- 1Department of Veterinary Medicine, HUTECH Institute of Applied Science, Ho Chi Minh City University of Technology, Ho Chi Minh City, Vietnam
- 2Department of Aquatic Life Medicine, College of Fisheries Science, Pukyong National University, Busan, South Korea
- 3Department of Biomedical Engineering, College of Engineering, Pukyong National University, Busan, South Korea
- 4Department of Chemistry, Center for Proteome Biophysics, Chemistry Institute for Functional Materials, Pusan National University, Busan, South Korea
- 5Department of Marine Biology and Aquaculture, College of Marine Science, Gyeongsang National University, Tongyeong, South Korea
The use of probiotics is considered an attractive biocontrol method. It is effective in growth promotion in aquaculture. However, the mode of action of probiotics in fish in terms of growth promotion remains unclear. The objective of the present study was to investigate growth promotion effect of dietary administration of host-derived probiotics, Lactococcus lactis WFLU12, on olive flounder compared to control group fed with basal diet by analyzing their intestinal and serum metabolome using capillary electrophoresis mass spectrometry with time-of flight (CE-TOFMS). Results of CE-TOFMS revealed that 53 out of 200 metabolites from intestinal luminal metabolome and 5 out of 171 metabolites from serum metabolome, respectively, were present in significantly higher concentrations in the probiotic-fed group than those in the control group. Concentrations of metabolites such as citrulline, tricarboxylic acid cycle (TCA) intermediates, short chain fatty acids, vitamins, and taurine were significantly higher in the probiotic-fed group than those in the control group. The probiotic strain WFLU12 also possesses genes encoding enzymes to help produce these metabolites. Therefore, it is highly likely that these increased metabolites linked to growth promotion in olive flounder are due to supplementation of the probiotic strain. To the best of our knowledge, this is the first study to show that dietary probiotics can greatly influence metabolome in fish. Findings of the present study may reveal important implications for maximizing the efficiency of using dietary additives to optimize fish health and growth.
Introduction
Application of probiotics is one of current approaches for biocontrol and growth promotion in aquaculture (Balcázar et al., 2006). Many different types of bacteria including lactic acid bacteria (LAB) are used as probiotics in fish. In particular, LAB can enhance fish survival by exerting metabolic effects at many levels because LAB can deal with low pH condition and bile acids toxicity presented throughout the gut (Corcoran et al., 2005; Martin et al., 2008). Recent studies have indicated that the use of host-derived probiotics can offer significant advantages in terms of survival and beneficial functions because their physiological activities are at optimum level in the same natural habitats (Lazado et al., 2015; Safari et al., 2016). Improved growth performance and decreased infection rate have been observed in olive flounder (Paralichthys olivaceus) fed with a host-derived LAB probiotic strain, Lactococcus lactis WFLU12 (Nguyen et al., 2017). Our recent study (Nguyen and Kim, 2018) has demonstrated that strain WFLU12 harbors genes supporting probiotic action based on genome-wide comparison of different L. lactis strains.
The growth of animals involves complex processes of protein synthesis and breakdown (Doherty and Whitfield, 2011). The intestine is an important site for the metabolism of dietary nutrients including high levels of protein and amino acids. These nutrients have to traverse intestinal mucosal epithelial cells before entering the bloodstream and body tissues. Metabolome is defined as biochemical compositions of small–molecule metabolites of amino acids, fatty acids, carbohydrates, vitamins, and lipids involved in metabolism. These small-molecule metabolites are required for the maintenance, growth, and normal function of cells (Harrigan and Goodacre, 2003). Metabolome analysis allows us to figure out biochemical reactions being catalyzed by proteins of the proteome which in turn can help us determine biological structure and function of the final phenotype of an organism. In addition, analysis of metabolites produced by intestinal microbiota is an ideal approach to assess health status of the host (Matsumoto et al., 2012). In fish, intestinal bacteria or probiotics possibly produce various kinds of enzymes such as cellulase, amylase, lipase, protease, and chitinase for digestion (Bairagi et al., 2002). They can also regulate the synthesis of specific essential nutrients such as amino acids, fatty acids, and vitamins for fish (Nayak, 2010; Semova et al., 2012). To the best of our knowledge, modes of actions of dietary probiotics used as supplement for fish growth have not been assessed, although large-scale metabolome analysis is rapidly evolving in recent years (Ohashi et al., 2008; Wikoff et al., 2009).
The objective of the present study was to clarify the mode of action involved in the growth promotion effect of probiotics administration in fish. Low-molecular-weight metabolites in samples obtained from healthy fish fed with or without probiotic strain WFLU12 were analyzed and expression levels of metabolites between the two groups were compared to determine changes in the probiotic-fed group. We also attempted to evaluate the benefit of dietary probiotics for host physiology through systemic circulation metabolome analysis. In addition, the whole genome of strain WFLU12 was analyzed to determine any potential genes involved in fish metabolism to increase metabolites.
Materials and Methods
Ethics Approval
Animal experiments performed in this study did not involve any endangered or protected species. All animal experiments were approved by the Animal Ethics Committee of Pukyong National University (Busan, Korea). These experiments were conducted under the guidelines of Animal Ethics Committee Regulations, No. 554 issued by Pukyong National University, Busan, Republic of Korea.
Animals and Probiotic Administration
Olive flounder (~165 g and ~26 cm fish−1) were purchased from a commercial fish farm in Korea and acclimatized to conditions of aerated and circulated fresh sea water under a 12:12 h light:dark photoperiod for several weeks. After acclimation, fish were randomly divided into a control group and a probiotic-treated group. Control fish were fed a commercial diet (DongA One Corporation, Korea) consisting of crude protein (52%), crude ash (15%), crude fat (10%), phosphorus (2.7%), crude fiber (2%), and calcium (1.2%), while the experimental group of fish were fed a probiotic-supplemented diet (strain WFLU12 at 109 CFU g−1 of commercial feed). Strain WFLU12 originally isolated from gastrointestinal tract of wild olive flounder has already been characterized biochemically and genomically in our previous studies (Nguyen et al., 2017; Nguyen and Kim, 2018). Both groups (n = 48 for each) were fed at a rate of 3% biomass per day with two daily feedings (9 a.m. and 5 p.m.) for 16 weeks. Weight and length of all the fish in each group were measured every two weeks as described previously (Nguyen et al., 2017). After obtaining growth rate and weight, the ration was adjusted accordingly for the next feeding period. At week 16, nine fish were randomly selected from each group to obtain serum and intestinal mucus samples to obtain three pooled serum and mucus samples for each group. All samples were subjected to analyses of ionic metabolites by CE-TOFMS.
CE-TOFMS
Metabolomics measurements were performed using an Agilent CE-TOFMS system (Agilent Technologies Inc.). Data were processed with Human Metabolome Technologies Inc. (Tsuruoka, Japan). Values of peaks were normalized to those of methionine sulfone (cationic internal standard) and D-camphor-10-sulfonic acid (anionic internal standard). Detailed information of specimen preparation and CE-TOFMS analysis used in this study is shown in Supplementary Material.
Ratio of Metabolite Concentration (Probiotics: Control Group)
In this study, we classified metabolites according to the ratio of values in probiotic-fed group to those in the control group (Pro/Con ratio) as described previously (Matsumoto et al., 2017). We defined threshold Pro/Con ratio to determine whether metabolites were present at higher or lower concentrations in probiotic-fed fish than those in control fish. In this study, the highest Pro/Con ratio for metabolites present at higher concentrations in fish fed with probiotics than those in control fish (the difference was significantly different) was 1.202 (Additional File 3). The lowest Pro/Con ratio for metabolites present at lower concentrations in fish fed with probiotics than those in control fish was 0.830 (Table S1). Therefore, the Pro/Con ratio of metabolites present at higher concentrations in fish fed with probiotics than those in control fish was defined at ≥ 1.20 and the Pro/Con ratio of metabolites present at lower concentrations in fish fed with probiotics than those in control fish was defined at <0.8.
Prediction of Genes of Strain WFLU12 Involved in Increase of Metabolites
To find genes of probiotic strain WFLU12 potentially involved in significant increase of metabolites in olive flounder, its whole genome sequence (Accession no. PKRZ00000000) (Nguyen and Kim, 2018) was exploited using KEGG analysis (Moriya et al., 2007).
Data Analysis and Statistics
Putative metabolites were assigned from HMT's standard compound library and Known-Unknown peak library based on m/z and MT. If several peaks were assigned to the same candidate, the candidate was given a branch number. Hierarchical cluster analysis (HCA) was performed with PeakStat ver. 3.18 (HMT's in-house software). Principal component analysis (PCA) was performed using SampleStat ver. 3.14 (HMT's in-house software). Differences in relative quantity of individual metabolites between probiotic-fed fish and control fish were evaluated by Welch's t-test. The ratio between the two was computed using averaged detection values. Differences in level distribution of whole metabolites between control and probiotic groups were compared using Wilcoxon–Mann–Whitney test. Statistical significance was considered at p < 0.05.
Results
Viability of Probiotic Cells in Fish Gut
Total bacterial counts grown on marine agar for gut mucus retrieved from control and probiotic-fed groups were 5.16 ± 0.72 and 4.79 ± 0.68 log CFU g−1 gut mucus, respectively, showing no significant difference between the two groups. Bacterial counts of L. lactis (confirmed by PCR) grown on MRS agar recovered from gut mucus of fish in the probiotic-fed group were found to be 4.65 ± 0.61 log CFU g−1. However, no LAB was found in gut mucus of fish from the control group.
Growth Measurements
Olive flounder were fed with or without probiotic-containing diet and their growths were monitored for 16 weeks. At the end of the experiment, mean weight and length were 329.98 (g) and 30.77 (cm), respectively, in the probiotic-fed group and 301.06 (g) and 29.60 (cm), respectively, in the control group (Figures 1A,B), showing significant differences between the two groups. Specific growth rate (SGR) and feed efficiency (FE) in the probiotic-fed group were 0.61 ± 0.18 and 0.72 ± 0.25, respectively, which were significantly higher than those (0.26 ± 0.12 and 0.30 ± 0.12, respectively) in the control group (Figures 1C,D).
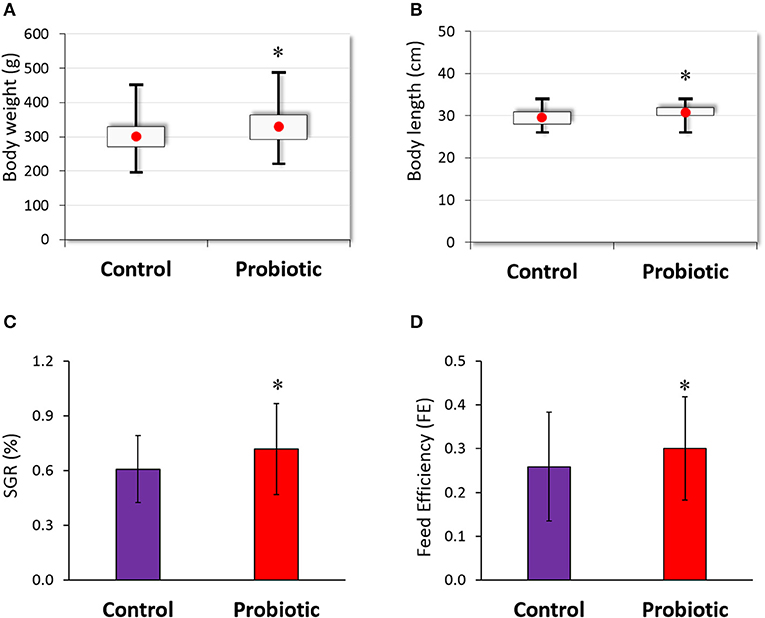
Figure 1. Growth performance of olive flounder (n = 48 for each group) fed with or without probiotic L. lactis WFLU12 (109 CFU/g of feed) supplemented diets. Weight gain (A), length gain (B), feed efficiency (C), and specific growth rate (D) of olive flounder were measured during the experiment period. Each bar represents mean value with standard error (SE). *p < 0.05 compared to the control.
Differences in Intestinal and Serum Metabolome Between the Two Dietary Groups
CE-TOFMS analyses of compounds present in the prepared pellet (including probiotic-mixed diets-Pellet-P and control diets-Pellet-C) showed 240 compounds, including 148 compounds in cation mode and 92 compounds in anion mode (Additional File 1). Levels of compounds between Pellet-P and Pellet-C were not significantly different (p > 0.05 based on Wilcoxon–Mann–Whitney test), indicating that these experimental diets equally distributed compounds to fish in both groups.
A total of 200 metabolites (110 cations and 90 anions) were identified from intestinal mucus samples by CE-TOFMS. Patterns of these metabolites from individual samples were subjected to hierarchical clustering and principal component analysis (PCA) as shown in Figure 2. Conspicuously, the intestinal metabolome displayed remarkable difference between the control and probiotic-fed fish groups (Figure 2C), showing that probiotic supplementation significantly modified intestinal metabolome of the host. Of these 200 metabolites, 147 metabolites showed no difference in concentration between the two groups (labeled as IFP ≈ IFC; Additional file 2). However, concentrations of 53 metabolites were significantly (p < 0.05) higher or at least 1.5-fold higher (p < 0.1) in the probiotic-fed fish group compared to those in the control group (labeled as IFP > IFC) (Figure 2D and Additional file 3).
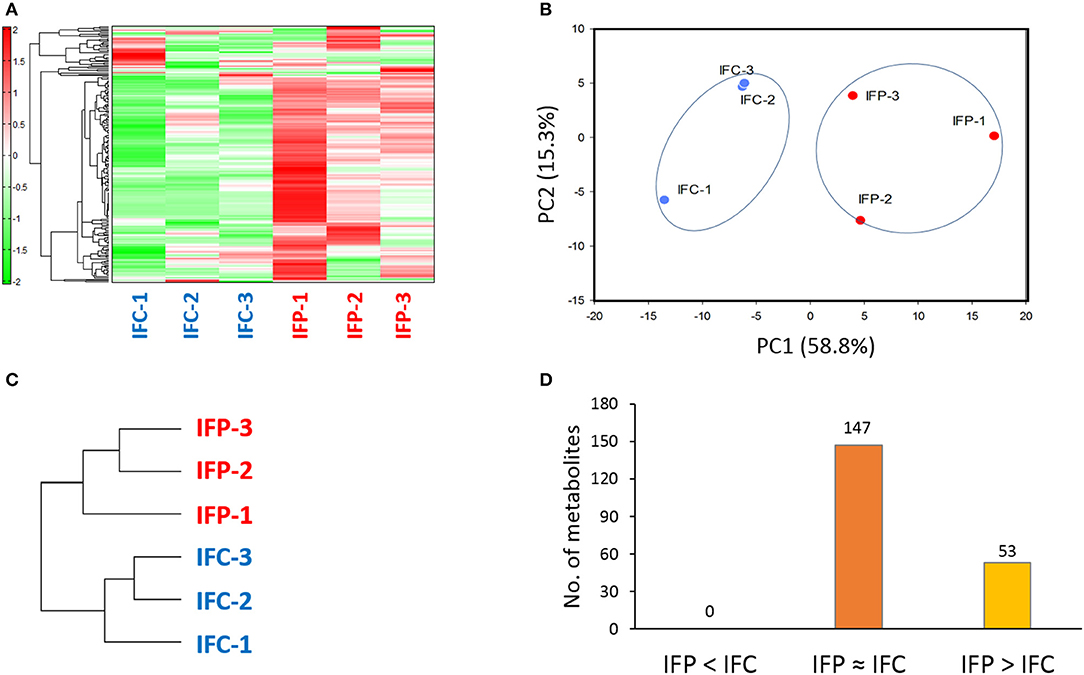
Figure 2. Difference in intestinal metabolome between control fish and probiotic-fed fish. (A) Hierarchical clustering showing patterns of intestinal metabolites. Red and green indicate high and low concentrations of metabolites, respectively. (B) PCA profiling of data from intestinal metabolome. (C) Phylogenetic tree displaying dissimilarity between control and probiotic-fed groups. (D) The number of intestinal metabolites in groups IFP > IFC, IFP ≈ IFC, and IFP < IFC.
A total of 171 metabolites (103 cations and 68 anions) were identified from serum samples from the two fish groups. PCA scores from plot and hierarchical clustering analysis showed that patterns of metabolites in the serum or the degree of clustering did not clearly show distint difference between the two groups (Figure 3). This indicates that probiotic treatment with strain WFLU12 did not significantly change serum metabolic profiles on fish. Mean values of five metabolites (carboxymethyllysine, citrulline, mannosamine, cystathionine, and caffeine) in the probiotic-fed fish group were significantly higher (p < 0.05) than those in the control group (denoted as SerP > SerC). However, six metabolites (glucuronic acid-1 or galacturonic acid-1, N,N-Dimethylglycine, prostaglandin F2α, phosphoenolpyruvic acid, homocarnosine, and γ-Glu-2-aminobutyric acid) in the serum were decreased markedly or at least 1.5-fold lower (p < 0.1) in the probiotic-fed group than those in the control group (denoted as SerP < SerC) (Table S1). Probiotic-fed fish also showed a tendency to have higher (p < 0.1) levels or unique detection of the following metabolites: 5-hydroxylysine, allantoic acid, acetoacetic acid, formiminoglutamic acid, Gln, tyrosine methyl ester, and penicillamine (Additional File 4).
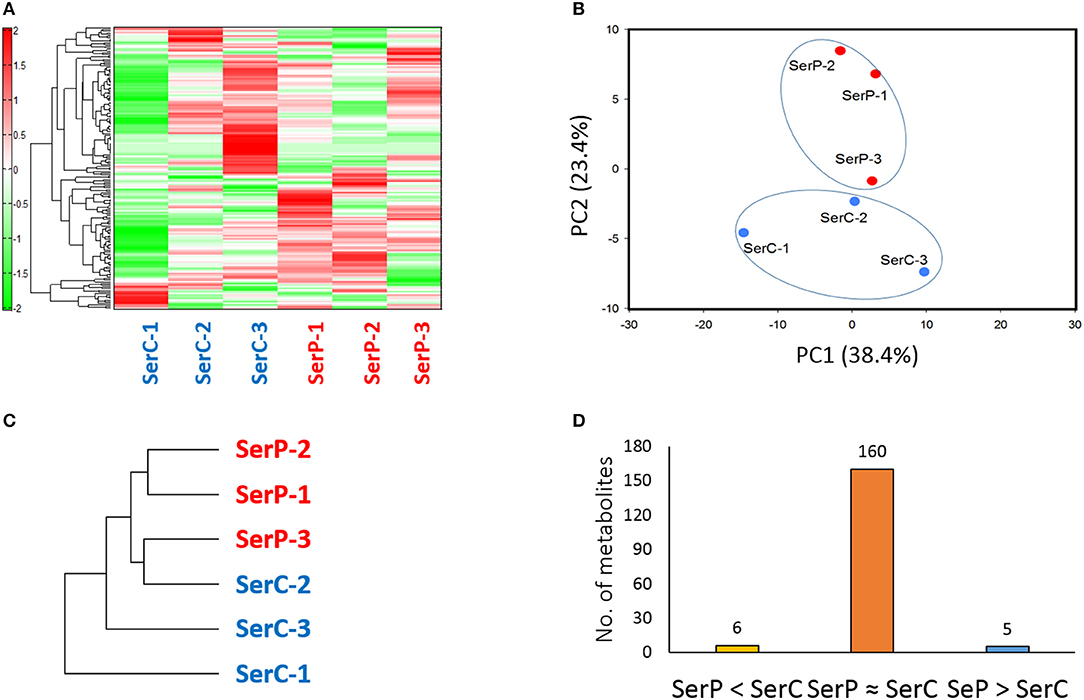
Figure 3. Difference in serum metabolome between control fish and probiotic-fed fish. (A) Hierarchical clustering showing patterns of serum metabolites. Red and green indicate high and low concentrations of metabolites, respectively. (B) PCA profiling of data from serum metabolome. (C) Phylogenetic tree displaying dissimilarity between control and probiotic-fed samples. (D) The number of serum metabolites in groups SerP > SerC, SerP ≈ SerC, and SerP < SerC.
Classification of Metabolites
To determine whether the probiotic supplement could help fish enhance feed conversion or maintain host physiology, we classified intestinal metabolites by comparing pellet compounds and serum metabolites. We then evaluated the influence of the supplemented probiotic on serum metabolome. Metabolites and/or compounds detected from the intestine and pellet were devived into five different groups: (a) metabolites belonging to group IFP > IFC but not detected from the pellet, (b) metabolites or compounds belonging to group IFP > IFC and detected from the pellet, (c) metabolites belonging to group IFP ≈ IFC but not detected from the pellet, (d) metabolites or compounds belonging to group IFP ≈ IFC and detected from the pellet, and (e) compounds only detected from the pellet (Figure 4A, Additional File 5). Of these metabolite and/or compound groups, this study focuses on groups a and b as they were likely to be invovled in changes in the metabolic processes in fish. Thus, these identified metabolites were classified into 10 functional groups (highlighted in Figure S1 and Additional File 3). In detail, nine metabolites belonging to group a might be produced by host/intestinal microbiota and enriched by the presence of probiotic bacteria in the intestine, while the 44 metabolites belonging to group b were those derived from the pellet and uplifted under the abundant survival of probiotic cells. In particular, amino acid derivatives (10 metabolites), central carbon metabolism intermediates (9 metabolites), and lipid metabolism relatives (8 metabolites) were found to be dominant in group IFP > IFC.
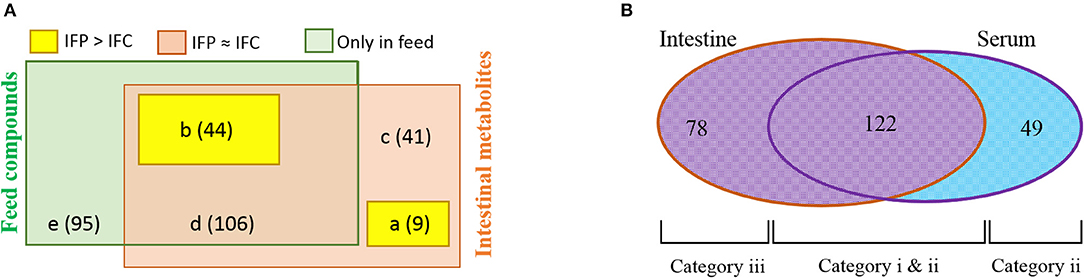
Figure 4. Venn diagram of metabolites and/or compounds noted in the pellet, intestine, and serum metabolome. (A) Classification of metabolites and/or compounds detected from fish intestine and pellets (five different groups). (B) Classification of metabolites detected from serum and intestine (see additional files and text for details).
Classification analysis of metabolites detected from fish intestine and serum revealed that 122 (71.3%) of 171 serum metabolites overlapped with intestinal metabolites (Figure 4B), suggesting that they might have been absorbed in the gut and conveyed to the body. A total of 78 metabolites were present only in the intestine profile. They might not have been transported to the body due to filtration by the intestinal wall and/or they are metabolized in internal organs before systemic circulation. The first chemical alteration (metabolization and/or bio-transformation) of many compounds can occur in the colonocyte and the liver (via portal vein) (Matsumoto et al., 2017). A total of 49 (28.7%) metabolites were detected only in the serum, implying that these metabolites might have been derived from metabolism in organs such as kidney, muscle, and liver (Wikoff et al., 2009; Torell et al., 2015). These metabolites detected in this study can be divided into three categories as shown in the Venn diagram (Figure 4B): (1) metabolites (similar pro/con ratios between the intestine and serum) that can be transported from the intestinal lumen to the systemic circulation independent of gateways (category i); (2) metabolites (different pro/con ratios between the intestine and serum) that are influenced by gateways such as the membrane transport system of enterocytes and/or metabolism during transcellular transport (category ii); and (3) metabolites that are not transported to the body or consumed by internal organs(category (iii). Of 122 metabolites (Table S2), 45 (No. 1 to No. 45) belonged to category i. They do not appear to be affected by any gateways. The remaining 77 metabolites (No. 46 to No. 122) belonged to category ii. They might be influenced by gateways.
Increased Metabolites and Weight Gain
Most metabolites (187 out of 200 in the intestine, and 114 out of 171 metabolites in the serum) detected in probiotic-fed fish had higher concentrations than these in the control (Figure S2). A right-skewed distribution of intestinal metabolite level was particularly indicated in the probiotic-fed group compared to that in the control group (Wilcoxon-Mann-Whitney test: p = 0.035 with test variant H1:a_con < b_pro). However, no significant differences in metabolite level was observed for the 171 metabolites detected from fish serum between the two groups. Relative quantities of annotated intestinal metabolites in principal metabolic pathways are represented as bar graphs as shown in Figure 5. Concentrations of metabolites involved in pathways of lipid and amino acid metabolism, polyamine, nucleotide metabolism, and central carbon metabolism were significantly higher in the probiotic-fed group than those in the control group (Table 1). Principal metabolic pathways of serum metabolites are depicted in Figure S3.
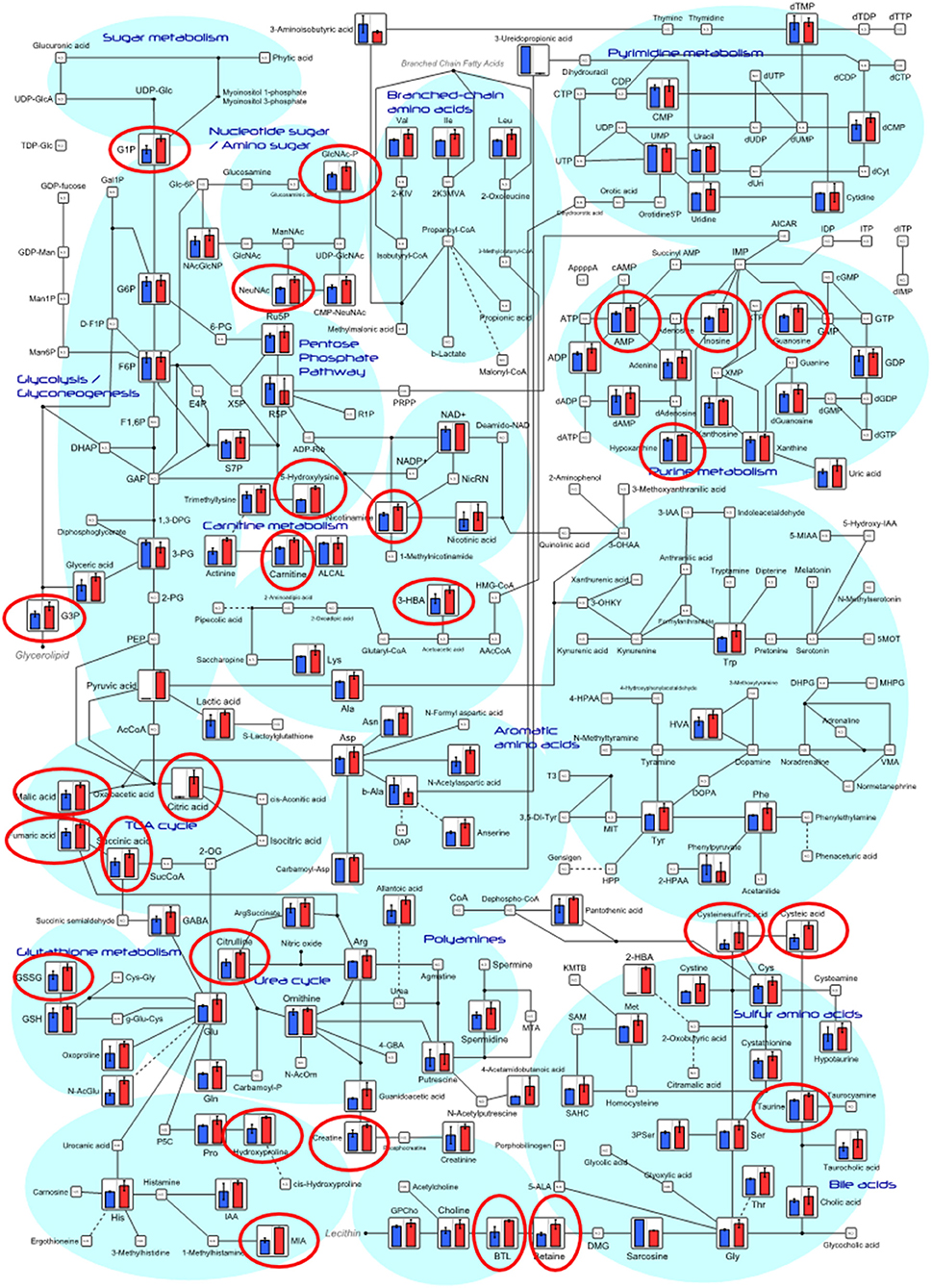
Figure 5. Differences of intestinal metabolites between control fish and probiotic-fed fish on systemic metabolic pathways. Relative quantities of annotated metabolites are represented as bar graphs (blue, control; red, probiotic). Metabolites surrounded by red circles are of higher concentrations in the probiotic group than those in the control group. ND, not detected.
Genes of L. lactis WFLU12 Involved in Increase of Metabolites
In the present study, 79 genes of L. lactis WFLU12 were found to be involved in 53 increased metabolites in the probiotic-fed group (Table 2). Functions of these genes included energy metabolism and nutrient absorption (e.g., vitamins, TCA cycle intermediates, taurine, and 3-hydroxybutyric acid), gut development (e.g., taurine, citrulline, and polyamines), and regulation of growth and development (e.g., citrulline, hydroxyproline, taurine). A better understanding for functions of genes involved in fish growth is needed because some metabolism pathways are found to be limited or even completely absent in fish due to the lack of host enzymes (for example, limited de novo synthesis of arginine in fish (Li et al., 2009), lack of nucleotides de novo synthesis in intestinal cells (Quan, 1992), and weak capacity of taurine biosynthesis in olive flounder (Wang et al., 2016).
Discussion
In this study, concentrations of metabolites measured in the probiotic-fed group were higher overall than those in the control group. In particular, concentrations of 53 and 5 metabolites were significantly higher in intestine and serum samples, respectively, of the probiotic-fed group than those in the control group. However, the number of significantly increased metabolites in the intestine differed somewhat from that in the serum of the probiotic-fed group. Such discrepancy might be due to several gateways from the gut lumen to systemic circulation in the blood. In this study, 45 (category i) metabolites were transported to the blood without being influenced by gateways while 77 (category ii) metabolites influenced by gateways were transported to the blood. Indeed, the types of metabolites that can and cannot be transported to the blood from the lumen remains unclear. A previous study (Matsumoto et al., 2017) showed that 11 basic amino acids including Phe, Lys, Ser, Gly, His, Thr, Arg, Pro, and branched chain amino acids (BCAAs) are transported independently from the colon to systemic circulation. In this study, however, most of the basic amino acids (18; see Table S2) were found to be filtrated and/or metabolized. This concurs with a previous study (Wu, 1998) showing that dietary amino acids can be catabolized by the small intestinal mucosa in the first pass. The intestine can modulate the availability of amino acids to extra-intestinal tissues. For example, the differences between the Pro/Con ratio of Arg (No. 74) and citrulline (No. 44) were observed in the intestinal mucus and serum samples in the present study. Citrulline is mainly synthesized from the conversion of glutamine in the enterocyte. Other amino acids such as arginine, proline, and ornithine can be converted into citrulline, and then released into the blood stream as an important source of endogenous arginine (Curis et al., 2007). Therefore, the higher Pro/Con ratio of citrulline in the serum than that in the intestine might be due to the metabolism of the amino acids by enterocytes. Moreover, the gastrointestinal tissue has been demonstrated to possess enzymes needed to metabolize methionine to cysteine (Finkelstein, 2000) or perform methionine transmethylation to release homocysteine and cystathionine into the circulation (Townsend et al., 2004). In this study, cysteine was only detected in intestinal mucus, and not in the pellet or serum (Additional File 2), indicating that methionine can be converted to cysteine by gut microbiota and enterocytes, and/or cysteine might be metabolized in the liver before systemic circulation. Cysteine might be extensively metabolized by intestinal enterocytes and the liver via cysteine dioxygenase to form cysteinesulfinate (Malmezat et al., 2000), a precursor of taurine. Higher Pro/Con ratio of cystathionine (No. 43) in serum metabolome might indicate transmethylation of methionine in the intestine. Cystathionine in the serum can be an important source of cysteine and glutathione synthesis in the body (Shoveller et al., 2005). Therefore, many metabolites might be influenced by intestinal metabolism. Pro/Con ratios of metabolites obtained in the present study are important for understanding the bioavailability of metabolites in fish.
It has been demonstrated that host circulating metabolites are linked to gut microbial species in human. They can cause body weight change by altering host energy harvest and storage (e.g., Wahl et al., 2015; Liu et al., 2017). Many studies have shown that the dietary supplementation of probiotics in farmed animals including fish can promote their growth without increasing fat mass (Khaksefidi and Rahimi, 2005; Šabatková et al., 2008). These observations might be due to improved gut function and resistance to infection rather than metabolic imbalance that causes overweight. Rogge (2009) has indicated that overweight is associated with decreased fatty acid β-oxidation. Therefore, overweight individuals are more dependent on the glycolytic pathway for ATP production, resulting in an increase in pyruvate production. The impact of gut microbiome on serum metabolome alterations has also been reported, showing that serum concentrations of many targeted metabolomics profiling of amino acids such as citrulline, cystine, lysine, and leucine are considerably higher in overweight individuals than those in lean controls (Liu et al., 2017). In our study, serum concentrations of citrulline, cystathionine (as an intermediate of sulfur amino acid cysteine), 2-hydroxy-4-methylvaleric acid (leucine derivative), and 5-hydroxylysine (an intermediate of lysine; 1.3-fold change; p < 0.1) were considerably higher in the probiotic-fed group of fish than those in the control group (Table S1, Additional File 4). The concentration of allantoic acid in the serum of the probiotic-fed group was also significantly higher compared to that in the control group (1.2-fold change; p = 0.05; Table 1 and Additional File 4). In most fish, the formed allantoin is further degraded to urea and glyoxalate via allantoic acid by allantoicase and allantoinase (Alvarez-Lario and Macarrón-Vivente, 2011). Since allantoin can be used for identifying serum uric acid level and predicting weight gain in humans (Masuo et al., 2003), increased level of this compound in the serum could be a sign of weight gain in fish.
Metabolites including amino acids are not only major fuels for intestinal mucosa, but also essential precursors for intestinal synthesis of glutathione, nitric oxide, polyamines, purine, and pyrimidine nucleotides as well as amino acids (e.g., citrulline, cysteine) which in turn will maintain intestinal mucosal mass and integrity (Wu, 1998). In this study, concentrations of citrulline in both the intestine and serum of probiotic-fed fish were significantly higher than those in the control. In general, arginine has a very weak gastrointestinal uptake rate. However, citrulline can be easily taken up in the gut and then converted to arginine in the kidney (Dhanakoti et al., 1990; Curis et al., 2007). The gut is known as the primary organ responsible for most circulating citrulline synthesis from glutamine (with ornithine carbamoyltransferase) (Wu, 1998; Bolotin et al., 2001). However, de novo synthesis of arginine in fish is limited or completely absent due to the lack of pyrroline-5-carboxylate (P5C) synthase in fish (Li et al., 2009). P5C is the key enzyme involved in the synthesis of proline and ornithine from glutamine/glutamate. These intermediates are then further metabolized to citrulline and arginine (Li et al., 2009). Therefore, fish have particularly high requirements for dietary arginine as a nutritionally indispensable and essential amino acid for growth. Kao et al. (2016) have shown that the unique gut microbiota might contribute to altered arginine and citrulline metabolism. It is well known that arginine is converted to citrulline through arginine deiminase (ADI) pathway (Noens et al., 2015). Similar to other L. lactis strains such as strain IL-1403 (Bolotin et al., 2001) and ATCC 7962 (Kim et al., 2007), strain WFLU12 harbors biosynthetic genes involved in the ADI pathway (Table 2). It has been demonstrated that the ADI pathway of L. lactis is a biocatalyst for citrulline production with a very high specific activity (140.3 U/mg) (Kim et al., 2007; Song et al., 2015).
Concentrations of polyamine (N8-acetylspermidine) and creatine in probiotics-fed fish were significantly higher than those in the control group (Table 1). This might be due to sufficient production of citrulline. Arginine derived from a precursor of citrulline can be catabolized to intermediates such as ornithine and guanidinoacetic acid (Figure S4), yielding polyamine and creatine as previously described (Morris, 2009; Andersen et al., 2016). Creatine is used as a storage molecule for energy in the form of phosphocreatine in the muscle. It has potential to increase muscle mass (Andersen et al., 2016). Creatine metabolism plays an essential role in epithelial cell barrier function and intestinal homeostasis in mice (Turer et al., 2017). Polyamines and their acetylated derivatives are essential in regulating and renewing intestinal mucosa (Gao et al., 2013; Ramani et al., 2014). Péres et al. (1997) have demonstrated that dietary polyamine spermine can increase digestive enzyme activity and promote intestinal maturation in sea bass larvae (Dicentrarchus labrax). Taken together, these results suggest that increased turnover of polyamine and creatine might be related to the increase of citrulline in probiotic-fed fish, implying its potential effects on energy metabolism and maintenance of robust gut health (Andersen et al., 2013) as well as symbiotic probiotics–host metabolic interactions.
It has been demonstrated that dietary supplementation with nucleotides (e.g., inosine, guanosine) can enhance the growth performance of many fish species, including olive flounder (Burrells et al., 2001; Song et al., 2012). Nucleotides might be very important for improving intestinal morphology (Cheng et al., 2011; Peng et al., 2013) because intestinal cells lack the ability to de novo synthesize nucleotides and must depend on exogenous supply (Quan, 1992). In the present study, purine derivatives including inosine, hypoxanthine, AMP, and guanosine were increased in the probiotic-fed group (Table 1, Figure S5), indicating that dietary supplementation of strain WFLU12 could induce de novo synthesis of purines in the intestine to meet the requirement for growth. Strain WFLU12 possesses gene add encoding adenosine deaminase (EC 3.5.4.4, Table 2). This gene can convert adenosine to inosine for utilizing general purine sources (Martinussen et al., 2003; Kilstrup et al., 2005).
In this study, concentrations of taurine and its precursors such as cysteic acid, homocysteic acid, and cysteinesulfinic acid were found to be significantly increased in the intestine of the probiotic-fed group compared to those in the control (Table 1; Figure S6). Taurine is a sulfonic acid found in high concentrations in animal tissues, including olive flounder (Sakaguchi and Murata, 1988; Jang et al., 2011). In recent years, several studies have demonstrated the essentiality (i.e., improved growth and survival) of dietary taurine for many commercially relevant species, especially marine teleosts including olive flounder (Park et al., 2002; Kim et al., 2003, 2008; Salze and Davis, 2015). Previous studies (Park et al., 2002; Wang et al., 2016) have demonstrated that olive flounder has a very weak taurine biosynthetic capability due to a mutation in enzyme cysteic acid decarboxylase (CAD). In the present study, KEGG analysis of L. lactis genome showed that it possessed genes encoding enzymes that might be involved in the biosynthesis of cysteic acid (Table 2) via serine/sulfate pathway (Machlin et al., 1955; Tevatia et al., 2015). Gene gadB (EC 4.1.1.15) encoding glutamate decarboxylase beta can potentially decarboxylate cysteic acid to yield taurine. In this study, analysis of molecular dynamics simulations and homology modeling for GAD protein of L. lactis WFLU12 and its substrates showed that both CSA and CA simulation shared binding sites of pyridoxal-5′-phosphate in GAD of the L. lactis structural model (Figure S7, Supplementary Material). Thus, GAD of L. lactis might be potentially involved in the bio-conversion of taurine and dietary supplementation of L. lactis might have contributed to the completion of the biosynthetic pathway of taurine in fish intestine.
It is worth noting that concentrations the 3-hydroxybutyric acid (3HBA) and 2-hydroxy-4-methylvaleric acid (HICA) values were significantly higher in the probiotic-fed group than those in the control group (Table 1). 3HBA is a storage compound of short chain fatty acids (SCFA) acetate (García Martín et al., 2006; Yagci et al., 2007) derived from fermentation of dietary fibers by intestinal microbiota (den Besten et al., 2013a,b). Amino acid derivative HICA is a protein-fermentation product of bacteria such as lactobacilli (Smit et al., 2004). The increase of 3HBA was correlated with increased acetoacetic acid (also diacetate) in the serum (Additional File 4), indicating that these ketone bodies might be readily reconverted to acetyl-CoA to produce energy (Laffel, 1999). Hence, metabolic shift of SCFA in the gut of fish can beneficially aid in nutrition source and ATP production for cells lining the intestine (den Besten et al., 2013b; Jost et al., 2014). It has been revealed that HICA can increase weight and muscle mass in chickens and rats (Boebel and Baker, 1982). In the present study, genes encoding enzyme 3-hydroxyisobutyrate dehydrogenase for conversion of 3HBA to acetoacetic acid and L-2-hydroxyisocaproate dehydrogenase (HicDH) for HICA production (de Cadiñanos de et al., 2013) were found in our probiotic genome (Table 2). However, functions of these metabolites in fish are not yet fully understood. Thus, further study is needed to clarify their functions in fish.
Concentrations of several metabolites involved in the TCA cycle were increased significantly in the intestine of fish fed with probiotics (Figure S8). TCA cycle intermediates are known to be able to improve growth, nutrients absorption, and bioavailability of minerals in several fish species (e.g., Pan et al., 2004; Sarker et al., 2005). In addition, increased carnitine, a key metabolite involved in fatty acid catabolism by β-oxidation after esterification to release stored energy from fatty acid (Xie et al., 2012) in the intestine of probiotic-fed group (Table 1), might be related to improvement of fat digestibility, thus increasing citric acid production through AcCoA intermediate (Figure S7). Digesting and absorbing dietary nutrients are critical physiological functions of the intestine necessary for maximal growth of the organism. In this context, the intestine has a high rate of energy expenditure because digestion and absorption processes are critically dependent on energy (Yang et al., 2016). Results of the present study suggest that energy generated from the citric acid cycle in the intestine might benefit fish digestion and absorption of nutrients in the probiotic-fed group, thus contributing to significant growth improvement.
Vitamins are important organic compounds that are essential for optimal growth and the health of animals. In general, vitamins are not synthesized by fish. They need to be supplied in the diet (Craig and Helfrich, 2009). It is well known that intestinal bacteria as well as lactic acid bacteria can synthesize and supply various vitamins (LeBlanc et al., 2013). In the present study, we found that fish in the probiotic-fed group showed increased levels of vitamin C (Table 1), known to be related to improvement of growth performance and feed utilization in different fish species (Fournier et al., 2000; Kim and Kang, 2015). In this study, increased level of hydroxyproline in the probiotic-fed group might be related to post-translational hydroxylation of proline by vitamin C-dependent prolyl hydroxylase (Li et al., 2009). Members of vitamin B complex are also needed in fish diet as they play major roles in growth, physiology, and cellular metabolism (Shiau and Lin, 2015). In this study, levels of co-enzymes such as riboflavin, thiamine, and nicotinamide were increased in fish fed with probiotics compared to those in the control group (Table 1), indicating that they might play an important role in energy metabolism of carbohydrates, fats, and proteins (LeBlanc et al., 2017). Similar to other L. lactis strains (Burgess et al., 2004; Shimizu-Kadota et al., 2013), our strain possesses various genes involved in the bioconversion of vitamins (Table 2). These features of strain WFLU12 might confer fish metabolic versatility of converting materials for energy in fish intestine.
Previous studies (e.g., Drissi et al., 2014) on weight gain-associated Lactobacillus spp. have shown that these Lactobacillus encode ubiquitous enzymes with key roles in β-oxidation pathway of fatty acid degradation (Thiolase I; EC 2.3.1.16) and various biosynthetic pathways (Thiolase II; EC 2.3.1.9) such as poly β-hydroxybutyric acid synthesis and steroid biogenesis (Haapalainen et al., 2006). Thus, genomes of weight gain-associated Lactobacillus might be able to mobilize energy and carbon stored in fatty acids through β-oxidation. In agreement with this, our strain harbored both thiolase I and II (Table 2). These might be responsible for the increase of poly β-hydroxybutyric acid and β-oxidation pathway (level of carnitine in Table 1). This is another evidence supporting that our probiotic strain could increase different kinds of metabolites as observed in this study.
Conclusion
Dietary supplementation with L. lactis WFLU12 greatly influenced metabolism in fish. Several metabolites including citrulline, TCA cycle intermediates, SCFA, vitamins, and taurine are likely to be linked to growth promotion in fish. In particular, our probiotic strain has a number of genes encoding diverse enzymes to help many important metabolic pathways such as urea cycle relating metabolism, taurine biosynthesis, and de novo synthesis of nucleotides in the intestine. In the field of gastrointestinal physiology, our pioneering study demonstrates that intestinal catabolism plays a very important role in modulating metabolite availability to extra-intestinal tissues. Our data also provide novel insights into the metabolomic mode of action of probiotics involved in fish health and growth.
Author Contributions
TN designed the experiments, performed data processing and analysis, and wrote the paper. W-KC, AK, NK, HR, and YL performed experiments. MY performed experiments and analyzed the data. SK and C-IP analyzed the data. D-HK conceived and designed the experiments, and wrote the paper. All authors edited the manuscript and approved the final version.
Conflict of Interest Statement
The authors declare that the research was conducted in the absence of any commercial or financial relationships that could be construed as a potential conflict of interest.
Acknowledgments
This research was a part of the project entitled Omics based on fishery disease control technology development and industrialization (20150242) funded by the Ministry of Oceans and Fisheries, Korea. This research was also supported by a grant (NRF-2015R1D1A1A01058964) of the Basic Science Research Program through the National Research Foundation of Korea (NRF) funded by the Ministry of Education.
Supplementary Material
The Supplementary Material for this article can be found online at: https://www.frontiersin.org/articles/10.3389/fmicb.2018.02059/full#supplementary-material
Figure S1. Classification of intestinal metabolites in four different groups displayed in Figure 4A. Identified metabolites were classified into 10 functional groups (see main text for details).
Figure S2. Ratio of metabolite concentration in probiotic-fed vs. control. (A) Intestinal metabolites. (B) Serum metabolites. The ratio is computed by using averaged detection values.
Figure S3. Differences of serum metabolites between control fish and probiotic-fed fish on systemic metabolic pathways. Relative quantities of annotated metabolites are presented as bar graphs (green, control; orange, probiotic). Metabolites surrounded by red circles are of higher concentrations in the probiotic group than those in the control group. ND, not detected.
Figure S4. Differences of intestinal metabolites between control fish and probiotic-fed fish on urea cycle related metabolism pathways. Relative areas of annotated metabolites are presented as bar graphs (blue, control; red, probiotic). Metabolites surrounded by red circles are of higher concentrations in the probiotic group than those in the control group. ND, not detected.
Figure S5. Differences of intestinal metabolites between control and probiotic fish on purine metabolism pathways. Relative quantities of annotated metabolites are presented as bar graphs (blue, control; red, probiotic). Metabolites surrounded by red circles are of higher concentrations in the probiotic group than those in the control group. ND, not detected.
Figure S6. Differences of intestinal metabolites between control fish and probiotic-fed fish on lipid and amino acid metabolism pathways. Relative areas of annotated metabolites are presented as bar graphs (blue, control; red, probiotic). Metabolites surrounded by red circles are of higher concentrations in the probiotic group than those in the control group. ND, not detected.
Figure S7. Binding sites of pyridoxal-5′-phosphate (PLP) and substrates in L. lactis GAD. The overall dimeric structure of LcGAD is indicated by ribbons and colored by blue and red for each chain (A). PLP and substrates (B: L-Glu, C: CSA, D: CA) are presented as thick sticks and residues forming the binding site are presented as thin sticks. Carbon, nitrogen, oxygen, phosphorus, and sulfur atoms are colored by cyan, blue, red, gold, and yellow, respectively.
Figure S8. Differences of intestinal metabolites between control fish and probiotic-fed fish on the central carbon metabolism pathways. Relative areas of annotated metabolites are presented as bar graphs (blue, control; red, probiotic). Metabolites surrounded by red circles are of higher concentrations in the probiotic group than those in the control group. ND, not detected.
Table S1. List of metabolites significantly changed in the serum. 1<, only detected in probiotic group. N.D. (Not Detected): Target peak or metabolite was below detection limits.
Table S2. Pro/Con ratio and possible influence of gateways on each metabolite detected in the intestine and serum.
Table S3. Binding sites of PLP and substrates in three simulation systems.
Additional File 1. Compounds detected in the pellet mixed with probiotics (Pellet-P) and control pellet (Pellet-C).
Additional File 2. List of 147 metabolites in group IFP ≈ IFC.
Additional File 3. List of 53 intestinal metabolites increased significantly in probiotic-fed fish (group IFP > IFC).
Additional File 4. List of 160 metabolites showing no significant change in the serum.
Additional File 5. Additional information on metabolite classification (Figure 4).
References
Alvarez-Lario, B., and Macarrón-Vivente, J. (2011). Is there anything good in uric acid? QJM 104, 1015–1024. doi: 10.1093/qjmed/hcr159
Andersen, S. M., Holen, E., Aksnes, A., Rønnestad, I., Zerrahn, J. E., and Espe, M. (2013). Dietary arginine affects energy metabolism through polyamine turnover in juvenile Atlantic salmon (Salmo salar). Br. J. Nutr. 110, 1968–1977. doi: 10.1017/S0007114513001402
Andersen, S. M., Waagbø, R., and Espe, M. (2016). Functional amino acids in fish nutrition, health and welfare. Front. Biosci. 8, 143–169. doi: 10.2741/e757
Bairagi, A., Ghosh, K., Sen, S. K., and Ray, A. K. (2002). Enzyme producing bacterial flora isolated from fish digestive tracts. Aquaculture Intl. 10, 109–121. doi: 10.1023/A:1021355406412
Balcázar, J. L., de Blas, I., Ruiz-Zarzuela, I., Cunningham, D., Vendrell, D., and Múzquiz, J. L. (2006). The role of probiotics in aquaculture. Vet. Microbiol. 114, 173–186. doi: 10.1016/j.vetmic.2006.01.009
Boebel, K. P., and Baker, D. H. (1982). Comparative utilization of the alpha-keto and D- and L-alpha-hydroxy analogs of leucine, isoleucine and valine by chicks and rats. J. Nutr. 112, 1929–1939. doi: 10.1093/jn/112.10.1929
Bolotin, A., Wincker, P., Mauger, S., Jaillon, O., Malarme, K., Weissenbach, J., et al. (2001). The complete genome sequence of the lactic acid bacterium Lactococcus lactis ssp. lactis IL1403. Genome. Res. 11, 731–753. doi: 10.1101/gr.169701
Burgess, C. M., O'Connell-Motherway, M., Sybesma, W., Hugenholtz, J., and van Sinderen, D. (2004). Riboflavin production in Lactococcus lactis: potential for in situ production of vitamin-enriched foods. Appl. Environ. Microbiol. 70, 5769–5777.
Burrells, C., William, P. D., Southgate, P. J., and Wadsworth, S. L. (2001). Dietary nucleotides: a novel supplement in fish feeds: 2. Effects on vaccination salt water transfer, growth rate and physiology of Atlantic salmon (Salmo salar L.). Aquaculture 199, 171–184. doi: 10.1016/S0044-8486(01)00576-2
Cheng, Z., Buentello, A., and Gatlin, D. M. (2011). Dietary nucleotides influence immune responses and intestinal morphology of red drum Sciaenops ocellatus. Fish Shellfish Immunol. 30, 143–147. doi: 10.1016/j.fsi.2010.09.019
Corcoran, B. M., Stanton, C., Fitzgerald, G. F., and Ross, R. P. (2005). Survival of probiotic lactobacilli in acidic environments is enhanced in the presence of metabolizable sugars. Appl. Environ. Microbiol. 71, 3060–3067. doi: 10.1128/AEM.71.6.3060-3067.2005
Craig, S., and Helfrich, L. A. (2009). Understanding Fish Nutrition, Feeds, and Feeding. Virginia cooperative extension, Communications and Marketing, College of Agriculture and Life Sciences, Virginia Polytechnic Institute and State University. 420–256.
Curis, E., Crenn, P., and Cynober, L. (2007). Citrulline and the gut. Curr. Opin. Clin. Nutr. Metab. Care. 10, 620–626. doi: 10.1097/MCO.0b013e32829fb38d
de Cadiñanos, L. P. G., García-Cayuela, T., Yvon, M., Martinez-Cuesta, M. C., Peláez, C., and Requena, T. (2013). Inactivation of the PanE gene in Lactococcus lactis enhances formation of cheese aroma. Appl. Environ. Microbiol. 79, 3503–3506. doi: 10.1128/AEM.00279-13
den Besten, G., Lange, K., Havinga, R., van Dijk, T. H., Gerding, A., van Eunen, K., et al. (2013b). Gut-derived short-chain fatty acids are vividly assimilated into host carbohydrates and lipids. Am. J. Physiol. Gastrointest. Liver Physiol. 305, G900–G910. doi: 10.1152/ajpgi.00265.2013
den Besten, G., van Eunen, K., Groen, A. K., Venema, K., Reijngoud, D. J., and Bakker, B. M. (2013a). The role of short-chain fatty acids in the interplay between diet, gut microbiota, and host energy metabolism. J. Lipid. Res. 54, 2325–2340. doi: 10.1194/jlr.R036012
Dhanakoti, S. N., Brosnan, J. T., Herzberg, G. R., and Brosnan, M. E. (1990). Renal arginine synthesis: studies in vitro and in vivo. Am. J. Physiol. 259 (Endocrinol. Metab. 22), E437–E442. doi: 10.1152/ajpendo.1990.259.3.E437
Doherty, M. K., and Whitfield, P. D. (2011). Proteomics moves from expression to turnover: update and future perspectives. Exp. Rev. Proteomics 8, 325–334. doi: 10.1586/epr.11.19
Drissi, F., Merhej, V., Angelakis, E., El Kaoutari, A., Carrière, F., Henrissat, B., et al. (2014). Comparative genomics analysis of Lactobacillus species associated with weight gain or weight protection. Nutr. Diabetes 4:e109. doi: 10.1038/nutd.2014.6
Finkelstein, J. D. (2000). Pathways and regulation of homocysteine metabolism in mammals. Semin. Thromb. Hemost. 26, 219–225. doi: 10.1055/s-2000-8466
Fournier, V., Gouillou-Coustans, M. F., and Kaushik, S. J. (2000). Hepatic ascorbic acid saturation is the most stringent response criterion for determining the vitamin C requirement of juvenile European sea bass (Dicentrarchus labrax). J. Nutr. 130, 617–620. doi: 10.1093/jn/130.3.617
Gao, J. H., Guo, L. J., Huang, Z. Y., Rao, J. N., and Tang, C. W. (2013). Roles of cellular polyamines in mucosal healing in the gastrointestinal tract. J. Physiol. Pharmacol. 64, 681–693.
García Martín, H., Ivanova, N., Kunin, V., Warnecke, F., Barry, K. W., McHardy, A. C., et al. (2006). Metagenomic analysis of two enhanced biological phosphorus removal (EBPR) sludge communities. Nat. Biotechnol. 24, 1263–1269. doi: 10.1038/nbt1247
Haapalainen, A. M., Meriläinen, G., and Wierenga, R. K. (2006). The thiolase superfamily:condensing enzymes with diverse reaction specificities. Trends Biochem. Sci. 31, 64–71. doi: 10.1016/j.tibs.2005.11.011
Harrigan, G. G., and Goodacre, R. (2003). Metabolic Profiling: its Role in Biomarker Discovery and Gene Function Analysis. New York, NY: Springer. doi: 10.1007/978-1-4615-0333-0
Jang, M. S., Park, H. Y., Kim, K. W., Kim, K. D., and Son, M. H. (2011). Comparison of free amino acids and nucleotides content in the olive flounder Paralichthys olivaceus fed with extruded pellet. Korean J. Food. Preserv. 18, 746–754. doi: 10.11002/kjfp.2011.18.5.746
Jost, T., Lacroix, C., Braegger, C., and Chassard, C. (2014). Stability of the maternal gut microbiota during late pregnancy and early lactation. Curr. Microbiol. 68, 419–427. doi: 10.1007/s00284-013-0491-6
Kao, C. C., Cope, J. L., Hsu, J. W., Dwarkanath, P., Karnes, J. M., Luna, R. A., et al. (2016). The microbiome, intestinal function, and arginine metabolism of healthy Indian women are different from those of American and Jamaican Women. J. Nutr. 146, 706–713. doi: 10.3945/jn.115.227579
Khaksefidi, A., and Rahimi, S. (2005). Effect of probiotic inclusion in the diet of broiler chickens on performance, feed efficiency and carcass quality. Asian-Australasian J. Anim. Sci. 18, 1153–1156. doi: 10.5713/ajas.2005.1153
Kilstrup, M., Hammer, K., Ruhdal Jensen, P., and Martinussen, J. (2005). Nucleotide metabolism and its control in lactic acid bacteria. FEMS Microbiol. Rev. 29, 555–590. doi: 10.1016/j.fmrre.2005.04.006
Kim, J. E., Jeong, D. W., and Lee, H. J. (2007). Expression, purification, and characterization of arginine deiminase from Lactococcus lactis ssp. lactis ATCC 7962 in Escherichia coli BL21. Protein Expr. Purif. 53, 9–15. doi: 10.1016/j.pep.2006.12.002
Kim, J. H., and Kang, J. C. (2015). Influence of dietary ascorbic acid on the immune responses of juvenile korean rockfish Sebastes schlegelii. J. Aquat. Anim. Health 27, 178–184. doi: 10.1080/08997659.2015.1050128
Kim, S. K., Matsunari, H., Nomura, K., Tanaka, H., Yokoyama, M., Murata, Y., et al. (2008). Effect of dietary taurine and lipid contents on conjugated bile acid composition and growth performance of juvenile Japanese flounder Paralichthys olivaceus. Fish. Sci. 74, 875–881. doi: 10.1111/j.1444-2906.2008.01602.x
Kim, S. K., Takeuchi, T., Yokoyama, M., and Murata, M. (2003). Effect of dietary supplementation with taurine, beta-alanine and GABA on the growth of juvenile and fingerling Japanese flounder Paralichthys olivaceus. Fish. Sci. 69, 242–248. doi: 10.1046/j.1444-2906.2003.00614.x
Laffel, L. (1999). Ketone bodies: a review of physiology, pathophysiology and application of monitoring to diabetes. Diabetes Metab. Res. Rev. 15, 412–426. doi: 10.1002/(SICI)1520-7560(199911/12)15:6<412::AID-DMRR72>3.0.CO;2-8
Lazado, C. C., Caipang, C. M., and Estante, E. G. (2015). Prospects of host-associated microorganisms in fish and penaeids as probiotics with immunomodulatory functions. Fish Shellfish Immunol. 45, 2–12. doi: 10.1016/j.fsi.2015.02.023
LeBlanc, J. G., Chain, F., Martín, R., Bermúdez-Humarán, L. G., Courau, S., and Langella, P. (2017). Beneficial effects on host energy metabolism of short-chain fatty acids and vitamins produced by commensal and probiotic bacteria. Microb. Cell Fact. 16:79. doi: 10.1186/s12934-017-0691-z
LeBlanc, J. G., Milani, C., de Giori, G. S., Sesma, F., van Sinderen, D., and Ventura, M. (2013). Bacteria as vitamin suppliers to their host: a gut microbiota perspective. Curr. Opin. Biotechnol. 24, 160–168. doi: 10.1016/j.copbio.2012.08.005
Li, P., Mai, K., Trushenski, J., and Wu, G. (2009). New developments in fish amino acid nutrition: towards functional and environmentally oriented aquafeeds. Amino Acids 37, 43–53. doi: 10.1007/s00726-008-0171-1
Liu, R., Hong, J., Xu, X., Feng, Q., Zhang, D., Gu, Y., et al. (2017). Gut microbiome and serum metabolome alterations in obesity and after weight-loss intervention. Nat. Med. 23, 859–868. doi: 10.1038/nm.4358
Machlin, L. J., Pearson, P. B., and Denton, C. A. (1955). The utilization of sulfate sulfur for the synthesis of taurine in the developing chick embryo. J. Biol. Chem. 212, 469–475.
Malmezat, T., Breuillé, D., Capitan, P., Mirand, P. P., and Obled, C. (2000). Glutathione turnover is increased during the acute phase of sepsis in rats. J. Nutr. 130, 1239–1246. doi: 10.1093/jn/130.5.1239
Martin, F. P., Wang, Y., Sprenger, N., Yap, I. K., Lundstedt, T., Lek, P., et al. (2008). Probiotic modulation of symbiotic gut microbial–host metabolic interactions in a humanized microbiome mouse model. Mol. Syst. Biol. 4:157. doi: 10.1038/msb4100190
Martinussen, J., Wadskov-Hansen, S. L., and Hammer, K. (2003). Two nucleoside uptake systems in Lactococcus lactis: competition between purine nucleosides and cytidine allows for modulation of intracellular nucleotide pools. J. Bacteriol. 185, 1503–1508. doi: 10.1128/JB.185.5.1503-1508.2003
Masuo, K., Kawaguchi, H., Mikami, H., Ogihara, T., and Tuck, M. L. (2003). Serum uric acid and plasma norepinephrine concentrations predict subsequent weight gain and blood pressure elevation. Hypertension 42, 474–480. doi: 10.1161/01.HYP.0000091371.53502.D3
Matsumoto, M., Kibe, R., Ooga, T., Aiba, Y., Kurihara, S., Sawaki, E., et al. (2012). Impact of intestinal microbiota on intestinal luminal metabolome. Sci. Rep. 2:233. doi: 10.1038/srep00233
Matsumoto, M., Ooga, T., Kibe, R., Aiba, Y., Koga, Y., and Benno, Y. (2017). Colonic absorption of low-molecular-weight metabolites influenced by the intestinal microbiome: a pilot study. PLoS ONE 12:e0169207. doi: 10.1371/journal.pone.0169207
Moriya, Y., Itoh, M., Okuda, S., Yoshizawa, A. C., and Kanehisa, M. (2007). KAAS: an automatic genome annotation and pathway reconstruction server. Nucleic Acids Res. 35, W182–W185 doi: 10.1093/nar/gkm321
Morris, S. M. Jr. (2009). Recent advances in arginine metabolism: roles and regulation of the arginases. Br. J. Pharmacol. 157, 922–930. doi: 10.1111/j.1476-5381.2009.00278.x
Nayak, S. K. (2010). Role of gastrointestinal microbiota in fish. Aquaculture Res. 41, 1553–1573. doi: 10.1111/j.1365-2109.2010.02546.x
Nguyen, T. L., and Kim, D. H. (2018). Genome-wide comparison reveals a probiotic strain Lactococcus lactis WFLU12 isolated from the gastrointestinal tract of olive flounder (Paralichthys olivaceus) harboring genes supporting probiotic action. Mar. Drugs 16:E140. doi: 10.3390/md16050140
Nguyen, T. L., Park, C. I., and Kim, D. H. (2017). Improved growth rate and disease resistance in olive flounder, Paralichthys olivaceus, by probiotic Lactococcus lactis WFLU12 isolated from wild marine fish. Aquaculture 471, 113–120. doi: 10.1016/j.aquaculture.2017.01.008
Noens, E. E., Kaczmarek, M. B., Zygo, M., and Lolkema, J. S. (2015). ArcD1 and ArcD2 arginine/ornithine exchangers encoded in the arginine deiminase pathway gene cluster of Lactococcus lactis. J. Bacteriol. 197, 3545–3553. doi: 10.1128/JB.00526-15
Ohashi, Y., Hirayama, A., Ishikawa, T., Nakamura, S., Shimizu, K., Ueno, Y., et al. (2008). Depiction of metabolome changes in histidine-starved Escherichia coli by CE-TOFMS. Mol. Biosyst. 4, 135–147. doi: 10.1039/B714176A
Pan, Q., Tan, Y. G., Bi, Y. Z., and Zheng, S. X. (2004). Effects of citric acid on growth, whole body composition and activities of digestive enzymes in hybrid tilapia Oreochromis niloticus × O. aureus. J. Fishery Sci. Chin. 11, 344–348.
Park, G. S., Takeuchi, T., Yokoyama, M., and Seikai, T. (2002). Optimal dietary taurine level for growth of juvenile Japanese flounder Paralichthys olivaceus. Fish. Sci. 68, 824–829. doi: 10.1046/j.1444-2906.2002.00498.x
Peng, M., Xu, W., Ai, Q., Mai, K., Liufu, Z., and Zhang, K. (2013). Effects of nucleotide supplementation on growth, immune responses and intestinal morphology in juvenile turbot fed diets with graded levels of soybean meal (Scophthalmus maximus L.). Aquaculture 392–395, 51–58. doi: 10.1016/j.aquaculture.2013.02.002
Péres, A., Cahu, C. L., and Zambonino-Infante, J. L. (1997). Dietary spermine supplementation induces intestinal maturation in sea bass (Dicentrarchus labrax) larvae. Fish. Biochem. Physiol. 16, 479–485. doi: 10.1023/A:1007786128254
Quan, R. (1992). “Dietary nucleotides: potential for immune enhancement.” in Foods, Nutrition, and Immunity. Effects of dairy and fermented milk products, eds M. Paubert-Braquet, C Dupont, R. Paoletti (Basel: Karger), 13–21.
Ramani, D., De Bandt, J. P., and Cynober, L. (2014). Aliphatic polyamines in physiology and diseases. Clin. Nutr. 33, 14–22. doi: 10.1016/j.clnu.2013.09.019
Rogge, M. M. (2009). The role of impaired mitochondrial lipid oxidation in obesity. Biol. Res. Nurs. 10, 356–373. doi: 10.1177/1099800408329408
Šabatková, J., Kumprecht, I., Zobač, P., and Suchý, P. Cermák, B. (2008). The probiotic bioplus 2B as an alternative to antibiotics in diets for broiler chickens. Acta Vet. Brno. 77, 569–574. doi: 10.2754/avb200877040569
Safari, R., Adel, M., Lazado, C. C., Caipang, C. M., and Dadar, M. (2016). Host-derived probiotics Enterococcus casseliflavus improves resistance against Streptococcus iniae infection in rainbow trout (Oncorhynchus mykiss) via immunomodulation. Fish Shellfish Immunol. 52, 198–205. doi: 10.1016/j.fsi.2016.03.020
Sakaguchi, M., and Murata, M. (1988). “Taurine,” in Extractive Components of Fish and Shellfish. ed Sakaguchi M (Tokyo: KouseishaKouseikaku), 56–65.
Salze, G. P., and Davis, D. A. (2015). Taurine: a critical nutrient for future fish feeds. Aquaculture 437, 215–229. doi: 10.1016/j.aquaculture.2014.12.006
Sarker, S. A., Satoh, S., and Kiron, V. (2005). Supplementation of citric acid and amino acid-chelated trace element to develop environment friendly feed for red sea bream, Pagrus major. Aquaculture 248, 3–11. doi: 10.1016/j.aquaculture.2005.04.012
Semova, I., Carten, J. D., Stombaugh, J., Mackey, L. C., Knight, R., Farber, S. A., et al. (2012). Microbiota regulate intestinal absorption and metabolism of fatty acids in the zebrafish. Cell Host Microbe 12, 277–288. doi: 10.1016/j.chom.2012.08.003
Shiau, S. Y., and Lin, Y. H. (2015). “Vitamins (Excluding C and E),” in Dietary Nutrients, Additives, and FISH Health, eds C. S. Lee, C. Lim, D. M. Gatlin, and C. D. Webster (Hoboken, NJ: John Wiley & Sons). doi: 10.1002/9781119005568.ch6
Shimizu-Kadota, M., Kato, H., Shiwa, Y., Oshima, K., Machii, M., Araya-Kojima, T., et al. (2013). Genomic features of Lactococcus lactis IO-1, a lactic acid bacterium that utilizes xylose and produces high levels of L-lactic acid. Biosci. Biotechnol. Biochem. 77, 1804–1808. doi: 10.1271/bbb.130080
Shoveller, A. K., Stoll, B., Ball, R. O., and Burrin, D. G. (2005). Nutritional and functional importance of intestinal sulphur amino acid metabolism. J. Nutr. 135, 1609–1612. doi: 10.1093/jn/135.7.1609
Smit, B. A., Engels, W. J., Wouters, J. T., and Smit, G. (2004). Diversity of L-leucine catabolism in various microorganisms involved in dairy fermentations, and identification of the rate controlling step in the formation of the potent flavour component 3-methylbutanal. Appl. Microbiol. Biotechnol. 64, 396–402. doi: 10.1007/s00253-003-1447-8
Song, J. W., Lim, S. J., and Lee, K. J. (2012). Effects of dietary supplementation of inosine monophosphate on growth performance, innate immunity and disease resistance of olive flounder (Paralichthys olivaceus). Fish Shellfish Immunol. 33, 1050–1054. doi: 10.1016/j.fsi.2012.07.011
Song, W., Sun, X., Chen, X., Liu, D., and Liu, L. (2015). Enzymatic production of L-citrulline by hydrolysis of the guanidinium group of L-arginine with recombinant arginine deiminase. J. Biotechnol. 208, 37–43. doi: 10.1016/j.jbiotec.2015.05.012
Tevatia, R., Allen, C., Rudrappa, D., White, D., Clemente, T. E., Cerutti, H., et al. (2015). The taurine biosynthetic pathway of microalgae. Algal. Res. 9, 21–26. doi: 10.1016/j.algal.2015.02.012
Torell, F., Bennett, K., Cereghini, S., Rännar, S., Lundstedt-Enkel, K., Moritz, T., et al. (2015). Multi-organ contribution to the metabolic plasma profile using hierarchical modelling. PLoS ONE 10:e0129260. doi: 10.1371/journal.pone.0129260
Townsend, J. H., Davis, S. R., Mackey, A. D., and Gregory, J. F. 3rd (2004). Folate deprivation reduces homocysteine remethylation in a human intestinal epithelial cell culture model: role of serine in one-carbon donation. Am. J. Gastrointest. Liver Physiol. 286, G588–G595. doi: 10.1152/ajpgi.00454.2003
Turer, E., McAlpine, W., Wang, K. W., Lu, T., Li, X., Tang, M., et al. (2017). Creatine maintains intestinal homeostasis and protects against colitis. Proc. Natl Acad. Sci. U.S.A 114, E1273–E1281. doi: 10.1073/pnas.1621400114
Wahl, S., Vogt, S., Stückler, F., Krumsiek, J., Bartel, J., Kacprowski, T., et al. (2015). Multi-omic signature of body weight change: results from a population-based cohort study. BMC Med. 13:48. doi: 10.1186/s12916-015-0282-y
Wang, X., He, G., Mai, K., Xu, W., and Zhou, H. (2016). Differential regulation of taurine biosynthesis in rainbow trout and Japanese flounder. Sci. Rep. 6:21231. doi: 10.1038/srep21231
Wikoff, W. R., Anfora, A. T., Liu, J., Schultz, P. G., Lesley, S. A., Peters, E. C., et al. (2009). Metabolomics analysis reveals large effects of gut microflora on mammalian blood metabolites. Proc. Natl Acad. Sci. U.S.A. 106, 3698–3703. doi: 10.1073/pnas.0812874106
Wu, G. (1998). Intestinal mucosal amino acid catabolism. J. Nutr. 128, 1249–1252. doi: 10.1093/jn/128.8.1249
Xie, B., Waters, M. J., and Schirra, H. J. (2012). Investigating potential mechanisms of obesity by metabolomics. J. Biomed. Biotechnol. 2012:805683. doi: 10.1155/2012/805683
Yagci, N., Cokgor, E. U., Artan, N., Randall, C., and Orhon, D. (2007). The effect of substrate on the composition of polyhydroxyalkanoates in enhanced biological phosphorus removal. J. Chem. Technol. Biotechnol. 82, 295–303. doi: 10.1002/jctb.1672
Keywords: probiotics, olive flounder, fish, growth promotion, metabolome, metabolite, CE-TOFMS
Citation: Nguyen TL, Chun W-K, Kim A, Kim N, Roh HJ, Lee Y, Yi M, Kim S, Park C-Il and Kim D-H (2018) Dietary Probiotic Effect of Lactococcus lactis WFLU12 on Low-Molecular-Weight Metabolites and Growth of Olive Flounder (Paralichythys olivaceus). Front. Microbiol. 9:2059. doi: 10.3389/fmicb.2018.02059
Received: 20 May 2018; Accepted: 13 August 2018;
Published: 05 September 2018.
Edited by:
Dimitris G. Hatzinikolaou, National and Kapodistrian University of Athens, GreeceReviewed by:
Maryam Dadar, Razi Vaccine and Serum Research Institute, IranAtte Von Wright, University of Eastern Finland, Finland
Copyright © 2018 Nguyen, Chun, Kim, Kim, Roh, Lee, Yi, Kim, Park and Kim. This is an open-access article distributed under the terms of the Creative Commons Attribution License (CC BY). The use, distribution or reproduction in other forums is permitted, provided the original author(s) and the copyright owner(s) are credited and that the original publication in this journal is cited, in accordance with accepted academic practice. No use, distribution or reproduction is permitted which does not comply with these terms.
*Correspondence: Chan-Il Park, dmludXM5NkBoYW5tYWlsLm5ldA==
Do-Hyung Kim, ZGhraW1AcGtudS5hYy5rcg==