- Laboratory of Molecular and Cellular Biology, Center of Nuclear Energy in Agriculture, University of São Paulo, Piracicaba, Brazil
Arbuscular mycorrhizal fungi (AMF) colonization in plants promotes both local and systemic changes in the gene expression profiles of the host that might be relevant for drought-stress perception and response. Drought-tolerant common bean plants (cv. BAT 477), colonized by a mixture of AMF (Glomus clarum, Acaulospora scrobiculata, and Gigaspora rosea), were exposed to a water deprivation regime of 96 h during pre-flowering. Root transcriptomes were accessed through RNA-Seq revealing a set of 9,965 transcripts with significant differential regulation in inoculated plants during a water deficit event, and 10,569 in non-inoculated. These data include 1,589 transcripts that are exclusively regulated by AMF-inoculation, and 2,313 under non-inoculation conditions. Relative gene expression analyses of nine aquaporin-related transcripts were performed in roots and leaves of plants harvested at initial stages of treatment. Significant shifts in gene expression were detected in AM water deficit-treated roots, in relation to non-inoculated, between 48 and 72 h. Leaves also showed significant mycorrhizal influence in gene expression, especially after 96 h. Root cortical cells, harboring or not arbuscules, were collected from both inoculation treatments through a laser microdissection-based technique. This allowed the identification of transcripts, such as the aquaporin PvPIP2;3 and Glucan 1,3 β-Glucosidase, that are unique to arbuscule-containing cells. During the water deficit treatment, AMF colonization exerted a fine-tune regulation in the expression of genes in the host. That seemed to initiate in arbuscule-containing cells and, as the stressful condition persisted, propagated to the whole-plant through secondary signaling events. Collectively, these results demonstrate that arbuscular mycorrhization leads to shifts in common bean’s transcriptome that could potentially impact its adaptation capacity during water deficit events.
Introduction
The great majority of plants are capable of establishing symbiotic relationships with arbuscular mycorrhizal fungi (AMF) that colonize root cortical cells forming intracellular structures named arbuscules (Parniske, 2008). AMF form an extensive hyphal network in the soil that heads through the roots depletion zone, therefore becoming able to acquire nutrients (mainly phosphates) and water in a more efficient way than the root system alone (Smith and Smith, 1990; Rapparini and Peñuelas, 2014). In return, AMF take up photosynthetically fixed carbon from plants and translocate it to their external mycelium mainly through synthesized lipid and glycogen (Bago et al., 2003). Besides, the ancient 400 million years’ history of co-evolution (Redecker et al., 2000), combined with the ability of around 80% of angiosperms to sustain this mutualistic relationship, suggests that plants might be able to sustain a wide system of genetic reprogramming related to the endosymbiotic process (Balestrini et al., 2007; Fiorilli et al., 2009; Gomez et al., 2009; Guether et al., 2009; Hogekamp et al., 2011; Gaude et al., 2012; Oldroyd et al., 2014).
Long-term climate changes have led to a global scale increase in the number of drought stress events in plants, and together with the constant population growth and consequent expansion of agricultural practices into marginal areas, have been generating more unstable production, therefore, severely diminishing food stocks all over the world (Parry et al., 2007). Water deficit is one of the most common abiotic stress factors that affect plants, directly interfering on its normal development and growth and having a major adverse effect on productivity (Colmenero-Flores et al., 1997). The development of new technologies to engineer drought tolerance in plants has huge economic importance (Umezawa et al., 2006) and the use of AMF inoculation on annual cultures with the objective of improving plants properties has emerged in the last few years as a more sustainable practice (Azcón-Aguilar and Barea, 1997; Barea et al., 2005; Cozzolino et al., 2013).
The possibility of increasing drought tolerance in plants through AMF inoculation has been extensively investigated (Augé, 2001; Bárzana et al., 2014, 2015; Rapparini and Peñuelas, 2014; Saia et al., 2014). The symbiosis protects plants against the adverse impacts of water deficit mainly through the maintenance of leaf water potential, relative water content (RWC), stomatal conductance, CO2 assimilation, photosystem II efficiency, and transpiration (Augé, 2001; Ruiz-Lozano, 2003; Sánchez-Blanco et al., 2004; Bárzana et al., 2012; Lee et al., 2012). This is achieved through a better nutritional status, that secures a more sustainable physiology throughout plant development, and the induction of drought avoidance mechanisms consisting on the preservation of an adequate plant hydration status that helps keeping constant cellular turgor pressure on leaves during low water potential (Augé, 2001; Augé et al., 2004; Rapparini and Peñuelas, 2014). Additionally, the hyphal natural characteristics, such as a diameter twice smaller the average root size (2–5 μm), allows the fungus to extend its network throughout the water depletion zones and inside thinner pores that might retain water through the dryness process (Ruiz-Lozano, 2003; Miransari et al., 2007; Rapparini and Peñuelas, 2014).
The interaction with AMF may result in both local and global changes in the expression profiles of genes that are vital for both biotic and abiotic resistance responses in plants (Porcel et al., 2012). Molecular techniques have been applied, and a significant number of transcripts have been described as differentially regulated in AM plants during water deficit. Two Δ-pyrroline-5-carboxylate synthetase genes, gmp5cs from Glycine max e lsp5cs from Lactuca sativa, and three genes encoding for dehydrins, gmlea8 and gmlea10 (G. max) and lslea 1 (L. sativa), were down-regulated in the roots of the respective plants under AM inoculation (Porcel and Ruiz-Lozano, 2004; Porcel et al., 2005). In Phaseolus vulgaris roots colonized by Glomus intraradices, drought treatment increased the expression of the aquaporin PvPIP1;1 gene, drastically diminished the expression of the PvPIP1;2 and PvPIP1;3 genes, and did not change the expression of the PvPIP2;1 gene. Non-AM control plants revealed higher gene expression of the analyzed transcripts during drought, except for PvPIP1;2 (Aroca et al., 2006).
Despite common bean has great importance in human populations diet of developing regions such as Latin America and Africa, it is still a low scale production culture, mostly performed in small-farming systems, with minimum technological input, being 60% of it relegated to regions that suffer with continuous or intermittent drought events during the year (CIAT, 1989; Thung and Rao, 1999). The highly diverse conditions under which common beans are cultivated, combined with local preferences for specific seed traits, have led to difficulties in establishing efficient breeding programs that are able to combine both improved performances under drought stress with enhanced growth and production (Graham and Ranalli, 1997; Beebe et al., 2009).
BAT 477, a type III-growth habit drought-resistant line, has deep rooting ability and high water absorption efficiency (Sponchiado et al., 1989), serving well as a biological model for drought tolerance in common bean studies. Terminal drought simulation studies in soil tubes indicated that BAT 477 has the ability to grow tap roots under drought conditions and inhibit lateral root growth (Rao et al., 2006). Although great advance has been made in root development and architecture, soil fertility in tropical soils is poor, mainly regarding phosphorous availability, and this factor can limit vegetative development and root growth (Thung and Rao, 1999). Therefore, since a shallow and abundant root system is more effective for nutrient acquisition, and a deep root system is more efficient for water absorption, the combination of tolerance with these two traits can be a great challenge (Beebe et al., 2009). Thus, the more efficient establishment of symbiotic relationships with soil microbiome in the roots, such as AMF, may provide an interesting strategy for overcoming this issue.
Since common beans have a simpler genomic structure (2n = 22), the culture fits well as a model for other more complex tropical legume crops, like soybean (G. max) (Galeano et al., 2008; McConnell et al., 2010). In this study, the symbiotic interaction established between common bean plants (BAT 477, drought-tolerant) and a mixture of three AMF strains (G. clarum, A. scrobiculata, and G. rosea) was explored to further evaluate the influence exerted by the colonization process over plants transcription profiles of important genes related to drought-stress response and adaptation. A whole-transcriptome sequencing (RNA-Seq) approach was adopted, and after careful statistical comparisons, provided an interesting set of candidate genes for a more detailed characterization of our model of study. A time-course experiment was conducted, enabling the detection of shifts in the relative expression of nine aquaporins, as evaluated through RT-qPCR considering whole-roots and -leaves harvested at 24, 48, 72, and 96 h of both water deficit and inoculation treatments. This step allowed the identification of a mycorrhizal influence over common bean transcriptome that varies both at a time and spatial configuration. Moreover, root cortical cells (harboring or not arbuscules) were obtained from water deficit treated plants through laser-capture microdissection microscopy (LCM), and the relative expression of 21 transcripts was compared between the different cell populations. This provided additional evidences for the occurrence of a site-specific modulation mechanism of the host transcriptome during AMF colonization.
Materials and Methods
Biological Material and Water Deficit Assay
BAT 477 seeds were superficially sterilized by rinsing them for 1 min in sodium hypochlorite 10% and washing three times with distilled water. Open-pot cultures were obtained by growing Brachiaria ruziziensis plants in a sand:vermiculite (2:1) substrate inoculated with the AMF mixture. The mycorrhizal inoculum for each endophyte (G. mossae, A. scrobiculata, and G. rosea) consisted of 50 ml soil containing spores, mycelium, and 75% infected root fragments obtained from open-pot cultures of Brachiaria decubens.
BAT 477 seeds were placed in 8 liter pots containing a mixture of sand:vermiculite (2:1), both pre-washed and sterilized at 120°C and 1 atm for 30 min. AMF inoculation was performed with 50 ml of a mixture containing an average 30 spores per gram, mycelia and hyphal structures of the AMF mixture obtained from the open-pot culture; non-inoculated treatments received 50 ml of a pre-sterilized mixture.
Experiments were conducted at greenhouse conditions (24–27°C). Four treatments with 18 replicates were applied: (i) AMF-inoculated plants under water deficit (Myc+/Strss); (ii) AMF-inoculated plants with regular irrigation (Myc+/Ctrl); (iii) non-inoculated plants under water deficit (Myc-/Strss); and (iv) non-inoculated plants with regular irrigation (Myc-/Ctrl). Plants were kept under a regular irrigation regime of approximately 200 ml distilled deionized water every 2 days. Irrigation was performed in order to keep plants at 80% of field capacity, which corresponds to 21–24% of soil moisture content that was monitored using a digital thermo-hygrometer equipped with a sensing probe.
Irrigation was kept constant until plants reached the pre-flowering developmental stage (R5), when plants dependence under stored soil–water intensifies and effects of terminal drought on subsequent flowering and pod-filling becomes more limiting (Rosales-Serna et al., 2004). Water deficit treated plants had water supply fully suspended during 96 h, thus simulating a severe water deficit event. Soil water moisture content (%) was monitored during the whole period. Three applications of nutrient solution (200 ml) were performed, after 10, 17, and 21 days, respectively, following Hoagland’s (Hoagland and Arnon, 1950) formulation. Inoculated plants received a modified formulation (0.25 mmol/l of KH2PO4 solution, added 1 mmol/l KNO3) in order to prevent an impairment in root colonization due to a high phosphate concentration (Balzergue et al., 2013). Plants harvestings, consisting of three randomly chosen biological replicates from each treatment, started after 24 h of water deficit treatment, being repeated after 48, 72, and 96 h.
After 96 h, from the nine biological replicates left in each treatment, three were randomly selected for water deficit assay validation, three were stored at -80°C for total RNA extraction and three applied for microscopy. Following, gas-exchange measurements were conducted in fully expanded intermediate leaves of three plants of each treatment (between 11:00 a.m. and 01:30 p.m.) with a gas-exchange system (IRGA LI-6400XT; Li-Cor). Leaves were first equilibrated at a photon density flux of 1000 mmol m-2 s-1 for at least 20 min. After this, photosynthesis was induced with 1000 mmol photons m-2 s-1 and ∼386 mmol mol-1 CO2 surrounding the leaf. Leaf temperature was maintained at 28°C, and the leaf-to-air vapor pressure deficit was kept between 1 and 1.3 kPa. These conditions were kept constant for the determination of net photosynthetic rate (AN), transpiration rate (E), stomatal conductance (gs), and water-use efficiency (WUE). WUE was calculated as the ratio between photosynthesis rate and stomatal conductance (AN/gs) (Dewar, 1997).
Moreover, three completely and recently expanded trifoliates from each plant were harvested and weighed for RWC determination according to Barrs and Weatherley’s (1962) instructions and following the equation: RWC (%) = [(Fw-Dw)]/[(Tw-Dw)] ∗ 100.
The root length density (RLD), the length of roots per unit volume of substrate, was measured by placing the whole root organs (three biological replicates for each treatment) on a board covered by a squared paper (1 × 1 cm). Boards were scanned, and length measurements were taken using ImageJ 1.46r software1. RLD was obtained by the average length of root segments (cm) proportional to the volume occupied by the substrate in the respective pot (cmł) – given by the calculation of the volume of a circular truncated cone. Total leaf and root dry mass (LDM and RDM) were determined by drying samples in a ventilated oven at 60°C (∼48 h) until reaching constant weight.
Mycorrhizal colonization rates MCR(%) were estimated from 1 g of roots obtained from each triplicate and treatment and estimated following previous protocol (Brundrett et al., 1996), using a stereo microscope system (Leica EZ4 HD – Leica Microsystems).
The JMP – Statistical DiscoveryTM from SAS software was used for an analysis of variance (ANOVA) to discriminate the effects of the water deficit event and AMF (+ or -) treatments. To perform the ANOVA, we also acknowledged prior steps toward the verification of normality and variance homogeneities of the data. Significant differences encountered through the ANOVA (p < 0.05) were further accessed for mean comparisons among the treatment combinations, using Tukey’s test with Laercio package from R (v.i.3.2.2)2.
Sample Collection and RNA Preparation for RNA-Seq Analysis
Whole root tissues were retrieved from the last three biological replicates in each treatment, ground in liquid nitrogen, and stored at -80°C prior to RNA extraction. Total RNA was isolated with Trizol (Trizol Reagent® LS, Invitrogen) following manufacturer’s recommendations. RNA samples were quantified and assessed for quality and molecular integrity using the RNA 6000 Nano LabChip® Kit (Agilent Technologies) through the Agilent 2100 Bioanalyzer (Agilent Technologies) system following manufacturer’s instructions. RNA samples were prepared for sequencing using the TruSeqTM RNA Sample Preparation Kit v2 (Illumina® Sequencing). The library clusterization was conducted using TruSeq® PE Cluster Kit v3 (para cBot – HiSeq/HiScan SQ) and sequencing was performed with TruSeqTM SBS kit v3 kit (for HiSeq/HiScan SQ) following manufacturer’s instructions in a HiScanTM Sq System (Illumina®).
Sequences Assembly and Data Analysis
Paired-end sequencing raw data were obtained through the CASAVA 1.8.2 (Illumina®) software. Reads were trimmed for adaptors and vectors sequences and purified from low-quality sequences using the SeqyClean v.1.9.7 software3 with cutoff of 24QScore and Univec database for contaminants4. Transcriptome mapping was conducted using the common bean reference genome data publicly available on Phytozome database v.11.15 (Schmutz et al., 2014). Paired-end filtered reads are available at NCBI’s Sequence Read Archive Public Database6, individual temporary submission IDs are available (Supplementary Table S1).
Reference genomes were indexed using Bowtie 2 v.2.1.0 software tool (Langmead and Salzberg, 2012). Individual mappings of the samples were achieved following Bowtie2 v2.1.0 software package taking indexed genomes and individual annotation files (public available by the Joint Genome Institute7) as references. The transcript assembly was achieved following the Cufflinks v2.1.1 package tools pipeline using the RABT option (Trapnell et al., 2010). The individual assemblies were merged using the function cuffmerge from Cufflinks. Raw reads abundance estimation counts were obtained through HTSeq-count v.05.4.p2 script8 through the annotation file obtained from Cufflinks assembly.
Differentially expressed genes (DEGs) between the optimal irrigation and water deficit treated samples, as well as inoculated and non-inoculated plants both under water deficit, were identified using the DESeq2 package from R/Bioconductor (Love et al., 2014). The -in function “estimate Size Factors” was used to obtain the normalized counts, i.e., baseMean values, which are the number of reads divided by the size factor or normalization constant. Transcripts with baseMean less than five considering all samples were removed to avoid artifacts due to low coverage. The method used to test for differential expression was the negative binomial distribution, followed by the FDR correction (<0.05) (Benjamini and Hochberg, 1995).
Putative DEGs transcripts annotation were obtained through blastx function with cutoff of 1e-10 using the blast+ package9. The green plant public database of NCBI10 was used as reference. Functional classification inside Gene Ontology terms11 and Pfam was obtained through Blast2GO software (Conesa et al., 2005). Hit files obtained through blastx were annotated by the GOSlim function followed by the enrichment of genes inside the most representative ontology terms in each of the four treatments using a Fisher-2 tailed test with FDR (<0.01) through Blast2GO software. Briefly, the four sets of DEGs retrieved from each differential expression statistical analysis were compared against the complete set of annotated transcripts of common bean. For those ontology terms where the number of represented DEGs were superior to that of the reference set, the term was considered over-represented for that particular treatment; and for those terms where the number of represented DEGs were inferior, it was considered under-represented.
Heat maps were constructed based on the means of normalized read counts assigned to individual transcripts in each sample after differential gene expression analysis. The Heatmap.2 function from gplots (v 2.12.1) R package (R i386 3.2.0) was used for designing the heat maps.
Temporal Analysis of Aquaporin-Genes Expression: RNA Extraction, RT-qPCR Assay, and Data Analysis
Total RNA was extracted from leaves and roots harvested in all time-periods of water deficit and control treatments described before. RNA extraction was performed by following the TRIzol® Reagent kit (Invitrogen) manufacturer’s instructions from 50 mg of grinded frozen tissues as described previously. Total RNA extract was diluted in 20 μl 0.1% DEPC-water. The RNA samples were quantified by spectrophotometry at 260 and 280 nm wavelengths using NanoDropTM 2000c, and RNA quality was verified by electrophoresis on a 1.6% agarose gel in TAE buffer. Purified total RNA was guaranteed by carrying out DNAse treatment using a DNAse1 RNAse-free kit (Fermentas), following the manufacturer’s instructions. For RT-qPCR assay, 100 ng of DNAse-treated total RNA was used for cDNA synthesis using the MaximaTM First Strand cDNA Synthesis Kit for two step RT-qPCR kit (FermentasTM) following the manufacturer’s guidance.
Reactions were carried out on a StepOnePlusTM Real Time PCR System (Applied Biosystems) using the Maxima® SYBR Green/ROX qPCR Master Mix kit (FementasTM), following manufacturer’s instructions. Each reaction was prepared to a final volume of 10 μl: 0.25 μM of each primer, 100 ng of cDNA, 2× of SYBR Green Mix. R reactions conditions: 10 min at 42°C; 10 min at 95°C; 40 cycles of cDNA amplification for 15 s at 95°C, 20 s at 59°C, and 30 s at 72°C with fluorescent signal recording. At the end, a final step of 15 s at 95°C, 1 min at 60°C, and fluorescence measured at each 0.7°C variation (from 60 to 95°C). Three reference genes were validated for our conditions and set as internal normalizers for relative expression assays, skip16 and IDE for roots, and Ubq and IDE for leaves (Borges et al., 2012). No-template control reactions were performed for each pair of primers by omitting cDNA template and completing the final volume of the reaction with water. Experimental design consisted of three technical replicates for each biological triplicate (3 × 3) per cell-type analyzed and amplified transcript.
Raw data (not baseline corrected) of fluorescence levels were submitted to LinRegPCR software (Ruijter et al., 2009), for baseline correction and linear regression analysis on each amplification curve. The optimal set of data points (window-of-linearity) was defined to allow the calculation of the threshold and quantification cycle (Cq) values. Furthermore, the efficiency was calculated based on slope of the line (E = 10slope), considering an ideal value range (1.8 ≤E ≤ 2) and correlation (R ≥ 0.995).
Relative expression data were obtained by REST software (Pfaffl, 2001) using average values of efficiency and Cq of target and reference genes. This software compares control and treatment Cq values to obtain the concentration of expression (C), where C = EmeanCq(Control) -meanCq(treatment); then, it calculates the relative expression (RE) ratio where RE = Ctarget gene/geometric average Creference gene; and performs a pairwise fixed reallocation randomization test (bootstrap = 2,000 permutation) to obtain p-values.
Sample Preparation for Light-Microscopy Visualization
Root segments (1 cm) were harvested from 96 h AMF-inoculated water deficit event treated plants (Myc+/Strss) and fixed in a Karnovsky modified solution (2% glutaraldehyde, 2% paraformaldehyde, 1 mM CaCl2, 50 mM sodium cacodylate pH 7.2) at 4°C for 48 h. After, samples were dehydrated in ascending ethanol series (35–100%) and infiltration was performed slowly in ethanol. Samples were embedded in Historesin (HistoResin Mounting Media kit – Leica Helderberg) following manufacturer’s instructions. Tissue section (5 μm thick) were obtained in a RM2155 microtome (Leica® Germany) and mounted in glass blades. Staining was conducted as follows: 2 min in pre-heated (40°C) 1% acid-Fuchsin, washed in tap water, 5 min in 0.05% Toluidine-blue, and washed in tap-water. Tissue sections were observed in a Leica LMD 7000 (Leica Microsystems®) microscope and images were processed using LAS.v.3.8 software.
Microscope-Based Laser-Capture Microdissection and Total RNA Extraction
Root fragments (1 cm) were collected from the three last biological replicates of both 96 h water deficit treatments (Myc+/Strss and Myc-/Strss) and fixed in 10% paraformaldehyde solution (diluted in 0.1% DEPC-treated distilled water) at 4°C for 24 h. Root fragments were retrieved from the same three biological replicates selected for the MCR(%) analysis. Transversal root sections were paraffin embedded following (Ludwig and Hochholdinger, 2014) protocol. Sections were dehydrated in an ethanol graded series (70, 80, 90, and 100%), followed by an ethanol and xylol series (3:1; 1:1; 1:3; and 100% ethanol) for 4 h at room temperature; samples were embedded through a gradient of dehydration solution and paraffin (3:1; 1:1; 100% paraffin) at 58°C by 4 h each. Transversal histological sections were 10 μm thick and mounted into PEN slides (Leica Microsystems®) and stored at -80°C. A minimum set of 24 blades were mounted for each biological triplicate consisting of root segments harvest from three different plants and relative to each treatment, Myc+/Strss and Myc-/Strss (24 × 3 × 2).
Prior to laser microdissection, slides were gradually thawed (20 min at -20°C and 15 min at 4°C), followed by sample paraffin removal through dehydration at room temperature consisting of total immersion on 100% xylol for 10 min, 1 min in 100% ethanol, additional ethanol graded series (95% and 70%) for 30 s each and 1 min in 0.01% DEPC-treated distilled water. Staining followed a modified version of Feder and O’Brien (1968) protocol by immersion on 1% acid-Fuchsin solution pre-heated at 40°C for 2 min, washed in water, dropped in 0.05% Toluidine blue solution for 5 min, and again washed in water. A second dehydration step was performed by dropping samples into 95% ethanol for 90 s, 100% ethanol for 1 min, and 100% xylol for 10 min.
Laser microdissection was performed using Leica LMD 7000 (Leica Microsystems®). Three cell populations were assessed for each biological triplicate: ctx+ – root cortical cells containing arbuscules and/or hyphal structures derived from Myc+/Strss treatments, ctx- – root neighboring cortical cells not containing arbuscules derived from Myc+/Strss treatments; and ctrl – root cortical cells derived from Myc-/Strss treatments (Figure 1). Each laser microdissection session consisted of around 1 million μm2 sections for each population type.
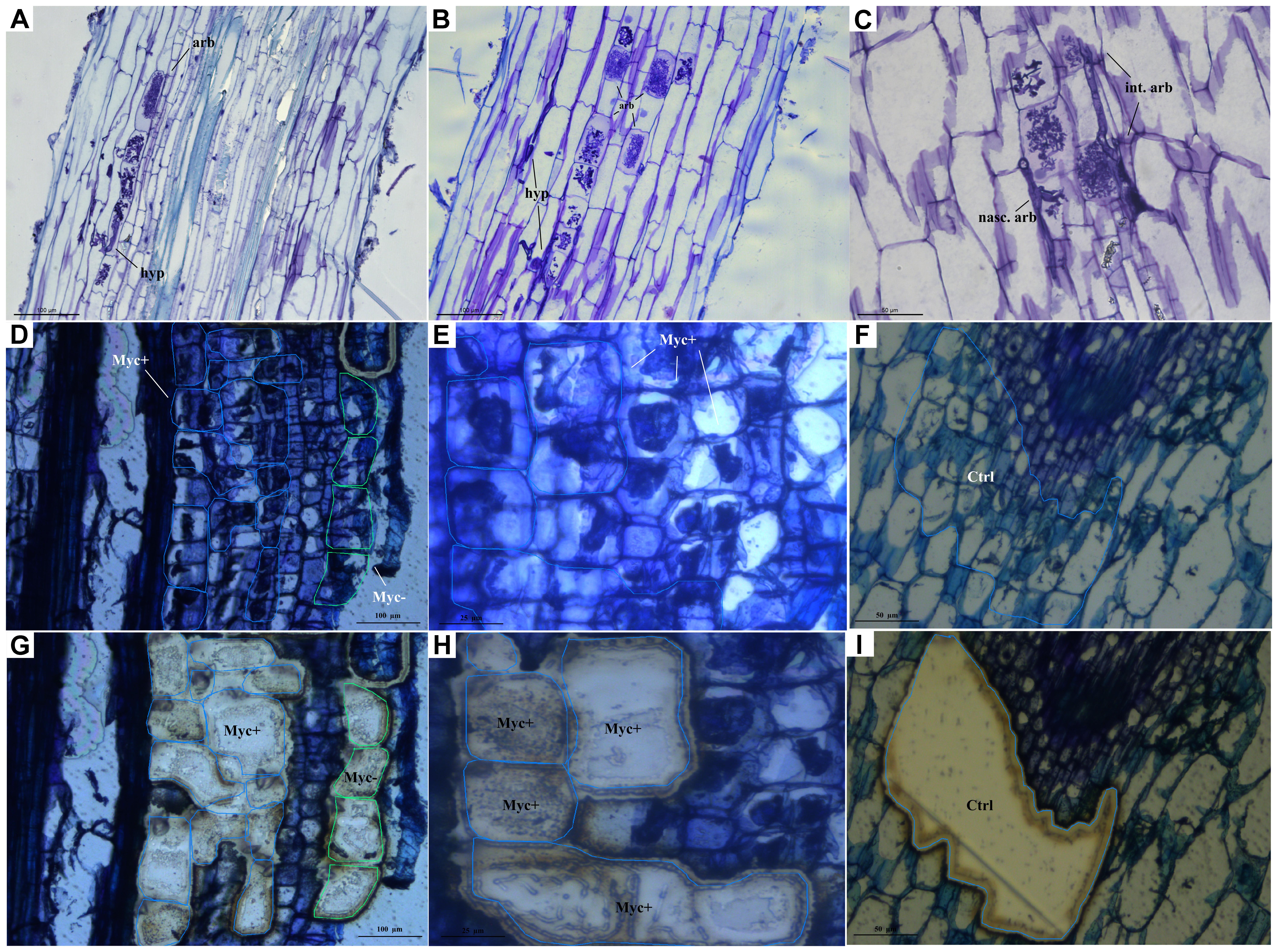
FIGURE 1. Histological section analysis. (A–C) Light microscopy of Historesin-embedded sections of water deficit treated AMF-inoculated roots. (D–I) Microscope-based laser-capture microdissection. (D) Arbusculated root-cortical cells (blue line) and neighboring non-arbusculated cortical cells (green line). (E) Arbusculated root cortical cells. (F) Root cortical cells from non-inoculated plants. (G, H) Tissue sections from AMF-inoculated water deficit treated roots after dissection. (I) Tissue sections from non-inoculated water deficit treated roots after dissection. arb, arbuscules; hyp, AMF hyphal structures; int. arb, interconnected arbuscules; nasc. arb, nascent arbuscules; Myc+, arbusculated root cortical cells; Myc–, non-arbusculated neighboring cortical cells; ctrl, root cortical cells from non-inoculated roots.
Total RNA extraction was performed using the Arcturus® PicoPure® RNA Isolation kit (Applied BiosystemsTM) following manufacturer’s instructions. For each cell type, the average amount (ng/μl) and quality (λ) of total RNA extracted was: ctx+ – 15.14 ng/μl and 1.85 λ; ctx- – 12.96 ng/μl and 1.79 λ; ctrl – 13.6 ng/μl and 1.83 λ (data not shown). Purified total RNA was guaranteed by carrying out DNAse treatment using a DNAse1 RNAse-free kit (Fermentas), following the manufacturer’s instructions. For RT-qPCR assay, 100 ng of DNAse-treated total RNA was used for cDNA synthesis using the MaximaTM First Strand cDNA Synthesis Kit for two step RT-qPCR kit (FermentasTM) following the manufacturer’s guidance.
RT-qPCR Primers Design
For temporal differential gene expression analysis, a total of nine aquaporin transcripts were selected: PvTIP1;1, PvPIP1;1, PvPIP1;2, PvPIP1;3, PvPIP1;5, PvPIP2;1, PvPIP2;3, PvPIP2;5, and PvPIP2;6. Primers for PvPIP1;2, PvPIP1;1, and PvPIP2;5, respectively, homologous to PvPIP1;1, PvPIP1;3, and PvPIP2;1 were retrieved from Aroca et al. (2007). The six other transcripts were chosen based on sequence homology to previously described plant’s aquaporins responsive to AM-inoculation or to a water deficit event stress response (Uehlein et al., 2007; Alexandersson et al., 2010; Recchia et al., 2013).
For LCM/RT-qPCR analysis a set of 23 common bean transcripts were selected among the most up- and down-regulated DEGs contrasting Myc+/Strss and Myc-/Strss treatments. PRIMER 3 software (version 0.4.012) was used for primers design considering the following parameters: product size range of 100–200 bp; primer size of 18–22 bp; primer Tm of 57–63°C. NetPrimer software was used for quality check13. The 23 primer pairs are described in Supplementary Table S2.
Endogenous Control
The endogenous control was based on PCR amplification of AML1–AML2 primers described by Lee et al. (2008). This pair of primers targets a small subunit rRNA gene and was designed to amplify all subgroups of AMF (Glomeromycota), but excluding sequences from other organisms, therefore allowing rapid identification of potential AM genomic-DNA contamination in ctx- and ctrl cell samples. Total DNA was extracted from the three tissue samples using QIAamp DNA Micro Kit (QIAGEN®) following manufacturer’s instructions. AML1–AML2 PCR amplification reactions were conducted as follows: 10× of PCR buffer, 50 mM of MgCl2 (50 mM), 2.5 mM of dNTP’s mix, 2.5 mM of each primer, 20 ng/μl of cDNA; 0.2 μl of Taq Polymerase (50U/1 μl), in a 25 μl final volume; the amplification conditions were: 2 min at 94°C, 30 cycles of 30 s at 94°C, 40 s at 58°C, 55 min at 72°C; 5 min at 72°C. The products from amplification were visualized in a 1.2% agarose gel in 1× TSB buffer.
RT-qPCR Assay and Data Analysis
Reactions were carried out on a StepOnePlusTM Real Time PCR System (Applied Biosystems) using the Maxima® SYBR Green/ROX qPCR Master Mix kit (FementasTM), following manufacturer’s instructions. Reactions were prepared for a 10 μl final volume: 0.25 μM of each primer, 100 ng of cDNA, and 2× of SYBR Green Mix. Reactions conditions: 10 min at 42°C, 10 min at 95°C, 40 cycles of cDNA amplification for 15 s at 95°C, 20 s at 59°C, 30 s at 72°C with fluorescent signal recording. At the end, a final step of 15 s at 95°C, 1 min at 60°C, and fluorescence measured at each 0.7°C variation (from 60 to 95°C) was included to obtain the melting curve. Two reference genes were validated for our conditions and set as internal normalizers for relative expression assays, skip16 and IDE (Borges et al., 2012). No-template control reactions were performed for each pair of primers by omitting cDNA template and completing the final volume of the reaction water. The experimental design consisted of three technical replicates for each biological triplicate (3 × 3) per cell-type analyzed and amplified transcript. Base line correction and linear regression of raw data, quantification cycle (Cq) values and efficiency calculations, and relative expression analysis were performed as previously described for time-shift aquaporin gene expression analysis.
Results
Plant Growth and Physiological Parameters
Soil moisture content (%) was monitored throughout the whole 96 h of water deprivation treatment (Supplementary Figure S1a). Pots from Myc+/Ctrl plants indicated a slightly superior soil moisture content (∼22.9%) than the Myc-/Ctrl (∼20.9%). For both Myc+/Strss and Myc-/Strss treatments, soil moisture content decreased during the whole period ranging from 21 to 6% for Myc+, and from 21.2 to 4.23% for Myc-. Roots mycorrhizal colonization rates (MCR%) (Supplementary Figure S1b) indicated a significant statistical difference (p < 0.05) between Myc+/Ctrl and Myc+/Strss, with 55.74% colonization for non-stressed control AM plants and 74.44% in water deficit treated AM plants.
The RWC quantification was performed to measure the plant water status of treated and control plants in terms of the physiological consequences of cellular water deficit. Statistical analysis of RWC data was able to distinguish the two groups, control and water deficit event treatments; however, no effect of AMF colonization was detected (Figure 2A). Additionally, plant growth parameters were accessed to further characterize the impact of AM-root colonization over common bean response and the adaptation to a water deficit event. The dry matter content of total leaves (LDM) and roots (RDM) was calculated. Regarding LDM, Myc+/Ctrl plants presented the highest dry matter accumulation (2.5 g), with no statistical differences detected among Myc+/Strss plants and both no-inoculated treated and control plants (Figure 2B). For RDM, no statistical significant differences were detected among the results (Figure 2C). RLD, the length of roots per unit volume of substrate, was measured with no statistical significant changes found between inoculated and non-inoculated plants, the only exception regarding a slightly increase in Myc+/Ctrl measurements (∼7.4 × 10-4 cm-2) (Figure 2D).
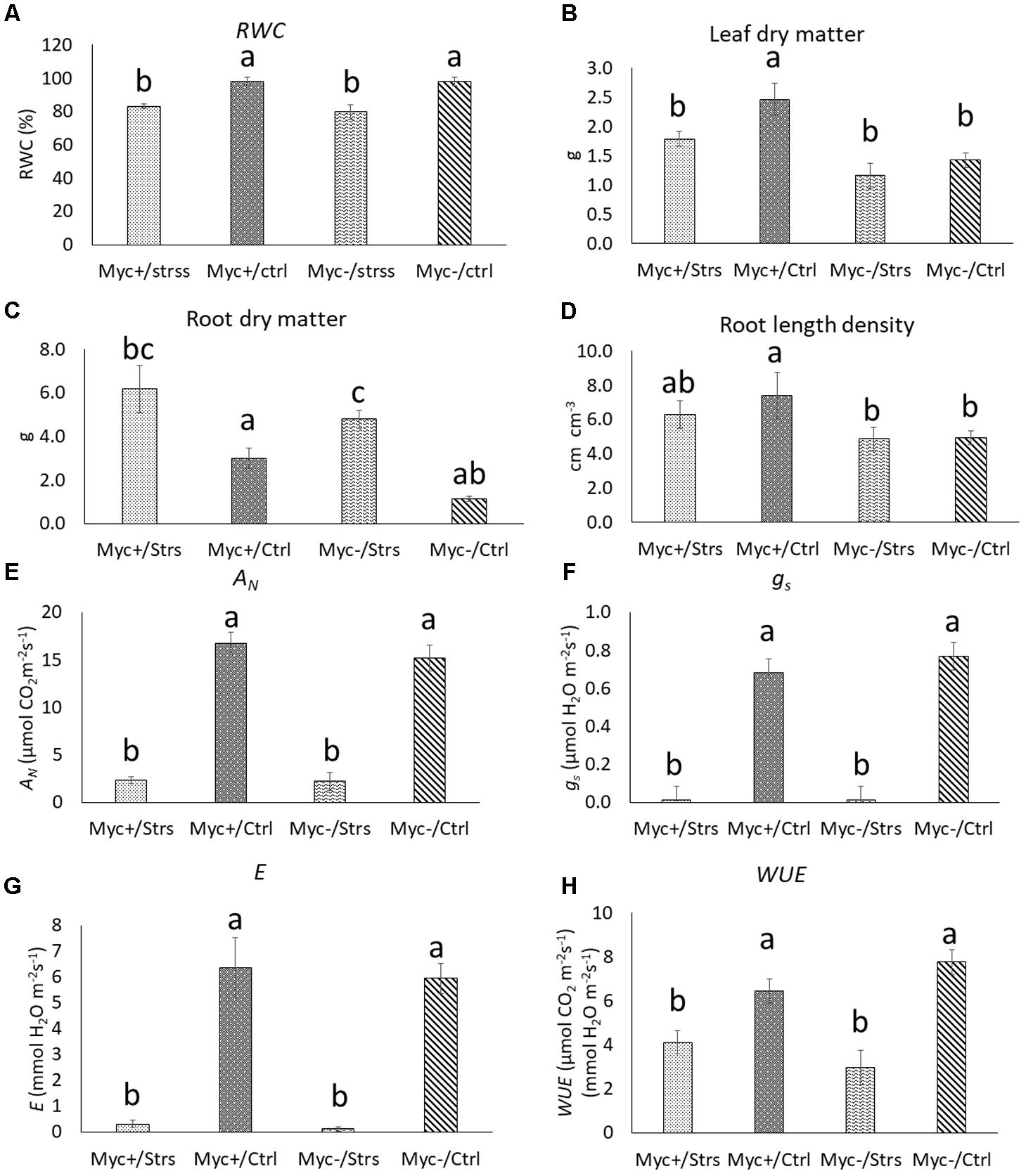
FIGURE 2. Physiological and growth parameters. (A) Relative water content (RWC) of leaf tissue; (B) leaf dry matter (LDM); (C) RLD; (D) root dry matter (RDM); (E) net photosynthetic rate (AN); (F) stomatal conductance (gs); (G) transpiration rate (E); (H) WUE. Myc+/Stress, AM plants under 96 h of water deficit; Myc+/Ctrl, AM plants under regular irrigation control; Myc–/Stress, no-AM plants under 96 h of water deficit; Myc–/Ctrl, no-AM plants under regular irrigation control. Bars represent standard deviation. Different letters indicate significant differences by Tukey’s test (p < 0.05).
The physiological status of the plants from the experiment was also accessed with the measurement of photosynthetic rates. The net photosynthetic rate (AN), stomatal conductance (gs), transpiration rate (E), and WUE all differed (p < 0.05) among water deficit treated plants and the control (Figures 2E–H). No differences, however, were detected between inoculated and non-inoculated plants under water deficit, except for the WUE, which was significant, but comparatively, with only slight modification.
Generation and Assembly of Transcript Reads
Twelve RNA-Seq libraries were constructed for paired-end sequencing consisting of biological triplicates from the four treatments: Myc+/Strss, Myc+/Ctrl, Myc-/Strss, and Myc-/Ctrl. The Illumina® HiScanTMSq sequencing of the 12 samples generated sequence reads of 101 bp long, with an average GC content of 45% and a phred value of 36. After sequences filtering, the average amount of reads for each dataset was: 21,611,562 for Myc+/Strss; 20,189,276 for Myc+/Ctrl; 22,664,734 for Myc-/Strss; and 20,247,646 for Myc-/Ctrl. The average amount of reads successfully mapped against P. vulgaris reference genome was: 68.32% for Myc+/Strss, 81.90% for Myc+/Ctrl, 85.50% for Myc-/Strss, and 86.25% for Myc-/Ctrl (Supplementary Table S1).
Differential Gene Expression Analysis
Individual gene and transcript annotation “track” files, retrieved for each triplicate, were merged per corresponding treatment, and tested for differential expression due to the “treatment” factor. Comparisons were made between group pairs and four statistical results were retrieved: Myc+/Ctrl vs. Myc+/Strss; Myc-/Strss vs. Myc+/Strss; Myc-/Ctrl vs. Myc+/Ctrl; and Myc-/Ctrl vs. Myc-/Strss (Supplementary Table S3).
The comparison that returned the highest amount of statistically significant (p-value with FDR < 0.05) DEGs, including transcripts isoforms, was related to non-inoculated plants, well-watered against 96 h’ water deficit treated (Myc-/Ctrl vs. Myc-/Strss), with a total of 10,569 DEG – 5,044 of them up-regulated under water deficit exposure and 5,525 down-regulated by the water deficit event. Inoculation treatments, control against water deficit (Myc+/Ctrl vs. Myc+/Strss), also produced a substantial level of differential expression, with 9,965 DEG – 4,668 were up-regulated by the water deficit treatment and 5,297 down-regulated (Supplementary Table S3).
For the differential gene expression analyses comparing non-inoculated against AMF-inoculated treatments, control (Myc-/Ctrl vs. Myc+/Ctrl) and water deficit (Myc-/Strss vs. Myc+/Strss), a list of 1,069 DEG was retrieved for control, with 712 DEG up-regulated by AMF inoculation, and 357 DEG down-regulated, and a small set of 15 DEG was obtained for water deficit treatments, with 11 DEG up-regulated by the AMF inoculation and 4 DEG down-regulated (Supplementary Table S3).
Differentially expressed genes that underwent significant changes in the four statistical comparisons can be depicted by the “red” dots in the Volcano plots displayed in Figure 3. The range in the expression levels of statistically significant DEGs varies considerably in all sets: from -11.4 to 13.29-fold change in Myc+/Ctrl vs. Myc+/Strss; from -12.73 to 11.73-fold change in Myc-/Ctrl vs. Myc-/Strss; from -12.88 to 11.56-fold change in Myc-/Ctrl vs. Myc+/Ctrl; and from -7.97 to 6.4-fold change in Myc-/Strss vs. Myc+/Strss (Figure 3 and Supplementary Table S3). DEGs showing the most significant statistical differences (p-value with FDR < 0.05) among biological replicates due to the “treatment” factor were selected and heat maps were constructed based on Manhattan distance and complete linkage for both comparisons: Myc+/Ctrl vs. Myc+/Strss and Myc-/Ctrl vs. Myc-/Strss (Figure 3). Two exclusive clusters of differential expressed genes were found, one for each comparison: “pink” bar for AMF-inoculated plants (Figure 3A) and “olive” bar for non-inoculated (Figure 3B).
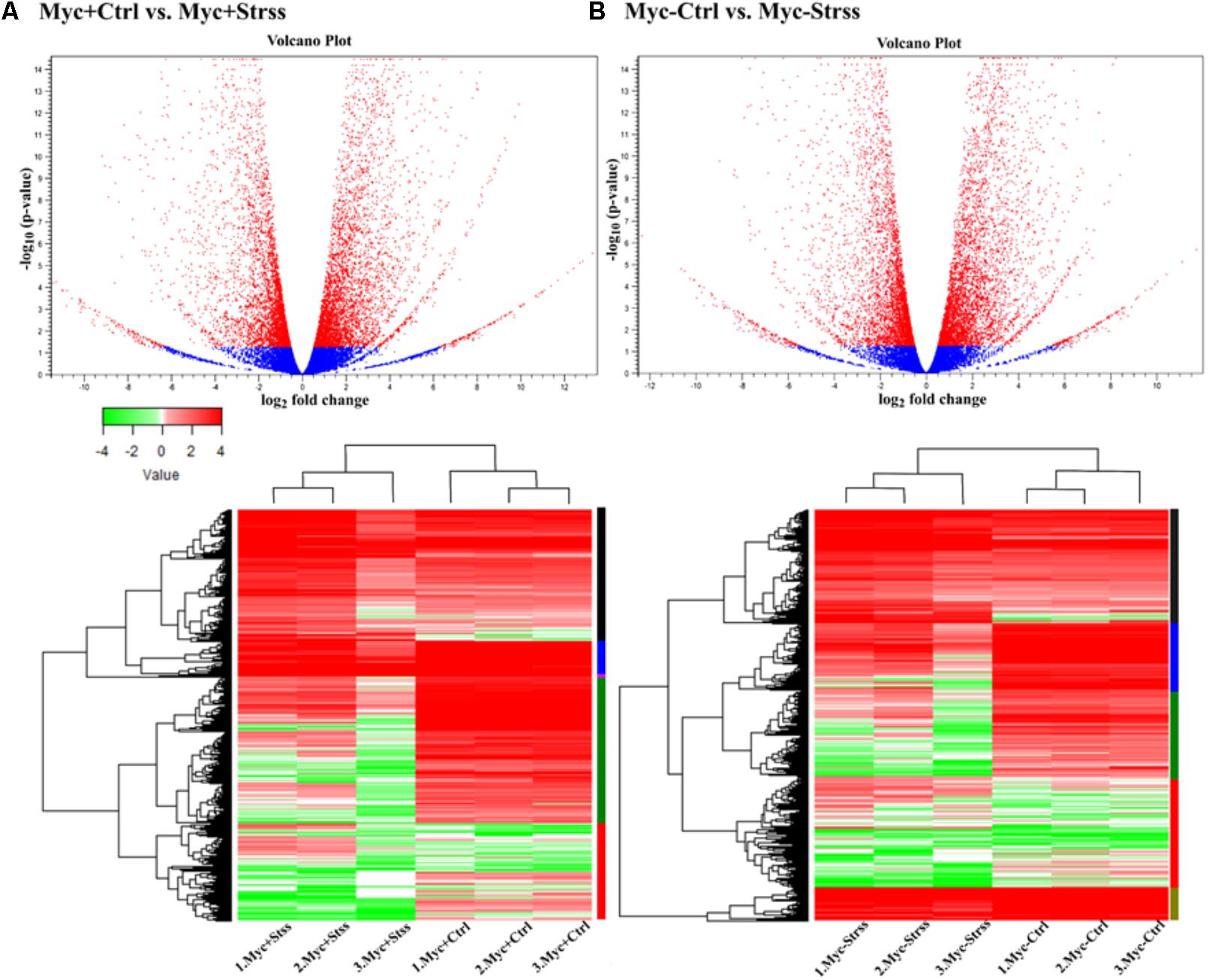
FIGURE 3. Differential expression analysis of RNA-Seq data. Statistical comparisons: (A) AMF-inoculated roots, control vs. water deficit (Myc+/Ctrl vs. Myc+/Strss); (B) non-inoculated roots, control vs. water deficit (Myc–/Ctrl vs. Myc–/Strss). At the top, Volcano plots of: (A) AMF-inoculated (right) and (B) non-inoculated roots (left); transcripts found to significantly up-/down-regulated (p-value with FDR < 0.05) are red colored dots. At the bottom, heat maps of all differentially expressed transcripts within biological replicates (p-value with FDR < 0.05): (A) AMF-inoculated (right) and (B) non-inoculated roots (left); colorized bars at the left of heat maps represent cluster of differentially expressed genes (DEGs). Heat maps based on Log2 transformed normalized expression data.
A Venn diagram was generated to further explore the relationships among the different sets of differential gene expression results (Figure 4). A total of 8,049 DEG were regulated in both AM- and non-AM, control against water deficit, comparisons. However, some exclusive DEGs were also evidenced for both sets, with non-inoculated plants showing 2,313 exclusive DEG, and AMF-inoculated with 1,589 exclusive DEG. For the list of DEG relative to the Myc-/Ctrl vs. Myc+/Ctrl set, 66 DEGs are exclusive, with the rest shared between both control against water deficit comparisons. From the list of DEG retrieved from the Myc-/Strss vs. Myc+/Strss comparison, only one (Phvul.003G105500.1 – PRMT4b) is exclusive, with the rest also shared with both control against water deficit comparisons. Both Myc-/Ctrl vs. Myc+/Ctrl and Myc-/Strss vs. Myc+/Strss sets do not share DEG in common. For a list of the exclusive DEG in each set and their related putative functions see Supplementary Table S4.
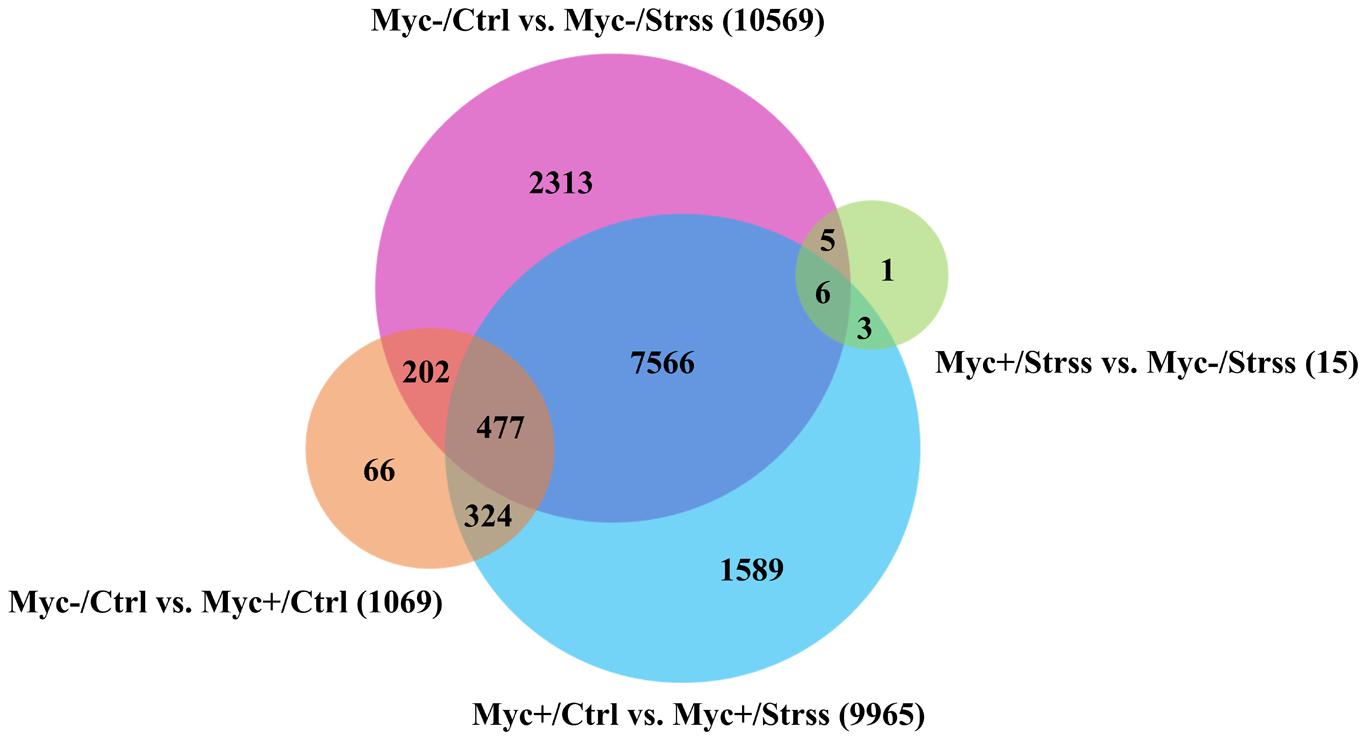
FIGURE 4. Venn diagram showing relationships among the sets of DEGs retrieved after differential gene expression analysis made between group pairs. Four statistical comparisons include: blue circle: AMF-inoculated roots, control vs. water deficit (Myc+/Ctrl vs. Myc+/Strss); pink-circle: non-inoculated roots, control vs. water deficit (Myc–/Ctrl vs. Myc–/Strss); orange-circle: control roots, non-inoculated vs. AMF-inoculated (Myc–/Ctrl vs. Myc+/Ctrl); green-circle: water deficit treated roots, AMF-inoculated vs. non-inoculated (Myc+/Strss vs. Myc–/Strss). Only DEGs, including transcripts isoforms, with a p-value with FDR < 0.05 were considered.
Following gene annotation and functional classification of the lists of DEG retrieved from each statistical comparison, the enrichment analyses of ontology terms were performed based on a Fisher-2 tailed test at FDR < 0.01, considering the total set of P. vulgaris annotated transcripts as the reference set. For the AMF-inoculated plants, control against water deficit comparison (Myc+/Ctrl vs. Myc+/Strss), a total of 98 ontology terms was differentially represented, with 36 of them over-represented in relation to the reference and 62 down-represented (Supplementary Table S5). For the non-inoculated set (Myc-/Ctrl vs. Myc-/Strss), 87 ontology terms showed differential representation, with 27 over-represented terms and 60 down-represented. Individual results of enrichment analysis tests are summarized according to the three major Gene Ontology classes: Biological Process, Molecular Functional, and Cellular Component (Figure 5).
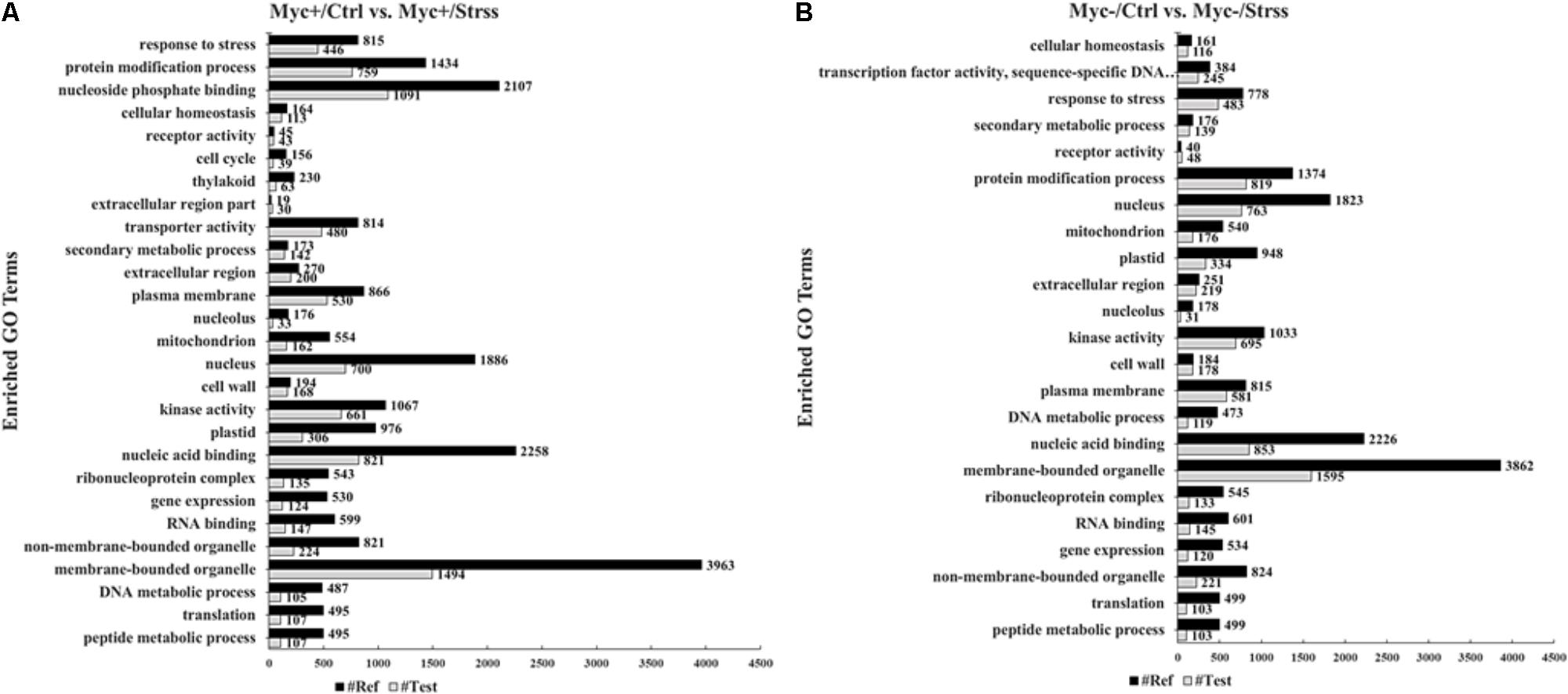
FIGURE 5. Gene ontology terms over-/underrepresented in both sets of DEGs (A, Myc+/Ctrl vs. Myc+/Strss and B, Myc-/Ctrl vs. Myc-/Strss) after enrichment analysis performed based on a Fisher-2 tailed test at FDR < 0.01. Numbers of DEGs assigned to each GO term are plotted at the right of the bars. Open bars: Test set expressed by the differentially regulated genes; closed bars: Reference set given by total set of Phaseolus vulgaris transcripts.
The enrichment analyses helped clarifying the dissimilarities found in the DEG’s list composition of both AM- and non-AM, control against water deficit, statistical comparisons displayed in the Venn diagram (Figure 4). The inoculation with AMF seemed to interfere with the cell cycle (GO:0007049), with this ontology term exclusively under-represented for the Myc+/Ctrl vs. Myc+/Strss comparison (Figure 5A). Moreover, the molecular functions nucleoside phosphate binding (GO:1901265) and transporter activity (GO:0005215) were only over-represented for the Myc+/Ctrl vs. Myc+/Strss set. Transcripts associated with thylakoid (GO:0009579) seemed to be under-represented exclusively in the Myc+/Ctrl vs. Myc+/Strss set, while those associated with the constituent parts of extracellular space (GO:0005615), including host cell environment outside an intracellular parasite, were over-represented. For the statistical comparison set Myc-/Ctrl vs. Myc-/Strss, the enrichment analysis showed an exclusive over-representation of terms associated with the molecular function transcription factor (TF) activity, sequence-specific DNA binding (GO:0003700) (Figure 5B).
The over-represented ontology term response to stress (GO:0006950), referring to changes in cellular homeostasis (GO:0019725) as a result of exogenous factors, was further explored aiming to target transcripts directly associated with host–symbiont interaction and water deficit event response that were exclusively regulated by both inoculation treatments. For the comparison between non-inoculated treatments, control against water deficit (Myc-/Ctrl vs. Myc-/Ctrl), 483 DEGs were assigned to response to stress, with 68 of them being exclusive to this condition, and 116 DEGs were associated with cellular homeostasis, with 21 of them exclusive. In the AMF-inoculated treatments comparison (Myc+/Ctrl vs. Myc+/Strss), 446 DEGs were assigned to response to stress, with 104 exclusives to this comparison, and 113 DEGs were associated with cellular homeostasis, with 18 of them exclusive (Supplementary Table S5).
This allowed the identification of some interesting common bean genes that are differentially regulated in response to water deficit during AMF inoculation, including those involved in TF activity, carbohydrate biosynthesis, osmoregulation, transport, DNA double-strand break repair, RNA processing, signal transduction, response to oxidative stress, protein ubiquitination, callose deposition, and response to auxin (Table 1). Among these genes, ethylene-responsive element binding factors (ERFs), a family of TFs regulated by the gaseous hormone ethylene induced by both biotic and abiotic stresses (Fujimoto et al., 2000), were the most abundant with a total of eight DEGs – including one transcript also containing the APETALA2 (AP2) domain. Also, another important biotic/abiotic stress responsive TFs were highlighted, like the WRKY (five DEGs), the auxin-responsive (six DEGs), and MADS box (one DEG), as well as some genes participating in osmotic adjustment like the aquaporin PvNIP2;4, LEA3 protein, Trehalose-phosphate phosphatases (3 DEG), and Na(+) and K(+) transporters (two DEGs).
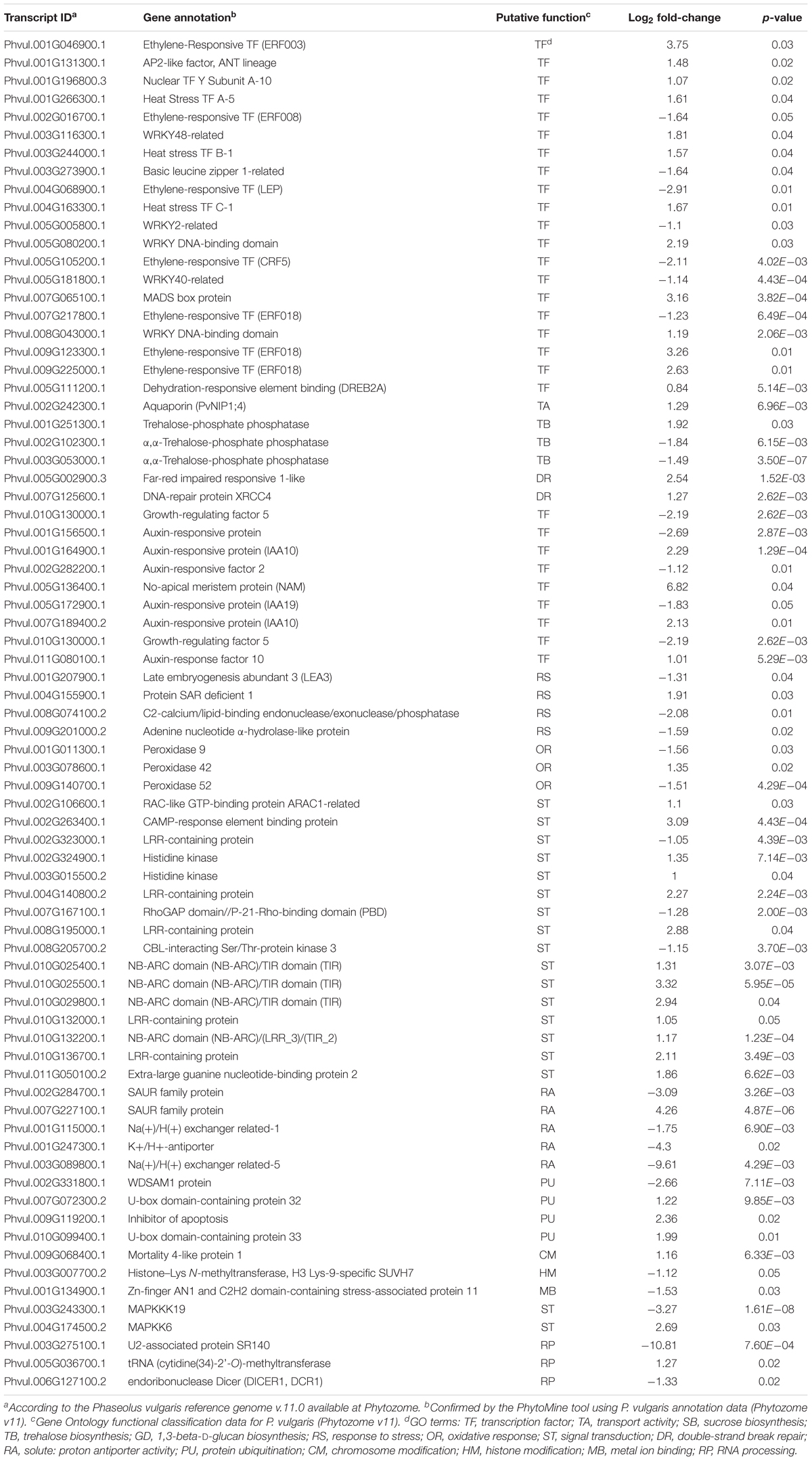
TABLE 1. Differentially expressed drought-responsive and symbiosis-related genes that were exclusively regulated in AMF-inoculated common bean roots comparing control and treatment (Myc+/Ctrl vs. Myc+/Strss).
The list of DEG retrieved from the statistical comparison between control treatments (Myc-/Ctrl vs. Myc+/Ctrl) revealed an exclusive enrichment for the biological process response to biotic stimulus (GO:0009607), and for transcripts associated with lysosome (GO:0005764) (Supplementary Table S5). For water deficit event treatments (Myc-/Strss vs. Myc+/Strss), an enrichment analysis of ontology terms was not possible due to the small amount of DEG retrieved. However, gene annotation allowed the assignment of putative function to 12, of the 15 DEG: one RNA-binding protein (REfBD) (Phvul.005G142200.1) (Glisovic et al., 2008); one single-strand-specific nuclease, ENDO 2 (Phvul.003G030500.1) (Desai and Shankar, 2003); one S-adenosyl-L-methionine-dependent methyltransferase (SAM) (Phvul.002G129100.2) (Kozbial and Mushegian, 2005); the TF AHL1 (Phvul.008G076100.2), usually associated with mitosis regulation (Fujimoto et al., 2004); two transcripts involved in mitochondrial metabolism, DTC (Phvul.002G238100.1) (Dolce et al., 2014) and MDM35 (Phvul.009G079400.2) (Miliara et al., 2015); one SAG gene (Phvul.011G120900.1), probably associated with the transference of senescence signals during drought (Wehner et al., 2016); and four transcripts associated with plant development, like the flowering time regulator PRMT4B (Phvul.003G105500.1) (Niu et al., 2008), and those related to root hair formation RHL1 (Phvul.004G139000.2) (Schneider et al., 1998), elongation PI4KBETA1 (Phvul.002G271500.2), and the TF NF-YB13 (Phvul.003G179300.2) implicated in primary root elongation and drought-stress response (Quach et al., 2015; Figure 6 and Supplementary Table S3).
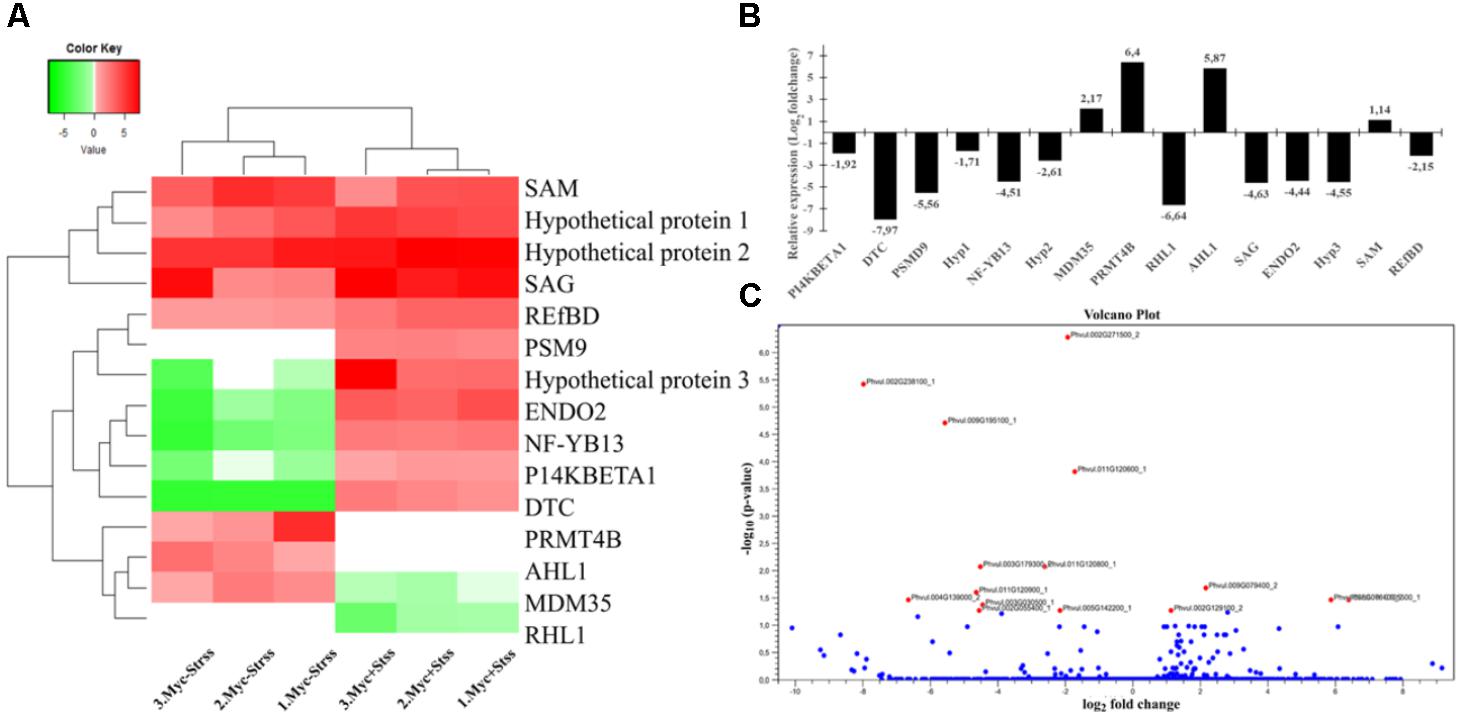
FIGURE 6. RNA-Seq differential gene expression analysis comparinganalysis comparing water deficit treatments non-inoculated against AMF-inoculated common bean roots (Myc–/Strss vs. Myc–/Strss). (A) Heat maps of all differentially expressed transcripts within biological replicates (p-value with FDR < 0.05). Heat maps based on individual Log2 transformed normalized expression data. (B) Log2 transformed relative expression data comparing the means of normalized counts retrieved for each DEG in both conditions. (C) Volcano plot; transcripts found to significantly up-/down-regulated (p-value with FDR < 0.05) are red colored dots.
Temporal Shift of Aquaporin Gene Expression in Roots and Leaves
Arbuscular mycorrhization has been implicated in the maintenance of common beans RWC, transpiration, and root hydraulic conductivity (LpR) through a fine-tune regulation in the expression levels of genes encoding aquaporins (Aroca et al., 2006), making this gene family perfect candidates for our time-course relative gene expression analysis by RT-qPCR.
Considering the 41 P. vulgaris aquaporin-related transcripts (Ariani and Gepts, 2015), differential gene expression analysis of RNA-Seq data showed that 18 transcripts were up-regulated, and four down-regulated, in AMF-inoculated roots under regular irrigation control conditions in relation to the water deficit event (Myc+/Ctrl vs. Myc+/Strss), with the gene PvNIP1;4 (Phvul.002G242300) exclusively regulated (1.29-fold change) under mycorrhizal colonization (Supplementary Table S6). For non-inoculated treatments, 22 transcripts were up-regulated and 5 were down-regulated, in control plants in relation to water deficit, with six of them exclusively regulated: PvPIP2;1 (Phvul.004G082600) (5.81-fold change), PvTIP2;1 (Phvul.005G170300) (4.34-fold change), PvSIP1;1 (Phvul.001G097000) (0.67-fold change), PvSIP1;3 (Phvul.011G102700) (3.68-fold change), PvSIP2;1 (Phvul.001G108800) (0.59-fold change), and PvXIP1;2 (Phvul.011G025800) (-3.92-fold change). Hence, genes belonging to the aquaporin subfamily small-intrinsic proteins (SIPs) were only found differentially regulated under non-inoculated conditions.
Nine aquaporin-related transcripts were selected for the RT-qPCR analysis based on their previous reported relevance for drought-stress response in common bean’s BAT477 genotype (Recchia et al., 2013). Total RNA was obtained from both roots and leaves harvested at four periods, 24, 48, 72, and 96 h of the water deficit event treatment. Three technical replicates were considered for each of the biological triplicates from the four initial treatments. Relative expression analysis between treatments were performed taking IDE and UBQ genes as internal normalizers for leaf samples and IDE and Skip16 genes for root samples (Borges et al., 2012). Three statistical comparisons were performed: Myc+/Strss vs. Myc+/Ctrl, Myc-/Strss vs. Myc-/Ctrl and Myc+/Strss vs. Myc-/Strss.
The overall performance of up- and down-regulation of aquaporin transcripts in both organs and inoculation treatments was very random through the 96 h of water deficit treatment (Figures 7, 8). Thus, grouping the differential expression results into clusters sharing similar profiles of up-/down-regulation was not possible for the statistical comparisons Myc+/Strss vs. Myc+/Ctrl and Myc-/Strss vs. Myc-/Ctrl. Statistical significant differences (p-value < 0.05) in the expression regulation of these transcripts in roots and leaves were found in the four periods tested. The only exceptions are the gene PvPIP1;2 that in roots was up-regulated by water deficit in relation to control in both inoculation conditions, and PvPIP2;3 that in leaves was down-regulated for the initial 48 h by water deficit when compared to the control, with an up-regulation at 96 h, also for both inoculation systems (Figures 7, 8).
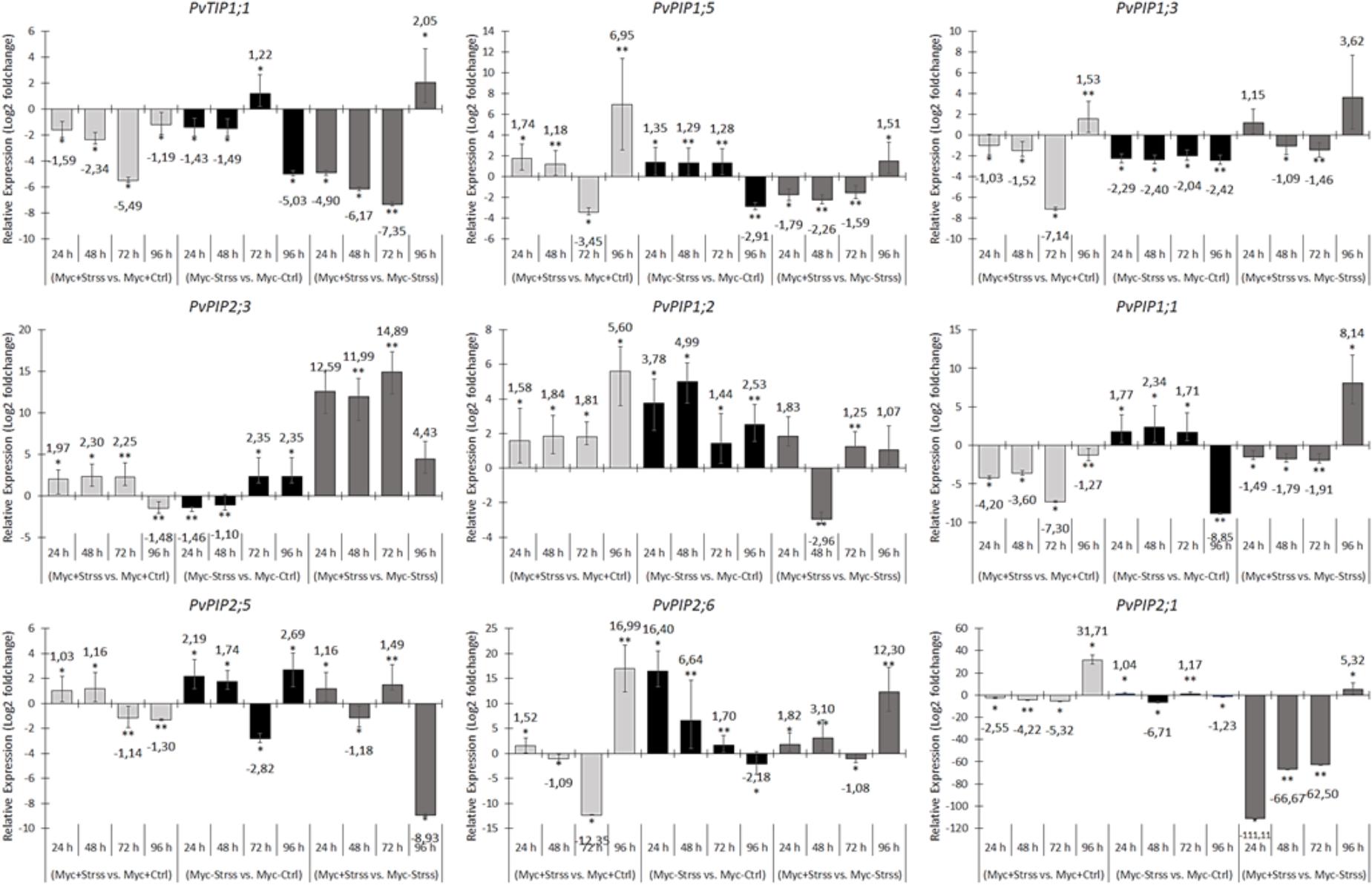
FIGURE 7. RT-qPCR-based relative gene expression analysis of aquaporin-related transcripts in common bean roots harvested at 24, 48, 72, and 96 h of treatment. For each transcript, three relative comparisons were made between treatments: Myc+/Stss vs. Myc+/Ctrl (AM-inoculated, water deficit vs. control), Myc–/Strss vs. Myc–/Ctrl (non-inoculated, water deficit vs. control) and, Myc+/Strss vs. Myc–/Strss (water deficit, AM-inoculated vs. non-inoculated). Relative expression levels were log2 transformed and the average fold changes in expression ratio for each gene is highlighted. Statistical hypothesis testing based on p-values were obtained by a pairwise reallocation randomization test (bootstrap = 2,000 permutations). Bars represent the standard error of the mean factor of relative expression results comparing sample and control groups.
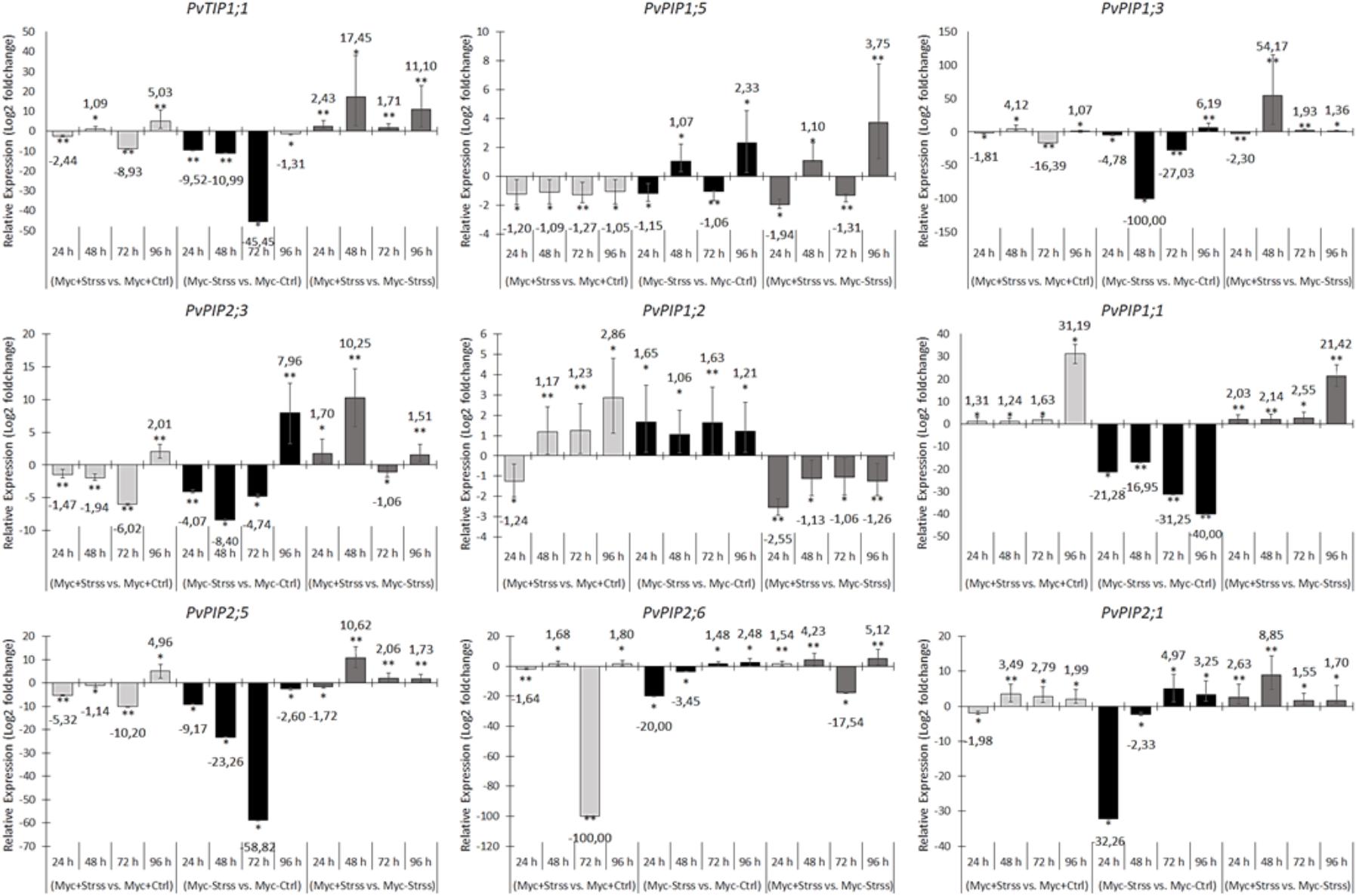
FIGURE 8. RT-qPCR-based relative gene expression analysis of aquaporin-related transcripts in common bean leaves harvested at 24, 48, 72, and 96 h of treatment. For each transcript, three relative comparisons were made between treatments: Myc+/Stss vs. Myc+/Ctrl (AM-inoculated, water deficit vs. control), Myc–/Strss vs. Myc–/Ctrl (non-inoculated, water deficit vs. control) and, Myc+/Strss vs. Myc–/Strss (water deficit, AM-inoculated vs. non-inoculated). Relative expression levels were log2 transformed and the average fold changes in expression ratio for each gene is highlighted. Statistical hypothesis testing based on p-values was obtained by a pairwise reallocation randomization test (bootstrap = 2,000 permutations). Bars represent the standard error of the mean factor of relative expression results comparing sample and control groups.
Relative gene expression data of root samples comparing both water deficit treatments (Myc+/Strss vs. Myc-/Strss) revealed no significant statistical changes at 24 and 96 h (Figure 7). The majority of aquaporin transcripts tested showed significant down-regulation in water deficit event AMF roots in relation to non-AM at both 48 and 72 h: PvTIP1;1, PvPIP1;5, PvPIP1;3, PvPIP1;1, and PvPIP2;1; one was up-regulated at both periods, PvPIP2;3; two were down-regulated at 48 h and down-regulated at 72 h: PvPIP1;2 and PvPIP2;5; and PvPIP2;6 was up-regulated at 48 h and down-regulated at 72 h.
Regarding leaf samples of water deficit event treated plants, the expression levels of PvPIP1;2 were lower in mycorrhizal plants, in relation to non-inoculation treatment, for the whole period of water deficit treatment (Figure 8). Three other genes, PvTIP1;1, PvPIP1;1, and PvPIP2;1, showed superior levels of expression in Myc+/Strss leaves in relation to Myc-/Strss for the whole period, with peaks of up-regulation of 17.45-fold at 48 h for PvTIP1;1, 21.42-fold at 96 h for PvPIP1;1 and 8.25-fold at 48 h for PvPIP2;1. Four other genes, PvPIP1;3, PvPIP2;5, PvPIP2;3, and PvPIP2;6, are up-regulated in Myc+/Strss samples in relation to Myc-/Strss for all periods, except for a down-regulation at the initial 24 h for PvPIP1;3 and PvPIP2;5, and at 76 h for PvPIP2;3 and PvPIP2;6. For PvPIP1;3, PvPIP2;5, and PvPIP2;3, this down-regulation is related to a lower repression rate of these genes in leaves of non-inoculated plants during the water deficit event, and a pronounced down-regulation of 100-fold for PvPIP2;6 in Myc+/Strss samples after 72 h of treatment.
Tissue-Specific Gene Expression Analysis
The establishment of AMF specialized colonization structures – termed “arbuscules” – in root cortical cells are accompanied by an intense modification in host’s transcriptome, both at local and systemic levels (Harrison, 2005; Balestrini and Lanfranco, 2006). To better characterize this influence in our model of study, the microscopy-based LCM technique was applied in combination with relative RT-qPCR analysis. A set of 23 transcripts were chosen among the main functional classes that were enriched by our treatments. Three cell populations were collected: ctx+ – root cortical cells containing arbuscules and/or hyphal structures derived from Myc+/Strss treatments; ctx- – root cortical cells not containing arbuscules derived from Myc+/Strss treatments; and ctrl – root cortical cells derived from Myc-/Strss treatments (Figures 1D–I).
The paraffin embedding and tissue staining protocols showed to be adequate to preserve internal root and AM colonization structures and was helpful to secure RNA molecules integrity. The complete protocol, from tissue sectioning, through RNA extraction and cDNA synthesis, was always performed in a row, with the newly obtained cDNA samples kept at -20°C to prevent contamination. For the relative RT-qPCR analysis, three biological replicates, containing technical triplicates each, were considered.
The combination of paraffin embedding and the selected staining protocol, however, affected the resolution of the histological sections. This did not compromise the proper identification of internal root and AM colonization structures but opened the possibility for a detrimental effect of such a prolonged water deficit treatment (96 h) over arbuscules integrity. Therefore, histological sections retrieved from the same pools were embedded in historesin and stained following the same protocols, offering superior resolution and confirming an extensive AM colonization net through root cortical cells that are interconnected by hyphal structures (Figures 1A,B) and arbuscules at alternating stages of development (Figure 1C), thus revealing that colonization is an ongoing process even during environmental stressful conditions.
RT-qPCR amplification products were visualized in a 1.2% agarose gel (Supplementary Figure S2a). From the selected internal normalizers, skip16 was the most stable in all samples analyzed (Supplementary Figure S2b). AML1 and AML2 amplification attested the quality of microdissected samples since no amplification products were detected on both ctx- and ctrl samples (Supplementary Figure S2c). Products from two transcripts, GH3 (Phvul.004G077000) and PvPIP2;3 (Phvul.007G094600), were only amplified in ctx+ samples. Five other transcripts, bhlh95 (Phvul.003G048000), CKX (Phvul.009G081800), bZIP (Phvul.010G018200), KHX (Phvul.001G051900), and ABHD (Phvul.006G155700), were amplified only in ctx+ and ctrl cells. Amplification products were obtained from the other 16 transcripts for the three cell types analyzed. No-template control confirmed the absence of genomic-DNA contamination and the formation of primer artifacts.
Two relative gene expression analyses were performed: ctx+ relative to ctx- and ctx+ relative to ctrl (Figure 9); both were applied to identify differences in transcription regulation due to the presence of arbuscules. Among those genes that underwent significant changes in expression in arbusculated root cortical cells (ctx+) relative to their neighboring non-arbusculated root-cortical cells (ctx-), nine were up-regulated in ctx+ samples: NB-ARC-LRR (Phvul.001G132700) (1.96-fold), TPS (Phvul.011G107200) (45.57-fold), ZFHD (Phvul.009G037900) (2.06-fold), LTP (Phvul.008G198300) (20.87-fold), utp23 (Phvul.005G025400) (5.44-fold), Apg9 (Phvul.007G194300) (4.67-fold), LPL (Phvul.008G198300) (1.48-fold), GDH (1.01-fold) (Phvul.002G122600), and PvPIP2;6 (Phvul.011G079300) (2.37-fold); and six were down-regulated: RCC1 (Phvul.008G230700) (-3.89-fold), hAT (Phvul.001G020700) (-1.33-fold), LEA5 (GI| 17325561|) (-1.40-fold), PvNAM4 (Phvul.009G038400) (-1.25-fold), PvNAC4 (Phvul.009G038400) (-4.55-fold), and SRF (Phvul.008G027900) (-2.95-fold) (Figure 9A).
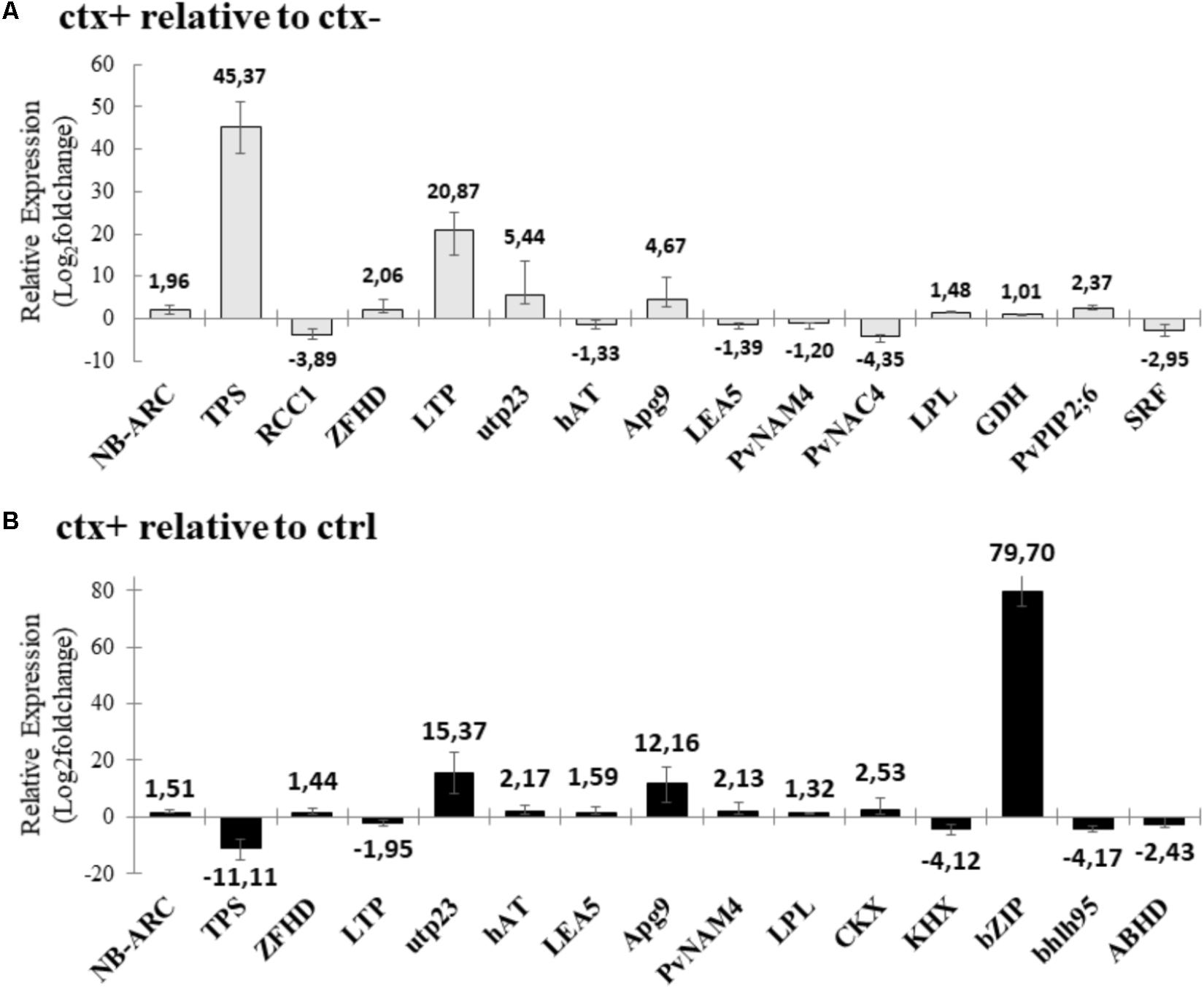
FIGURE 9. Relative gene expression data analysis using real-time quantitative PCR. (A) Water deficit treated root-cortical cells containing arbuscules (ctx+) relative to water deficit treated non-arbusculated AM-root cortical cells (ctx–); (B) water deficit treated root cortical cells containing arbuscules (ctx+) relative to water deficit treated root-cortical cells retrieved from non-inoculated plants (ctrl). Relative expression levels were log2 transformed and the average fold changes in expression ratio for each gene is highlighted. Only genes with significant changes (p < 0.05) are shown. p-values were obtained by a pairwise reallocation randomization test (bootstrap = 2,000 permutations). Bars represent the standard error of the mean factor of relative expression results comparing sample and control groups.
Relative gene expression analysis between arbusculated cells from AMF-inoculated roots (ctx+) relative to cortical cells from non-inoculated roots (ctrl) showed that 10 transcripts were up-regulated: NB-ARC-LRR (1.51-fold), ZFHD (1.44-fold), utp23 (15.37-fold), hAT (2.17-fold), LEA5 (1.59-fold), Apg9 (12.16-fold), PvNAM4 (2.13-fold), LPL (1.32-fold), CKX (Phvul.009G081800) (2.53-fold), and bZIP (Phvul. 010G018200) (79.7-fold); and five were down-regulated: TPS (-11.11-fold), LTP (-1.95-fold), KHX (Phvul.011G019400) (-4.12-fold), bhlh95 (Phvul.003G48000) (-4.17-fold), and ABHD (Phvul.006G155700) (-2.43-fold) (Figure 9B).
Some of the selected genes were amplified in the three cell types, but relative expression did not reveal and significant statistical changes between the conditions tested. This was the case for PvNAM28 (Phvul.005G047300) in the ctx+ vs. ctx- analysis. For the ctx+ vs. ctrl, in addition to PvNAM28, five other transcripts could be pointed: RCC1, LEA5, PvNAC4, GDH, and PvPIP2;5. The SRF gene was only amplified in AMF-inoculated roots, with a down-regulation in ctx+ vs. ctx- (Figure 9A). For the transcripts with significant differential regulation exclusively for the ctx+ vs. ctrl comparison, two were up-regulated in ctx+ samples, CKX and bZIP, and three were down-regulated, KHX, bhlh95, and ABDH (Figure 9B). Genes GH3 and PvPIP2;3 were only amplified in ctx+ samples, therefore not producing relative gene expression data.
Discussion
The examination of the AMF colonization influence over the gene expression profile of plants has been conducted for a variety of species (Güimil et al., 2005; Zouari et al., 2014; Handa et al., 2015; Vangelisti et al., 2018). In all studies, AMF colonization seemed to affect a diverse range of metabolic pathways during plant development, including primary and secondary metabolisms, ion transport, signal transduction, and transcriptional regulation, indicating a strong dependency by the hosts to this kind of symbiosis.
In our study, AMF colonization did not produce any distinguishable morphologic alterations in plants during the water deficit regime. Although a slightly increase in RLD and leaf dry matter (LDM) was observed for the AMF-inoculated well-watered plants (Myc+/Ctrl), no statistical significant changes were observed for the AMF-inoculated water deficit treated plants (Myc+/Strss) when compared with non-inoculated treatments, despite root AMF colonization being 1.33 times higher in average for the Myc+/Strss treatment (74.44%) than for Myc+/Ctrl (55.74%). Although an increase in AM-root colonization during water scarcity regimes is not expected, especially due to a reduced availability of carbon from the host (Subramanian and Charest, 1995; Zhang et al., 2014; Gong et al., 2015), studies involving potted plants with relatively short water deficit treatments demonstrated that the duration of drought has not necessarily appeared to favor or discourage colonization, with both observations being reported (Augé, 2001).
Similarly, photosynthetic variables were mostly altered due to the water deficit treatment, but no significant differences, in general, were detected between inoculated and non-inoculated plants under stress. Mycorrhizal influences on tissue hydration and foliar gas exchange are often subtle, transient, and probably circumstance and symbiont specific. Although several reports have indicated an increase in photosynthetic rates of plants during the symbiosis (Augé, 2001; Bárzana et al., 2012; Rapparini and Peñuelas, 2014), many investigators found no differences between AM and non-AM plants, especially regarding stomatal conductance and transpiration (Augé, 2001).
At the molecular level, however, from the 31,638 annotated transcripts in the P. vulgaris genome (Schmutz et al., 2014), 9,965 were differentially regulated in AM-inoculated water deficit treated plants in relation to the respective control (Myc+/Ctrl vs. Myc+/Strss), and in non-inoculated conditions this number was even higher, with 10,569 DEG. From these, 1,596 DEGs were exclusively regulated in AM roots and 2,313 DEG in non-AM roots (Figure 4 and Supplementary Tables S3, S4). Enrichment analysis revealed that 559 transcripts could be directly assigned to stress response (125 DEG exclusive) and maintenance of cellular water homeostasis in AM-inoculated roots, while for the non-inoculation conditions rates are higher, with 599 DEGs (86 DEG exclusive) (Supplementary Table S5). Thus, during arbuscular mycorrhization, common bean plants showed alternative mechanisms to cope with the water deficit event (Table 1).
Arbuscular mycorrhizal fungi-inoculated and non-inoculated gene expression profiles were statistically compared to check whether those shifts in plants metabolism could interfere with common beans response to the water deficit event. Although a considerable number of transcripts were differentially regulated between the well-watered control treatments (Myc-/Ctrl vs. Myc+/Ctrl), only 15 statistically significant DEGs were retrieved from the Myc-/Strss vs. Myc+/Strss comparison (Supplementary Table S3). Thus, those differences highlighted in both control versus water deficit differential analysis might be more related to the dissimilarities guarded between both control treatments (Myc+/Ctrl and Myc-/Ctrl) considered as basis for both statistical comparisons.
Nevertheless, the up-regulation in Myc+/Strss samples, in relation to Myc-/Strss, of two genes, DTC and MDM35, both associated with mitochondrial metabolism, the exclusive down-regulation of the flowering-time regulator PRMT4b, and the up-regulation of genes related to root hair formation RHL1, and elongation, PI4KBETA1 and NF-YB13, the latter also involved in water deficit event response, indicate that changes in the plant metabolism and development, especially those associated with root growth and architecture, might occur during the symbiosis, and could potentially affect common beans capacity of responding to a water deficit event. Up-regulation of these genes could also be associated with the discreet increase in RLD and RDM of mycorrhizal roots in relation to non-inoculated samples (Figure 2).
Nearly all plant hormones are employed by plants to regulate the symbiosis with AMF (Cosme et al., 2016). Among these signaling molecules, abscisic acid (ABA), a sesquiterpenoid hormone derived from carotenoids, functions at multiple levels to regulate AM symbiosis (Martín-Rodríguez et al., 2016). In many plant species it has been observed that the concentration of ABA rises during the establishment of mycorrhization (Herrera-Medina et al., 2007; Ludwig-Müller, 2010), with evidences of an impact over the proper formation of arbuscules and a sustained colonization of plant roots (Herrera-Medina et al., 2007). During drought, the increased concentration of ABA in leaves promotes the closure of stomata to minimize transpirational water loss. It also mitigates stress damage through the activation of many stress-responsive genes that encode enzymes for the biosynthesis of compatible osmolytes and LEA-like proteins, increasing plant tolerance (Herrera-Medina et al., 2007). Root colonization favors the production of essential isoprenoids, such as carotenoids, therefore, with a direct impact in the source of important growth regulators such as ABA. Thus, it was proposed that a higher demand of carotenoid-derived compounds and pigments is expected in AM plants, especially under stress conditions where these isoprenoid compounds might play a role in plant protection and defense (Rapparini and Peñuelas, 2014).
The relevance of ABA in the response of plants to a water deficit event, especially under AMF colonization, is highlighted in our study by the differential regulation of target genes directly associated with the ABA biosynthesis pathway in both statistical comparisons Myc+/Ctrl vs. Myc+/Strss and Myc-/Ctrl vs. Myc-/Strss (Supplementary Table S3). The expression of a zeatin peroxidase AtZEP gene homolog (Phvul.002G18700.1) was induced by water deficit in both inoculated (3.51-fold) and non-inoculated treatments (3.19-fold). The 9-cys-eppoxycarotenoid dioxygenase genes (NCED) were also differentially regulated; an AtNCED9 homolog (Phvul.005G051600.1) underwent a 4.54-fold change in water deficit treated AM-inoculated plants and a 5.12-fold-change in non-inoculated plants, while the AtNCED1 homolog (Phvul.009G109100.1) was repressed in water deficit treated plants, with a -8-fold change in AM-inoculated plants and a -10.03-fold change in non-AM plants. A short-chain alcohol dehydrogenase/reductase ATSDR1 gene homolog (Phvul.011G097200.1) also underwent repression under water deficit conditions in both inoculation treatments, with a -3.51-fold change for AM plants and a -4.71-fold change for non-AM plants. Thus, the differential regulation of these pivotal genes helps not only to validate the efficacy of the water deficit assay, but also highlights that, considering both statistical comparisons, Myc+/Ctrl vs. Myc+/Strss and Myc-/Ctrl vs. Myc-/Strss, the lack of variation between the levels of induction/repression of those genes helps to corroborate the absence of significant statistical differences displayed by the gas exchange measurements considering Myc+/Strss and Myc-/Strss plants (Figures 2E–H).
Moreover, two ABF2 homolog genes (Phvul.009G065500 and Phvul003G291800), both coding for an ABRE-BINDING protein factor (AREB/ABF), were up-regulated with statistical significance only in water deficit treated AM-inoculated plants over the respective control (Myc+/Ctrl vs. Myc+/Strss); with a 2.01-fold change for Phvul.009G065500 and a 1.35-fold change for Phvul003G291800. Both in vitro and in vivo data indicate that the three AREB/ABF members (ABF1, ABF2, and ABF3) could recognize the ABRE sequence in the DREB2A gene promoter in Arabidopsis plants and activate it in response to osmotic stress conditions under which activation levels were reduced (Kim et al., 2018). In fact, a DREB2a gene homolog (Phvul.005G111200.1) was exclusively regulated in AM-inoculated plants (0.84-fold-change) (Table 1). Together, these results point toward alternative mechanisms by which mycorrhizal colonization might influence water deficit response in plants.
As evidences for a mycorrhizal-based water deficit event response in common beans were offered, we decided to test whether a prolonged water deficit treatment (96 h) could impose limiting conditions for AM development, therefore potentially affecting its influence over plant’s metabolism. A RT-qPCR-based relative differential gene expression analysis was performed considering whole roots and leaves, harvested at 24, 48, 72, and 96 h of water deficit treatment – from both AMF-inoculated and non-inoculated, control and stressed plants – aiming to further investigate the influence of mycorrhization over plant’s expression of drought-responsive genes in earlier periods of water deprivation. For this analysis, candidate genes encoding putative aquaporins were considered as markers for water deficit stress response.
Aquaporins provide a low resistance pathway for the movement of water across a membrane, and since aquaporins can be gated, this provides greater control for the movement of water along plant tissues (Bárzana et al., 2014). PIP and TIP isoforms have been recognized as central pathways for transcellular and intracellular water transport (Maurel et al., 2008). Thus, it seems likely that mycorrhizal symbiosis causes significant changes in aquaporin activity of host plants (Porcel et al., 2006; Aroca et al., 2007; Uehlein et al., 2007) and some of the plant aquaporins might be important for these mycorrhizal responses.
In fact, RNA-Seq differential gene expression analysis data collected from both inoculation conditions (Myc+/Ctrl vs. Myc+/Strss and Myc-/Ctrl vs. Myc-/Strss) were further investigated and significant differences in the regulation of the 41 P. vulgaris aquaporin-related transcripts were observed; including the existence of certain genes being exclusively regulated in each inoculation condition. In general, a statistical significant down-regulation was observed in most of those transcripts accessed in both inoculation treatments when exposed to the water deficit events. Similar results were found by Bárzana et al. (2014) when analyzing AMF-inoculated maize plants exposed to a severe drought regime, with the down-regulation associated to a mechanism of reducing osmotic root hydraulic conductance in order to prevent excessive water loss to the soil.
Regarding the time-course RT-qPCR analysis, aquaporin genes showed distinct patterns of up-/down-regulation among the different inoculation treatments, organs and time-periods analyzed, therefore not allowing the grouping of those transcripts per common features. This might be a reflect of the redundant nature of the MIP family (membrane intrinsic protein) with members that show specific spatial and/or temporal expression patterns, limited to certain organs or cell types, or developmental stages (Javot and Maurel, 2002; Alexandersson et al., 2010). Moreover, Valot et al. (2005) and Aroca et al. (2006) found that several plasma membrane (PM) proteins were differently regulated by inoculation with G. intraradices, with some of them being down- or up-regulated. Also, a compensatory mechanism that balances the down-regulation of host’s aquaporins with the heightened activity of AMF aquaporins to maintain high root hydraulic conductance in AM roots has been proposed (Aroca et al., 2009).
Comparing the differential expression profiles of aquaporin genes obtained for water deficit treated roots (Myc+/Strss vs. Myc-/Strss), statistical significant results (p-value < 0.05) were retrieved only for intermediate time-periods (48 and 72 h), thus validating initial RNA-Seq results and pointing toward a scenario were AMF inoculation might be more relevant for the regulation of root hydraulic properties at initial stages of water deficit periods. Additionally, although the transcriptional control of aquaporins in water deficit treated leaves is often reported as more complex and a tendency to overall aquaporin gene down-regulation has been reported (Maurel et al., 2008), in our analyses, up-regulation of aquaporin genes in leaves was detected for all the periods analyzed, including PvPIP1;1, up-regulated by water deficit in AMF-inoculated plants during the whole period; the same happening for PvPIP1;2 in non-inoculated plants (Figure 8).
Leaves harvested from water deficit event treated samples showed significant up-regulation of aquaporin transcripts (excepting PvPIP1;2) in AMF-inoculated plants, in relation to non-inoculated samples, after 96 h (Figure 8). Leaf aquaporins, especially those localized in stomata guard cells, play a pivotal role in transpiration (Maurel et al., 2008), and many studies have shown an enhancement in the rates of gas exchange parameters (stomatal conductance, transpiration, and photosynthetic rates) in mycorrhizal plants under water limited conditions (Rapparini and Peñuelas, 2014). The lack of statistically significant differences in the expression levels of drought-related genes between AMF-inoculated and non-inoculated roots could be part of a differentiated response mediated by AMF that prioritizes the regulation of the water relations in aerial parts of the plant in detriment of the root system. Therefore, as the water deficit event increases, mycorrhizal colonization would reinforce previous phenotypic observations made for common bean drought-tolerant lines involving a fine control of stomatal conductance, and an efficient CO2 diffusion and fixation, that leads to an effective water use that favors seed filling rather than the maintenance of leaf turgor and growth (Rosales et al., 2012).
Transcripts of a number of genes expressed in mycorrhizal roots occur in cortical cells containing the arbuscules (Balestrini and Lanfranco, 2006), and an AM-specific signal may be responsible for the activation of these genes (Harrison, 2005). Some other genes might be expressed in cortical cells in the vicinity of colonized cells, or elsewhere in the plant, like in leaves, suggesting the presence of a second mobile signal acting in the colonized region of the root (Balestrini and Lanfranco, 2006). Thus, a lot have been questioned about in which extent whole-organs transcriptomic analysis wouldn’t mask the real changes in the transcription levels of genes that are relevant for mycorrhizal symbiosis and are restricted to specific tissues.
The LCM technique was applied and provided successful isolation of root cortical tissues in water deficit treated samples of both AM- and non-AM common bean plants. A set of 23 transcripts were selected among the statistical comparisons of differential gene expression analyses. The selection took into consideration the potential role of these genes in coordinating drought-tolerance responses in common beans under the influence of mycorrhizal colonization and other important ontology terms that were significantly impacted by our treatments, like host–symbiont interaction, plant cellular modifications to harbor arbuscules, transcriptional and post-transcriptional regulation, and genes associated with the control of plant hormonal levels.
Arbuscules are surrounded in cortical cells by a plant-derived pathogen-associated molecule (PAM) that is continuous to the PM of plants and excludes the fungus from the cytoplasm (Parniske, 2008). Thus, the lipid composition of PAM might differ from that of PM (Pumplin and Harrison, 2009). The LPL transcript, a lipoprotein lipase (LPL) gene, was up-regulated in arbusculated cortical cells (ctx+), both in relation to ctx- (1.48-fold) and to ctrl (1.32-fold). These results are in agreement with the up-regulation of a class 3 lipase (mtr.13800.1s1) in root cortical cells of Medicago truncatula inoculated with G. intraradices (Gaude et al., 2012).
Pathogen-associated molecule also holds a unique protein composition, including exclusively expressed phosphate transporters (Harrison et al., 2002), H+-ATPases (Krajinski et al., 2000), and major intrinsic proteins (MIPs) (Uehlein et al., 2007). Two putative common bean aquaporin transcripts, PvPIP2;3 and PvPIP2;6, were evaluated in our LCM/RT-qPCR analysis. PvPIP2;3 was exclusively amplified in arbusculated cells (ctx+) (Supplementary Figure S2). Since the time-based RT-qPCR analyses showed a down-regulation of this gene in the whole root organ after 96 h of water deficit treatment in AMF-inoculated plants this could be an evidence of potential masking effects of whole transcriptomics analyses in relevant genes regulated by the symbiosis. Moreover, the 16.99-fold up-regulation of PvPIP2;6 in 96 h water deficit treated AMF-inoculated roots in relation to the respective control and the down-regulation (-2.18-fold) at the same period for non-inoculation conditions (Figure 7) could offer, in combination with LCM/RT-qPCR data, the basis for an extra scenario in water deficit tolerance mediated by AMF where transcripts associated with water transport and osmoregulation are induced in arbusculated cortical cells and in neighboring cells through a signalization cascade.
The osmoregulation of cytosolic water homeostasis, a vital process required to sustain cell turgor and support expansion growth of the root system, is achieved by absorption, transport, and compartmentalization of water and solutes, being the intracellular K+ levels a prerequisite for the optimal plant metabolic machinery (Shabala and Pottosin, 2014). Liu et al. (2006) indicated that during dehydration, transmembrane channels for water and K+ are down-regulated in a coordinated manner, reducing membrane water permeability and promoting cellular water conservation. The KHX transcript, coding for a K+/H+ antiporter, was down-regulated in ctx+ cells in relation to ctrl (Figure 9B). Since KHX was not amplified in ctx- samples, signalization events mediated by arbuscules could work both ways, whether by inducing/repressing genes depending on its role in the regulation of root water relations. Another important agent of osmotic adjustment in BAT477 genotype during drought, the hydrophilic protein LEA5 (Late embryogenesis abundant) (Recchia et al., 2013), underwent differential regulation in cortical cell, with a -1.39-fold down-regulation in ctx+ cells in relation ctx-, and a 1.59-fold up-regulation in relation to ctrl (Figure 9). Since some exclusive LEA proteins related transcripts were found differentially regulated between non-inoculated conditions (Myc-/Ctrl vs. Myc-/Strss) (Supplementary Table S4), with a down-regulation in water deficit treated roots, the accumulation of LEA5 transcripts in cortical tissues would offer a potential mechanism for drought tolerance in mycorrhizal roots.
Another important molecular function in drought-stress response is the regulation mediated by the binding of TFs into the cis-regulatory promoter region of downstream stress-responsive genes (Joshi et al., 2016). The TF activity was exclusively enriched by the Myc-/Ctrl vs. Myc-/Strss comparison, exhibiting an over-representation of TF-related transcripts (Figure 5B). Six TFs were chosen for our LCM/RT-qPCR analysis and distinct patterns of up-/down-regulation between cortical cells were found, probably due to the diversity of metabolic functions they are associated with. Transcripts of bZIP and bhlh95 were not amplified in ctx- cells (Supplementary Figure S2). bZIP holds a basic region/leucine zipper motif and in common bean roots were enrolled in drought tolerance (Rodriguez-Uribe and O’Connell, 2006). This gene showed the highest level of differential regulation between two samples with a 79.70-fold up-regulation in ctx+ cells in relation to ctrl. bhlh95, a putative circadian-clock protein, a time-keeping mechanism used to coordinate the physiology of an organism to its surrounding environment (Uno et al., 2000; Xue and Loveridge, 2004), was down-regulated by -4.75-fold in ctx+ in relation to ctl. The MADS-box TF-related SRF transcripts were only quantified in AM-root tissues with a -2.9-fold down-regulation in ctx+ cells in relation to ctx- (Figure 9). This same pattern was observed for another MADS-box TF gene in M. truncatula roots colonized by the AMF G. intraradices (Gaude et al., 2012), indicating the relevance of this transcripts accumulation in neighboring epidermal cells during AM colonization. The two No-apical meristem-related genes, PvNAM4 and PvNAM28, both belonging to the stress-responsive superfamily of TFs NAC (NAM/Ataf/CUC) (Puranik et al., 2012; Recchia et al., 2013), were detected in the three cell types (Supplementary Figure S2), although differential analysis was only possible for PvNAM4 with a similar pattern of regulation of LEA5. The ZFHD, that encodes a zinc-finger homo-domain dimerization protein associated with the regulation of plant development, was the only one detected in all cell types analyzed as up-regulated in the ctx+ cells in relation to both ctx- (2.1-fold) and ctrl (1.4-fold) samples (Figure 9).
Genes putatively associated with nucleolar pre-rRNA processing (Utp23) (Rempola et al., 2006), ribosome targeting to the ER membrane (PvNAC4) (Wiedmann and Prehn, 1999), Ran-GTP accumulation around chromosomes and coordination of mitotic spindle formation (RCC1) (Makde et al., 2011), and transposase activity (hAT) (Rubin et al., 2001) were also tested (Figure 9) providing additional insights about the range of arbuscular mycorrhizal impact over cellular metabolism covering localized protein biosynthesis, mediated by ribosomal assembly and transport, and regulation of cell cycle and DNA recombination.
The CKX transcript, a cytokinin dehydrogenase, was not amplified in ctx- cells (Supplementary Figure S2), with a 2.53-fold up-regulation in ctx+ cells in relation to ctrl (Figure 9B). Cosme et al. (2016) indicated that reduced CK levels in roots not only caused root and shoot growth depression, but also impaired AMF colonization and suggested that root CK might regulate the carbon availability from the roots to the fungus during stressful conditions.
Terpenoid biosynthesis occurs within specific tissues, with its regulation induced in response to herbivore feeding, attack by pathogen or abiotic stresses (Singh and Sharma, 2015). The TPS (terpene synthase) gene was 45.4-fold up-regulated in ctx+ cells in relation to ctx- (Figure 9A). This gene is potentially associated with the endogenous regulation of another important plant hormone, the brassinosteroid strigolactone, implicated in the signaling communication established between both partners during the symbiosis (Martín-Rodríguez et al., 2011). Additionally, this gene was down-regulated in ctx+ relative to ctrl, indicating a reduction in terpenoids concentration in arbusculated cells during water deficit.
The colonization process in AM plants is not a synchronized event, and after an initial step of colonization of the root cortex, secondary events of infection are initiated and the invasion process is restarted (Figure 1D; Foo et al., 2013). Therefore, a series of genes related to cell-wall, membrane, and cytosolic organization; apoptosis initiation; cellular division; and developmental progression are differentially regulated during the symbiosis. An α/β hydrolase, ABHD, probably enrolled in cell-wall organization or biogenesis, was not amplified in ctx- cells (Supplementary Figure S2), with a -2.43-fold down-regulation in ctx+ cells in relation to the ctrl (Figure 9B). Apg9, related to addressing cytosolic compounds to autophagosomes (Noda et al., 2000), was up-regulated in ctx+ cells in relation to both other cell types, ctx- and ctrl (Figure 9).
The fact that the spread of mycelium occurs only in the root cortex suggests that the plant may exert a modulated control over fungal proliferation, and although typical structure defense barriers such as papillae or wall appositions containing callose, phenolic compounds, or lignin are not elicited, other elements such as phenylpropanoid biosynthesis, enzymes involved in ROS scavenging, and the regulation of PR genes have been described (Hause and Fester, 2005). From the DEG associated with defense and response to pathogenicity, NB-ARC-LRR, a pathogen-associated molecular patterns (PAMP) receptor, associated to apoptosome formation and programed cell death (DeYoung and Innes, 2007), and GDH, a glucose-dehydrogenase belonging to the (GMC)-oxidoreductase family, a powerful biocontrol agent in plants (Kawabe et al., 2011), were both up-regulated in ctx+ cells in relation to ctrl, with NB-ARC-LRR also up-regulated in relation to ctx- (Figure 9). The GH3, coding for a glucan 1,3-β-glucosidase, an abundant PR2-class protein involved in cell division, trafficking of materials through plasmodesmata, abiotic stress tolerance, flower maturation, and defense against fungal pathogens (Balasubramanian et al., 2012), was amplified only in ctx+ cell (Supplementary Figure S2). Additionally, the lipid-transfer protein (LTP) family LTP, a protease inhibitor containing a LTP2 domain associated with lipid movement and solubilization between membranes, involved in a series of processes like pathogen-defense reactions and adaptation to environmental changes (Kader, 1997), was repressed in ctx+ cells in relation to ctrl, although up-regulated when compared to ctx-. These results corroborate previous observations that the process of plant-AMF recognition involves similar mechanisms related to pathogen elicitation and infection response, probably inducing a series of systemic responses which could be sufficient for a priming effect in secondary stress events (Hause and Fester, 2005).
Conclusion
Although AMF colonization revealed no significant phenotypical alterations in common bean plants during a water deficit event, differential gene expression analyses of NGS data offered the evidences of a profound shift in the transcriptional regulation profiles of these plants that might have a considerable impact over some key metabolic pathways associated with drought response. Complementary analyses pointed aquaporin genes as potential targets for this differential regulation and that in roots, substantial AMF influence over common bean’s transcriptome profiles might occur between 48 and 72 h. After that periods, AMF influences were more intense in leaves, with eight aquaporins, out of nine, exhibiting up-regulation in water deficit treated AMF-inoculated plants in relation to non-inoculated ones. In addition, we also provide the basis for the existence of a controlled mechanism, mediated by the presence of arbuscules at root cortical cells that might exert both local and systemic influence over plants gene expression profiles.
Author Contributions
GR, EK, and ST conceived and designed the experiments. GR, EK, and FC performed and analyzed the greenhouse water deficit assay. GR performed the RNA-Seq experiment and bioinformatic analysis. GR and FC performed light-microscopy and LCM analysis. GR, EK, and DC were responsible for the design, execution, and analysis of all RT-qPCR experiments. All authors were involved in this article writing. ST revised the paper critically for important intellectual content. GR, EK, FC, DC, and ST read and approved the final version of the manuscript.
Funding
This work was funded by the São Paulo Research Foundation (FAPESP Process Number: 2011/12485-3).
Conflict of Interest Statement
The authors declare that the research was conducted in the absence of any commercial or financial relationships that could be construed as a potential conflict of interest.
Acknowledgments
We would like to thank the Laboratory of Soil Microbiology (ESALQ/USP, Piracicaba – Brazil), for providing the AMF inoculums, Prof. Dr. Luiz L. Coutinho from the Laboratory of Animal Biotechnology (ESALQ/USP, Piracicaba – Brazil) for the RNA-sequencing, Prof. Dr. Adriana Pinheiro Martinelli and Mônica Lanzoni Rossi from the Laboratory of Plant Biotechnology (CENA/USP, Piracicaba – Brazil) for helping with samples preparation for light microscopy, and the Department of Oral Diagnosis of FOP-UNICAMP (Piracicaba – Brazil) for helping with samples preparation for LCM analysis.
Supplementary Material
The Supplementary Material for this article can be found online at: https://www.frontiersin.org/articles/10.3389/fmicb.2018.01339/full#supplementary-material
FIGURE S1 | Soil moisture content and root arbuscular mycorrhizal fungi colonization rate. (a) Moisture content (%) of soil samples collected from pots containing plants after at the beginning of irrigation interruption (0), and after 24, 48, 72, and 96 h of water deprivation. (b) Average AMF colonization rates (%) of fresh roots harvested from the four biological samples from each treatment. Myc+/Stress, AM plants under 96 h of water deficit; Myc+/Ctrl, AM plants under regular irrigation control; Myc-/Stress, no-AM plants under 96 h of water deficit; Myc-/Ctrl, no-AM plants under regular irrigation control.
FIGURE S2 | Amplification products. (a) Amplification products obtained after transcripts amplification through RT-qPCR. (b) Amplification stability test for the housekeeping gene skip16 applied as an internal normalizer in relative expression analysis. (c) Amplification of AMF1/AMF2 primers (endogenous control) for each cell-type considering biological triplicates. Legends: ctx+, arbusculated root cortical cells from Myc+/Strss plants; ctx-, non-arbusculated neighboring root cortical cells from Myc+/Strss plants; ctrl, root cortical cells from Myc-/Strss plants. The absence of bands indicate no-amplification of a given transcript.
TABLE S1 | General RNA-Seq statistics. Number of raw reads obtained after paired-end sequencing. Total amount (%) of reads after quality-check and filtration of raw data. Number of reads mapped using the P. vulgaris reference genome v.11.0. Mapping Statistics. NCBI’s SRA Submission ID.
TABLE S2 | Primers designed for cell-type RT-qPCR analysis. Primers name, amplicon gene annotation according to P. vulgaris public database, nucleotide sequence, annealing temperature (°C), GC(%) content, and amplicon size (bp).
TABLE S3 | Complete set of DEGs obtained after treatments comparison. Four statistical comparisons were obtained and are shown in separated sheets: Myc+/Ctrl × Myc+/Strss (AMF-inoculated, control vs. water deficit); Myc-/Ctrl × Myc-/Strss (non-inoculated, control vs. water deficit); Myc-/Strss × Myc+/Strss (water deficit, AMF-inoculated vs. non-inoculated); Myc-/Ctrl vs. Myc+/Ctrl (control, AMF-inoculated vs. non-inoculated) (separated in different spreadsheets). DEGs were annotated following P. vulgaris reference genome (v.11.0). Relative gene expression values are indicated in log2FoldChange. DEGs are ordered according to adjusted p-value at a false-discovery rate (FRD) <0.05 (FDR p-value).
TABLE S4 | List of DEGs that are unique for each statistical comparison: Myc+/Ctrl × Myc+/Strss (AMF-inoculated, control vs. water deficit); Myc-/Ctrl × Myc-/Strss (non-inoculated, control vs. water deficit); Myc-/Strss × Myc+/Strss (water deficit, AMF-inoculated vs. non-inoculated); Myc-/Ctrl vs. Myc+/Ctrl (control, AMF-inoculated vs. non-inoculated) (separated in different spreadsheets). Relative gene expression values are indicated in log2FoldChange. DEGs are ordered according to adjusted p-value at a FRD <0.05 (FDR p-value).
TABLE S5 | Enrichment analysis of ontology terms. Test set: list of DEGs retrieved after the statistical comparisons: Myc+/Ctrl × Myc+/Strss (AMF-inoculated, control vs. water deficit); Myc-/Ctrl × Myc-/Strss (non-inoculated, control vs. water deficit); Myc-/Ctrl vs. Myc+/Ctrl (control, AMF-inoculated vs. non-inoculated) (separated in different spreadsheets). Reference set: total set of Phaseolus vulgaris transcripts. Enriched ontology terms are defined according to Gene Ontology ids. C, cellular component; F, molecular function; P, biological process. Data are organized according to p-value adjusted with a FDR <0.01.
TABLE S6 | Relative expression levels of the 41 P. vulgaris aquaporin-related genes after differential analysis of RNA-Seq data. Two statistical comparisons are provided: Myc+/Ctrl × Myc+/Strss (AMF-inoculated, control vs. water deficit); Myc-/Ctrl × Myc-/Strss (non-inoculated, control vs. water deficit) (separated in different spreadsheets). Genes highlighted in red are up-regulated at control conditions and in green are down-regulated. Genes that are not-highlighted genes did not show statistical significant changes (p-value at a FRD <0.05). Relative gene expression values are indicated in log2FoldChange.
Footnotes
- ^HTTP://imagej.nih.gov/ij
- ^https://cran.r-project.org/web/packages/laercio/index.html
- ^https://github.com/ibest/seqyclean
- ^http://www.ncbi.nlm.nih.gov/VecScreen/UniVec.html
- ^http://phytozome.jgi.doe.gov/pz/portal.html
- ^http://www.ncbi.nlm.nih.gov/sra/SRP070570
- ^http://jgi.doe.gov/
- ^http://www-huber.embl.de/users/anders/HTSeq/doc/index.html
- ^http://www.ncbi.nlm.nih.gov/books/NBK52640/
- ^www.ncbi.nlm.nih.gov, accessed in: 12/11/2013
- ^www.geneontology.org
- ^http://bioinfo.ut.ee/primer3-0.4.0/
- ^www.premierbiosoft.com/netprimer
References
Alexandersson, E., Danielson, J., Rade, J., Moparthi, V., Fontes, M., Kjellbom, P., et al. (2010). Transcriptional regulation of aquaporins in accessions of Arabidopsis in response to drought stress. Plant J. 61, 650–660. doi: 10.1111/j.1365-313X.2009.04087.x
Ariani, A., and Gepts, P. (2015). Genome-wide identification and characterization of aquaporin gene family in common bean (Phaseolus vulgaris L.). Mol. Genet. Genomics 290, 1771–1785. doi: 10.1007/s00438-015-1038-2
Aroca, R., Bago, A., Sutka, M., Paz, J. A., Cano, C., Amodeo, G., et al. (2009). Expression analysis of the first arbuscular mycorrhizal fungi aquaporin described reveals concerted gene expression between salt-stressed and nonstressed mycelium. Mol. Plant Microbe Interact. 22, 1169–1178. doi: 10.1094/MPMI-22-9-1169
Aroca, R., Porcel, R., and Ruiz-lozano, J. M. (2006). How does arbuscular mycorrhizal symbiosis regulate root hydraulic properties and plasma membrane aquaporins in Phaseolus vulgaris under drought, cold or salinity stresses? New Phytol. 173, 808–816. doi: 10.1111/j.1469-8137.2006.01961.x
Aroca, R., Porcel, R., and Ruiz-Lozano, J. M. (2007). How does arbuscular mycorrhizal symbiosis regulate root hydraulic properties and plasma membrane aquaporins in Phaseolus vulgaris under drought, cold or salinity stresses? New Phytol. 173, 808–816. doi: 10.1111/j.1469-8137.2006.01961.x
Augé, R. (2001). Water relations, drought and vesicular-arbuscular mycorrhizal symbiosis. Mycorrhiza 11, 3–42. doi: 10.1007/s005720100097
Augé, R. M., Sylvia, D. M., Park, S., Buttery, B. R., Saxton, A. M., Moore, J. L., et al. (2004). Partitioning mycorrhizal influence on water relations of Phaseolus vulgaris into soil and plant components. Can. J. Bot. 82, 503–514. doi: 10.1139/b04-020
Azcón-Aguilar, C., and Barea, J. M. (1997). Applying mycorrhiza biotechnology to horticulture: significance and potentials. Sci. Hortic. 68, 1–24. doi: 10.1016/S0304-4238(96)00954-5
Bago, B., Pfeffer, P. E., Abubaker, J., Jun, J., Allen, J. W., Brouillette, J., et al. (2003). Carbon export from arbuscular mycorrhizal roots involves the translocation of carbohydrate as well as lipid. Plant Physiol. 131, 1496–1507. doi: 10.1104/pp.102.007765
Balasubramanian, V., Vashisht, D., Cletus, J., and Sakthivell, N. (2012). Plant β-1, 3-glucanases?: their biological functions and transgenic expression against phytopathogenic fungi. Biotechnol. Lett. 34, 1983–1990. doi: 10.1007/s10529-012-1012-6
Balestrini, R., Gómez-Ariza, J., Lanfranco, L., and Bonfante, P. (2007). Laser microdissection reveals that transcripts for five plant and one fungal phosphate transporter genes are contemporaneously present in arbusculated cells. Mol. Plant. Microbe Interact. 20, 1055–1062. doi: 10.1094/MPMI-20-9-1055
Balestrini, R., and Lanfranco, L. (2006). Fungal and plant gene expression in arbuscular mycorrhizal symbiosis. Mycorrhiza 16, 509–524. doi: 10.1007/s00572-006-0069-2
Balzergue, C., Chabaud, M., Barker, D. G., Bécard, G., and Rochange, S. F. (2013). High phosphate reduces host ability to develop arbuscular mycorrhizal symbiosis without affecting root calcium spiking responses to the fungus. Front. Plant Sci. 4:426. doi: 10.3389/fpls.2013.00426
Barea, J., Pozo, M., Azcón, R., and Azcón-Aguilar, C. (2005). Microbial co-operation in the rhizosphere. J. Exp. Bot. 56, 1761–1778. doi: 10.1093/jxb/eri197
Barrs, H., and Weatherley, P. (1962). A re-examination of the relative turgidity technique for estimating water deficits in leaves. Aust. J. Biol. Sci. 15, 413–428. doi: 10.1071/BI9620413
Bárzana, G., Aroca, R., Bienert, G. P., Chaumont, F., and Ruiz-Lozano, J. M. (2014). New insights into the regulation of aquaporins by the arbuscular mycorrhizal symbiosis in maize plants under drought stress and possible implications for plant performance. Mol. Plant Microbe Interact. 27, 349–363. doi: 10.1094/MPMI-09-13-0268-R
Bárzana, G., Aroca, R., Paz, J. A., Chaumont, F., Martinez-Ballesta, M. C., Carvajal, M., et al. (2012). Arbuscular mycorrhizal symbiosis increases relative apoplastic water flow in roots of the host plant under both well-watered and drought stress conditions. Ann. Bot. 109, 1009–1017. doi: 10.1093/aob/mcs007
Bárzana, G., Aroca, R., and Ruiz-lozano, J. M. (2015). Localized and non-localized effects of arbuscular mycorrhizal symbiosis on accumulation of osmolytes and aquaporins and on antioxidant systems in maize plants subjected to total or partial root drying. Plant Cell Environ. 38, 1613–1627. doi: 10.1111/pce.12507
Beebe, S., Rao, I. M., Blair, M. W., and Butare, L. (2009). Breeding for abiotic stress tolerance in common bean: present and future challenges. SABRAO J. Breed. Genet. 41, 1–11.
Benjamini, Y., and Hochberg, Y. (1995). Controlling the false discovery rate: a practical and powerful approach to multiple testing. J. R. Stat. Soc. 57, 289–300. doi: 10.2307/2346101
Borges, A., Tsai, S. M., and Caldas, D. G. (2012). Validation of reference genes for RT-qPCR normalization in common bean during biotic and abiotic stresses. Plant Cell Rep. 31, 827–838. doi: 10.1007/s00299-011-1204-x
Brundrett, M., Bougher, N., Dell, B., Grove, T., and Malajczuk, N. (1996). in Working with Mycorrhizas in Forestry and Agriculture, 1st Edn, ed. P. Lynch Canberra (Canberra, ACT: Australian Centre for International Agricultural Research).
CIAT (1989). Bean Production Problems in the Tropics, 2nd Edn, eds H. Schwartz and M. Pastor-Corrales Cali (Cali: Centro Internacional de Agricultura Tropical (CIAT)).
Colmenero-Flores, J. M., Campos, F., Garciarrubio, A., and Covarrubias, A. A. (1997). Characterization of Phaseolus vulgaris cDNA clones responsive to water deficit: identification of a novel late embryogenesis abundant-like protein. Plant Mol. Biol. 35, 393–405. doi: 10.1023/A:1005802505731
Conesa, A., Götz, S., García-Gómez, J. M., Terol, J., Talón, M., and Robles, M. (2005). Blast2GO: a universal tool for annotation, visualization and analysis in functional genomics research. Bioinformatics 21, 3674–3676. doi: 10.1093/bioinformatics/bti610
Cosme, M., Ramireddy, E., Franken, P., Schmülling, T., and Wurst, S. (2016). Shoot- and root-borne cytokinin influences arbuscular mycorrhizal symbiosis. Mycorrhiza 26, 709–720. doi: 10.1007/s00572-016-0706-3
Cozzolino, V., Meo, V., and Di Piccolo, A. (2013). Impact of arbuscular mycorrhizal fungi applications on maize production and soil phosphorus availability. J. Geochem. Explor. 129, 40–44. doi: 10.1016/j.gexplo.2013.02.006
Desai, N. A., and Shankar, V. (2003). Single-strand-specific nucleases. FEMS Microbiol. Rev. 26, 457–491. doi: 10.1111/j.1574-6976.2003.tb00626.x
Dewar, R. C. (1997). A simple model of light and water use evaluated for Pinus radiata. Tree Physiol. 17, 259–265. doi: 10.1093/treephys/17.4.259
DeYoung, B., and Innes, R. (2007). Plant NBS-LRR proteins in pathogen sensing and host defense. Nat. Immunol. 7, 1243–1249. doi: 10.1038/ni1410.Plant
Dolce, V., Cappello, A. R., and Capobianco, L. (2014). Critical review mitochondrial tricarboxylate and dicarboxylate – tricarboxylate carriers?: from animals to plants. IUBMB Life 66, 462–471. doi: 10.1002/iub.1290
Feder, N., and O’Brien, T. (1968). Plant microtechnique: some principles and new methods. Am. J. Bot. 55, 123–142. doi: 10.1038/147222b0
Fiorilli, V., Catoni, M., Miozzi, L., Novero, M., Accotto, G. P., and Lanfranco, L. (2009). Global and cell-type gene expression profiles in tomato plants colonized by an arbuscular mycorrhizal fungus. New Phytol. 184, 975–987. doi: 10.1111/j.1469-8137.2009.03031.x
Foo, E., Ross, J. J., Jones, W. T., and Reid, J. B. (2013). Plant hormones in arbuscular mycorrhizal symbioses?: an emerging role for gibberellins. Ann. Bot. 111, 769–779. doi: 10.1093/aob/mct041
Fujimoto, S., Matsunaga, S., Yonemura, M., Uchiyama, S., Azuma, T., and Fukui, K. (2004). Identification of a novel plant MAR DNA binding protein localized on chromosomal surfaces. Plant Mol. Biol. 56, 225–239. doi: 10.1007/s11103-004-3249-5
Fujimoto, S. Y., Ohta, M., Usui, A., Shinshi, H., and Ohme-takagi, M. (2000). Arabidopsis ethylene-responsive element binding factors act as transcriptional activators or repressors of GCC Box – mediated gene expression. Plant Cell 12, 393–404. doi: 10.1105/tpc.12.3.393
Galeano, C., Gomez, M., Rodriguez, L., and Blair, M. (2008). CEL I nuclease digestion for SNP discovery and marker development in common bean (Phaseolus vulgaris L.). Crop Sci. Soc. Am. 49, 381–394. doi: 10.2135/cropsci2008.07.0413
Gaude, N., Bortfeld, S., Duensing, N., Lohse, M., and Krajinski, F. (2012). Arbuscule-containing and non-colonized cortical cells of mycorrhizal roots undergo extensive and specific reprogramming during arbuscular mycorrhizal development. Plant J. 69, 510–528. doi: 10.1111/j.1365-313X.2011.04810.x
Glisovic, T., Bachorik, J. L., Yong, J., and Dreyfuss, G. (2008). RNA-binding proteins and post-transcriptional gene regulation. FEBS Lett. 582, 1977–1986. doi: 10.1016/j.febslet.2008.03.004
Gomez, S. K., Javot, H., Deewatthanawong, P., Torres-Jerez, I., Tang, Y., Blancaflor, E. B., et al. (2009). Medicago truncatula and Glomus intraradices gene expression in cortical cells harboring arbuscules in the arbuscular mycorrhizal symbiosis. BMC Plant Biol. 9:10. doi: 10.1186/1471-2229-9-10
Gong, M., You, X., and Zhang, Q. (2015). Effects of Glomus intraradices on the growth and reactive oxygen metabolism of foxtail millet under drought. Ann. Microbiol. 65, 595–602. doi: 10.1007/s13213-014-0895-y
Graham, P. H., and Ranalli, P. (1997). Common bean (Phaseolus vulgaris L.). Field Crop Res. 53, 131–146. doi: 10.1016/S0378-4290(97)00112-3
Guether, M., Balestrini, R., Hannah, M., He, J., Udvardi, M. K., and Bonfante, P. (2009). Genome-wide reprogramming of regulatory networks, transport, cell wall and membrane biogenesis during arbuscular mycorrhizal symbiosis in Lotus japonicus. New Phytol. 182, 200–212. doi: 10.1111/j.1469-8137.2008.02725.x
Güimil, S., Chang, H., Zhu, T., Sesma, A., Osbourn, A., Roux, C., et al. (2005). Comparative transcriptomics of rice reveals an ancient pattern of response to microbial colonization. Proc. Natl. Acad. Sci. U.S.A. 102, 8066–8070. doi: 10.1073/pnas.0502999102
Handa, Y., Nishide, H., Takeda, N., Suzuki, Y., Kawaguchi, M., and Saito, K. (2015). RNA-seq transcriptional profiling of an arbuscular mycorrhiza provides insights into regulated and coordinated gene expression in lotus japonicus and rhizophagus irregularis. Plant Cell Physiol. 56, 1490–1511. doi: 10.1093/pcp/pcv071
Harrison, M. J. (2005). Signaling in the arbuscular mycorrhizal symbiosis. Annu. Rev. Microbiol. 59, 19–42. doi: 10.1146/annurev.micro.58.030603.123749
Harrison, M. J., Dewbre, G. R., Liu, J., Samuel, T., Noble, R., and Parkway, S. N. (2002). A phosphate transporter from Medicago truncatula involved in the acquisition of phosphate released by arbuscular mycorrhizal fungi. Plant Cell 14, 2413–2429. doi: 10.1105/tpc.004861.the
Hause, B., and Fester, T. (2005). Molecular and cell biology of arbuscular mycorrhizal symbiosis. Planta 221, 184–196. doi: 10.1007/s00425-004-1436-x
Herrera-Medina, M. J., Steinkellner, S., Vierheilig, H., and Ocampo Bote, J. A. (2007). Abscisic acid determines arbuscule development and functionality in the tomato arbuscular mycorrhiza. New Phytol. 175, 554–564. doi: 10.1111/j.1469-8137.2007.02107.x
Hoagland, D., and Arnon, D. (1950). The Water-Culture Method for Growing Plants without Soil. Berkeley, CA: University of California, Berkeley.
Hogekamp, C., Arndt, D., Pereira, P. A., Becker, J. D., Hohnjec, N., and Kuster, H. (2011). Laser microdissection unravels cell-type-specific transcription in arbuscular mycorrhizal roots, including CAAT-Box transcription factor gene expression correlating with fungal contact and spread. Plant Physiol. 157, 2023–2043. doi: 10.1104/pp.111.186635
Javot, H., and Maurel, C. (2002). The role of aquaporins in root water uptake. Ann. Bot. 90, 301–313. doi: 10.1093/aob/mcf199
Joshi, R., Wani, S. H., Singh, B., Bohra, A., Dar, Z. A., Lone, A. A., et al. (2016). Transcription factors and plants response to drought stress?: current understanding and future directions. Front. Plant Sci. 7:1029. doi: 10.3389/fpls.2016.01029
Kader, J. (1997). Lipid-transfer proteins?: a puzzling family of plant proteins. Trends Plant Sci. 2, 66–70. doi: 10.1016/S1360-1385(97)82565-4
Kawabe, M., Onokubo, A. O., Arimoto, Y., Yoshida, T., Azegami, K., Teraoka, T., et al. (2011). GMC oxidoreductase, a highly expressed protein in a potent biocontrol agent Fusarium oxysporum Cong?: 1-2, is dispensable for biocontrol activity. J. Gen. Appl. Microbiol. 57, 207–217. doi: 10.2323/jgam.57.207
Kim, J.-S., Mizoi, J., Yoshida, T., Fujita, Y., Nakajima, J., Ohori, T., et al. (2018). An ABRE promoter sequence is involved in osmotic stress-responsive expression of the DREB2A gene, which encodes a transcription factor regulating drought-inducible genes in Arabidopsis. Plant Cell Physiol. 52, 2136–2146. doi: 10.1093/pcp/pcr143
Kozbial, P. Z., and Mushegian, A. R. (2005). Natural history of S -adenosylmethionine-binding proteins. BMC Struct. Biol. 5:19. doi: 10.1186/1472-6807-5-19
Krajinski, F., Biela, A., Schubert, D., Gianinazzi-Pearson, V., Kaldenhoff, R., and Franken, P. (2000). Arbuscular mycorrhiza development regulates the mRNA abundance of Mtaqp1 encoding a mercury-insensitive aquaporin of Medicago truncatula. Planta 211, 85–90. doi: 10.1007/s004250000263
Langmead, B., and Salzberg, S. L. (2012). Fast gapped-read alignment with Bowtie 2. Nat. Methods 9, 357–359. doi: 10.1038/nmeth.1923
Lee, B. R., Muneer, S., Avice, J. C., Jung, W. J., and Kim, T. H. (2012). Mycorrhizal colonisation and P-supplement effects on N uptake and N assimilation in perennial ryegrass under well-watered and drought-stressed conditions. Mycorrhiza 22, 525–534. doi: 10.1007/s00572-012-0430-6
Lee, J., Lee, S., and Young, J. P. (2008). Improved PCR primers for the detection and identification of arbuscular mycorrhizal fungi. FEMS Microbiol. Ecol. 65, 339–349. doi: 10.1111/j.1574-6941.2008.00531.x
Liu, H., Sun, W., Su, W., and Tang, Z. (2006). Co-regulation of water channels and potassium channels in rice. Physiol. Plant. 128, 58–69. doi: 10.1111/j.1399-3054.2006.00709.x
Love, M. I., Huber, W., and Anders, S. (2014). Moderated estimation of fold change and dispersion for RNA-seq data with DESeq2. Genome Biol. 15:550. doi: 10.1186/s13059-014-0550-8
Ludwig, Y., and Hochholdinger, F. (2014). “Laser microdissection of plant cells,” in Plant Cell Morphogenesis. Methods and Protocols, eds V. Žárský and F. Cvrèková (New York, NY: Springer Science+Business Media), 249–258. doi: 10.1007/978-1-62703-643-6
Ludwig-Müller, J. (2010). “Hormonal responses in host plants triggered by arbuscular mycorrhizal fungi,” in Arbuscular Mycorrhizas: Physiology and Function, eds H. Koltai and Y. Kapulnik (Dordrecht: Springer), 169–190.
Makde, R. D., England, J. R., Yennawar, H. P., and Tan, S. (2011). Structure of RCC1 chromatin factor bound to the nucleosome core particle. Nature 467, 562–566. doi: 10.1038/nature09321.Structure
Martín-Rodríguez, J., Leon-Morcillo, R., Vierheilig, H., Ocampo, J., Luwig-Müller, J., and García-Garrido, J. (2011). Ethylene-dependent / ethylene-independent ABA regulation of tomato plants colonized by arbuscular mycorrhiza fungi. New Phytol. 190, 193–205. doi: 10.1111/j.1469-8137.2010.03610.x
Martín-Rodríguez, J. A., Huertas, R., Ho-Plágaro, T., Ocampo, J. A., Turecková, V., Tarkowská, D., et al. (2016). Gibberellin – abscisic acid balances during arbuscular mycorrhiza formation in tomato. Front. Plant Sci. 7:1273. doi: 10.3389/fpls.2016.01273
Maurel, C., Verdoucq, L., Luu, D.-T., and Santoni, V. (2008). Plant aquaporins: membrane channels with multiple integrated functions. Annu. Rev. Plant Biol. 59, 595–624. doi: 10.1146/annurev.arplant.59.032607.092734
McConnell, M., Mamidi, S., Lee, R., Chikara, S., Rossi, M., Papa, R., et al. (2010). Syntenic relationships among legumes revealed using a gene-based genetic linkage map of common bean (Phaseolus vulgaris L.). Theor. Appl. Genet. 121, 1103–1116. doi: 10.1007/s00122-010-1375-9
Miliara, X., Garnett, J. A., Tatsuta, T., Ali, F. A., Baldie, H., Simpson, P., et al. (2015). Structural insight into the TRIAP 1 / PRELI-like domain family of mitochondrial phospholipid transfer complexes. EMBO Rep. 16, 824–835. doi: 10.15252/embr.201540229
Miransari, M., Bahrami, H. A., Rejali, F., Malakouti, M. J., and Torabi, H. (2007). Using arbuscular mycorrhiza to reduce the stressful effects of soil compaction on corn (Zea mays L.) growth. Soil Biol. Biochem. 39, 2014–2026. doi: 10.1016/j.soilbio.2007.02.017
Niu, L., Zhang, Y., Pei, Y., Liu, C., and Cao, X. (2008). Redundant requirement for a Pair of PROTEIN ARGININE METHYLTRANSFERASE4 homologs for the proper regulation of Arabidopsis flowering time 1 [ C ][ OA ]. Plant Physiol. 148, 490–503. doi: 10.1104/pp.108.124727
Noda, T., Kim, J., Huang, W., Baba, M., Tokunaga, C., Ohsumi, Y., et al. (2000). Apg9p / Cvt7p is an integral membrane protein required for transport vesicle formation in the Cvt and autophagy pathways. J. Cell Biol. 148, 465–479. doi: 10.1083/jcb.148.3.465
Oldroyd, G., Harrison, M., and Paszkowski, U. (2014). Reprograming plant cells for endosymbiosis. Science 324, 753–755. doi: 10.1126/science.1171644
Parniske, M. (2008). Arbuscular mycorrhiza: the mother of plant root endosymbioses. Nat. Rev. Microbiol. 6, 763–775. doi: 10.1038/nrmicro1987
Parry, M., Canziani, O., Palutikof, J., van der Linden, P., and Hanson, C. (2007). Climate Change 2007: Impacts, Adaptation and Vulnerability. Cambridge: Cambridge University Press.
Pfaffl, M. W. (2001). A new mathematical model for relative quantification in real-time RT-PCR. Nucleic Acids Res. 29:e45. doi: 10.1093/nar/29.9.e45
Porcel, R., Aroca, R., Azcón, R., and Ruiz-Lozano, J. M. (2006). PIP aquaporin gene expression in arbuscular mycorrhizal Glycine max and Lactuca sativa plants in relation to drought stress tolerance. Plant Mol. Biol. 60, 389–404. doi: 10.1007/s11103-005-4210-y
Porcel, R., Aroca, R., and Ruiz-Lozano, J. M. (2012). Salinity stress alleviation using arbuscular mycorrhizal fungi. A review. Agron. Sustain. Dev. 32, 181–200. doi: 10.1007/s13593-011-0029-x
Porcel, R., Azcón, R., and Ruiz-Lozano, J. M. (2005). Evaluation of the role of genes encoding for dehydrin proteins (LEA D-11) during drought stress in arbuscular mycorrhizal Glycine max and Lactuca sativa plants. J. Exp. Bot. 56, 1933–1942. doi: 10.1093/jxb/eri188
Porcel, R., and Ruiz-Lozano, J. M. (2004). Arbuscular mycorrhizal influence on leaf water potential, solute accumulation, and oxidative stress in soybean plants subjected to drought stress. J. Exp. Bot. 55, 1743–1750. doi: 10.1093/jxb/erh188
Pumplin, N., and Harrison, M. J. (2009). Live-Cell imaging reveals periarbuscular membrane domains and organelle location in Medicago truncatula roots during arbuscular Mycorrhizal Symbiosis. Plant Physiol. 151, 809–819. doi: 10.1104/pp.109.141879
Puranik, S., Sahu, P. P., Srivastava, P. S., and Prasad, M. (2012). NAC proteins: regulation and role in stress tolerance. Trends Plant Sci. 17, 369–381. doi: 10.1016/J.Tplants.2012.02.004
Quach, T. N., Nguyen, H. T., Valliyodan, B., Joshi, T., Xu, D., and Nguyen, H. T. (2015). Genome - wide expression analysis of soybean NF - Y genes reveals potential function in development and drought response. Mol. Genet. Genomics 290, 1095–1115. doi: 10.1007/s00438-014-0978-2
Rao, I. M., Polanía, J., and García, R. (2006). 2006 PROJECT IP - 1 Bean Improvement for the Tropics. Development of a Greenhouse Soil Tube Method to Quantify Phenotypic Differences among Advanced Lines in Root Development and Distribution under Drought Stress. Cali: CIAT.
Rapparini, F., and Peñuelas, J. (2014). “Mycorrhizal fungi to alleviate drought stress on plant growth,” in Use of Microbes for the Alleviation of Soil Stresses, ed. M. Miransari (New York, NY: Springer-Verlag), 1. doi: 10.1007/978-1-4614-9466-9
Recchia, G., Caldas, D., Ahern, A., Silva, M., and Tsai, S. (2013). Transcriptional analysis of drought-induced genes in the roots of a tolerant genotype of the common bean (Phaseolus vulgaris L.). Int. J. Mol. Sci. 14, 7155–7179. doi: 10.3390/ijms14047155
Redecker, D., Kodner, R., and Graham, L. E. (2000). Glomalean fungi from the Ordovician. Science 289, 1920–1921. doi: 10.1126/science.289.5486.1920
Rempola, B., Karkusiewicz, I., Piekarska, I., and Rytka, J. (2006). Fcf1p and Fcf2p are novel nucleolar Saccharomyces cerevisiae proteins involved in pre-rRNA processing. Biochem. Biophys. Res. Commun. 346, 546–554. doi: 10.1016/j.bbrc.2006.05.140
Rodriguez-Uribe, L., and O’Connell, M. A. (2006). A root-specific bZIP transcription factor is responsive to water deficit stress in tepary bean (Phaseolus acutifolius) and common bean (P. vulgaris). J. Exp. Bot. 57, 1391–1398. doi: 10.1093/jxb/erj118
Rosales, M. A., Ocampo, E., Rodríguez-Valentín, R., Olvera-Carrillo, Y., Acosta-Gallegos, J., and Covarrubias, A. A. (2012). Physiological analysis of common bean (Phaseolus vulgaris L.) cultivars uncovers characteristics related to terminal drought resistance. Plant Physiol. Biochem. 56, 24–34. doi: 10.1016/j.plaphy.2012.04.007
Rosales-Serna, R., Kohashi-Shibata, J., Acosta-Gallegos, J. A., Trejo-López, C., Ortiz-Cereceres, J., and Kelly, J. D. (2004). Biomass distribution, maturity acceleration and yield in drought-stressed common bean cultivars. Field Crop Res. 85, 203–211. doi: 10.1016/S0378-4290(03)00161-8
Rubin, E., Lithwick, G., and Levy, A. A. (2001). Structure and evolution of the hAT transposon superfamily. Genetics 158, 949–957.
Ruijter, J. M., Ramakers, C., Hoogaars, W. M., Karlen, Y., Bakker, O., van den Hoff, M. J., et al. (2009). Amplification efficiency: linking baseline and bias in the analysis of quantitative PCR data. Nucleic Acids Res. 37:e45. doi: 10.1093/nar/gkp045
Ruiz-Lozano, J. M. (2003). Arbuscular mycorrhizal symbiosis and alleviation of osmotic stress. New perspectives for molecular studies. Mycorrhiza 13, 309–317. doi: 10.1007/s00572-003-0237-6
Saia, S., Amato, G., Frenda, A. S., Giambalvo, D., and Ruisi, P. (2014). Influence of arbuscular mycorrhizae on biomass production and nitrogen fixation of berseem clover plants subjected to water stress. PLoS One 9:e90738. doi: 10.1371/journal.pone.0090738
Sánchez-Blanco, M. J., Ferrández, T., Morales, M. A., Morte, A., and Alarcón, J. J. (2004). Variations in water status, gas exchange, and growth in Rosmarinus officinalis plants infected with Glomus deserticola under drought conditions. J. Plant Physiol. 161, 675–682. doi: 10.1078/0176-1617-01191
Schmutz, J., McClean, P. E., Mamidi, S., Wu, G. A., Cannon, S. B., Grimwood, J., et al. (2014). A reference genome for common bean and genome-wide analysis of dual domestications. Nat. Genet. 46, 707–713. doi: 10.1038/ng.3008
Schneider, K., Mathur, J., Boudonck, K., Wells, B., Dolan, L., and Roberts, K. (1998). The ROOT HAIRLESS 1 gene encodes a nuclear protein required for root hair initiation in Arabidopsis. Genes Dev. 12, 2013–2021. doi: 10.1101/gad.12.13.2013
Shabala, S., and Pottosin, I. (2014). Regulation of potassium transport in plants under hostile conditions?: implications for abiotic and biotic stress tolerance. Physiol. Plant. 151, 257–279. doi: 10.1111/ppl.12165
Singh, B., and Sharma, R. A. (2015). Plant terpenes?: defense responses, phylogenetic analysis, regulation and clinical applications. 3 Biotech. 5, 129–151. doi: 10.1007/s13205-014-0220-2
Smith, S., and Smith, F. (1990). Structure and function of the interfaces in biotrophic symbioses as they relate to nutrient transport. New Phytol. 114, 1–38. doi: 10.1111/j.1469-8137.1990.tb00370.x
Sponchiado, B. N., White, J. W., and Castillo, J. A. (1989). Research article root growth of four common bean cultivars in relation to drought tolerance in environments with contrasting soil types. Exp. Agric. 25, 249–257. doi: 10.1017/S0014479700016756
Subramanian, K., and Charest, C. (1995). Influence of arbuscular mycorrhizae on the metabolism of maize under drought stress. Mycorrhiza 5, 273–278. doi: 10.1007/BF00204961
Thung, M., and Rao, I. (1999). “Integrated management of abiotic stresses,” in Common Bean Improvement in the Twenty-First Century, ed. S. Singh (Netherlands: Springer), 331–370. doi: 10.1007/978-94-015-9211-6_13
Trapnell, C., Williams, B. A., Pertea, G., Mortazavi, A., Kwan, G., and van Baren, M. J. (2010). Transcript assembly and quantification by RNA-Seq reveals unannotated transcripts and isoform switching during cell differentiation. Nat. Biotechnol. 28, 511–515. doi: 10.1038/nbt.1621
Uehlein, N., Fileschi, K., Eckert, M., Bienert, G. P., Bertl, A., and Kaldenhoff, R. (2007). Arbuscular mycorrhizal symbiosis and plant aquaporin expression. Phytochemistry 68, 122–129. doi: 10.1016/j.phytochem.2006.09.033
Umezawa, T., Fujita, M., Fujita, Y., Yamaguchi-shinozaki, K., and Shinozaki, K. (2006). Engineering drought tolerance in plants?: discovering and tailoring genes to unlock the future. Curr. Opin. Biotechnol. 17, 113–122. doi: 10.1016/j.copbio.2006.02.002
Uno, Y., Furihata, T., Abe, H., Yoshida, R., Shinozaki, K., and Yamaguchi-Shinozaki, K. (2000). Arabidopsis basic leucine zipper transcription factors involved in an abscisic acid-dependent signal transduction pathway under drought and high-salinity conditions. Proc. Natl. Acad. Sci. U.S.A. 97, 11632–11637. doi: 10.1073/pnas.190309197
Valot, B., Dieu, M., Recorbet, G., Gianinazzi, S., and Dumas-Gaudot, E. (2005). Identification of membrane-associated proteins regulated by the arbuscular mycorrhizal symbiosis. Plant Mol. Biol. 59, 568–580. doi: 10.1007/s11103-005-8269-2
Vangelisti, A., Natali, L., Bernardi, R., Sbrana, C., Hassani-pak, K., Hughes, D., et al. (2018). Transcriptome changes induced by arbuscular mycorrhizal fungi in sunflower (Helianthus annuus L.) roots. Sci. Rep. 8, 1–14. doi: 10.1038/s41598-017-18445-0
Wehner, G., Balko, C., Humbeck, K., Zyprian, E., and Ordon, F. (2016). Expression profiling of genes involved in drought stress and leaf senescence in juvenile barley. BMC Plant Biol. 16:3. doi: 10.1186/s12870-015-0701-4
Wiedmann, B., and Prehn, S. (1999). The nascent polypeptide-associated complex ( NAC ) of yeast functions in the targeting process of ribosomes to the ER membrane. FEBS Lett. 458, 51–54. doi: 10.1016/S0014-5793(99)01118-7
Xue, G., and Loveridge, C. W. (2004). HvDRF1 is involved in abscisic acid-mediated gene regulation in barley and produces two forms of AP2 transcriptional activators, interacting preferably with a CT-rich element. Plant J. 37, 326–339. doi: 10.1046/j.1365-313X.2003.01963.x
Zhang, Z., Zhang, J., and Huang, Y. (2014). Effects of arbuscular mycorrhizal fungi on the drought tolerance of Cyclobalanopsis glauca seedlings under greenhouse conditions. New For. 45, 545–556. doi: 10.1007/s11056-014-9417-9
Keywords: arbuscular mycorrhizal fungi, Phaseolus vulgaris, water deficit event, root, RNA-Seq, laser-capture microdissection, aquaporins
Citation: Recchia GH, Konzen ER, Cassieri F, Caldas DGG and Tsai SM (2018) Arbuscular Mycorrhizal Symbiosis Leads to Differential Regulation of Drought-Responsive Genes in Tissue-Specific Root Cells of Common Bean. Front. Microbiol. 9:1339. doi: 10.3389/fmicb.2018.01339
Received: 14 April 2018; Accepted: 31 May 2018;
Published: 21 June 2018.
Edited by:
Raffaella Balestrini, Consiglio Nazionale delle Ricerche (CNR), ItalyReviewed by:
Ricardo Aroca, Estación Experimental del Zaidín (EEZ), SpainWalter Chitarra, Consiglio per la Ricerca in Agricoltura e l’Analisi dell’Economia Agraria (CREA), Italy
Copyright © 2018 Recchia, Konzen, Cassieri, Caldas and Tsai. This is an open-access article distributed under the terms of the Creative Commons Attribution License (CC BY). The use, distribution or reproduction in other forums is permitted, provided the original author(s) and the copyright owner are credited and that the original publication in this journal is cited, in accordance with accepted academic practice. No use, distribution or reproduction is permitted which does not comply with these terms.
*Correspondence: Gustavo H. Recchia, gust_henr@yahoo.com.br