a turbulent ocean, for which no underlying “mean” circulation may exist. Errors may also stem from uncertainties in tracer-derived water ages and age-based rate estimates: The differential effects of mixing on water age versus nutrient tracers may lead to errors in tracer-based N2 fixation estimates (Koeve and Kähler, 2016).
Model-Based Estimates
Global N2 fixation estimates have been derived from models using both implicit (diagnostic) and explicit (prognostic) N2 fixation parameterizations. Implicit N2 fixation parameterizations used in some IPCC-type earth-system-models (ESM) are based on restoring-type approaches that simulate N2 fixation by restoring upper-ocean nutrient ratios toward Redfield NO3:PO4 stoichiometry (MPI-ESM, Ilyina et al., 2013). This implies that N2 fixation is mainly restricted to areas with observed low NO3:PO4 ratios. Other models restrict N2 fixation to N limited areas, but account also for light and temperature dependence (Can ESM, Zahariev et al., 2008) and phosphate and iron limitation (IPSL-PISCES, Aumont et al., 2015). These methods implicitly assume a constant standing stock of diazotrophs and thereby require fewer assumptions on not well-known ecophysiological processes and parameters compared to models that explicitly model the dynamics of diazotrophs. Explicit parameterizations of N2 fixation are mostly based on the assumption that diazotrophs are slow-growing photosynthetic organisms that can fix N2. This simple mechanistic approach results in diazotrophs being outcompeted by the faster growing ordinary phytoplankton in N-replete regions, yielding a control by excess PO4, or P∗, in early on simple box-models (Tyrrell, 1999). Controls by other environmental factors such as temperature, light, iron (CESM, Moore et al., 2013) and also specific trade-offs (e.g., DOP-uptake, Landolfi et al., 2015) or nitrate and oxygen inhibition (GFDL-TOPAZ, Dunne et al., 2013) have been implemented in more complex functional-types ecosystem-ocean circulation models, modulating the rates and regional patterns of fixation. Some studies also parameterize different groups of diazotrophs, in particular Trichodesmium-type, unicellular cyanobacteria, and diatom-cyanobacterial associations (Monteiro et al., 2010). There is a significant spread in global and regional N2 fixation estimates by ESMs (Table 1). This arises from differences in model parameterizations and parameter values, and the different underlying circulation fields. In restoring approaches, N2 fixation is strongly associated with regions of NO3 deficits, or high P∗, from denitrification in low-oxygen waters (Ilyina et al., 2013). This tight coupling is relaxed in parameterizations with temperature-dependent and Fe-limited growth rates, and also with NO3 uptake by diazotrophs (Moore et al., 2013; Landolfi et al., 2017) or NO3 inhibition of N2 fixation (Zahariev et al., 2008; Dunne et al., 2013; Aumont et al., 2015), which all tend to reduce the simulated rates of N2 fixation in NO3-replete regions (e.g., Eastern Tropical South Pacific upwelling) and enhance them toward warm, dusty and P-rich waters in line with the traditional paradigm (Redfield et al., 1963; Falkowski, 1997). Most models do not reproduce the observed high fixation rates in the oligotrophic, dusty, North Atlantic due to the modeled low supply of P∗ in this region. This implies that unaccounted factors may also be important in controlling marine diazotrophs and require to go beyond the traditional paradigm. In a recent model study, we found that preferential phosphorus remineralization, dissolved organic phosphors uptake (DOP), or Fe-limited growth, were all not able to significantly enhance P∗ supply and expand the diazotrophs’ niche in this region. Only accounting for the elevated N-cost of dissolved organic P scavenging allowed the success of diazotrophs in the N-rich P-poor North Atlantic (Landolfi et al., 2015; Figure 1C). This theory, however, awaits experimental testing. Current global models do not account for non-cyanobacterial N2 fixation, which requires a more in-depth understanding of the relevant players, their ecophysiology and their environmental controls.
Implications for the N Budget
As N2 fixation and denitrification may be uncoupled, the oceanic N inventory is susceptible to imbalances. Despite the relatively short residence time of oceanic N of less than 3000 years (e.g., Somes et al., 2013), the geological isotopic record suggests a close-to-balanced budget over the past 3000 years (Altabet, 2007). This indicates the existence of negative feedbacks that stabilize the marine N reservoir. The nature, intensities and timescales of these feedbacks are still debated. Their effect on time scales shorter than those associated with the global ocean overturning circulation is assumed to require a spatial proximity of regions of N2 fixation and N removal, such that any N deficit generated by denitrification in ODZs is rapidly compensated for by N2 fixation (Deutsch et al., 2007). However, because denitrification consumes more N than is fixed per mole of organic P, a too tight spatial coupling would lead to net N removal (Landolfi et al., 2013). Thus any N deficit may need to be transported and compensated for far away from ODZ-influenced regions, suggesting that longer (ocean-circulation) timescales may be required to counteract any N imbalance (Landolfi et al., 2013; Somes et al., 2016).
Some studies suggested that N loss by denitrification may exceed, by more than 100 Tg N y−1, the inputs of N from N2 fixation, atmospheric and riverine supply, as a result of anthropogenic perturbations (Codispoti et al., 2001; Codispoti, 2007). While the recent revised estimates of N2 fixation (Table 1), atmospheric and river N supply (39 and 34 Tg N y−1, respectively, Jickellset al., 2017), and denitrification (120–240 Tg N y−1, DeVries et al., 2013) may suggest a reduced imbalance to less than 100 Tg N y−1, the annual rate and distribution at which N enters the ocean is still highly uncertain, making current and future N inventory projections speculative. The persistence of a 50–100 Tg N y−1 imbalance for 100 years would cause the N inventory to decline by 0.75–1.5% (N inventory of 6.6 105 Tg N, Gruber and Galloway, 2008) possibly weakening the biological carbon pump.
Quantification of non-cyanobacterial N2 fixation is currently hampered by the lack of reliable data (Moisander et al., 2017; Benavides et al., 2018) and knowledge of their metabolisms and environmental controls (Riemann et al., 2010; Bombar et al., 2016). However, biogeochemical observations can provide strong constraints on the potential magnitude of this process, which should leave its imprint on the spatial and vertical cumulative distributions of nutrients and the δ15NO3 signature in the ocean. Although thermodynamically favorable (ΔG < 0), the reduction of N2 to NH3 requires a large input of energy, from light or organic matter degradation, to proceed at measurable rates (Postgate, 1982) and maintain a low-oxygen cellular environment for the functioning of the enzyme complex nitrogenase. In ODZs the energy requirements may be reduced and iron limitation, a further constraint in oxic surface waters, may be relaxed. Here fixing N2 might be energetically advantageous compared with NO3-uptake (Großkopf and LaRoche, 2012). However, the low energetic efficiency of the anaerobic metabolism may quantitatively constrain the rates of non-cyanobacterial N2 fixation, which may result in invisible (“cryptic”) nutrient and isotopic tracer-distributions patterns as denitrification and anammox may override its signature. Further investigations should asses the contribution of this process.
Our current understanding of stabilizing N-inventory feedbacks stands on the regulatory-competition between N2 fixers and non-fixing phytoplankton (Redfield, 1963; Gruber, 2004). N2 fixers fertilize the ocean with N until fixed N levels are, relative to P, high enough for their competitive exclusion. The potential for N inputs via non-cyanobacterial N2 fixation independent of N deficits would fail this regulatory mechanism. Overall, the detailed mechanisms by which N2 fixation responds to N losses are not well understood to have high confidence of marine nutrient cycles future predictions. In this context, the extent to which the North Atlantic stands out as a region of high N2 fixation may be a pivotal question. Answering it will help to better understand how tight the feedback is between N2 fixation and N loss processes.
Synthesis and Outlook
All approaches to constrain global rates of N2 fixation (experimental, geochemical, and model-based estimates) have their own uncertainties and biases. Albeit the recent progress we still lack a comprehensive knowledge of the relevant environmental and ecological interactions, which prevent us from making robust N2 fixation rates estimates and predictions. To reduce the current level of uncertainty an improved understanding of the players, their ecological interactions, and the factors that control N2 fixation in the ocean must be drawn from a collective research effort. This requires to take novel approaches that go beyond assuming simple correlations/sensitivities, but rather consider a combination of environmental factors and emerging ecological interactions, both in the field and in the laboratory. To this end, identifying essential traits and ecological interactions experimentally, and develop mechanistic based models, may help us decipher the complexity of marine N2 fixation and allow robust fixation estimates. As of now, the large uncertainties in existing N2 fixation estimates and in the underlying feedbacks with N loss processes rule out reliable predictions. This task needs a fresh turn.
Author Contributions
AL designed and wrote the paper. All authors contributed to the writing of the paper.
Funding
This work was supported by the Deutsche Forschungsgemeinschaft (DFG) project SFB754.
Conflict of Interest Statement
The authors declare that the research was conducted in the absence of any commercial or financial relationships that could be construed as a potential conflict of interest.
Acknowledgments
We would like to thank S. Bonnet, C. Löscher, T. Shiozaki, and A. Singh for providing their N2 fixation measurements. We acknowledge the Coupled Model Intercomparison Project (CMIP) for providing accessible model output (http://pcmdi9.llnl.gov). We acknowledge the World Climate Research Program’s Working Group on Coupled Modeling, which is responsible for CMIP, and we thank the climate modeling groups (listed in Table 1) for producing and making available their model output. For CMIP the United States Department of Energy’s Program for Climate Model Diagnosis and Intercomparison provides coordinating support and led development of software infrastructure in partnership with the Global Organization for Earth System Science Portals. Many thanks to G. Volpe for his helpful input. Data to reproduce Figure 1 is available on https://data.geomar.de.
Footnotes
References
Altabet, M. A. (2007). Constraints on oceanic N balance/imbalance from sedimentary 15N records. Biogeoscience 4, 75–86. doi: 10.5194/bg-4-75-2007
Aumont, O., Ethé, C., Tagliabue, A., Bopp, L., and Gehlen, M. (2015). PISCES-v2: An ocean biogeochemical model for carbon and ecosystem studies. Geosci. Model Dev. 8, 2465–2513. doi: 10.5194/gmd-8-2465-2015
Benavides, M., Bonnet, S., Berman-Frank, I., and Riemann, L. (2018). Deep into oceanic N2 fixation. Front. Mar. Sci. 5:108. doi: 10.3389/fmars.2018.00108
Benavides, M., Bonnet, S., Hernández, N., Martínez-Pérez, A. M., Nieto-Cid, M., Álvarez-Salgado, X. A., et al. (2016). Basin-wide N2 fixation in the deep waters of the Mediterranean Sea. Glob. Biogeochem. Cycles 30, 1–19. doi: 10.1002/2015GB005326
Benavides, M. H., Moisander, P., Berthelot, H., Dittmar, T., Grosso, O., and Bonnet, S. (2015). Mesopelagic N2 fixation related to organic matter composition in the Solomon and Bismarck Seas (Southwest pacific). PLoS One 10:e0143775. doi: 10.1371/journal.pone.0143775
Berthelot, H., Benavides, M., Moisander, P. H., Grosso, O., and Bonnet S. (2017). High-nitrogen fixation rates in the particulate and dissolved pools in the Western Tropical Pacific (Solomon and Bismarck Seas). Geophys. Res. Lett. 44, 8414–8423. doi: 10.1002/2017GL073856
Bombar, D., Paerl, R. W., Anderson, R., and Riemann, L. (2018). Filtration via conventional glass fiber filters in 15N2 tracer assays fails to capture all nitrogen-fixing prokaryotes. Front. Mar. Sci. 5:6. doi: 10.3389/fmars.2018.00006
Bombar, D., Paerl, R. W., and Riemann, L. (2016). Marine non-cyanobacterial diazotrophs: moving beyond molecular detection. Trends Microbiol. 24, 916–927. doi: 10.1016/j.tim.2016.07.002
Bonnet, S., and Biegala, I. C., Dutrieux, P., Slemons, L. O., and. Capone, D. G. (2009). Nitrogen fixation in the western equatorial Pacific: rates, diazotrophic cyanobacterial size class distribution, and biogeochemical significance. Global Biogeochem. Cycles 23:GB3012. doi: 10.1029/2008GB003439
Bonnet, S., Caffin, M., Berthelot, H., and Moutin, T. (2017). Hot spot of N2 fixation in the western tropical South Pacific pleads for a spatial decoupling between N2 fixation and denitrification. Proc. Natl. Acad. Sci. U.S.A. 114, E2800–E2801. doi: 10.1073/pnas.1619514114
Bonnet, S., Dekaezemacker, J., Turk-Kubo, K. A., Moutin, T., Hamersley, R. M., Grosso, O., et al. (2013). Aphotic N2 fixation in the eastern tropical South Pacific Ocean. PLoS One 8:e81265. doi: 10.1371/journal.pone.0081265
Bonnet, S., Rodier, M., Turk-Kubo, K. A., Germineaud, C., Menkes, C., Ganachaud, A., et al. (2015). Contrasted geographical distribution of N2 fixation rates and nifH phylotypes in the Coral and Solomon Seas (southwestern Pacific) during austral winter conditions. Global Biogeochem. Cycles 29, 1874–1892. doi: 10.1002/2015GB005117
Bonnet, S., Caffin, M., Berthelot, H., Grosso, O., Benavides, M., Helias-Nunige, S., et al. (2018). In depth characterization of diazotroph activity across the western tropical south pacific hot spot of N2 fixation (OUTPACE cruise). Biogeosciences 15, 4215–4232. doi: 10.5194/bg-15-4215-2018
Böttjer, D., Dore, Karl, J. E., D. M. Letelier, R. M., Mahaffey, C., Wilson, S. T., Zehr, J., et al. (2016). Temporal variability of nitrogen fixation and particulate nitrogen export at Station ALOHA. Limnol. Oceanogr. 62, 200–216. doi: 10.1002/lno.10386
Cabré, A., Marinov, I., Bernardello, R., and Bianchi, D. (2015). Oxygen minimum zones in the tropical Pacific across CMIP5 models: mean state differences and climate change trends. Biogeosciences 12, 5429–5454. doi: 10.5194/bg-12-5429-2015
Capone, D. G. (1993). “Determination of nitrogenase activity in aquatic samples using the acetylene reduction procedure,” in Handbook of Methods in Aquatic Microbial Ecology, eds P. F. Kemp, B. F. Sherr, E. B. Sherr, and J. J. Cole (Boca Raton, FL: Lewis Publishers), 62–631.
Capone, D. G., Burns, J. A., Montoya, J. P., Subramaniam, A., Mahaffey, C., Gunderson, T., et al. (2005). Nitrogen fixation by Trichodesmium spp.: an important source of new nitrogen to the tropical and subtropical North Atlantic Ocean. Global Biogeochem. Cycles 19:GB2024. doi: 10.1029/2004GB002331
Caputo, A., Stenegren, M., Pernice, M. C., and Foster, R. A. (2018). A short comparison of two marine planktonic diazotrophic symbioses highlights an un-quantified disparity. Front. Mar. Sci. 5:2. doi: 10.3389/fmars.2018.00002
Church, M. J., Mahaffey, C., Letelier, R. M., Lukas, R., Zehr, J. P., and Karl, D. M. (2009). Physical forcing of nitrogen fixation and diazotroph community structure in the North Pacific Subtropical Gyre. Glob. Biogeochem. Cycles 23:GB2020. doi: 10.1029/2008GB003418
Codispoti, L. (2007). An oceanic fixed nitrogen sink exceeding 400 Tg N a21 vs the concept of homeostasis in the fixednitrogen inventory. Biogeoscience 4, 233–253. doi: 10.5194/bg-4-233-2007
Codispoti, L. A., Brandes, J. A., Christensen, J. P., Devol, A. H., Naqvi, S. W. A., Paerl, H. W., et al. (2001). The oceanic fixed nitrogen and nitrous oxide budgets: moving targets as we enter the anthropocene? Sci. Mar. 65, 85–105. doi: 10.3989/scimar.2001.65s285
Conkright, M. E., Locarnini, R. A., Garcia, H. E., O’Brien, T. D., Boyer, T. P., Stephens, C., et al. (2002). World Ocean Atlas 2001: Objective Analyses, Data Statistics, and Figures [CD-ROM Documentation]. Silver Spring, MD: National Oceanographic Data Center, 1–17.
Dabundo, R., Lehmann, M. F., Treibergs, L., Tobias, C. R., Altabet, M. A., Moisander, P. H., et al. (2014). The contamination of commercial 15N2 gas stocks with 15N-labeled nitrate and ammonium and consequences for nitrogen fixation measurements. PLoS One 9:e110335. doi: 10.1371/journal.pone.0110335
Deutsch, C., Sarmiento, J. L., Sigman, D. M., Gruber, N., and Dunne J. P. (2007). Spatial coupling of nitrogen inputs and losses in the ocean. Nature 445, 163–167. doi: 10.1038/nature05392
DeVries, T., Deutsch, C., Rafter, P. A., and Primeau, F. (2013). Marine denitrification rates determined from a global 3-D inverse model. Biogeosciences 10, 2481–2496. doi: 10.5194/bg-10-2481-2013
Dietze, H., and Löptien, U. (2013). Revisiting “Nutrient Trapping” in global coupled biogeochemical Ocean Circulation Models. Global Biogeochem. Cycles 27, 265–284. doi: 10.1002/gbc.20029
Dunne, J. P., John, J. G., Shevliakova, E., Stouffer, R. J., Krasting, J. P., Malyshev, S. L., et al. (2013). GFDL’s ESM2 global coupled climate-carbon earth system models. Part II: carbon system formulation and baseline simulation characteristics. J. Climate. 26, 2247–2267. doi: 10.1175/jcli-d-12-00150.1
Falkowski, P. G. (1997). Evolution of the nitrogen cycle and its influence on the biological sequestration of CO2 in the ocean. Nature 387, 272–275. doi: 10.1038/387272a0
Fernandez, C., Farías, L., and Ulloa, O. (2011). Nitrogen fixation in denitrified marine waters. PLoS One 6:e20539. doi: 10.1371/journal.pone.0020539
Foster, R. A., Kuypers, M. M. M., Vagner, T., Paerl, R. W., Musat, N., and Zehr, J. P. (2011). Nitrogen fixation and transfer in open ocean diatom – Cyanobacterial symbioses. ISME J. 5, 1484–1493. doi: 10.1038/ismej.2011.26
Fulweiler, R. W., Heiss, E. M., Rogener, M. K., Newell, S. E., LeCleir, G. R., Kortebein, S. M., et al. (2015). Examining the impact of acetylene on N-fixation and the active sediment microbial community. Front. Microbiol. 6:418. doi: 10.3389/fmicb.2015.00418
Gradoville, M. R., Bombar, D., Crump, B. C., Letelier, R. M., Zehr, J. P., and White, A. E. (2017). Diversity and activity of nitrogen-fixing communities across ocean basins. Limnol. Oceanogr. 62, 1895–1909. doi: 10.1002/lno.10542
Großkopf, T., and LaRoche, J. (2012). Direct and indirect costs of dinitrogen fixation in crocosphaera watsonii WH8501 and possible implications for the nitrogen cycle. Front. Microbiol. 3:236. doi: 10.3389/fmicb.2012.00236
Großkopf, T., Mohr, W., Baustian, T., Schunck, H., Gill, D., Kuypers, M. M. M., et al. (2012). Doubling of marine dinitrogen-fixation rates based on direct measurements. Nature 488, 361–364. doi: 10.1038/nature11338
Gruber, N. (2004). “The dynamics of the marine nitrogen cycle and its influence on atmospheric CO2 variations,” in The Ocean Carbon Cycle and Climate, NATO ASI Series, eds M. Follows and T. Oguz (Dordrecht: Kluwer Academic),97–148.
Gruber, N. (2016). Elusive marine nitrogen fixation. Proc. Natl. Acad. Sci. U.S.A. 113, 4246–4248. doi: 10.1073/pnas.1603646113
Gruber, N., and Galloway, J. N. (2008). An earth-system perspective of the global nitrogen cycle. Nature 451, 293–296. doi: 10.1038/nature06592
Gruber, N., and Sarmiento, J. L. (1997). Global patterns of marine nitrogen fixation and denitrification. Global Biogeochem. Cycles 11, 235–266. doi: 10.1029/97GB00077
Hansell, D. A., Bates, N. R., and Olson, D. B. (2004). Excess nitrate and nitrogen fixation in the North Atlantic Ocean. Mar. Chem. 84, 243–265. doi: 10.1016/j.marchem.2003.08.004
Hansell, D. A., Olson, D. B., Dentener, F., and Zamora, L. M. (2007). Assessment of excess nitrate development in the subtropical North Atlantic. Mar. Chem. 106, 562–579. doi: 10.1016/j.marchem.20070605
Ilyina, T., Six, K. D., Segschneider, J., Maier-Reimer, E., Li, H. M., and Nunez-Riboni, I. (2013). Global ocean biogeochemistry model HAMOCC: model architecture and performance as component of the MPI-Earth system model in different CMIP5 experimental realizations. J. Adv. Model. Earth Sys. 5, 287–315. doi: 10.1029/2012ms000178
Jayakumar, A., Al-Rshaidat, M., Ward, B. B., and Mulholland, M. R. (2012). Diversity, distribution, and expression of diazotroph nifH genes in oxygen-deficient waters of the Arabian Sea. FEMS Microbiol. Ecol. 82, 597–606. doi: 10.1111/j.1574-6941.2012.01430.x
Jickells, T. D., Buitenhuis, E., Altieri, K., Baker, A. R., Capone, D., Duce, R. A., et al. (2017). A reevaluation of the magnitude and impacts of anthropogenic atmospheric nitrogen inputs on the ocean. Global Biogeochem. Cycles 31, 289–305. doi: 10.1002/2016GB005586
Kähler, P., Oschlies, A., Dietze, H., and Koeve, W. (2010). Oxygen, carbon, and nutrients in the oligotrophic eastern subtropical North Atlantic. Biogeoscience 7, 1143–1156. doi: 10.5194/bg-7-1143-2010
Keeling, R. F., Körtzinger, A., and Gruber, N. (2010). Ocean deoxygenation in a warming world. Annu. Rev. Mar. Sci. 2, 199–229. doi: 10.1146/annurev.marine.010908.163855
Knapp, A. N., Casciotti, L., Berelson, W. M., Prokopenko, M. G., and Capone, D. G. (2016). Low rates of nitrogen fixation in eastern tropical South Pacific surface waters. Proc. Natl. Acad. Sci. U.S.A. 113, 4398–4403. doi: 10.1073/pnas.1515641113
Koeve, W., and Kähler, P. (2016). Oxygen utilization rate (OUR) underestimates ocean respiration: a model study. Global Biogeochem. Cycles 30, 1166–1182. doi: 10.1002/2015GB005354
Kustka, A., Sanudo-Wilhelmy, S., Carpenter, E. J., Capone, D. G., and Raven, A. (2003). A revised estimate of the iron use efficiency of nitrogen fixation, with special reference to the marine cyanobacterium Trichodesmium spp. (Cyanophyta). J. Phycol. 39, 12–25. doi: 10.1046/j.1529-8817.2003.01156.x
Landolfi, A., Dietze, H., Koeve, W., and Oschlies, A. (2013). Overlooked runaway feedback in the marine nitrogen cycle: the vicious cycle. Biogeoscience 10, 1351–1363. doi: 10.5194/bg-10-1351-2013
Landolfi, A., Dietze, H., and Volpe, G. (2016). Longitudinal variability of organic nutrients in the North Atlantic subtropical gyre. Deep Sea Res. Part I 111, 50–60. doi: 10.1016/j.dsr.2015.11.009
Landolfi, A., Koeve, W., Dietze, H., Kähler, P., and Oschlies, A. (2015). A new perspective on environmental controls of marine nitrogen fixation. Geoph. Res. Lett. 42, 4482–4489. doi: 10.1002/2015GL063756
Landolfi, A., Oschlies, A., and Sanders R. (2008). Organic nutrients and excess nitrogen in the North Atlantic subtropical gyre. Biogeoscience 5, 1199–1213. doi: 10.5194/bg-5-1199-2008
Landolfi, A., Somes, C. J., Koeve, W., Zamora, L. M., and Oschlies, A. (2017). Oceanic nitrogen cycling and N2O flux perturbations in the Anthropocene. Global Biogeochem. Cycles 31, 1236–1255. doi: 10.1002/2017GB005633
Löscher, C. R., Bourbonnais, A., Dekaezemacker, J., Charoenpong, C. N., Altabet, M. A., Bange, H. W., et al. (2016). N2 fixation in eddies of the eastern tropical South Pacific Ocean. Biogeosciences 13, 2889–2899. doi: 10.5194/bg-13-2889-2016
Löscher, C. R., Großkopf, T., Desai, F. D., Gill, D., Schunck, H., Croot, P. L., et al. (2014). Facets of diazotrophy in the oxygen minimum zone waters off Peru. ISME J. 8, 2180–2192. doi: 10.1038/ismej.2014.71
Luo, Y.-W., Doney, S. C., Anderson, L. A., Benavides, M., Berman-Frank, I., Bode, A., et al. (2012). Database of diazotrophs in global ocean: abundance, biomass and nitrogen fixation rates. Earth Syst. Sci. Data 4, 47–73. doi: 10.5194/essd-4-47-2012
Michaels, A. F., Olson, D., Sarmiento, J. L., Ammerman, J. W., Fanning, K., Jahnke, R., et al. (1996). Inputs, losses and transformations of nitrogen and phosphorus in the pelagic North Atlantic Ocean. Biogeochemistry 35, 181–226. doi: 10.1007/BF02179827
Mills, M. M., and Arrigo, K. R. (2010). Magnitude of oceanic nitrogen fixation influenced by the nutrient uptake ratio of phytoplankton. Nat. Geosci. 1, 439–443. doi: 10.1038/ngeo856
Mohr, W., Großkopf, T., Wallace, D. W., R., and Laroche J. (2010). Methodological underestimation of oceanic nitrogen fixation rates. PLoS One 5:e0012583. doi: 10.1371/journal.pone.0012583
Moisander, P. H., Benavides, M., Bonnet, S., Berman-Frank, I., White, A. E., and Riemann, L. (2017). Chasing after non-cyanobacterial nitrogen fixation in marine pelagic environments. Front. Microbiol. 8:1736. doi: 10.3389/fmicb.2017.01736
Monteiro, F., Follows, M. J., and Dutkiewicz, S. (2010). Distribution of diverse nitrogen fixers in the global ocean. Global Biogeochem. Cycles 24:GB3017. doi: 10.1029/2009GB003731
Montoya, J. P., Holl, C. M., Zehr, J. P., Hansen, A., Villareal, T. A., and Capone, D. G. (2004). High rates of N2 fixation by unicellular diazotrophs in the oligotrophic Pacific Ocean. Nature 430, 1027–1032. doi: 10.1038/nature02824
Montoya, J. P., Voss, M., Kähler, P., and Capone, D. G. (1996). A simple, high-precision, high-sensitivity tracer assay for N2 fixation. Appl. Environ. Microbiol. 62, 986–993.
Moore, J. K., Lindsay, K., Doney, S. C., Long, M. C., and Misumi, K. (2013). Marine ecosystem dynamics and biogeochemical cycling in the community Earth system model [CESM1(BGC)]: comparison of the 1990s with the 2090s under the RCP4.5 and RCP8.5 scenarios. J. Clim. 26, 9291–9312. doi: 10.1175/JCLI-D-12-00566.1
Mouriño-Carballido, B., Graña, R., Fernández, A., Bode, A., Varela, M., Domínguez, J. F., et al. (2011). Importance of N2 fixation vs. nitrate eddy diffusion along a latitudinal transect in the Atlantic Ocean. Limnol. Oceanogr. 56, 999–1007. doi: 10.4319/lo.2011.56.3.0999
Postgate, J. R. (1982). The Fundamentals of Nitrogen Fixation. Cambridge: Cambridge University Press.
Redfield, A. C., Ketchum, B. H., Richards, F. A. (1963). “The influence of organisms on the composition of sea-water,” in The Composition of Seawater: Comparative and Descriptive Oceanography. The Sea: Ideas and Observations on Progress in the Study of the Seas, Vol. 2, ed. M. N. Hill (Hoboken, NJ: Wiley-Interscience), 26–77.
Riemann, L., Farnelid, H., and Steward, G. F. (2010). Nitrogenase genes in non-cyanobacterial plankton: prevalence, diversity and regulation in marine waters. Aquat. Microb. Ecol. 61, 235–247. doi: 10.3354/ame01431
Shiozaki, T., Bombar, D., Riemann, L., Hashihama, F., Takeda, S., Yamaguchi, T., et al. (2017). Basin scale variability of active diazotrophs and nitrogen fixation in the North Pacific, from the tropics to the subarctic Bering Sea. Global Biogeochem. Cycles 31, 996–1009. doi: 10.1002/2017GB005681
Shiozaki, T., Ijichi, M., Kodama, T., Takeda, S., and Furuya. K. (2014a). Heterotrophic bacteria are major nitrogen fixers in the euphotic zone of the Indian Ocean. Global Biogeochem. Cycles 28, 1096–1110. doi: 10.1002/2014GB004886
Shiozaki, T., Kodama, T., and Furuya K. (2014b). Large-scale impact of the island mass effect through nitrogen fixation in the western South Pacific Ocean. Geophys. Res. Lett. 41, 2907–2913. doi: 10.1002/2014GL059835
Shiozaki, T., Kodama, T., Kitajima, S., Sato, M., and Furuya, K. (2013). Advective transport of diazotrophs and importance of their nitrogen fixation on new and primary production in the western Pacific warm pool. Limnol. Oceanogr. 58, 49–60. doi: 10.4319/lo.2013.58.1.0049
Shiozaki, T., Nagata, T., Ijichi, M., and Furuya, K. (2015a). Nitrogen fixation and the diazotroph community in the temperate coastal region of the northwestern North Pacific. Biogeoscience 12, 4751–4764. doi: 10.5194/bg-12-4751-2015
Shiozaki, T., Takeda, S., Itoh, S., Kodama, T., Liu, X., Hashihama, F., et al. (2015b). Why is Trichodesmium abundant in the Kuroshio? Biogeoscience 12, 6931–6943. doi: 10.5194/bg-12-6931-2015
Singh, A., Bach, L. T., Fischer, T., Hauss, H., Kiko, R., Paul, A. J., et al. (2017). Niche construction by non-diazotrophs for N2 fixers in the eastern tropical North Atlantic Ocean. Geophys. Res. Lett. 44, 6904–6913. doi: 10.1002/2017GL074218
Somes, C. J., Landolfi, A., Koeve, W., and Oschlies, A. (2016). Limited impact of atmospheric nitrogen deposition on marine productivity due to biogeochemical feedbacks in a global ocean model. Geophys. Res. Lett. 43, 4500–4509. doi: 10.1002/2016GL068335
Somes, C. J., Oschlies, A., and Schmittner, A. (2013). Isotopic constraints on the pre-industrial oceanic nitrogen budget. Biogeosciences 10, 5889–5910. doi: 10.5194/bg-10-5889-2013
Turk-Kubo, K. A., Karamchandani, M., Capone, D. G., and Zehr, J. P. (2014). The paradox of marine heterotrophic nitrogen fixation: abundances of heterotrophic diazotrophs do not account for nitrogen fixation rates in the Eastern Tropical South Pacific. Environ. Microbiol. 16, 3095–3114. doi: 10.1111/1462-2920.12346
Tyrrell, T. (1999). The relative influences of nitrogen and phosphorus on oceanic primary production. Nature 400, 525–553. doi: 10.1038/22941
Wannicke, N., Benavides, M., Dalsgaard, T., Dippner, J. W., Montoya, J. P., and Voss, M. (2018). New perspectives on nitrogen fixation measurements using 15N2 Gas. Front. Mar. Sci. 5:120. doi: 10.3389/fmars.2018.00120
Weber, T., and Deutsch, C. (2012). Oceanic nitrogen reservoir regulated by plankton diversity and ocean circulation. Nature 489, 419–422. doi: 10.1038/nature11357
Wilson, S. T., Böttjer, D., Church, M. J., and Karl, D. M. (2012). Comparative assessment of nitrogen fixation methodologies, conducted in the oligotrophic North Pacific Ocean. Appl. Environ. Microbiol. 78, 6516–6523. doi: 10.1128/AEM.01146-12
Zahariev, K., Christian, J. R., and Denman, K. L. (2008). Preindustrial, historical, and fertilization simulations using a global ocean carbon model with new parameterizations of iron limitation, calcification, and N2 fixation. Progr. Oceanogr. 77, 56–82. doi: 10.1016/j.pocean.2008.01.007
Zamora, L. M., Landolfi, A., Oschlies, A., Hansell, D. A., Dietze, H., and Dentener, F. (2010). Atmospheric deposition of nutrients and excess N formation in the North Atlantic. Biogeosciences 7, 777–793. doi: 10.5194/bg-7-777-2010
Keywords: marine N2 fixation, N-cycle balance, denitrification, marine productivity, marine heterotrophic diazotrophy, nitrogen isotopes
Citation: Landolfi A, Kähler P, Koeve W and Oschlies A (2018) Global Marine N2 Fixation Estimates: From Observations to Models. Front. Microbiol. 9:2112. doi: 10.3389/fmicb.2018.02112
Received: 17 May 2018; Accepted: 20 August 2018;
Published: 19 September 2018.
Edited by:
Lasse Riemann, University of Copenhagen, DenmarkReviewed by:
Carolin Regina Löscher, University of Southern Denmark, DenmarkBess B. Ward, Princeton University, United States
Copyright © 2018 Landolfi, Kähler, Koeve and Oschlies. This is an open-access article distributed under the terms of the Creative Commons Attribution License (CC BY). The use, distribution or reproduction in other forums is permitted, provided the original author(s) and the copyright owner(s) are credited and that the original publication in this journal is cited, in accordance with accepted academic practice. No use, distribution or reproduction is permitted which does not comply with these terms.
*Correspondence: Angela Landolfi, YWxhbmRvbGZpQGdlb21hci5kZQ==