- 1State Key Laboratory of Food Science and Technology, School of Biotechnology, Jiangnan University, Wuxi, China
- 2International Joint Laboratory on Food Safety, Jiangnan University, Wuxi, China
- 3The Wellman Center for Photomedicine, Massachusetts General Hospital, Boston, MA, United States
- 4Department of Dermatology, Harvard Medical School, Boston, MA, United States
- 5Center of Digital Dentistry, Peking University School and Hospital of Stomatology, Beijing, China
- 6Harvard-MIT Division of Health Sciences and Technology, Cambridge, MA, United States
Biofilm describes a microbially-derived sessile community in which microbial cells are firmly attached to the substratum and embedded in extracellular polymeric matrix. Microbial biofilms account for up to 80% of all bacterial and fungal infections in humans. Biofilm-associated pathogens are particularly resistant to antibiotic treatment, and thus novel antibiofilm approaches needed to be developed. Antimicrobial Photodynamic therapy (aPDT) had been recently proposed to combat clinically relevant biofilms such as dental biofilms, ventilator associated pneumonia, chronic wound infections, oral candidiasis, and chronic rhinosinusitis. aPDT uses non-toxic dyes called photosensitizers (PS), which can be excited by harmless visible light to produce reactive oxygen species (ROS). aPDT is a multi-stage process including topical PS administration, light irradiation, and interaction of the excited state with ambient oxygen. Numerous in vitro and in vivo aPDT studies have demonstrated biofilm-eradication or substantial reduction. ROS are produced upon photo-activation and attack adjacent targets, including proteins, lipids, and nucleic acids present within the biofilm matrix, on the cell surface and inside the microbial cells. Damage to non-specific targets leads to the destruction of both planktonic cells and biofilms. The review aims to summarize the progress of aPDT in destroying biofilms and the mechanisms mediated by ROS. Finally, a brief section provides suggestions for future research.
Introduction
One major goal of modern clinical microbiology is to develop effective strategies to treat infections caused by microbial pathogen. Microbial biofilms account for up to 80% of all bacterial and fungal infections in humans (Høiby, 2017). A biofilm is a matrix-embedded microbial community attached to biological or non-biological surfaces (Hall-Stoodley et al., 2004). The microbial cells are embedded in extracellular polymeric substances (EPS) secreted by the cells. Compared to free-floating planktonic cells, biofilm cells exhibit altered physiological and metabolic states (Donlan, 2002). Biofilm formation is crucial for microbial survival in diverse and difficult environments (Hall-Stoodley et al., 2004), and in clinical situations, biofilms lead to difficult-to-eradicate infections by protecting microbes from attack by host defense systems and increasing microbial resistance to many antibiotics and biocides (Stewart, 2003). Biofilms are particularly involved in the pathogenesis of stubborn chronic infectious diseases (Wolcott and Ehrlich, 2008). Therefore, it is imperative to develop new approaches to fight against biofilm infections, and antimicrobial photodynamic therapy (aPDT) has been suggested as an efficient alternative approach. aPDT uses non-toxic photosensitizers (PS) to generate cytotoxic reactive oxygen species (ROS) upon irradiation by harmless visible light at specific wavelength. In this review, we critically summarize the killing effect of aPDT against clinically relevant biofilms in vitro and in vivo in the recent literature, and analyze the multiple underlying mechanisms of action.
Biofilms and Factors Influencing Their Formation
Biofilm is a matrix-embedded microbial population enclosed by a self-secreted EPS, composed of polysaccharides, proteins, extracellular DNA, membrane vesicles, etc (Davey and O'Toole, 2000). Biofilm growth allows a protected lifestyle that allows pathogenic bacteria and fungi to survive in hostile environments (Hall-Stoodley et al., 2004), such as on medical devices and wound surfaces and on living tissues, inside animals or humans (Donlan, 2002). Microbes embedded in biofilm can tolerate 10–1000 times higher levels of antibiotics than their planktonic counterparts (Ceri et al., 1999). Biofilms have attracted increasing attention from researchers over the last 10 years. “Biofilms and Microbiomes,” a new Nature partner journal was launched recently (http://www.stm-publishing.com/open-access-nature-partner-journal-npj-biofilms-and-microbiomes/). The development process of biofilms involves the initial deposition of microbial cells onto a surface, reversible attachment to the substratum, irreversible attachment and colonization, aggregation and expansion, biofilm maturation, and finally biofilm dispersal and controlled detachment. A multidisciplinary review recently reported global understanding of the process of biofilm-formation (Karunakaran et al., 2011). Biofilm-determinants mainly belong to three categories: EPS (the external microbially produced environment), the microbial cell surface, and intracellular biomolecules (Karunakaran et al., 2011). During aPDT-mediated inactivation of biofilm, oxidative damage occurs in this order from external to internal. Thus, the main components of biofilms are described below.
Components Within the Biofilm
Extracellular Polymeric Substances
EPS accounts for at least 90% by weight of the total biofilm content, and contributes to the structural complexity and strength of the biofilm (Chandra et al., 2001), thus EPS represents the first line of defense against diffusion of antibiotics, biocides, and also restricts the penetration of PS (Nett et al., 2010; Billings et al., 2013; Hengzhuang et al., 2013). The main components of EPS include a variety of carbohydrates, proteins, DNA, membrane vesicles, etc. These macromolecules are crucial for the initial adhesion of cells, since EPS can overcome the electrostatic repulsion between microbial cells and the substratum through a “polymer bridging” process and thereby ensure firm attachment or anchoring of microbial cells to the substratum (Neu et al., 2010). Moreover, some EPS molecules can affect the rheological properties and improve stability of the biofilm.
Cell-Surface
The cell surface not only participates in the adherence of microbes to the substratum but also mediates the adhesion between neighboring microbial cells. There exist both similarities and significant differences between the structure and composition of cell walls and cytoplasmic membranes between gram-negative (G−) bacteria, gram-positive (G+) bacteria, and fungi (Figure 2). All of them have cytoplasmic membranes, which consist of a phospholipid bilayer containing embedded proteins. This membrane separates the cell interior from the external environment and is selectively permeable to inorganic ions and some organic molecules, and controls the uptake and excretion of metabolic substances. However, G+ bacteria possess a thick cell wall containing many layers of peptidoglycan and teichoic acids, while G− bacteria have a relatively thin cell wall consisting of layers of peptidoglycan surrounded by a second outer membrane containing lipopolysaccharides (LPS) and lipoproteins. In contrast, Candida albicans (a fungal yeast) possesses a complex cell wall mainly containing chitin, glucan, and glycoproteins. Several research groups have investigated the properties of the bacterial cell surface in relation to colloidal stability and adhesion. According to a theory proposed by Derjaguin, Landau, Verwey, and Overbeek, the stability of colloidal particles is influenced by overall hydrophobicity and surface charge (Karunakaran et al., 2011). For example, Wang et al. reported that a mutant strain of Cronobacter sakazakii with an defective outer membrane had enhanced biofilm-forming ability, due to its higher surface hydrophobicity resulting from a defect in its coating of LPS (Wang et al., 2012).
Intracellular Biomolecules
There are numerous biomolecules within microbial cell, carrying out countless biochemical reactions and metabolic processes that control the overall behavior of microbes. The microbial metabolism in the biofilm state differs markedly from that in the planktonic state, and these changes influence the physiology of pathogens and therefore can regulate biofilm development. For examples, by comparing nuclear magnetic resonance (NMR) metabolite profiling of methicillin-resistant S. aureus (MRSA) and methicillin-sensitive S. aureus (MSSA) in both biofilm and planktonic phenotypes, Ammons et al. found that the key differences included matrix deposition, and metabolism shifts, including selective amino acid uptake, lipid catabolism, butanediol fermentation, and a shift from metabolic energy production to assembly of cell wall components (Ammons et al., 2014). Alen et al. also discovered that the altered intracellular activity of Neisseria meningitidis in a biofilm environment contributed to enhanced survival, persistence and adaption to biofilm growth (van Alen et al., 2010). Furthermore, there also exist indirect effects on biofilm. For example, a change in the proteome can influence the structure and content of macromolecules expressed on the cell surface, and thence the EPS secreted into the matrix (Karunakaran et al., 2011), thereby affecting biofilm formation and cell aggregation.
Biofilm-Specific Antibiotic Resistance
The viscous matrix of biofilm can slow down diffusion of drugs and even act as a complete barrier against drug penetration (Singh et al., 2016). Moreover, for mixed-species biofilms, the various polymer can interact with each other, thereby further the increasing the viscosity and lowering drug penetration (Skillman et al., 1998). Moreover, microbial cells embedded in the deeper layers of the biofilm, change their metabolism to a state of near-dormancy, and became even less sensitive to antibiotics (Høiby et al., 2010). Furthermore, the high cell density within biofilms, encourages horizontal gene transfer and gene mutation, due to the physical proximity of the microbial cells and availability of extracellular DNA in the EPS (Molin and Tolker-Nielsen, 2003; Khemiri et al., 2016). The increase in genetic diversity within biofilms, facilitates adaption to antibiotics, and various drug-resistant variants have been reported to arise due to chromosomal mutation, induction of expression of latent genes, and exchange of genetic material between cells (Neu, 1992). For the reasons mentioned above, bacteria in biofilms are less susceptible to antimicrobial drugs, and bactericidal treatments (even aPDT) in comparison to their planktonic counterparts (Vickery et al., 2012).
Furthermore, some multi-species biofilms display lower susceptibility than mono-species biofilms. In a mixed-species biofilm of S. mutans and Candida, the hyphal formation of Candida was depressed due to its co-existence with S. mutans (Pereira-Cenci et al., 2008). Since it was easier for aPDT to kill hyphal forms of Candida compared to yeast forms (Chabrier-Roselló et al., 2008), the mixed-species biofilm displayed less susceptibility (Pereira-Cenci et al., 2008).
Biofilm and Quorum Sensing (QS)
Biofilm formation of bacteria is a collective behavior involving bacterial populations embedded in a self-produced extracellular matrix. Moreover, the proximity of cells embedded in biofilms facilitates cell-to-cell communication. Thus, biofilm development are closely associated with QS, a cell–cell communication mechanism that senses population and orchestrate gene expression. QS describes the regulation of gene expression in response to fluctuations in population density, by which single-celled microorganisms (particularly bacteria) coordinate gene expression to suit their local population density and availability of nutrients. QS bacteria synthesize and release signaling molecules called “autoinducers,” that naturally increase in concentration as a function of cell density, until a threshold concentration of autoinducer is reached resulting in alteration in expression of related genes (Miller and Bassler, 2001). There are four different types of autoinducers reported to date, including N-acyl-l-homoserine lactone (AHL) in G− bacteria, the autoinducing peptide in G+ bacteria, the autoinducer-2 in both G− and G+ bacteria, and farnesol in C. albicans and other fungi. AHL in G− bacteria has been shown to coordinate expression of genes responsible for diverse biological functions (Hentzer and Givskov, 2003; Linares et al., 2006). Compared to planktonic cells, bacterial cells within biofilms are much better protected from the attack by noxious agents. Consequently it is very difficult to control biofilm infections by antibiotics. The components within biofilm, as well as autoinducers, are targets of biofilm-controlling strategies including aPDT.
Clinically Relevant Biofilms
Biofilms contribute to the majority of bacterial and fungal infections occurring in different anatomical regions of the body, such as urinary tract infections, catheter infections, middle-ear infections, gingivitis, caries, periodontitis, orthopedic implants, and many others (de Melo et al., 2013).
Biofilm Infections in Different Regions of the Body
Oral Biofilms and Dental Plaque
Bacterial biofilms on the tooth surface, also known as dental plaque, play a key role in the pathogenesis of periodontal diseases, endodontic infections, caries, and numerous other conditions extending beyond the oral cavity (Howlin et al., 2015). Dental pathogens including Streptoccus mutans (Takahashi and Nyvad, 2011), non-mutans acidogenic and aciduric bacteria (van Houte et al., 1996), such as streptococci, Actinomyces spp., lactobacilli, and Bifidobacterium spp. (van Ruyven et al., 2000). Among therapies designed to remove dental plaque, mechanical disruption and antimicrobial agents have been frequently employed, but the effectiveness is limited by amount of effort required and presence of resistant pathogens (Konopka and Goslinski, 2007). A high power laser showed some effectiveness, but caused thermal injuries to dental tissues (Nunes et al., 2011). In contrast, aPDT can remove oral biofilms on the teeth with negligible side-effects (Garcez et al., 2010; Rios et al., 2011; Vaziri et al., 2012; Yildirim et al., 2013; Muhammad et al., 2014; Zand et al., 2014), and thus in some clinical studies, aPDT has been used as an adjunctive treatment procedure to the conventional methods (Prasanth et al., 2014).
Chronic Wound Infections
Chronic wound infections lead to considerable morbidity and even amputation, and contribute to escalation in health care costs. Wound infections may progress from initial bacterial colonization followed by biofilm formation, which protects the bacteria from host defenses, while at the same time increasing resistance to conventional antibiotics (Edwards and Harding, 2004; Leaper et al., 2015). Staphylococcus aureus and coagulase-negative staphylococci are the most commonly isolated bacteria, and other common organisms include Enterobacter cloacae, Enterococcus faecalis, Pseudomonas aeruginosa, Peptococcus magnus, and anaerobic bacteria (Siddiqui and Bernstein, 2010). Most chronic wound pathogens are typical biofilm producers.
Currently, one of the most successful strategies for the management of biofilm-related conditions is physical removal of biofilm. Additionally, aPDT has been reported to be effective in eradicating wound infections caused by various pathogens (Wong et al., 2005; Zolfaghari et al., 2009; Lin et al., 2010).
Chronic Rhinosinusitis (CRS)
Chronic rhinosinusitis (CRS) is one of the most common chronic conditions in the U.S., and a significant subpopulation of CRS patients is difficult to cure (Biel et al., 2013). MRSA and multidrug resistant P. aeruginosa have been isolated frequently from CRS patients (Wood et al., 2011; Li et al., 2012). Bacterial colonization and biofilm formation in the sinus cavity is implicated in the resistance mechanism. Recently, Biel et al reported that aPDT could effectively treat polymicrobial biofilms in a maxillary sinus cavity model (Biel et al., 2013).
Prosthetic Joint Infections (PJI)
As a major complication of total joint arthroplasty, prosthetic joint infections (PJI) are becoming a significant health concern. Although occurring at only a relatively low rate (ranging from 1 to 2%), PJI can lead to increased costs in health care (Sia et al., 2005). Bacterial biofilm that forms on prosthetic orthopedic implants is a common cause for PJI (Zimmerli et al., 2004). Highly virulent microorganisms such as S. aureus and G− bacilli (such as E. coli) can participate in early infection, while coagulase-negative staphylococci and Propionibacterium acnes are responsible for some delayed infections, and S. aureus, streptococci, and G− bacilli can contribute to late infections (Gbejuade et al., 2015). Recently, aPDT was reported as an efficient means of treating bacteria causing PJI (Briggs et al., 2018).
Ventilator Associated Pneumonia (VAP)
Ventilator associated pneumonia (VAP) is one of the most frequent hospital-acquired infections occurring in intubated patients, and can result in a mortality rate approaching 50% VAP is caused by a microbial biofilm forming on the inner surface of endotracheal tubes (ETT) (Azoulay et al., 2006). The most common species isolated from tracheal secretions and ETT-associated biofilm including S. aureus, Enterococci, Enterobacteriaceae, P. aeruginosa, Acinetobacter baumannii, and Candida species (Adair et al., 1999; Gil-Perotin et al., 2012). MB-aPDT can effectively eradicate polymicrobial biofilms in the ETT tube, which had long been recognized as a critical factor leading to VAP (Biel et al., 2011b).
APDT and Antimicrobial Photodynamic Inactivation
aPDT is a two-step technique employing a photosensitizer (PS) that is first administered systemically or topically to a confined area, followed by illumination with a specific wavelength of light that can excite the PS to cause production of cytotoxic ROS in the presence of ambient molecular oxygen. The burst of ROS produced during illumination can exert lethal effects on both cancer cells and/or microbial pathogens. aPDT has dual selectivity; the PS can be designed to be specific for cancers or infections, and the light can be spatially confined to the lesion area. The detailed features, types of PS and application in in vitro microbial cells are described below.
PS Structures and Mechanisms
aPDT employs specific-wavelength visible light to excite the PS. As shown in Figure 1, upon irradiation, the PS in its lowest energy level (ground singlet state, 1PS) is changed to the short-lived excited singlet state (1PS*), which can be converted to the long-lived excited triplet state, 3PS*. In presence of ambient oxygen, the triplet PS can undergo two types of chemical reactions: the type I mechanism is an transfer electrons to form toxic reactive oxygen species (such as superoxide, H2O2, and hydroxyl radicals, etc.) and the type II mechanism involves an energy transfer ground state triplet oxygen to produce highly reactive singlet oxygen (1O2). The two reactions can take place simultaneously.
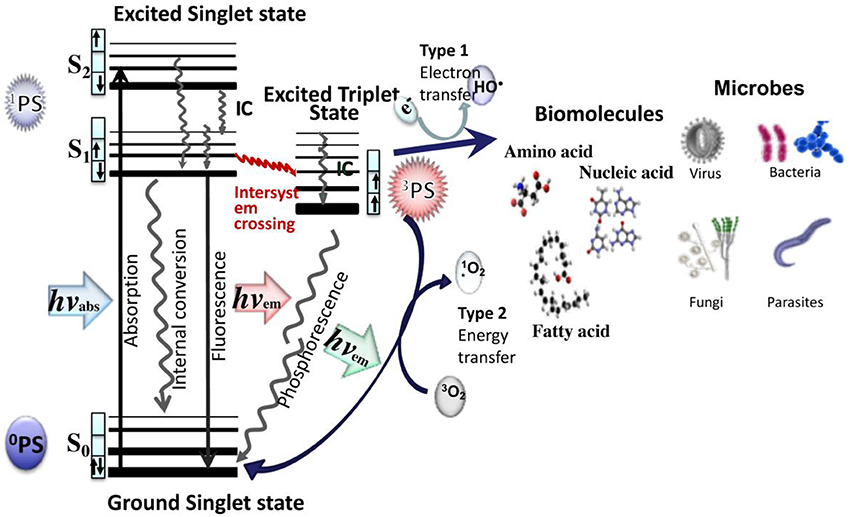
Figure 1. Jablonski diagram for ROS generation by aPDT via type I and type II photodynamic mechanisms. Both types of ROS can damage biomolecules and destroy or kill all known classes of pathogenic microorganisms.
PSs are usually highly conjugated unsaturated organic molecules with a large absorption coefficient in the visible spectrum and preferably in the long wavelength red near infrared region to ensure good tissue penetration of the light. However, not all dyes can undergo the intersystem crossing to produce the triplet state necessary for photochemical reactions to occur. Among PS structures commonly employed in aPDT (Table 1), acridine orange was the first used, and tetrapyrrole macrocycles such as porphyrins, chlorins, bacteriochlorins, and synthetic phthalocyanines can be highlighted. A number of non-tetrapyrrole dyes and natural compounds had also been used, such as rose bengal (RB), toluidine blue O (TBO), methylene blue (MB). As summarized by Abrahamse and Hamblin recently (Abrahamse and Hamblin, 2016), several PS such as HpD (haematoporphyrin derivative), Photofrin, PPIX (Protoporphyrin IX), Verteporfin (benzoporphyrin derivative), Radachlorin (now Bremachlorin), Fullerenes, Temoporfin, or Foscan (mtetrahydroxyphenylchlorin) had received clinical approval.
Furthermore in recent years, nanotechnology has been employed to improve PS performance (Chen et al., 2012; Khan et al., 2012). Figure 2 shows the targets of PS-generated ROS within microbial biofilms.
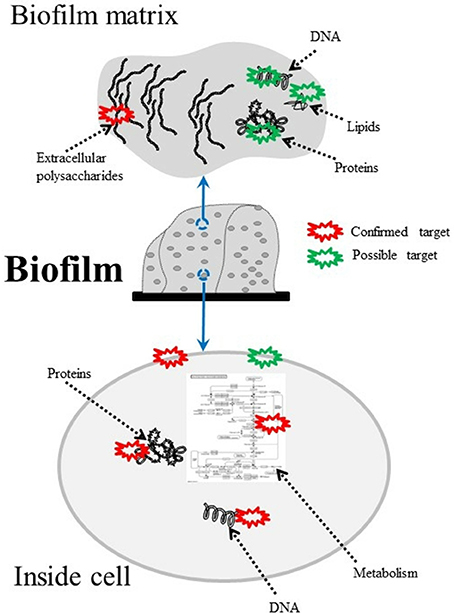
Figure 2. Composition of bacterial biofilms and possible aPDT targets. DNA, lipids (Alves et al., 2013a,b; Lopes D. et al., 2014), proteins (Konopka and Goslinski, 2007; Gracanin et al., 2009; Dosselli et al., 2012), DNA (Rabea et al., 2003; Lam et al., 2011), and polysaccharides (Beirão et al., 2014) can all be damaged by aPDT-generated ROS.
Here the PS molecules are chosen bind to the microbial cell surface, most often to the cytoplasmic membrane, so aPDI can take place. Thus, the attachment efficiency of PS has a great influence on the efficiency of aPDI. Considering the architectural differences in the cell surface structure, different microbial cells show different susceptibilities to aPDI depending on the PS chemical structure (George et al., 2009). In general, G+ bacteria and yeasts are easily affected by neutral and anionic PS, while G− bacteria were not, because these species possess an additional asymmetric outer membrane containing numerous strongly negatively charged molecules such as LPS (Figure 2). This outer membrane reduces the permeability and weakens the attachment of neutral PS or repels anionic PS. To overcome the disadvantage, several strategies have been developed, such as synthesizing positively charged PS, coupling PS to other positively charged entities such as polyethylenimine, or pre-treating bacterial cells with Tris-EDTA or polymyxin B non-apetide (Nitzan et al., 1992).
Susceptibility of Biofilm Forming Pathogens to APDI
Up to now, a variety of pathogens have been isolated from different biofilm infections. As shown in Supplementary Table 1, aPDT has a broad-spectrum of activity against biofilm microrganisms including both naive and drug-resistant pathogens isolated from oral cavity, urinary tract infections, endocarditis, and biomaterial implant infections. These susceptible bacterial and fungal species cover the most frequently isolated pathogens, including G− bacteria (P. aeruginosa, E. coli, Fusobacterium nucleatum, Moraxella catarrhalis, Aggregatibacter actinomycetemcomitans), G+ bacteria (Enterococcus faecalis, MRSA, MSSA, Streptococcus spp., Actinomyces naeslundii), and yeast (C. albicans).
Investigative Control Strategies for Biofilms
Before we look in detail at the ability of aPDT/aPDI to combat biofilms, we will present a brief survey of other innovative strategies that have been tested to eradicate biofilms.
Inhibition of QS
QS coordinate the behavior depending on cell density, and thus interfering with QS signaling can decrease biofilm stability. Down-regulation of autoinducers or interference with their receptors is an approach that has been studied by several researchers (Davies et al., 1998; Geske et al., 2005; Ishida et al., 2007; Amara et al., 2009; O'Loughlin et al., 2013). The chemical reactivity of AHL was tuned by modifying the lactone head (Smith et al., 2003; Glansdorp et al., 2004; Ishida et al., 2007), and metabromo-thiolactone depressed biofilm formation by inhibiting QS receptor RhlR. Autoinducers operate via intracellular signal transduction by secondary messengers, such as cyclic diguanylate c-di-GMP. Thus, there existed a new approach to combat biofilms by modulating c-di-GMP signaling pathway (Römling et al., 2013). Ebselen could inhibit binding of c-di-GMP to allosteric sites, thereby inhibiting P. aeruginosa biofilm formation (Lieberman et al., 2014).
Some plant-derived natural products can interfere with QS, that might explain their use to treat microbial infections, such as Quercus infectoria Olivier (Chusri et al., 2012), Curcuma longa L. (Packiavathy et al., 2014), Allium sativum L. (Bjarnsholt et al., 2005), essential oils from Cinnamomum verum Pres (Niu et al., 2006), and Syzygium aromaticum (L.) Merrill & Perry (Khan et al., 2009), and methanol extract of Kalanchoe blossfeldiana (Sarkar et al., 2015). Other compounds targeting QS such as synthetic furanones (Wu et al., 2004), the fatty acid messenger cis-2-decenoic acid (Davies and Marques, 2009), and QS inhibitor “yd 47” have all displayed some efficacy against biofilms (Cevizci et al., 2015). Some proteins and peptides can also suppress biofilm by interfering with QS, such as RNA-III-inhibiting peptide (RIP) (Balaban et al., 2007), a competence-stimulating peptide (LoVetri and Madhyastha, 2010), and an analog of competence stimulating peptide (KBI-3221). Some combinations of QS inhibitors have exhibited synergistic efficacy, such as FS3 and daptomycin in treatment of staphylococcal biofilm (Cirioni et al., 2013).
Enzymatic Degradation of Extracellular Polymeric Substances
These EPS-destructing enzymes include dispersin B (Kaplan et al., 2004; Itoh et al., 2005; Izano et al., 2008), an alginate lyase that can decompose polysaccharide (Alkawash et al., 2006; Alipour et al., 2009; Lamppa and Griswold, 2013), nucleases that can degrade extracellular DNA (also an important component of the biofilm matrix) (Okshevsky et al., 2015), and proteases such as proteinase K (Nicholson et al., 2013).
Improvement of Cleaning and Disinfection of Surfaces
The traditional cleaning and disinfection of surfaces could be improved by employing cold atmospheric plasma and reactive discharge gases (Traba and Liang, 2011; Pei et al., 2012); photocatalysis with TiO2 nanoparticles (Cieplik et al., 2013); nanoparticles of silver and gold (Adetunji et al., 2014); dense phase carbon dioxide (Mun et al., 2009); electrolyzed oxidizing water (Kim et al., 2001); antimicrobial mouth-rinse (Pedrazzi et al., 2014); vegetable oil (Brazil nut oil) and mineral oil (liquid petrolatum) (Filogoniô Cde et al., 2011); sanitizer treatments (Adetunji et al., 2014); a diode laser (de Oliveira Guaré et al., 2010); antibiofilm agent (El-Feky et al., 2009); UV-based oxidation process (Lakretz et al., 2011); and electrochemical biofilm control (Istanbullu et al., 2012).
Other Approaches
Bacteriophage therapy is effective in combating wound biofilm infections and implant- and catheter-related infections (Burrowes et al., 2011; Alemayehu et al., 2012; Seth et al., 2013; Soothill, 2013; Yilmaz et al., 2013; Chan and Abedon, 2015). Through blocking biogenesis of curli and type 1 pili (bacterial amyloids), two analogs of FN075 and BibC6 of ring-fused 2-pyridones were successfully applied to decrease biofilm formation (Cegelski et al., 2009). Some other antibiofilm compounds have been reported such as natural products secreted by marine sponges (Stowe et al., 2011), compounds based on the 2-aminobenzimidazole structure found in bromoageliferin and oroidin (Wright et al., 2014), and diterpenoid derivatives such as (-)-ageloxime-D from Agelas (Stowe et al., 2011). Other antibiofilm methods such as electrical treatments and ultrasound treatments have also been tested.
The antibiofilm strategies mentioned above aimed at specific and limited targets. In comparison, aPDT had multiple targets (described in section Underlying Anti-biofilm Mechanisms of aPDT), since the PS-generated ROS could non-specifically attack various molecules such as proteins, EPS, DNA and lipids. Therefore, it appeared to be promising and potentially applicable in very diverse contexts where PS and light can be easily delivered.
The Effect of APDT on Biofilms in vitro and the Underlying Multiple Mechanisms
To date, there is a growing body of literature indicating that aPDI represents an attractive approach to eradicate biofilms especially for treatment of recurrent and chronic biofilm infections. aPDI has multiple targets, and can not only efficiently kill the microbial cells themselves, but at the same time the ROS can weaken and degrade the matrix structure and the EPS by attacking numerous biomolecules. Therefore, aPDI appears to be promising and potentially applicable in many diverse contexts where PS and light can be easily delivered (Orlandi et al., 2015).
G− Bacterial Biofilms
As shown in Supplementary Table 1, most work has focused on P. aeruginosa and A. actinomycetemcomitans, and the highest bacterial killing efficiency was achieved with rates of about 7 log10 (Orlandi et al., 2015) and 6 log10 (Prasanth et al., 2014). aPDI using Tetra-Py+-Me was proposed to target polysaccharides in the biofilm matrix of P. aeruginosa first, and then inactivating the bacteria (Beirão et al., 2014). A more detailed mechanism of action on P. aeruginosa was proposed for RLP068/Cl (Vassena et al., 2014). This firstly targeted the cell wall and cell membrane by hydrophobic and electro-static interactions, then disrupted the external components on the cell surface through various oxidative reactions upon irradiation, and finally entered the cell by a self-promoted uptake process (Hamblin et al., 2002). Other susceptible G− bacteria include Fusobacterium nucleatum, E. coli, and Moraxella catarrhalis clinical isolates. Especially, M. catarrhalis showed prominent morphological changes with visibly distorted cell membranes visualized by means of scanning electron microscopic (SEM) (Luke-Marshall et al., 2014).
G+ Bacterial Biofilms
The most frequently employed G+ species have been S. aureus, E. faecalis, and Streptococcus, with highest killing efficiency reported to be 6.3 log10 (Beirão et al., 2014), ca 6 log10 (Prasanth et al., 2014), and ca 4.5 log10 (Chen et al., 2012), respectively. Especially, aPDT based on chitosan nanoparticles functionalized with rose-bengal (CSRBnp) decreased the viable bacteria of E. faecalis, and reduced biofilm thickness from 39.2 to 13.1 μm, through a mechanism where CSRBnp was propsed to adhere to the cell surface, permeabilize the cell membrane, and finaly lysed the cells (Shrestha et al., 2014). aPDT also showed antibiofilm activity for A. naeslundii and Enterococcus strains (Shrestha and Kishen, 2014).
Yeast Biofilms
Candida spp. can form biofilms on various implanted biomaterials such as pacemakers and intravascular catheters and thus can cause various clinical problems. Traditional treatment with antifungal agents is now facing challenges, and aPDI, either alone or in conjunction with other agents, has been proven to be effective. As shown in Supplementary Table 1, aPDT employing different PS (especially MB and TB) had been shown to control biofilms of C. albicans and Candida parapsilosis. 1 × 106 cells were inoculated in 96-wells polystyrene microtiter plate to form biofilm, and aPDT treatment completely inactivated them (Lopes M. et al., 2014). The multiple mechanisms include inhibition of cell metabolism (Ribeiro et al., 2013), cell growth (Ribeiro et al., 2013), damage to the cytoplasmic membrane (Ribeiro et al., 2013), and increased cell permeability (Rosseti et al., 2014). When used as PS, N-(5-(3-hydroxypropylamino)-10-methyl-9H-benzo[a]phenoxazin-9-ylidene)ethanaminium chloride (FSc) and N-(5-(11-hydroxyundecylamino)-10-methyl-9H-benzo[a]phenoxazin-9-ylidene)ethanaminium chloride were absorbed by the biofilm matrix and cells. ROS were generated at these sites destroying the biofilm via oxidization of the matrix polysaccharide and damage to the cell wall (Lopes M. et al., 2014).
Mixed-Species Biofilms
Almost all microbial biofilms occurring in nature are composed of various different species of microorganisms. Inter-species interactions, which can be synergistic interactions (metabolic cooperation) or antagonistic interactions (competition for nutritional resources), make mixed-species biofilms more complicated in architecture, and structure than mono-species biofilms. As shown in Supplementary Table 1, aPDT also showed some antibiofilm effects on mixed-species biofilms. When S. mutans ATCC25175 and Lactobacillus acidophilus ATCC ITAL-523 were cultivated together, the biofilm formed in 96-well plates could be completely removed by aPDT using a stock solution of curcumin and curcuminoids as the PS (Araújo et al., 2014). The underlying killing mechanism was similar to that for mono-species biofilms, including PS adhering to the cell surface, permeabilizing the cell membrane and finally lysing the cells (Cieplik et al., 2013). When the PS was functionalized by attaching it to bioactive nanoparticles, it increased the affinity to the cell membrane and allowed deeper penetration into the biofilm structure (Shrestha and Kishen, 2014).
Underlying Anti-Biofilm Mechanisms of aPDT
Based on the previous publications as mentioned below, it can be concluded that the anti-biofilm process of aPDT includes two steps. Firstly the PS initially binds to the biofilm matrix. In a few cases, the PS was totally sequestrated by EPS, while in other cases, the PS partially passed through EPS and penetrated further to contact the microbial cells. Some types of PS only bind to the cell surface, while other types of PS can pass through the cytoplasmic membrane and reach into the cellular cytoplasm and even enter organelles such as the nucleus in fungal cells. Wherever the PS was localized, ROS are generated upon illumination to initiate the second step, namely multitarget oxidative damage. Production of large quantities of ROS can overwhelm the antioxidant defenses of microbial cells. As soon as ROS is generated, it attacks a variety of adjacent molecules, including targets within the biofilm matrix (e.g., polysaccharides), on the cell surface (e.g., lipids), and inside the cells (e.g., proteins and DNA), resulting in the collapse of the biofilm matrix and disintegration of microbial cells. The generation of ROS has been extensively reviewed elsewhere (Melo et al., 2013), thus a detailed description is provided here with a focus on chemical reactions during the 2nd step.
Generally speaking, the burst of ROS (•OH and 1O2 are the two most commonly studied and damaging species) leads to oxidative damage of multiple non-specific targets, such as amino acids, nucleic acid bases, lipids, etc. The exact targets depend on the PS localization and abundance, since most ROS cannot travel very far before disappearing (Vatansever et al., 2013). Consequently, damage to proteins, DNA and membranes occurs, and cell death is induced. Furthermore, aPDT does not affect surrounding host cells or organs without PS. Based on the localization of PS in the biofilm, there exist three types of oxidative damage induced by aPDT as follows.
Biofilm Matrix Destruction
EPS-containing matrix (containing polysaccharides, proteins, hexosamine, uronic acid, and DNA) is the first line of defense against most environmental stress including aPDT. Moreover EPS matrix limits inward diffusion of the PS. For example, a slime-producing strain of S. aureus hampered PS uptake and showed lower sensitivity to aPDT than strains without slime (Gad et al., 2004). On the other hand, various components of the matrix can be attacked by ROS (Beirão et al., 2014). SEM imaging of P. aeruginosa or E. faecalis biofilms revealed damage to the architecture after MB-based aPDT (Garcez et al., 2013).
The most abundant constituent in matrix is polysaccharide, and its photodamage has attracted most attention (Garcez et al., 2013; Beirão et al., 2014). In 2014, Beirao et al. investigated photodynamic damage of polysaccharides within P. aeruginosa biofilms. PS Tetra-Py+-Me alone caused a 33% reduction of EPS content, while light alone did not. Because PS-matrix interactions in the absence of light likely affect the cohesiveness and stability of the EPS, and thereby can lead to polysaccharide detachment from the matrix (Beirão et al., 2014). However, when aPDT was conducted (after light delivery), the polysaccharide level was further reduced up to 80% (Beirão et al., 2014), which was crucial for the 2.8 log10 reduction in bacterial counts.
Besides polysaccharide, other constituents of the biofilm matrix such as proteins, lipids, DNA, and other components can easily be oxidized by ROS. It has been reported that macromolecules such as proteins and DNA in the microbial cytoplasm and lipids in the outer membrane could be irreversibly damaged by ROS (Konopka and Goslinski, 2007). Conjugation of PS to natural macromolecules such as chitosan also influenced EPS and contributed to the anti-biofilm effect (Shrestha and Kishen, 2012). The chitosan can intercalate with extracellular DNA and disrupt the biofilm structure (Upadya and Kishen, 2010).
Damage to the Cell Surface
Up to now, little is known about the actual location of various PS in microbial cells and biofilms. Some PSs are tightly bound to the cell surface and can penetrate into the cells, such as poly-L-lysine chlorin(e6) conjugate (pL-ce6) (Merchat et al., 1996; Soukos et al., 1998), while other PSs may be only loosely bound and some not bound at all. Whether they are attached to cell surface or not, photoactivated PS result in damage to the cell membrane in most reports, via ROS oxidation (Shrestha et al., 2010; Luke-Marshall et al., 2014; Shrestha and Kishen, 2014).
Damage to the cell membrane has been observed by SEM after Photofrin-based aPDT treatment of a clinical M. catarrhalis biofilm (Luke-Marshall et al., 2014), and details of membrane damage were revealed after aPDT inactivation of MRSA biofilms using Ru(II) complexes as PS (Wood et al., 2006). Membrane damage causes morphological changes, such as appearance of bubble-like structures induced by osmotic fragility after cell wall damage (Wang et al., 2015). Besides, transmission electron microscope (TEM) clearly showed a discontinuous, detached and wrinkled cell profile, due to damage to the cytoplasmic membrane, which was similar to the shrunken cell envelope of E. coli seen after aPDT (Caminos et al., 2008). These changes always occurred at the polar regions of cells, which was attributed to their cardiolipin-rich domains (Mileykovskaya and Dowhan, 2000) and also to anionic phospholipids mostly located at polar regions (Oliver et al., 2014), where more intensive negative charge allowed a stronger affinity to the cationic Ru(II) complexes (Wang et al., 2015). Generally speaking, aPDT can irreversibly oxidize vital components of the cells as follows.
Oxidation of Lipids
As soon as they are generated, ROS attack a variety of biomolecules, such as oxidizing unsaturated lipids, destructing DNA (particularly guanine bases) and inactivating proteins by attacking sulfur containing and aromatic amino acids. Recent studies highlighted lipid peroxidation upon photodynamic treatment of planktonic bacteria. Phosphatidylglycerol (PG) and cardiolipin (CL) are two major phospholipid components of Staphylococcus warneri. After aPDT treatment, the relative abundance of PG was increased while new oxidized species from CL such as hydroxyl and hydroperoxy derivatives appeared immediately (Alves et al., 2013a). The oxidation of lipids was also analyzed in E. coli (Alves et al., 2013b) by using a lipidomic approach. For E. coli ATCC 25922, aPDT induced appearance of lipid hydroperoxides, hydroxy and hydroperoxy derivatives, along with a decrease in unsaturated C16:1 and C18:1 species (Alves et al., 2013b). Melo et al investigated the oxidation of PE standards upon white light and cationic porphyrins, and identified photooxidation products of POPE (1-palmitoyl-2-oleoyl-sn-glycero-3-phosphoethanolamine, 16:0/18:1), PLPE (1-palmitoyl-2-linoleoyl-sn-glycero- 3-phosphoethanolamine, 16:0/18:2), and PAPE (1-palmitoyl-2-arachidonoyl-sn-glycero-3-phosphatidylcholine, 16:0/20:4) as hydroxy-, hydroperoxy- and keto- derivatives (Melo et al., 2013). Using 5 porphyrin derivatives with different molecular charges and analyzing lipid extracts from E. coli, it was revealed that content of lipid hydroperoxides depended on the PS charge and distribution (Lopes D. et al., 2014).
Change in Permeability of the Outer Membrane
The primary consequence of damaging the outer membrane is a change in membrane permeability, responsible for death of the microbial cells, such as reported for TBO-mediated aPDT killing of C. albicans biofilms (Rosseti et al., 2014). Therefore, when some permeability-enhancing agents (such as chitosan and saponins) were combined with PS to mediate aPDT, the anti-biofilm efficiency could be further enhanced.
Coleman et al screened 12 saponins (biological detergents) and applied them together with PS to control C. albicans biofilms. The fungal susceptibility to aPDT was increased when combined with saponins, due to the formation of pores in the cell membrane that facilitated PS uptake (Coleman et al., 2010b). This observation also suggests that saponins could be an ideal supplement for conventional antifungal therapy (Coleman et al., 2010b). Some bioactive natural polymers such as chitosan can also permeabilize the cell surface of bacteria, due to the electrostatic interaction between groups of chitosan acetate and phosphoryl groups of the phospholipids embedded in the cell membrane (Liu et al., 2004). The conjugation of PS with chitosan helped to enable more targeted action, increase PS uptake and improve antibiofilm efficacy (Rabea et al., 2003; Liu et al., 2004). By employing chitosan conjugated RB (CSRB), a higher killing effect on E. faecalis and P. aeruginosa biofilms compared to RB alone was obtained (Shrestha and Kishen, 2012).
The dramatic increase in permeability of outer membrane can improve PS uptake and stimulate metabolite leakage. Atomic force microscope (AFM) showed that RB-functionalized chitosan nanoparticles (CSRBnps) adhered to the cell surface of E. faecalis, and thereby resulted in pitting and disruption of cell surface (Shrestha and Kishen, 2014), which facilitated further penetration of CSRBnps (Shrestha and Kishen, 2014).
Another consequence of damage to the cell surface is interference with membrane function after a short aPDT treatment, such as reported for aPDT inactivation of E. coli biofilms (Caminos et al., 2008). This interference included inactivation of membrane transport systems (Hamblin and Hasan, 2004; Tavares et al., 2010), and other unknown mechanisms. In the latter case, damage to the cell surface of Streptococcus mutans was not serious; the bacteria did not die but could not replicate and grow, contributing to removal of S. mutans from dental plaque (Manoil et al., 2014).
Penetration and the Self-Promoted Uptake Pathway
In aPDT mediated by cationic PS such as the phthalocyanine RLP068/Cl, oxidative damage to the cell wall was followed by the self-promoted uptake pathway (Hamblin et al., 2002).
The self-promoted uptake pathway has been widely observed in polycationic antimicrobial agents in bacteria (Hancock and Bell, 1988). The initial step seems to occur at negatively-charged sites such as phosphate and carboxyl groups on lipopolysaccharide (LPS) molecules, which are the main lipid on the surface of G− bacteria (Figure 2) and contribute to the relative impermeability of the outer membrane to neutral or anionic PSs (Nitzan et al., 1995). The non-covalent association between LPS and divalent cations (Ca2+ and Mg2+) is essential for the integrity for the G− outer membrane (Leive, 1974), and cationic PS can weaken the attachment of adjacent LPS molecules by displacing these divalent cations (Minnock et al., 2000). Through the self-promoted uptake pathway, positively charged PS such as the cationic pyridinium zinc phthalocyanine (PPC) (Minnock et al., 2000) and the polycationic poly-L-lysine PS conjugate, pL-ce6 (Soukos et al., 1998) can gain access across the outer membrane of E. coli. pL-ce6 distorted the structure of outer membrane through the formation of channels (Soukos et al., 1998).
Intracellular Damage
Some PS can pass through the cytoplasmic membrane, while other PS cannot immediately, but can arrive in the cytoplasm after irradiation. The intracellular PS can cause damage to countless biomolecules within the cytoplasm such as DNA, proteins, and lipids, which work together to interfere with vital microbial metabolic pathways and cell functions. Besides, for fungal cells, some organelles such as the nucleus and mitochondria can be damaged (Lam et al., 2011).
DNA damage is one crucial mechanism driving aPDI. aPDT leads to breaks in single-stranded and double-stranded DNA, and the disappearance of the super-coiled fraction of plasmid DNA in both G+ and G− species (Bertoloni et al., 2000). A recent study revealed DNA cleavage at guanine residues caused by photoactivated Nile blue (NB) via electrostatic interaction with nucleic acids, which is thought to be an important mechanism in low oxygen environments (Hirakawa et al., 2014). On the other hand, with some less powerful PS, subtle non-lethal damage to DNA could be repaired by various DNA repair systems. The gene uvrA of H. pylori was implicated in the repair of DNA photodamage (Goosen and Moolenaar, 2008). However, for PS with powerful photodynamic effects, the burst of ROS induced significant DNA damage, which was impossible to be repaired. It was notable that Pc 4-mediated aPDT led to changes in nuclear morphology of C. albicans, which showed characteristics of apoptosis (Lam et al., 2011).
Proteins are another target, which are easily damaged, owing to their high abundance and rapid reaction rate with ROS. ROS can react with various amino acid residues in proteins resulting in loss of histidine residues, radical-induced cross links producing dityrosine moieties, introduction of carbonyl groups, formation of protein-centered alkyl, alkoxyl, alkylperoxyl, and ROO• radicals, and cleavage of peptide bonds (Gracanin et al., 2009). Especially, the sulfur-containing amino acids, cysteine, and methionine are particularly susceptible. The membrane proteomics of S. aureus after sub-lethal aPDT treatment using meso-tetra-4-N-methyl pyridyl-porphine revealed that expression of functional proteins involved in metabolic activities, such as oxidative stress defense, cell division, and sugar uptake were selectively affected (Dosselli et al., 2012).
It should be noted that due to the damage caused to protein and DNA, the cellular metabolism in biofilms can be significantly reduced by aPDT (Figure 3). de Aguiar Coletti et al. employed the 2,3-bis-(2-methoxy-4-nitro-5-sulfophenyl)-2H-tetrazolium-5-carboxanilide (XTT) reduction method to assess biofilm metabolic activity, because the biofilm need not be disrupted, and the intensity of the orange color of the formazan product is proportional to the metabolic activity. The results showed that aPDT led to decreases of 58 and 30% in cellular metabolism for E. coli and S. aureus biofilms respectively.
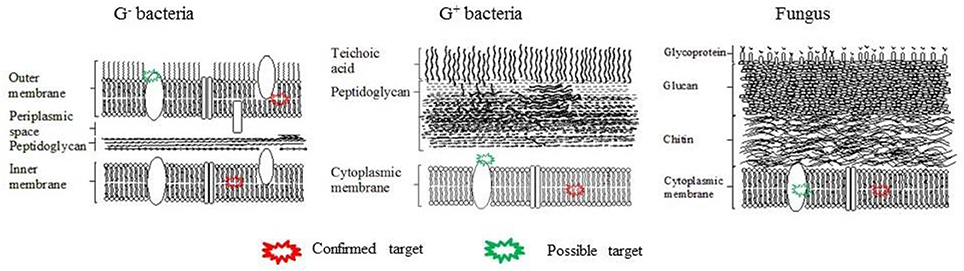
Figure 3. Cell wall structures of G− bacteria, G+ bacteria and fungus and possible aPDT targets. The plasma membrane (Alves et al., 2013a,b; Melo et al., 2013; Lopes D. et al., 2014) and transmembrane proteins (Konopka and Goslinski, 2007). can be destroyed by aPDT-generated ROS.
In addition to PS, chitosan can also interact with DNA and inhibit mRNA and protein synthesis (Rabea et al., 2003), partly contributing to the higher antibiofilm effect with chitosan-based RB (Shrestha and Kishen, 2012).
Because of the synergistic effect of cell surface and intracellular photo-damage, some phenotypic changes can be induced under sub-lethal aPDT treatment, such as reduced growth and lower glucose consumption in S. aureus (Dosselli et al., 2012), and inhibition of hyphae and blastoconidia formation of fungal species (Seneviratne et al., 2008). C. albicans biofilm is a complex mixture of blastoconidia, pseudohyphae and hyphae, and the co-existence of multiple cell forms indicates a mature biofilm (Seneviratne et al., 2008). Costa et al reported that erythrosine- and RB-mediated aPDT coupled with LED irradiation destroyed C. albicans biofilms by reducing blastoconidia and hyphae (Costa et al., 2012b). Another interesting finding was that 4-hydroxynonenal, a derivative of linoleic acid oxidation (Schneider et al., 2008), may serve as a cell stress-signaling factor that triggers stress responses (Dwivedi et al., 2007; Catala, 2009).
Preclinical In Vivo Animal Studies Using APDT Against Biofilms
Before applying aPDT to clinical trials in humans, many in vivo studies have been carried out using animal models of various biofilm infections, including wounds, burns, middle-ear infections, endodontic infections, oral infections, osteomyelitis, nasal infections, Helicobacter pylori, leishmaniasis, tuberculosis, and different fungal infections (Table 1).
Wounds and Burns
Wounds and burns can be easily infected because the barrier function of the skin is disrupted. Burns are particularly susceptible to bacterial (especially multidrug resistant S. aureus) infections due to destruction of the cutaneous barrier (Cook, 1998).
For superficial wound infections where PS and light can be easily delivered, aPDT is thought to be particularly applicable to conquer bacterial biofilms. In a mouse model, Park et al assessed the aPDT effects on a mouse model of subcutaneous skin infection with bioluminescent S. aureus Xen29. After IV injection of chlorin e(6) and irradiation with a diode laser, lower bioluminescent signals were observed, suggesting growth inhibition. Furthermore, aPDT-treated mice had less neutrophilic infiltration and no massive bacterial colonies which the control group showed (Park et al., 2012). Nafee et al investigated aPDT using hypericin-loaded nanoparticles (HY-NP) in rats. aPDT treatment led to faster wound healing, better epithelialization, keratinization, and development of collagen fibers. The infection disappeared after 10 d, and an 80% reduction in the wound diameter was obtained (Nafee et al., 2013). Morimoto et al. evaluated the photodynamic effects of 5-aminolevulinic acid (5-ALA, precursor of protoporphyrin IX) on MRSA infection in mice. After intraperitoneal administration of 5-ALA, protoporphyrin IX accumulated in MRSA on the ulcer surface and aPDT exerted an anti-infective effect. 5-ALA-based aPDT accelerated wound healing and decreased the bacterial density on the ulcer surface (Morimoto et al., 2014).
A set of interesting models of localized infections in mouse skin has been investigated by Hamblin's group. Recently, skin abrasions infected by MRSA could be cured with aPDT mediated by PEI-ce6 conjugate (Dai et al., 2010). aPDT led to more than 3 log10 of bacterial reduction immediately but did not inhibit wound healing (Dai et al., 2010).
Oral Infections
The oral cavity provides an optimal environment for biofilm formation (Zaura et al., 2009). Up to now, three types of PS, erythrosine (Costa et al., 2012a), MB (Cernáková et al., 2015), and Photogem (Mima et al., 2010) have been employed to suppress oral biofilms in animal models.
Topical application of MB and laser irradiation using a diffusing fiber reduced C. albicans by 2.74 log10 steps in an immunosuppressed murine model (Teichert et al., 2002). Recently, Cernáková et al. also reported that photoactivated MB could totally eradicate biofilms of C. parapsilosis formed on the tongue of BALB/c female mice (Cernáková et al., 2015).
Photogem also displayed antibiofilm effects for C. albicans that was grown on the tongue of mice. For blue LED light, the maximal killing efficiency was achieved at 1.41 log10 after treatment, while for red LED light, a 1.59 log10 reduction rate was achieved, without any harm to the tongue tissue (Mima et al., 2010). Using erythrosine, aPDT reduced C. albicans in the lesions by 0.73 log10 and decreased its adherence to buccal epithelial cells by 35% without damaging adjacent tissue (Costa et al., 2012a).
Osteomyelitis
Pathogenic bacterial and yeast infections in bone result in the formation of osteomyelitis. In a rat model, bioluminescent S. aureus biofilms were implanted into the rat tibial bone. ALA-aPDT was generally effective against not only S. aureus biofilms in bone, even against recurrent infection in some cases (Bisland and Burch, 2006), but also could destroy biofilms growing on implants in bone (Bisland et al., 2006).
Nasal Infection
The anterior nares are commonly infected by S. aureus, which could further spread to other anatomical regions after major surgery. Cale et al. evaluated the utility of aPDT for nasal MRSA decolonization using a custom nasal reservoir model. The MB-aPDT employed a 670 nm diode laser fiber and a disposable light diffusing tip (Street et al., 2009). aPDT eliminated sustained MRSA colonization on cultured human epithelial surfaces, and completely eradicated MRSA from the nose in humans with a treatment times less than 10 min (Street et al., 2009).
Superficial Fungal Skin Infection
Superficial fungal skin infections affect millions of people, where C. albicans and Trichophyton rubrum are the most frequently encountered fungal pathogens. Dai et al. employed a new MB-based model of aPDT in a mouse model of skin abrasion infected with C. albicans (Dai et al., 2011). aPDT initiated at 30 min or at 24 h post infection could reduce 95.4 and 97.4% cells of C. albicans in the skin abrasion wounds respectively.
Baltazar et al investigated the antifungal effect of TBO-aPDT in a mouse model of cutaneous dermatophytosis caused by T. rubrum. Topical application of a TBO gel was followed by red light irradiation given each day for 7 days. The fungal burden was reduced by 87%, and skin architecture was improved (Baltazar et al., 2015).
Tuberculosis
Tuberculosis is caused by Mycobacterium tuberculosis infection, and the death rate in drug-resistant infections is among the highest for infectious diseases worldwide. aPDT had been applied to treat Mycobacterium infection in a mouse model, through injecting PS into the lesion and illumination using a fiber-optic. The subcutaneous granulomas formed from collagen gels were infected with M. bovis. Benzoporphyrin derivative (BPD), activated by a diode laser, led to 0.7 log10 reduction in viable bacterial numbers (O'Riordan et al., 2006), and another PS 5-ethylamino-9-diethylaminobenzo[a]phenoselenazinium chloride (EtNBSe) resulted in at least a 2 log10 decrease (O'Riordan et al., 2007).
Otitis Media
Otitis media (OM) is a very common childhood infection that responds poorly to standard antibiotics. Jung et al. investigated the preclinical effect of Photogem-aPDT. Two days after injection of Streptococcus pneumoniae or Haemophilus influenzae cells into the bullae of gerbil ears to produce a model of OM, Photogem was injected into the bullae, followed by transcanal irradiation with a 632-nm diode laser (Jung et al., 2009). aPDT was effective in eradicating S. pneumoniae in 87% of the infected bullae with OM, and H. influenzae in 50% (Jung et al., 2009).
Clinical Trials and Patient Studies
As shown in Table 2, aPDT has been employed in several clinical trials of localized biofilm infections in humans.
Wound and Ulcer Infections
Two clinical trials were carried out for control of infections in non-healing chronic wounds (venous ulcers) and diabetic foot ulcers, using the phenothiazinium dye PP904 (3,7-bis(N,N-dibutylamino)-phenothiazinium bromide) (Morley et al., 2013) and tetracatiomic phthalocyanine RLP068 (Mannucci et al., 2014) as PS respectively. For 16 patients with chronic leg ulcers and 16 patients with diabetic foot ulcers with an ulcer duration longer than 3 months, PPA904 treatment for 15 min and red light irradiation at 50 J/cm2 were tolerated with no reports of pain and showed a reduction in bacterial load immediately post-treatment. Furthermore, half of the patients with actively treated chronic leg ulcers showed complete healing after 3 months, while only 12% of patients on placebo showed complete healing (Morley et al., 2013).
The RLP068 trial (Mannucci et al., 2014) was a randomized, double-blind, placebo-controlled phase IIa trial on 62 patients. aPDT was performed with topical application of three doses of RLP068 gel and 1 h later, red light irradiation with 60 J/cm2 was conducted. The results showed a reduction in microbial load dependent on dose, with the maximal killing efficiency (up to 3 log10) immediately post-illumination, and no safety issues.
Dental Infections
Clinical application of aPDT in dentistry have focused on treatment of infections arising from oral plaque biofilms. Root canals are commonly infected by bacteria, and the biofilm-forming E. faecalis is the most common. In order to sterilize root canals in patients, aPDT can be combined with the traditional approach of mechanical debridement for endodontic treatment (Tegos et al., 2006). aPDT was mediated by a conjugate between polyethylenimine and chlorin(e6) PEI-ce6 (Tegos et al., 2006) and illumination with a 660-nm laser. This approach improved the antibacterial effect evidenced by successive microbial sampling. Similarly, TBO-based aPDT and urea peroxide treatment achieved 82.59% reduction of viable bacteria via a synergistic effect (Pinheiro et al., 2009).
aPDT can be administered as an adjuvant after scaling and root planing (SRP) to treat patients with localized chronic periodontitis. In a study using competitive polymerase chain reaction (PCR) (Sigusch et al., 2010), a significant reduction of F. nucleatum DNA content was shown after 12 weeks, and significant reductions in reddening, bleeding on probing, and mean probing depth and a better level of clinical attachment were obtained. The adjuvant application of aPDT was shown to reduce intracellular DNA of F. nucleatum from 700–780 pg/ml (control group) to 50–180 pg/ml (aPDT group) and reduce periodontal inflammatory symptoms (Sigusch et al., 2010).
Nasal Decontamination
After preclinical testing of aPDT inactivation of nasal MRSA, a large clinical trial was conducted in Canada (Street et al., 2009). In combination with chlorhexidine gluconate, MB-aPDT led to 5.1 log10 reduction immediately and 5.9 log10 reduction after 24 h (Street et al., 2009). aPDT also reduced number of MRSA isolated from nasal swabs and number of post-operative surgical-site infections.
Helicobacter pylori Infection
H. pylori (HP) can cause gastritis and gastroduodenal ulceration in humans and can even lead to stomach cancer. In 13 HP positive volunteers, a zone of gastric antrum was irradiated with laser or endoscopic white light after oral 5-ALA. Results showed that 4 h post-irradiation, the maximum eradication efficiency was achieved at 85% of biopsies for laser treatment group (58% HP-negative in the control), and 66% in white light irradiated group (33% HP-negative in the control) (Wilder-Smith et al., 2002).
Hamblin's group reported that H. pylori naturally accumulated large quantities of photoactive free porphyrins, and was thus susceptible to inactivation with violet light (405 nm) even without addition of exogenous PS (Hamblin et al., 2005), which led to the conduction of two clinical trials. The first trial used a small 1 cm diameter spot from a 405-nm laser to irradiate a part of the gastric antrum, with biopsies taken before and after to quantify H. pylori colony unit forming (CFU) (Ganz et al., 2005). Promising results from that trial led to a second trial with whole stomach illumination with 405 nm light (Lembo et al., 2009). The second trial showed that intra-gastric violet light phototherapy may represent a feasible and safe approach for eradicating H. pylori, especially in patients who had failed standard antibiotic treatment (Lembo et al., 2009).
Possible Side-Effects
Up to now, aPDT has been shown to effectively eliminate bacteria and fungi with few side effects for the host tissues (Takasaki et al., 2009). The major side-effect after systemic administration of PS is the existence of a period of residual skin photosensitivity owing to PS accumulation. When activated by daylight, PS can cause burns, redness, and swelling until the drug is eliminated from the skin. These photosensitivity reactions could occur in minutes, therefore, exposure to bright light or direct sunlight must be strictly avoided. Other side-effects have included temporary coughing, trouble swallowing, stomach pain, or pain occurring at the area treated. There exist other potential side-effects that happen infrequently, such as allergic reaction, and change in liver function parameters (Vrouenraets et al., 2003; Kübler, 2005).
However the vast majority of the clinical applications for infections and biofilms, rely on topical or local administration of the PS, rather than systemic administration (intravenous or oral routes). Since the PS directly contact with the infected area, systemic absorption is thought to be minimal. Moreover, binding and uptake of the PS by the microbial cells and biofilms is rapid compared to the uptake by the surrounding host cells. Therefore, use of aPDI for localized infections usually requires a short drug-light interval (a few minutes) during which very little PS is expected to be taken up by host tissues, and subsequent light delivery will cause little damage to the tissue on which the biofilm is growing. The main issue with using aPDI for biofilm infections, is that after cessation of illumination, the production of actively antimicrobial ROS also ceases. Therefore, in animal models there have been problems with recurrence of microbial growth in the days following aPDT. This does not seem to have been such a big problem with clinical applications.
Along with development of novel PS, aPDT for infections will likely be more often clinically tested in the future and even receive more regulatory approvals as time goes on.
It is notable that in some preclinical in vivo animal studies (Jung et al., 2009; Baltazar et al., 2015) and clinical trials (Pinheiro et al., 2009), aPDT showed fungicidal efficiency or bactericidal efficiency below 3 log10CFU, thus much improvements of the aPDT method (alone and/or with conventional chemotherapy) are needed to increase the biofilm eradication rate.
Another point is that there are many light sources with a wide range of wavelengths had been employed in PDT, and no one light source is the optimum choice for different treatment regimes, even using the same PS (Coleman et al., 2010a; Agostinis et al., 2011). Both eye and skin injuries may occur from overexposure to high intensity light radiation, such as blue light can be toxic at high fluences (hundreds of J/cm2), thus it is important to avoid hazards from the light sources (Bullough, 2000). Fortunately, based on the publications in Tables 1, 2, no toxicity of light sources had been reported. While in the future, it should be investigated more deeply.
Concluding Remarks and Future Directions
Recently, the application of novel aPDT approaches in controlling biofilm infections had been reported both experimentally and clinically. Moreover, increasing evidence supports their effectiveness against other pathogens. In order to translate these findings into future clinical applications, several aspects deserve particular attention.
Detailed Mechanism of Action
Up to now, the multiple targets of ROS-mediated aPDT have not been investigated systematically. aPDT attacks biofilm on three separate fronts: biofilm matrix, cell surface, and cytoplasm. The successful elimination of biofilms can be attributed to the synergistic effects of damage on these three fronts. Thus, a more holistic understanding of aPDT-susceptible targets is urgently needed. A detailed understanding of intracellular effects by means of transcriptomics, proteomics, and metabolomics would help to understand the underlying anti-biofilm mechanism of aPDT.
More Efficient Delivery of PS and Use of Complementary Interventions
aPDT treatment is initiated by the delivery of the PS. Fabrication of PS into suitable drug delivery forms may be critical for a high anti-biofilm efficacy. Various nanoparticles such as chitosan nanoparticles (Darabpour et al., 2016), silver nanoparticles (Zhou et al., 2017), and graphene oxide (Xie et al., 2017) have been shown to be useful, while the particle size and incubation time appear to be crucial for controlling S. mutans and C. albicans biofilm and require further optimization (Chen et al., 2012). Moreover, the PS drug concentration also requires to be optimized (Araújo et al., 2014; Luke-Marshall et al., 2014). If the concentration is too high it can act as an “optical shield” preventing the light from penetrating deeply enough. Some complementary compounds can be combined with aPDT to stimulate the anti-biofilm efficiency. For instance a QS inhibitor (QSI) would be a promising addition to PS. Some reported QSI such as diketopiperazines (de Carvalho and Abraham, 2012) have shown excellent potential for treatment of biofilm infections (Chung and Toh, 2014). Besides, efflux pump inhibitors could play a role, considering that some PS (such as phenothiazinium salts) have been shown to be substrates of microbial efflux pumps (Tegos and Hamblin, 2006). When verapamil, an efflux pump inhibitor, was combined with aPDT, a lower light dose was needed to get a higher reduction of cellular metabolism and reduced viability of bacterial biofilms, compared to that with PS alone, indicating the combination of aPDT with effux pump inhibitors may be more effective (de Aguiar Coletti et al., 2017).
Possibility of Inducing Lower Susceptibility to aPDT and Higher Virulence
Factors that affect the susceptibility of different microbial strains to aPDT need to be studied. After sub-lethal doses of aPDT, the biofilm-forming ability of S. aureus clinical isolates was increased (Kashef et al., 2013), which could make it less susceptible to a second application of aPDT. Blue light can activate production of colonic acid, a biofilm polysaccharide, produced under the influence of the biofilm-associated bdm gene in E. coli (Tschowri et al., 2009).
Other virulence factors such as a higher resistance to antibiotics could appear after aPDT (Kashef et al., 2013). Another virulence factor is modified LPS with altered expression of negatively charged phosphate groups (Renzi et al., 2015), and this change would likely weaken the binding of the PS. Besides, LPS can affect the polysaccharide chain itself (Díaz et al., 2015) to alter the hydrophobicity of the cell surface, which can influence the biofilm-forming ability of the cells. Future work is also needed to study effects on virulence factors after sub-lethal aPDT.
aPDT had been recently proposed to combat clinically relevant biofilms. The harmless visible light excited PS to generate ROS, which non-specifically attacked adjacent targets to damage both planktonic cells and biofilms. Numerous in vitro and in vivo aPDT studies demonstrated biofilm-eradication activities, and future work should be made to further improve efficiency in controlling biofilm.
Author Contributions
XH wrote the manuscript. Y-YH and YW helped to write the section of preclinical and clinical studies. XW helped to prepare the section of underlying mechanism. MH prepared Figure 1 and Table 3, rearranged and improved the manuscript.
Conflict of Interest Statement
The authors declare that the research was conducted in the absence of any commercial or financial relationships that could be construed as a potential conflict of interest.
Acknowledgments
XH was funded by grants from National Key R&D Program of China (2017YFC1600102), China National Natural Science Foundation (31201290), and national first-class discipline program of Light Industry Technology and Engineering (LITE2018-10). MH was supported by US NIH grants R01AI050875 and R21AI121700.
Supplementary Material
The Supplementary Material for this article can be found online at: https://www.frontiersin.org/articles/10.3389/fmicb.2018.01299/full#supplementary-material
References
Abrahamse, H., and Hamblin, M. R. (2016). New photosensitizers for photodynamic therapy. Biochem. J. 473, 347–364. doi: 10.1042/BJ20150942
Adair, C. G., Gorman, S. P., Feron, B. M., Byers, L. M., Jones, D. S., Goldsmith, C. E., et al. (1999). Implications of endotracheal tube biofilm for ventilator-associated pneumonia. Intens. Care Med. 25, 1072–1076. doi: 10.1007/s001340051014
Adetunji, V. O., Kehinde, A. O., Bolatito, O. K., and Chen, J. (2014). Biofilm formation by Mycobacterium bovis: influence of surface kind and temperatures of sanitizer treatments on biofilm control. Biomed. Res. Int. 2014, 1–7. doi: 10.1155/2014/210165
Afkhami, F., Akbari, S., and Chiniforush, N. (2017). Entrococcus faecalis elimination in root canals using silver nanoparticles, photodynamic therapy, diode laser, or laser-activated nanoparticles: an in vitro study. J. Endod. 43, 279–282. doi: 10.1016/j.joen.2016.08.029
Agostinis, P., Berg, K., Cengel, K. A., Foster, T. H., Girotti, A. W., Gollnick, S. O., et al. (2011). Photodynamic therapy of cancer: an update. CA Cancer J. Clin. 61, 250–281. doi: 10.3322/caac.20114
Al-Ahmad, A., Walankiewicz, A., Hellwig, E., Follo, M., Tennert, C., Wittmer, A., et al. (2016). Photoinactivation using visible light plus water-filtered infrared-A (vis+wIRA) and chlorine e6 (Ce6) eradicates planktonic periodontal pathogens and subgingival biofilms. Front. Microbiol. 7:1900. doi: 10.3389/fmicb.2016.01900
Alemayehu, D., Casey, P. G., McAuliffe, O., Guinane, C. M., Martin, J. G., Shanahan, F., et al. (2012). Bacteriophages phiMR299-2 and phiNH-4 can eliminate Pseudomonas aeruginosa in the murine lung and on cystic fibrosis lung airway cells. MBio 3:e00029–e00012. doi: 10.1128/mBio.00029-12
Alipour, M., Suntres, Z. E., and Omri, A. (2009). Importance of DNase and alginate lyase for enhancing free and liposome encapsulated aminoglycoside activity against Pseudomonas aeruginosa. J. Antimicrob. Chemother. 64, 317–325. doi: 10.1093/jac/dkp165
Alkawash, M. A., Soothill, J. S., and Schiller, N. L. (2006). Alginate lyase enhances antibiotic killing of mucoid Pseudomonas aeruginosa in biofilms. APMIS 114, 131–138. doi: 10.1111/j.1600-0463.2006.apm_356.x
Alvarenga, L. H., Prates, R. A., Yoshimura, T. M., Kato, I. T., Suzuki, L. C., Ribeiro, M. S., et al. (2015). Aggregatibacter actinomycetemcomitans biofilm can be inactivated by methylene blue-mediated photodynamic therapy. Photodiagnosis Photodyn. Ther. 12, 131–135. doi: 10.1016/j.pdpdt.2014.10.002
Alves, E., Melo, T., Simões, C., Faustino, M. A., Tomé, J. P., Neves, M. G., et al. (2013a). Photodynamic oxidation of Staphylococcus warneri membrane phospholipids: new insights based on lipidomics. Rapid Commun. Mass. Spectrom. 27, 1607–1618. doi: 10.1002/rcm.6614
Alves, E., Santos, N., Melo, T., Maciel, E., Dória, M. L., Faustino, M. A., et al. (2013b). Photodynamic oxidation of Escherichia coli membrane phospholipids: new insights based on lipidomics. Rapid Commun. Mass Spectrom. 27, 2717–2728. doi: 10.1002/rcm.6739
Alves, F., de Oliveira Mima, E. G., Passador, R. C. P., Bagnato, V. S., Jorge, J. H., and Pavarina, A. C. (2017). Virulence factors of fluconazole-susceptible and fluconazole-resistant Candida albicans after antimicrobial photodynamic therapy. Lasers Med. Sci. 32, 815–826. doi: 10.1007/s10103-017-2177-y
Amara, N., Mashiach, R., Amar, D., Krief, P., Spieser, S. A., Bottomley, M. J., et al. (2009). Covalent inhibition of bacterial quorum sensing. J. Am. Chem. Soc. 131, 10610–10619. doi: 10.1021/ja903292v
Ammons, M. C., Tripet, B. P., Carlson, R. P., Kirker, K. R., Gross, M. A., Stanisich, J. J., et al. (2014). Quantitative NMR metabolite profiling of methicillin-resistant and methicillin-susceptible Staphylococcus aureus discriminates between biofilm and planktonic phenotypes. J. Proteome Res. 13, 2973–2985. doi: 10.1021/pr500120c
Andrade, M. C., Ribeiro, A. P., Dovigo, L. N., Brunetti, I. L., Giampaolo, E. T., Bagnato, V. S., et al. (2013). Effect of different pre-irradiation times on curcumin-mediated photodynamic therapy against planktonic cultures and biofilms of Candida spp. Arch. Oral Biol. 58, 200–210. doi: 10.1016/j.archoralbio.2012.10.011
Araújo, N. C., Fontana, C. R., Bagnato, V. S., and Gerbi, M. E. (2014). Photodynamic antimicrobial therapy of curcumin in biofilms and carious dentine. Lasers Med. Sci. 29, 629–635. doi: 10.1007/s10103-013-1369-3
Azoulay, E., Timsit, J. F., Tafflet, M., de Lassence, A., Darmon, M., Zahar, J. R., et al. (2006). Candida colonization of the respiratory tract and subsequent pseudomonas ventilator-associated pneumonia. Chest 129, 110–117. doi: 10.1378/chest.129.1.110
Balaban, N., Cirioni, O., Giacometti, A., Ghiselli, R., Braunstein, J. B., Silvestri, C., et al. (2007). Treatment of Staphylococcus aureus biofilm infection by the quorum-sensing inhibitor RIP. Antimicrob. Agents Chemother. 51, 2226–2229. doi: 10.1128/AAC.01097-06
Baltazar, L. M., Werneck, S. M., Carneiro, H. C., Gouveia, L. F., de Paula, T. P., Byrro, R. M., et al. (2015). Photodynamic therapy efficiently controls dermatophytosis caused by Trichophyton rubrum in a murine model. Br. J. Dermatol. 172, 801–804. doi: 10.1111/bjd.13494
Beirão, S., Fernandes, S., Coelho, J., Faustino, M. A., Tomé, J. P., Neves, M. G., et al. (2014). Photodynamic inactivation of bacterial and yeast biofilms with a cationic porphyrin. Photochem. Photobiol. 90, 1387–1396. doi: 10.1111/php.12331
Bertoloni, G., Lauro, F. M., Cortella, G., and Merchat, M. (2000). Photosensitizing activity of hematoporphyrin on Staphylococcus aureus cells. Biochim. Biophys. Acta 1475, 169–174. doi: 10.1016/S0304-4165(00)00071-4
Biel, M. A., Pedigo, L., Gibbs, A., and Loebel, N. (2013). Photodynamic therapy of antibiotic-resistant biofilms in a maxillary sinus model. Int. Forum Allergy Rh. 3, 468–473. doi: 10.1002/alr.21134
Biel, M. A., Sievert, C., Usacheva, M., Teichert, M., and Balcom, J. (2011a). Antimicrobial photodynamic therapy treatment of chronic recurrent sinusitis biofilms. Int. Forum Allergy Rh. 1, 329–334. doi: 10.1002/alr.20089
Biel, M. A., Sievert, C., Usacheva, M., Teichert, M., Wedell, E., Loebel, N., et al. (2011b). Reduction of endotracheal tube biofilms using antimicrobial photodynamic therapy. Lasers Surg. Med. 43, 586–590. doi: 10.1002/lsm.21103
Billings, N., Millan, M., Caldara, M., Rusconi, R., Tarasova, Y., Stocker, R., et al. (2013). The extracellular matrix component Psl provides fast-acting antibiotic defense in Pseudomonas aeruginosa biofilms. PLoS Pathog. 9:e1003526. doi: 10.1371/journal.ppat.1003526
Bisland, S. K., and Burch, S. (2006). Photodynamic therapy of diseased bone. Photodiagn. Photodyn. Ther. 3, 147–155. doi: 10.1016/S1572-1000(06)00036-6
Bisland, S. K., Chien, C., Wilson, B. C., and Burch, S. (2006). Pre-clinical in vitro and in vivo studies to examine the potential use of photodynamic therapy in the treatment of osteomyelitis. Photochem. Photobiol. Sci. 5, 31–38. doi: 10.1039/B507082A
Bjarnsholt, T., Jensen, P. Ø., Rasmussen, T. B., Christophersen, L., Calum, H., Hentzer, M., et al. (2005). Garlic blocks quorum sensing and promotes rapid clearing of pulmonary Pseudomonas aeruginosa infections. Microbiology 151(Pt 12), 3873–3880. doi: 10.1099/mic.0.27955-0
Briggs, T., Blunn, G., Hislop, S., Ramalhete, R., Bagley, C., McKenna, D., et al. (2018). Antimicrobial photodynamic therapy-a promising treatment for prosthetic joint infections. Lasers Med. Sci. 33, 523–532. doi: 10.1007/s10103-017-2394-4
Bullough, J. D. (2000). The blue-light hazard: a review. J. Illumin. Eng. Soc. 29, 6–14. doi: 10.1080/00994480.2000.10748312
Burrowes, B., Harper, D. R., Anderson, J., McConville, M., and Enright, M. C. (2011). Bacteriophage therapy: potential uses in the control of antibiotic-resistant pathogens. Expert Rev. Anti Infect. Ther. 9, 775–785. doi: 10.1586/eri.11.90
Caminos, D. A., Spesia, M. B., Pons, P., and Durantini, E. N. (2008). Mechanisms of Escherichia coli photodynamic inactivation by an amphiphilic tricationic porphyrin and 5,10,15,20-tetra(4-N,N,N-trimethylammoniumphenyl) porphyrin. Photochem. Photobiol. Sci. 7, 1071–1078. doi: 10.1039/b804965c
Carmello, J. C., Alves, F., Mima, E. G. O., Jorge, J. H., Bagnato, V. S., and Pavarina, A. C. (2017). Photoinactivation of single and mixed biofilms of Candida albicans and non-albicans Candida species using Phorodithazine(R). Photodiagn. Photodyn. Ther. 17, 194–199. doi: 10.1016/j.pdpdt.2016.11.013
Caruso, E., Banfi, S., Barbieri, P., Leva, B., and Orlandi, V. T. (2012). Synthesis and antibacterial activity of novel cationic BODIPY photosensitizers. J. Photochem. Photobiol. B 114, 44–51. doi: 10.1016/j.jphotobiol.2012.05.007
Carvalho, C. M., Alves, E., Costa, L., Tomé, J. P., Faustino, M. A., Neves, M. G., et al. (2010). Functional cationic nanomagnet – porphyrin hybrids for the photoinactivation of microorganisms. ACS Nano 4, 7133–7140. doi: 10.1021/nn1026092
Carvalho, C. M., Gomes, A. T., Fernandes, S. C., Prata, A. C., Almeida, M. A., Cunha, M. A., et al. (2007). Photoinactivation of bacteria in wastewater by porphyrins: bacterial beta-galactosidase activity and leucine-uptake as methods to monitor the process. J. Photochem. Photobiol. B 88, 112–118. doi: 10.1016/j.jphotobiol.2007.04.015
Catala, A. (2009). Lipid peroxidation of membrane phospholipids generates hydroxy-alkenals and oxidized phospholipids active in physiological and/or pathological conditions. Chem. Phys. Lipids 157, 1–11. doi: 10.1016/j.chemphyslip.2008.09.004
Cegelski, L., Pinkner, J. S., Hammer, N. D., Cusumano, C. K., Hung, C. S., Chorell, E., et al. (2009). Small-molecule inhibitors target Escherichia coli amyloid biogenesis and biofilm formation. Nat. Chem. Biol. 5, 913–919. doi: 10.1038/nchembio.242
Ceri, H., Olson, M. E., Stremick, C., Read, R. R., Morck, D., and Buret, A. (1999). The Calgary Biofilm Device: new technology for rapid determination of antibiotic susceptibilities of bacterial biofilms. J. Clin. Microbiol. 37, 1771–1776.
Cernáková, L., Chupacova, J., Zidlikova, K., and Bujdakova, H. (2015). Effectiveness of the photoactive dye methylene blue versus Caspofungin on the Candida parapsilosis biofilm in vitro and ex vivo. Photochem. Photobiol. 91, 1181–1190. doi: 10.1111/php.12480
Cevizci, R., Düzlü, M., Dündar, Y., Noyanalpan, N., Sultan, N., Tutar, H., et al. (2015). Preliminary results of a novel quorum sensing inhibitor against pneumococcal infection and biofilm formation with special interest to otitis media and cochlear implantation. Eur. Arch. Otorhinolaryngol. 272, 1389–1393. doi: 10.1007/s00405-014-2942-5
Chabrier-Roselló, Y., Foster, T. H., Mitra, S., and Haidaris, C. G. (2008). Respiratory deficiency enhances the sensitivity of the pathogenic fungus Candida to photodynamic treatment. Photochem. Photobiol. 84, 1141–1148. doi: 10.1111/j.1751-1097.2007.00280.x
Chan, B. K., and Abedon, S. T. (2015). Bacteriophages and their enzymes in biofilm control. Curr. Pharm. Des. 21, 85–99. doi: 10.2174/1381612820666140905112311
Chandra, J., Kuhn, D. M., Mukherjee, P. K., Hoyer, L. L., McCormick, T., and Ghannoum, M. A. (2001). Biofilm formation by the fungal pathogen Candida albicans: development, architecture, and drug resistance. J. Bacteriol. 183, 5385–5394. doi: 10.1128/JB.183.18.5385-5394.2001
Chen, C. P., Chen, C. T., and Tsai, T. (2012). Chitosan nanoparticles for antimicrobial photodynamic inactivation: characterization and in vitro investigation. Photochem. Photobiol. 88, 570–576. doi: 10.1111/j.1751-1097.2012.01101.x
Chien, H. F., Chen, C. P., Chen, Y. C., Chang, P. H., Tsai, T., and Chen, C. T. (2013). The use of chitosan to enhance photodynamic inactivation against Candida albicans and its drug-resistant clinical isolates. Int. J. Mol. Sci. 14, 7445–7456. doi: 10.3390/ijms14047445
Cho, K., Lee, S. Y., Chang, B. S., Um, H. S., and Lee, J. K. (2015). The effect of photodynamic therapy on Aggregatibacter actinomycetemcomitans attached to surface-modified titanium. J. Periodontal Implant Sci. 45, 38–45. doi: 10.5051/jpis.2015.45.2.38
Chung, P. Y., and Toh, Y. S. (2014). Anti-biofilm agents: recent breakthrough against multi-drug resistant Staphylococcus aureus. Pathog. Dis. 70, 231–239. doi: 10.1111/2049-632X.12141
Chusri, S., Phatthalung, P. N., and Voravuthikunchai, S. P. (2012). Anti-biofilm activity of Quercus infectoria G. Olivier against methicillin-resistant Staphylococcus aureus. Lett. Appl. Microbiol. 54, 511–517. doi: 10.1111/j.1472-765X.2012.03236.x
Cieplik, F., Spath, A., Regensburger, J., Gollmer, A., Tabenski, L., Hiller, K. A., et al. (2013). Photodynamic biofilm inactivation by SAPYR–an exclusive singlet oxygen photosensitizer. Free Radic. Biol. Med. 65, 477–487. doi: 10.1016/j.freeradbiomed.2013.07.031
Cirioni, O., Mocchegiani, F., Cacciatore, I., Vecchiet, J., Silvestri, C., Baldassarre, L., et al. (2013). Quorum sensing inhibitor FS3-coated vascular graft enhances daptomycin efficacy in a rat model of staphylococcal infection. Peptides 40, 77–81. doi: 10.1016/j.peptides.2012.12.002
Coleman, A., Fedele, F., Khazova, M., Freeman, P., and Sarkany, R. (2010a). A survey of the optical hazards associated with hospital light sources with reference to the Control of Artificial Optical Radiation at Work Regulations 2010. J. Radiol. Prot. 30, 469–489. doi: 10.1088/0952-4746/30/3/004
Coleman, J. J., Okoli, I., Tegos, G. P., Holson, E. B., Wagner, F. F., Hamblin, M. R., et al. (2010b). Characterization of plant-derived saponin natural products against Candida albicans. ACS Chem. Biol. 5, 321–332. doi: 10.1021/cb900243b
Cook, N. (1998). Methicillin-resistant Staphylococcus aureus versus the burn patient. Burns 24, 91–98. doi: 10.1016/S0305-4179(97)00114-9
Costa, A. C., Campos Rasteiro, V. M., da Silva Hashimoto, E. S., Araújo, C. F., Pereira, C. A., Junqueira, J. C., et al. (2012a). Effect of erythrosine- and LED-mediated photodynamic therapy on buccal candidiasis infection of immunosuppressed mice and Candida albicans adherence to buccal epithelial cells. Oral Surg. Oral Med. Oral Pathol. Oral Radiol. 114, 67–74. doi: 10.1016/j.oooo.2012.02.002
Costa, A. C., Rasteiro, V. M., Pereira, C. A., Rossoni, R. D., Junqueira, J. C., and Jorge, A. O. (2012b). The effects of rose bengal- and erythrosine-mediated photodynamic therapy on Candida albicans. Mycoses 55, 56–63. doi: 10.1111/j.1439-0507.2011.02042.x
Dai, T., Bil de Arce, V. J., Tegos, G. P., and Hamblin, M. R. (2011). Blue dye and red light, a dynamic combination for prophylaxis and treatment of cutaneous Candida albicans infections in mice. Antimicrob. Agent Chemother. 55, 5710–5717. doi: 10.1128/AAC.05404-11
Dai, T., Tegos, G. P., Zhiyentayev, T., Mylonakis, E., and Hamblin, M. R. (2010). Photodynamic therapy for methicillin-resistant Staphylococcus aureus infection in a mouse skin abrasion model. Laser Surg. Med. 42, 38–44. doi: 10.1002/lsm.20887
Darabpour, E., Kashef, N., and Mashayekhan, S. (2016). Chitosan nanoparticles enhance the efficiency of methylene blue-mediated antimicrobial photodynamic inactivation of bacterial biofilms: an in vitro study. Photodiagn. Photodyn. Ther. 14, 211–217. doi: 10.1016/j.pdpdt.2016.04.009
DaSilva, L., Finer, Y., Friedman, S., Basrani, B., and Kishen, A. (2013). Biofilm formation within the interface of bovine root dentin treated with conjugated chitosan and sealer containing chitosan nanoparticles. J. Endod. 39, 249–253. doi: 10.1016/j.joen.2012.11.008
Davey, M. E., and O'Toole, G. A (2000). Microbial biofilms: from ecology to molecular genetics. Microbiol. Mol. Biol. Rev. 64, 847–867. doi: 10.1128/MMBR.64.4.847-867.2000
Davies, D. G., and Marques, C. N. (2009). A fatty acid messenger is responsible for inducing dispersion in microbial biofilms. J. Bacteriol. 191, 1393–1403. doi: 10.1128/JB.01214-08
Davies, D. G., Parsek, M. R., Pearson, J. P., Iglewski, B. H., Costerton, J. W., and Greenberg, E. P. (1998). The involvement of cell-to-cell signals in the development of a bacterial biofilm. Science 280, 295–298. doi: 10.1126/science.280.5361.295
de Aguiar Coletti, T. M. S. F., de Freitas, L. M., Almeida, A. M. F., and Fontana, C. R. (2017). Optimization of antimicrobial photodynamic therapy in biofilms by inhibiting efflux pump. Photomed. Laser Surg. 35, 378–385. doi: 10.1089/pho.2016.4246
de Carvalho, M. P., and Abraham, W. R. (2012). Antimicrobial and biofilm inhibiting diketopiperazines. Curr. Med. Chem. 19, 3564–3577. doi: 10.2174/092986712801323243
de Freitas, L. M., Calixto, G. M., Chorilli, M., Giusti, J. S., Bagnato, V. S., Soukos, N. S., et al. (2016). Polymeric nanoparticle-based photodynamic therapy for chronic periodontitis in vivo. Int. J. Mol. Sci. 17, 769–781. doi: 10.3390/ijms17050769
de Freitas-Pontes, K. M., Gomes, C. E., de Carvalho, B. M., Sabóia Rde, S., and Garcia, B. A. (2014). Photosensitization of in vitro biofilms formed on denture base resin. J. Prosthet. Dent. 112, 632–637. doi: 10.1016/j.prosdent.2014.01.001
de Melo, W. C., Avci, P., de Oliveira, M. N., Gupta, A., Vecchio, D., Sadasivam, M., et al. (2013). Photodynamic inactivation of biofilm: taking a lightly colored approach to stubborn infection. Expert Rev. Anti Infect. Ther. 11, 669–693. doi: 10.1586/14787210.2013.811861
de Oliveira Guaré, R., Costa, S. C., Baeder, F., de Souza Merli, L. A., and Dos Santos, M. T. (2010). Drug-induced gingival enlargement: biofilm control and surgical therapy with gallium-aluminum-arsenide (GaAlAs) diode laser-A 2-year follow-up. Spec. Care Dent. 30, 46–52. doi: 10.1111/j.1754-4505.2009.00126.x
Díaz, L., Hoare, A., Soto, C., Bugueño, I., Silva, N., Dutzan, N., et al. (2015). Changes in lipopolysaccharide profile of Porphyromonas gingivalis clinical isolates correlate with changes in colony morphology and polymyxin B resistance. Anaerobe 33, 25–32. doi: 10.1016/j.anaerobe.2015.01.009
Donlan, R. M. (2002). Biofilms: microbial life on surfaces. Emerg. Infect. Dis. 8, 881–890. doi: 10.3201/eid0809.020063
Dosselli, R., Millioni, R., Puricelli, L., Tessari, P., Arrigoni, G., Franchin, C., et al. (2012). Molecular targets of antimicrobial photodynamic therapy identified by a proteomic approach. J. Proteomics 77, 329–343. doi: 10.1016/j.jprot.2012.09.007
Dovigo, L. N., Carmello, J. C., Carvalho, M. T., Mima, E. G., Vergani, C. E., Bagnato, V. S., et al. (2013). Photodynamic inactivation of clinical isolates of Candida using Photodithazine(R). Biofouling 29, 1057–1067. doi: 10.1080/08927014.2013.827668
Dwivedi, S., Sharma, A., Patrick, B., Sharma, R., and Awasthi, Y. C. (2007). Role of 4-hydroxynonenal and its metabolites in signaling. Redox Rep. 12, 4–10. doi: 10.1179/135100007X162211
Dworniczek, E., Piwowarczyk, J., Seniuk, A., and Gościniak, G. (2014). Enterococcus–virulence and susceptibility to photodynamic therapy of clinical isolates from Lower Silesia, Poland. Scand. J. Infect. Dis. 46, 846–853. doi: 10.3109/00365548.2014.952244
Edwards, R., and Harding, K. G. (2004). Bacteria and wound healing. Curr. Opin. Infect. Dis. 17, 91–96. doi: 10.1097/00001432-200404000-00004
Eick, S., Meier, I., Spoerlé, F., Bender, P., Aoki, A., Izumi, Y., et al. (2017). In vitro-activity of Er: YAG laser in comparison with other treatment modalities on biofilm ablation from implant and tooth surfaces. PLoS ONE 12:e0171086. doi: 10.1371/journal.pone.0171086
El-Feky, M. A., El-Rehewy, M. S., Hassan, M. A., Abolella, H. A., Abd El-Baky, R. M., and Gad, G. F. (2009). Effect of ciprofloxacin and N-acetylcysteine on bacterial adherence and biofilm formation on ureteral stent surfaces. Pol. J. Microbiol. 58, 261–267.
Filogoniô Cde, F., Soares, R. V., Horta, M. C., Penido, C. V., and Cruz Rde, A. (2011). Effect of vegetable oil (Brazil nut oil) and mineral oil (liquid petrolatum) on dental biofilm control. Braz. Oral Res. 25, 556–561. doi: 10.1590/S1806-83242011000600014
Frade, V. H. J., Coutinho, P. J. G., Moura, J. C. V. P., and Gonçalves, M. S. T. (2007). Functionalised benzo[a]phenoxazine dyes as long-wavelength fluorescent probes for amino acids. Tetrahedron 63, 1654–1663. doi: 10.1016/j.tet.2006.12.005
Freire, F., Costa, A. C., Pereira, C. A., Beltrame Junior, M., Junqueira, J. C., and Jorge, A. O. (2014). Comparison of the effect of rose bengal- and eosin Y-mediated photodynamic inactivation on planktonic cells and biofilms of Candida albicans. Lasers Med. Sci. 29, 949–955. doi: 10.1007/s10103-013-1435-x
Freire, F., de Barros, P. P., da Silva Ávila, D., Brito, G. N., Junqueira, J. C., and Jorge, A. O. (2015). Evaluation of gene expression SAP5, LIP9, and PLB2 of Candida albicans biofilms after photodynamic inactivation. Lasers Med. Sci. 30, 1511–1518. doi: 10.1007/s10103-015-1747-0
Gad, F., Zahra, T., Hasan, T., and Hamblin, M. R. (2004). Effects of growth phase and extracellular slime on photodynamic inactivation of gram-positive pathogenic bacteria. Antimicrob. Agent Chemother. 48, 2173–2178. doi: 10.1128/AAC.48.6.2173-2178.2004
Ganz, R. A., Viveiros, J., Ahmad, A., Ahmadi, A., Khalil, A., Tolkoff, M. J., et al. (2005). Helicobacter pylori in patients can be killed by visible light. Lasers Surg. Med. 36, 260–265. doi: 10.1002/lsm.20161
Garcez, A. S., Núñez, S. C., Azambuja, N. Jr., Fregnani, E. R., Rodriguez, H. M., Hamblin, M. R., et al. (2013). Effects of photodynamic therapy on Gram-positive and Gram-negative bacterial biofilms by bioluminescence imaging and scanning electron microscopic analysis. Photomed. Laser Surg. 31, 519–525. doi: 10.1089/pho.2012.3341
Garcez, A. S., Nuñez, S. C., Hamblim, M. R., Suzuki, H., and Ribeiro, M. S. (2010). Photodynamic therapy associated with conventional endodontic treatment in patients with antibiotic-resistant microflora: a preliminary report. J. Endod. 36, 1463–1466. doi: 10.1016/j.joen.2010.06.001
Gbejuade, H. O., Lovering, A. M., and Webb, J. C. (2015). The role of microbial biofilms in prosthetic joint infections. Acta Orthop. 86, 147–158. doi: 10.3109/17453674.2014.966290
George, S., Hamblin, M. R., and Kishen, A. (2009). Uptake pathways of anionic and cationic photosensitizers into bacteria. Photochem. Photobiol. Sci. 8, 788–795. doi: 10.1039/b809624d
Geske, G. D., Wezeman, R. J., Siegel, A. P., and Blackwell, H. E. (2005). Small molecule inhibitors of bacterial quorum sensing and biofilm formation. J. Am. Chem. Soc. 127, 12762–12763. doi: 10.1021/ja0530321
Giannelli, M., Landini, G., Materassi, F., Chellini, F., Antonelli, A., Tani, A., et al. (2017). Effects of photodynamic laser and violet-blue led irradiation on Staphylococcus aureus biofilm and Escherichia coli lipopolysaccharide attached to moderately rough titanium surface: in vitro study. Lasers Med. Sci. 32, 857–864. doi: 10.1007/s10103-017-2185-y
Gil-Perotin, S., Ramirez, P., Marti, V., Sahuquillo, J. M., Gonzalez, E., Calleja, I., et al. (2012). Implications of endotracheal tube biofilm in ventilator-associated pneumonia response: a state of concept. Crit. Care 16, R93. doi: 10.1186/cc11357
Glansdorp, F. G., Thomas, G. L., Lee, J. K., Dutton, J. M., Salmond, G. P., Welch, M., et al. (2004). Synthesis and stability of small molecule probes for Pseudomonas aeruginosa quorum sensing modulation. Org. Biomol. Chem. 2, 3329–3336. doi: 10.1039/b412802h
Gomes, M. C., Woranovicz-Barreira, S. M., Faustino, M. A., Fernandes, R., Neves, M. G., Tomé, A. C., et al. (2011). Photodynamic inactivation of Penicillium chrysogenum conidia by cationic porphyrins. Photochem. Photobiol. Sci. 10, 1735–1743. doi: 10.1039/c1pp05174a
Gonzales, F. P., Felgenträger, A., Bäumler, W., and Maisch, T. (2013). Fungicidal photodynamic effect of a twofold positively charged porphyrin against Candida albicans planktonic cells and biofilms. Future Microbiol. 8, 785–797. doi: 10.2217/fmb.13.44
Goosen, N., and Moolenaar, G. F. (2008). Repair of UV damage in bacteria. DNA Repair 7, 353–379. doi: 10.1016/j.dnarep.2007.09.002
Gracanin, M., Hawkins, C. L., Pattison, D. I., and Davies, M. J. (2009). Singlet-oxygen-mediated amino acid and protein oxidation: formation of tryptophan peroxides and decomposition products. Free Radic. Biol. Med. 47, 92–102. doi: 10.1016/j.freeradbiomed.2009.04.015
Grinholc, M., Rapacka-Zdonczyk, A., Rybak, B., Szabados, F., and Bielawski, K. P. (2014). Multiresistant strains are as susceptible to photodynamic inactivation as their naive counterparts: protoporphyrin IX-mediated photoinactivation reveals differences between methicillin-resistant and methicillin-sensitive Staphylococcus aureus strains. Photomed. Laser Surg. 32, 121–129. doi: 10.1089/pho.2013.3663
Hall-Stoodley, L., Costerton, J. W., and Stoodley, P. (2004). Bacterial biofilms: from the natural environment to infectious diseases. Nat. Rev. Microbiol. 2, 95–108. doi: 10.1038/nrmicro821
Hamblin, M. R., and Hasan, T. (2004). Photodynamic therapy: a new antimicrobial approach to infectious disease? Photochem. Photobiol. Sci. 3, 436–450. doi: 10.1039/b311900a
Hamblin, M. R., O'Donnell, D. A., Murthy, N., Rajagopalan, K., Michaud, N., Sherwood, M. E., et al. (2002). Polycationic photosensitizer conjugates: effects of chain length and Gram classification on the photodynamic inactivation of bacteria. J. Antimicrob. Chemother. 49, 941–951. doi: 10.1093/jac/dkf053
Hamblin, M. R., Viveiros, J., Yang, C., Ahmadi, A., Ganz, R. A., and Tolkoff, M. J. (2005). Helicobacter pylori accumulates photoactive porphyrins and is killed by visible light. Antimicrob. Agent Chemother. 49, 2822–2827. doi: 10.1128/AAC.49.7.2822-2827.2005
Hancock, R. E., and Bell, A. (1988). Antibiotic uptake into gram-negative bacteria. Eur. J. Clin. Microbiol. Infect. Dis. 7, 713–720. doi: 10.1007/BF01975036
Hengzhuang, W., Ciofu, O., Yang, L., Wu, H., Song, Z., Oliver, A., et al. (2013). High beta-lactamase levels change the pharmacodynamics of beta-lactam antibiotics in Pseudomonas aeruginosa biofilms. Antimicrob. Agent Chemother. 57, 196–204. doi: 10.1128/AAC.01393-12
Hentzer, M., and Givskov, M. (2003). Pharmacological inhibition of quorum sensing for the treatment of chronic bacterial infections. J. Clin. Invest. 112, 1300–1307. doi: 10.1172/JCI20074
Hirakawa, K., Ota, K., Hirayama, J., Oikawa, S., and Kawanishi, S. (2014). Nile blue can photosensitize DNA damage through electron transfer. Chem. Res. Toxicol. 27, 649–655. doi: 10.1021/tx400475c
Hoiby, N. (2017). A short history of microbial biofilms and biofilm infections. APMIS 125, 272–275. doi: 10.1111/apm.12686
Høiby, N., Bjarnsholt, T., Givskov, M., Molin, S., and Ciofu, O. (2010). Antibiotic resistance of bacterial biofilms. Int. J. Antimicrob. Agent 35, 322–332. doi: 10.1016/j.ijantimicag.2009.12.011
Howlin, R. P., Fabbri, S., Offin, D. G., Symonds, N., Kiang, K. S., Knee, R. J., et al. (2015). Removal of dental biofilms with an ultrasonically activated water stream. J. Dent. Res. 94, 1303–1309. doi: 10.1177/0022034515589284
Ishida, T., Ikeda, T., Takiguchi, N., Kuroda, A., Ohtake, H., and Kato, J. (2007). Inhibition of quorum sensing in Pseudomonas aeruginosa by N-acyl cyclopentylamides. Appl. Environ. Microbiol. 73, 3183–3188. doi: 10.1128/AEM.02233-06
Istanbullu, O., Babauta, J., Duc Nguyen, H., and Beyenal, H. (2012). Electrochemical biofilm control: mechanism of action. Biofouling 28, 769–778. doi: 10.1080/08927014.2012.707651
Itoh, Y., Wang, X., Hinnebusch, B. J., Preston, J. F. III., and Romeo, T. (2005). Depolymerization of beta-1,6-N-acetyl-D-glucosamine disrupts the integrity of diverse bacterial biofilms. J. Bacteriol. 187, 382–387. doi: 10.1128/JB.187.1.382-387.2005
Izano, E. A., Amarante, M. A., Kher, W. B., and Kaplan, J. B. (2008). Differential roles of poly-N-acetylglucosamine surface polysaccharide and extracellular DNA in Staphylococcus aureus and Staphylococcus epidermidis biofilms. Appl. Environ. Microbiol. 74, 470–476. doi: 10.1128/AEM.02073-07
Jung, J. Y., Kwon, P. S., Ahn, J. C., Ge, R., Suh, M. W., and Rhee, C. K. (2009). In vitro and in vivo photodynamic therapy of otitis media in gerbils. Laryngoscope 119, 1781–1787. doi: 10.1002/lary.20568
Kaplan, J. B., Ragunath, C., Velliyagounder, K., Fine, D. H., and Ramasubbu, N. (2004). Enzymatic detachment of Staphylococcus epidermidis biofilms. Antimicrob. Agent Chemother. 48, 2633–2636. doi: 10.1128/AAC.48.7.2633-2636.2004
Karunakaran, E., Mukherjee, J., Ramalingam, B., and Biggs, C. A. (2011). “Biofilmology”: a multidisciplinary review of the study of microbial biofilms. Appl. Microbiol. Biotechnol. 90, 1869–1881. doi: 10.1007/s00253-011-3293-4
Kashef, N., Akbarizare, M., and Kamrava, S. K. (2013). Effect of sub-lethal photodynamic inactivation on the antibiotic susceptibility and biofilm formation of clinical Staphylococcus aureus isolates. Photodiagnosis Photodyn. Ther. 10, 368–373. doi: 10.1016/j.pdpdt.2013.02.005
Kashef, N., Karami, S., and Djavid, G. E. (2015). Phototoxic effect of hypericin alone and in combination with acetylcysteine on Staphylococcus aureus biofilms. Photodiagn. Photodyn. Ther. 12, 186–192. doi: 10.1016/j.pdpdt.2015.04.001
Ke, M. R., Eastel, J. M., Ngai, K. L., Cheung, Y. Y., Chan, P. K., Hui, M., et al. (2014). Photodynamic inactivation of bacteria and viruses using two monosubstituted zinc(II) phthalocyanines. Eur. J. Med. Chem. 84, 278–283. doi: 10.1016/j.ejmech.2014.07.022
Khan, M. S., Zahin, M., Hasan, S., Husain, F. M., and Ahmad, I. (2009). Inhibition of quorum sensing regulated bacterial functions by plant essential oils with special reference to clove oil. Lett. Appl. Microbiol. 49, 354–360. doi: 10.1111/j.1472-765X.2009.02666.x
Khan, S., Alam, F., Azam, A., and Khan, A. U. (2012). Gold nanoparticles enhance methylene blue-induced photodynamic therapy: a novel therapeutic approach to inhibit Candida albicans biofilm. Int. J. Nanomed. 7, 3245–3257. doi: 10.2147/IJN.S31219
Khemiri, A., Jouenne, T., and Cosette, P. (2016). Proteomics dedicated to biofilmology: What have we learned from a decade of research? Med. Microbiol. Immunol. 205, 1–19. doi: 10.1007/s00430-015-0423-0
Kim, C., Hung, Y. C., Brackett, R. E., and Frank, J. F. (2001). Inactivation of Listeria monocytogenes biofilms by electrolyzed oxidizing water. J. Food Process. Preserv. 25, 91–100. doi: 10.1111/j.1745-4549.2001.tb00446.x
Konopka, K., and Goslinski, T. (2007). Photodynamic therapy in dentistry. J. Dent. Res. 86, 694–707. doi: 10.1177/154405910708600803
Kübler, A. C. (2005). Photodynamic therapy. Med. Laser Appl. 20, 37–45. doi: 10.1016/j.mla.2005.02.001
Lakretz, A., Ron, E. Z., Harif, T., and Mamane, H. (2011). Biofilm control in water by advanced oxidation process (AOP) pre-treatment: effect of natural organic matter (NOM). Water Sci. Technol. 64, 1876–1884. doi: 10.2166/wst.2011.077
Lam, M., Jou, P. C., Lattif, A. A., Lee, Y., Malbasa, C. L., Mukherjee, P. K., et al. (2011). Photodynamic therapy with Pc 4 induces apoptosis of Candida albicans. Photochem. Photobiol. 87, 904–909. doi: 10.1111/j.1751-1097.2011.00938.x
Lambrechts, S. A., Demidova, T. N., Aalders, M. C., Hasan, T., and Hamblin, M. R. (2005). Photodynamic therapy for Staphylococcus aureus infected burn wounds in mice. Photochem. Photobiol. Sci. 4, 503–509. doi: 10.1039/b502125a
Lamppa, J. W., and Griswold, K. E. (2013). Alginate lyase exhibits catalysis-independent biofilm dispersion and antibiotic synergy. Antimicrob. Agent Chemother. 57, 137–145. doi: 10.1128/AAC.01789-12
Leaper, D., Assadian, O., and Edmiston, C. E. (2015). Approach to chronic wound infections. Br. J. Dermatol. 173, 351–358. doi: 10.1111/bjd.13677
Leive, L. (1974). The barrier function of the gram-negative envelope. Ann. N. Y. Acad. Sci. 235, 109–129. doi: 10.1111/j.1749-6632.1974.tb43261.x
Lembo, A. J., Ganz, R. A., Sheth, S., Cave, D., Kelly, C., Levin, P., et al. (2009). Treatment of Helicobacter pylori infection with intra-gastric violet light phototherapy: a pilot clinical trial. Lasers Surg. Med. 41, 337–344. doi: 10.1002/lsm.20770
Li, H., Wang, D., Sun, X., Hu, L., Yu, H., and Wang, J. (2012). Relationship between bacterial biofilm and clinical features of patients with chronic rhinosinusitis. Eur. Arch. Otorhinolaryngol. 269, 155–163. doi: 10.1007/s00405-011-1683-y
Li, X., Guo, H., Tian, Q., Zheng, G., Hu, Y., Fu, Y., et al. (2013). Effects of 5-aminolevulinic acid-mediated photodynamic therapy on antibiotic-resistant staphylococcal biofilm: an in vitro study. J. Surg. Res. 184, 1013–1021. doi: 10.1016/j.jss.2013.03.094
Lieberman, O. J., Orr, M. W., Wang, Y., and Lee, V. T. (2014). High-throughput screening using the differential radial capillary action of ligand assay identifies ebselen as an inhibitor of diguanylate cyclases. ACS Chem. Biol. 9, 183–192. doi: 10.1021/cb400485k
Lin, J., Bi, L. J., Zhang, Z. G., Fu, Y. M., and Dong, T. T. (2010). Toluidine blue-mediated photodynamic therapy of oral wound infections in rats. Lasers Med. Sci. 25, 233–238 doi: 10.1007/s10103-009-0700-5
Linares, J. F., Gustafsson, I., Baquero, F., and Martinez, J. L. (2006). Antibiotics as intermicrobial signaling agents instead of weapons. Proc. Natl. Acad. Sci. U.S.A. 103, 19484–19489. doi: 10.1073/pnas.0608949103
Liu, H., Du, Y., Wang, X., and Sun, L. (2004). Chitosan kills bacteria through cell membrane damage. Int. J. Food Microbiol. 95, 147–155. doi: 10.1016/j.ijfoodmicro.2004.01.022
Lopes, D., Melo, T., Santos, N., Rosa, L., Alves, E., Clara Gomes, M., et al. (2014). Evaluation of the interplay among the charge of porphyrinic photosensitizers, lipid oxidation and photoinactivation efficiency in Escherichia coli. J. Photochem. Photobiol. B 141, 145–153. doi: 10.1016/j.jphotobiol.2014.08.024
Louvado, A, Coelho, F. J., Domingues, P., Santos, A. L., Gomes, N. C., Almeida, A., et al. (2012). Isolation of surfactant-resistant Pseudomonads from the estuarine surface microlayer. J. Microbiol. Biotechnol. 22, 283–291. doi: 10.4014/jmb.1110.10041
Lopes, M., Alves, C. T., Rama Raju, B., Gonçalves, M. S., Coutinho, P. J., Henriques, M., et al. (2014). Application of benzo[a]phenoxazinium chlorides in antimicrobial photodynamic therapy of Candida albicans biofilms. J. Photochem. Photobiol. B 141, 93–99. doi: 10.1016/j.jphotobiol.2014.09.006
López-Jimenez, L., Fusté, E., Martínez-Garriga, B., Arnabat-Domínguez, J., Vinuesa, T., and Viñas, M. (2015). Effects of photodynamic therapy on Enterococcus faecalis biofilms. Lasers Med. Sci. 30, 1–8. doi: 10.1007/s10103-015-1749-y
LoVetri, K., and Madhyastha, S. (2010). Antimicrobial and antibiofilm activity of quorum sensing peptides and Peptide analogues against oral biofilm bacteria. Methods Mol. Biol. 618, 383–392. doi: 10.1007/978-1-60761-594-1_24
Luke-Marshall, N. R., Mang, T. S., Hansen, L. A., and Campagnari, A. A. (2014). Moraxella catarrhalis is susceptible to antimicrobial photodynamic therapy with Photofrin. Lasers Surg. Med. 46, 712–717. doi: 10.1002/lsm.22287
Mang, T. S., Tayal, D. P., and Baier, R. (2012). Photodynamic therapy as an alternative treatment for disinfection of bacteria in oral biofilms. Lasers Surg. Med. 44, 588–596. doi: 10.1002/lsm.22050
Mannucci, E., Genovese, S., Monami, M., Navalesi, G., Dotta, F., Anichini, R., et al. (2014). Photodynamic topical antimicrobial therapy for infected foot ulcers in patients with diabetes: a randomized, double-blind, placebo-controlled study—the D.A.N.T.E (Diabetic ulcer Antimicrobial New Topical treatment Evaluation) study. Acta Diabetol. 51, 435–440. doi: 10.1007/s00592-013-0533-3
Manoil, D., Filieri, A., Gameiro, C., Lange, N., Schrenzel, J., Wataha, J. C., et al. (2014). Flow cytometric assessment of Streptococcus mutans viability after exposure to blue light-activated curcumin. Photodiagn. Photodyn. Ther. 11, 372–379. doi: 10.1016/j.pdpdt.2014.06.003
Melo, T., Santos, N., Lopes, D., Alves, E., Maciel, E., Faustino, M. A., et al. (2013). Photosensitized oxidation of phosphatidylethanolamines monitored by electrospray tandem mass spectrometry. J. Mass Spectrom. 48, 1357–1365. doi: 10.1002/jms.3301
Merchat, M., Bertolini, G., Giacomini, P., Villanueva, A., and Jori, G. (1996). Meso-substituted cationic porphyrins as efficient photosensitizers of gram-positive and gram-negative bacteria. J. Photochem. Photobiol. B 32, 153–157. doi: 10.1016/1011-1344(95)07147-4
Mileykovskaya, E., and Dowhan, W. (2000). Visualization of phospholipid domains in Escherichia coli by using the cardiolipin-specific fluorescent dye 10-N-nonyl acridine orange. J. Bacteriol. 182, 1172–1175. doi: 10.1128/JB.182.4.1172-1175.2000
Miller, M. B., and Bassler, B. L. (2001). Quorum sensing in bacteria. Annu. Rev. Microbiol. 55, 165–199. doi: 10.1146/annurev.micro.55.1.165
Mima, E. G., Pavarina, A. C., Dovigo, L. N., Vergani, C. E., Costa, C. A., Kurachi, C., et al. (2010). Susceptibility of Candida albicans to photodynamic therapy in a murine model of oral candidosis. Oral Surg. Oral Med. Oral Pathol. Oral Radiol. Endod. 109, 392–401. doi: 10.1016/j.tripleo.2009.10.006
Minnock, A., Vernon, D. I., Schofield, J., Griffiths, J., Parish, J. H., and Brown, S. B. (2000). Mechanism of uptake of a cationic water-soluble pyridinium zinc phthalocyanine across the outer membrane of Escherichia coli. Antimicrob. Agents Chemother. 44, 522–527. doi: 10.1128/AAC.44.3.522-527.2000
Miyata, S., Miyaji, H., Kawasaki, H., Yamamoto, M., Nishida, E., Takita, H., et al. (2017). Antimicrobial photodynamic activity and cytocompatibility of Au25(Capt)18 clusters photoexcited by blue LED light irradiation. Int. J. Nanomed. 12, 2703–2716. doi: 10.2147/IJN.S131602
Molin, S., and Tolker-Nielsen, T. (2003). Gene transfer occurs with enhanced efficiency in biofilms and induces enhanced stabilisation of the biofilm structure. Curr. Opin. Biotechnol. 14, 255–261. doi: 10.1016/S0958-1669(03)00036-3
Morimoto, K., Ozawa, T., Awazu, K., Ito, N., Honda, N., Matsumoto, S., et al. (2014). Photodynamic therapy using systemic administration of 5-aminolevulinic acid and a 410-nm wavelength light-emitting diode for methicillin-resistant Staphylococcus aureus-infected ulcers in mice. PLoS ONE 9:e105173. doi: 10.1371/journal.pone.0105173
Morley, S., Griffiths, J., Philips, G., Moseley, H., O'Grady, C., Mellish, K., et al. (2013). Phase IIa randomized, placebo-controlled study of antimicrobial photodynamic therapy in bacterially colonized, chronic leg ulcers and diabetic foot ulcers: a new approach to antimicrobial therapy. Br. J. Dermatol. 168, 617–624. doi: 10.1111/bjd.12098
Muhammad, O. H., Chevalier, M., Rocca, J. P., Brulat-Bouchard, N., and Medioni, E. (2014). Photodynamic therapy versus ultrasonic irrigation: interaction with endodontic microbial biofilm, an ex vivo study. Photodiagn. Photodyn. Ther. 11, 171–181. doi: 10.1016/j.pdpdt.2014.02.005
Mun, S., Jeong, J. S., Kim, J., Lee, Y. W., and Yoon, J. (2009). Inactivation of Pseudomonas aeruginosa biofilm by dense phase carbon dioxide. Biofouling 25, 473–479. doi: 10.1080/08927010902874876
Nafee, N., Youssef, A., El-Gowelli, H., Asem, H., and Kandil, S. (2013). Antibiotic-free nanotherapeutics: hypericin nanoparticles thereof for improved in vitro and in vivo antimicrobial photodynamic therapy and wound healing. Int. J. Pharm. 454, 249–258. doi: 10.1016/j.ijpharm.2013.06.067
Nett, J. E., Sanchez, H., Cain, M. T., and Andes, D. R. (2010). Genetic basis of Candida biofilm resistance due to drug-sequestering matrix glucan. J. Infect. Dis. 202, 171–175. doi: 10.1086/651200
Neu, H. C. (1992). The crisis in antibiotic resistance. Science 257, 1064–1073. doi: 10.1126/science.257.5073.1064
Neu, T. R., Manz, B., Volke, F., Dynes, J. J., Hitchcock, A. P., and Lawrence, J. R. (2010). Advanced imaging techniques for assessment of structure, composition and function in biofilm systems. FEMS Microbiol. Ecol. 72, 1–21. doi: 10.1111/j.1574-6941.2010.00837.x
Nicholson, T. L., Shore, S. M., Smith, T. C., and Frana, T. S. (2013). Livestock-associated methicillin-resistant Staphylococcus aureus (LA-MRSA) isolates of swine origin form robust biofilms. PLoS ONE 8:e73376. doi: 10.1371/journal.pone.0073376
Nitzan, Y., Dror, R., Ladan, H., Malik, Z., Kimel, S., and Gottfried, V. (1995). Structure-activity relationship of porphines for photoinactivation of bacteria. Photochem. Photobiol. 62, 342–347. doi: 10.1111/j.1751-1097.1995.tb05279.x
Nitzan, Y., Gutterman, M., Malik, Z., and Ehrenberg, B. (1992). Inactivation of gram-negative bacteria by photosensitized porphyrins. Photochem. Photobiol. 55, 89–96. doi: 10.1111/j.1751-1097.1992.tb04213.x
Niu, C., Afre, S., and Gilbert, E. S. (2006). Subinhibitory concentrations of cinnamaldehyde interfere with quorum sensing. Lett. Appl. Microbiol. 43, 489–494. doi: 10.1111/j.1472-765X.2006.02001.x
Nunes, M. R., Mello, I., Franco, G. C., de Medeiros, J. M., Dos Santos, S. S., Habitante, S. M., et al. (2011). Effectiveness of photodynamic therapy against Enterococcus faecalis, with and without the use of an intracanal optical fiber: an in vitro study. Photomed. Laser Surg. 29, 803–808. doi: 10.1089/pho.2011.2995
Okshevsky, M., Regina, V. R., and Meyer, R. L. (2015). Extracellular DNA as a target for biofilm control. Curr. Opin. Biotechnol. 33, 73–80. doi: 10.1016/j.copbio.2014.12.002
Oliver, P. M., Crooks, J. A., Leidl, M., Yoon, E. J., Saghatelian, A., and Weibel, D. B. (2014). Localization of anionic phospholipids in Escherichia coli cells. J. Bacteriol. 196, 3386–3398 doi: 10.1128/JB.01877-14
O'Loughlin, C. T., Miller, L. C., Siryaporn, A., Drescher, K., Semmelhack, M. F., and Bassler, B. L. (2013). A quorum-sensing inhibitor blocks Pseudomonas aeruginosa virulence and biofilm formation. Proc. Natl. Acad. Sci. U.S.A. 110, 17981–17986. doi: 10.1073/pnas.1316981110
O'Riordan, K., Akilov, O. E., Chang, S. K., Foley, J. W., and Hasan, T. (2007). Real-time fluorescence monitoring of phenothiazinium photosensitizers and their anti-mycobacterial photodynamic activity against Mycobacterium bovis BCG in in vitro and in vivo models of localized infection. Photochem. Photobiol. Sci. 6, 1117–1123. doi: 10.1039/b707962a
O'Riordan, K., Sharlin, D. S., Gross, J., Chang, S., Errabelli, D., Akilov, O. E., et al. (2006). Photoinactivation of Mycobacteria in vitro and in a new murine model of localized Mycobacterium bovis BCG-induced granulomatous infection. Antimicrob. Agent Chemother. 50, 1828–1834. doi: 10.1128/AAC.50.5.1828-1834.2006
Orlandi, V. T., Rybtke, M., Caruso, E., Banfi, S., Tolker-Nielsen, T., and Barbieri, P. (2015). Antimicrobial and anti-biofilm effect of a novel BODIPY photosensitizer against Pseudomonas aeruginosa PAO1. Biofouling 30, 883–891. doi: 10.1080/08927014.2014.940921
Packiavathy, I. A., Priya, S., Pandian, S. K., and Ravi, A. V. (2014). Inhibition of biofilm development of uropathogens by curcumin - an anti-quorum sensing agent from Curcuma longa. Food Chem. 148, 453–460. doi: 10.1016/j.foodchem.2012.08.002
Park, J. H., Ahn, M. Y., Kim, Y. C., Kim, S. A., Moon, Y. H., Ahn, S. G., et al. (2012). In vitro and in vivo antimicrobial effect of photodynamic therapy using a highly pure chlorin e6 against Staphylococcus aureus Xen29. Biol. Pharm. Bull. 35, 509–514. doi: 10.1248/bpb.35.509
Pedrazzi, V., Escobar, E. C., Cortelli, J. R., Haas, A. N., Andrade, A. K., Pannuti, C. M., et al. (2014). Antimicrobial mouthrinse use as an adjunct method in peri-implant biofilm control. Braz. Oral Res. 28, 1–9. doi: 10.1590/1807-3107BOR-2014.vol28.0022
Pei, X., Lu, X., Liu, J., Liu, D., Yang, Y., Ostrikov, K., et al. (2012). Inactivation of a 25.5 lm Enterococcus faecalis biofilm by a room-temperature, battery-operated, handheld air plasma jet. J. Phys. D Appl. Phys. 45:165205. doi: 10.1088/0022-3727/45/16/165205
Pereira, C. A., Costa, A. C., Carreira, C. M., Junqueira, J. C., and Jorge, A. O. (2013). Photodynamic inactivation of Streptococcus mutans and Streptococcus sanguinis biofilms in vitro. Lasers Med. Sci. 28, 859–864. doi: 10.1007/s10103-012-1175-3
Pereira-Cenci, T., Deng, D. M., Kraneveld, E. A., Manders, E. M., Del Bel Cury, A. A., Ten Cate, J. M., et al. (2008). The effect of Streptococcus mutans and Candida glabrata on Candida albicans biofilms formed on different surfaces. Arch. Oral Biol. 53, 755–764. doi: 10.1016/j.archoralbio.2008.02.015
Pinheiro, S. L., Schenka, A. A., Neto, A. A., de Souza, C. P., Rodriguez, H. M., and Ribeiro, M. C. (2009). Photodynamic therapy in endodontic treatment of deciduous teeth. Lasers Med. Sci. 24, 521–526. doi: 10.1007/s10103-008-0562-2
Prasanth, C. S., Karunakaran, S. C., Paul, A. K., Kussovski, V., Mantareva, V., Ramaiah, D., et al. (2014). Antimicrobial photodynamic efficiency of novel cationic porphyrins towards periodontal Gram-positive and Gram-negative pathogenic bacteria. Photochem. Photobiol. 90, 628–640. doi: 10.1111/php.12198
Quishida, C. C., Carmello, J. C., Mima, E. G., Bagnato, V. S., Machado, A. L., and Pavarina, A. C. (2015). Susceptibility of multispecies biofilm to photodynamic therapy using Photodithazine(R). Lasers Med. Sci. 30, 685–694. doi: 10.1007/s10103-013-1397-z
Rabea, E. I., Badawy, M. E., Stevens, C. V., Smagghe, G., and Steurbaut, W. (2003). Chitosan as antimicrobial agent: applications and mode of action. Biomacromolecules 4, 1457–1465. doi: 10.1021/bm034130m
Renzi, F., Zähringer, U., Chandler, C. E., Ernst, R. K., Cornelis, G. R., and Ittig, S. J. (2015). Modification of the 1-phosphate group during biosynthesis of Capnocytophaga canimorsus lipid A. Infect. Immun. 84, 550–561. doi: 10.1128/IAI.01006-15
Ribeiro, A. P., Andrade, M. C., Bagnato, V. S., Vergani, C. E., Primo, F. L., Tedesco, A. C., et al. (2015). Antimicrobial photodynamic therapy against pathogenic bacterial suspensions and biofilms using chloro-aluminum phthalocyanine encapsulated in nanoemulsions. Lasers Med. Sci. 30, 549–559. doi: 10.1007/s10103-013-1354-x
Ribeiro, A. P., Andrade, M. C., da Silva Jde, F., Jorge, J. H., Primo, F. L., Tedesco, A. C., et al. (2013). Photodynamic inactivation of planktonic cultures and biofilms of Candida albicans mediated by aluminum-chloride-phthalocyanine entrapped in nanoemulsions. Photochem. Photobiol. 89, 111–119. doi: 10.1111/j.1751-1097.2012.01198.x
Rios, A., He, J., Glickman, G. N., Spears, R., Schneiderman, E. D., and Honeyman, A. L. (2011). Evaluation of photodynamic therapy using a light-emitting diode lamp against Enterococcus faecalis in extracted human teeth. J. Endod. 37, 856–859. doi: 10.1016/j.joen.2011.03.014
Römling, U., Galperin, M. Y., and Gomelsky, M. (2013). Cyclic di-GMP: the first 25 years of a universal bacterial second messenger. Microbiol. Mol. Biol. Rev. 77, 1–52. doi: 10.1128/MMBR.00043-12
Rosa, L. P., da Silva, F. C., Nader, S. A., Meira, G. A., and Viana, M. S. (2015a). Antimicrobial photodynamic inactivation of Staphylococcus aureus biofilms in bone specimens using methylene blue, toluidine blue ortho and malachite green: an in vitro study. Arch. Oral Biol. 60, 675–680. doi: 10.1016/j.archoralbio.2015.02.010
Rosa, L. P., da Silva, F. C., Nader, S. A., Meira, G. A., and Viana, M. S. (2014). In vitro effectiveness of antimicrobial photodynamic therapy (APDT) using a 660 nm laser and malachite green dye in Staphylococcus aureus biofilms arranged on compact and cancellous bone specimens. Lasers Med. Sci. 29, 1959–1965. doi: 10.1007/s10103-014-1613-5
Rosa, L. P., Silva, F. C., Nader, S. A., Meira, G. A., and Viana, M. S. (2015b). Effectiveness of antimicrobial photodynamic therapy using a 660nm laser and methyline blue dye for inactivating Staphylococcus aureus biofilms in compact and cancellous bones: an in vitro study. Photodiagn. Photodyn. Ther. 12, 276–281. doi: 10.1016/j.pdpdt.2015.01.001
Rosseti, I. B., Chagas, L. R., and Costa, M. S. (2014). Photodynamic antimicrobial chemotherapy (PACT) inhibits biofilm formation by Candida albicans, increasing both ROS production and membrane permeability. Lasers Med. Sci. 29, 1059–1064. doi: 10.1007/s10103-013-1473-4
Rossoni, R. D., Barbosa, J. O., de Oliveira, F. E., de Oliveira, L. D., Jorge, A. O., and Junqueira, J. C. (2014). Biofilms of Candida albicans serotypes A and B differ in their sensitivity to photodynamic therapy. Lasers Med. Sci. 29, 1679–1684. doi: 10.1007/s10103-014-1570-z
Sabino, C. P., Garcez, A. S., Núñez, S. C., Ribeiro, M. S., and Hamblin, M. R. (2014). Real-time evaluation of two light delivery systems for photodynamic disinfection of Candida albicans biofilm in curved root canals. Lasers Med. Sci. 30, 1657–1665. doi: 10.1007/s10103-014-1629-x
Sarkar, R., Mondal, C., Bera, R., Chakraborty, S., Barik, R., Roy, P., et al. (2015). Antimicrobial properties of Kalanchoe blossfeldiana: a focus on drug resistance with particular reference to quorum sensing-mediated bacterial biofilm formation. J. Pharm. Pharmacol. 67, 951–962. doi: 10.1111/jphp.12397
Schneider, C., Porter, N. A., and Brash, A. R. (2008). Routes to 4-hydroxynonenal: fundamental issues in the mechanisms of lipid peroxidation. J. Biol. Chem. 283, 15539–15543. doi: 10.1074/jbc.R800001200
Seneviratne, C. J., Jin, L., and Samaranayake, L. P. (2008). Biofilm lifestyle of Candida: a mini review. Oral Dis. 14, 582–590. doi: 10.1111/j.1601-0825.2007.01424.x
Seth, A. K., Geringer, M. R., Nguyen, K. T., Agnew, S. P., Dumanian, Z., Galiano, R. D., et al. (2013). Bacteriophage therapy for Staphylococcus aureus biofilm-infected wounds: a new approach to chronic wound care. Plast Reconstr. Surg. 131, 225–234. doi: 10.1097/PRS.0b013e31827e47cd
Shrestha, A., and Kishen, A. (2012). Polycationic chitosan-conjugated photosensitizer for antibacterial photodynamic therapy. Photochem. Photobiol. 88, 577–583. doi: 10.1111/j.1751-1097.2011.01026.x
Shrestha, A., and Kishen, A. (2014). Antibiofilm efficacy of photosensitizer-functionalized bioactive nanoparticles on multispecies biofilm. J. Endod. 40, 1604–1610. doi: 10.1016/j.joen.2014.03.009
Shrestha, A., Hamblin, M. R., and Kishen, A. (2014). Photoactivated rose bengal functionalized chitosan nanoparticles produce antibacterial/biofilm activity and stabilize dentin-collagen. Nanomedicine 10, 491–501. doi: 10.1016/j.nano.2013.10.010
Shrestha, A., Shi, Z., Neoh, K. G., and Kishen, A. (2010). Nanoparticulates for antibiofilm treatment and effect of aging on its antibacterial activity. J. Endod. 36, 1030–1035. doi: 10.1016/j.joen.2010.02.008
Sia, I. G., Berbari, E. F., and Karchmer, A. W. (2005). Prosthetic joint infections. Infect. Dis. Clin. North Am. 19, 885–914. doi: 10.1016/j.idc.2005.07.010
Siddiqui, A. R., and Bernstein, J. M. (2010). Chronic wound infection: facts and controversies. Clin. Dermatol. 28, 519–526. doi: 10.1016/j.clindermatol.2010.03.009
Sigusch, B. W., Engelbrecht, M., Völpel, A., Holletschke, A., Pfister, W., and Schütze, J. (2010). Full-mouth antimicrobial photodynamic therapy in Fusobacterium nucleatum-infected periodontitis patients. J. Periodontol. 81, 975–981. doi: 10.1902/jop.2010.090246
Silva, T. C., Pereira, A. F., Buzalaf, M. A., Machado, M. A., Crielaard, W., and Deng, D. M. (2014). Diverse outcomes of photodynamic antimicrobial chemotherapy on five Enterococcus faecalis strains. Photodiagn. Photodyn. Ther. 11, 283–289. doi: 10.1016/j.pdpdt.2014.04.003
Singh, R., Sahore, S., Kaur, P., Rani, A., and Ray, P. (2016). Penetration barrier contributes to bacterial biofilm-associated resistance against only select antibiotics, and exhibits genus-, strain- and antibiotic-specific differences. Pathog. Dis. 74, 1–6. doi: 10.1093/femspd/ftw056
Skillman, L. C., Sutherland, I. W., and Jones, M. V. (1998). The role of exopolysaccharides in dual species biofilm development. J. Appl. Microbiol. 85(Suppl. 1), 13S−18S. doi: 10.1111/j.1365-2672.1998.tb05278.x
Smith, K. M., Bu, Y., and Suga, H. (2003). Library screening for synthetic agonists and antagonists of a Pseudomonas aeruginosa autoinducer. Chem. Biol. 10, 563–571. doi: 10.1016/S1074-5521(03)00107-8
Soothill, J. (2013). Use of bacteriophages in the treatment of Pseudomonas aeruginosa infections. Expert Rev. Anti Infect. Ther. 11, 909–915. doi: 10.1586/14787210.2013.826990
Soukos, N. S., Ximenez-Fyvie, L. A., Hamblin, M. R., Socransky, S. S., and Hasan, T. (1998). Targeted antimicrobial photochemotherapy. Antimicrob. Agent Chemother. 42, 2595–2601.
Stewart, P. S. (2003). New ways to stop biofilm infections. Lancet 361:97. doi: 10.1016/S0140-6736(03)12245-3
Stowe, S. D., Richards, J. J., Tucker, A. T., Thompson, R., Melander, C., and Cavanagh, J. (2011). Anti-biofilm compounds derived from marine sponges. Mar. Drugs 9, 2010–2035. doi: 10.3390/md9102010
Street, C. N., Pedigo, L., Gibbs, A., and Loebel, N. G. (2009). Antimicrobial photodynamic therapy for the decolonization of methicillin-resistant Staphylococcus aureus from the anterior nares. Proc. SPIE 7380, 73803B-73801–73803B-73816. doi: 10.1117/12.828279
Takahashi, N., and Nyvad, B. (2011). The role of bacteria in the caries process: ecological perspectives. J. Dent. Res. 90, 294–303. doi: 10.1177/0022034510379602
Takasaki, A. A., Aoki, A., Mizutani, K., Schwarz, F., Sculean, A., Wang, C. Y., et al. (2009). Application of antimicrobial photodynamic therapy in periodontal and peri-implant diseases. Periodontol. 2000 51, 109–140. doi: 10.1111/j.1600-0757.2009.00302.x
Tavares, A., Carvalho, C. M., Faustino, M. A., Neves, M. G., Tomé, J. P., Tomé, A. C., et al. (2010). Antimicrobial photodynamic therapy: study of bacterial recovery viability and potential development of resistance after treatment. Mar. Drugs 8, 91–105. doi: 10.3390/md8010091
Tegos, G. P., Anbe, M., Yang, C., Demidova, T. N., Satti, M., Mroz, P., et al. (2006). Protease-stable polycationic photosensitizer conjugates between polyethyleneimine and chlorin(e6) for broad-spectrum antimicrobial photoinactivation. Antimicrob. Agent Chemother. 50, 1402–1410. doi: 10.1128/AAC.50.4.1402-1410.2006
Tegos, G. P., and Hamblin, M. R. (2006). Phenothiazinium antimicrobial photosensitizers are substrates of bacterial multidrug resistance pumps. Antimicrob. Agent Chemother. 50, 196–203. doi: 10.1128/AAC.50.1.196-203.2006
Teichert, M. C., Jones, J. W., Usacheva, M. N., and Biel, M. A. (2002). Treatment of oral candidiasis with methylene blue-mediated photodynamic therapy in an immunodeficient murine model. Oral Surg. Oral Med. Oral Pathol. Oral Radiol. Endod. 93, 155–160. doi: 10.1067/moe.2002.120051
Tennert, C., Feldmann, K., Haamann, E., Al-Ahmad, A., Follo, M., Wrbas, K. T., et al. (2014). Effect of photodynamic therapy (PDT) on Enterococcus faecalis biofilm in experimental primary and secondary endodontic infections. BMC Oral Health 14:132. doi: 10.1186/1472-6831-14-132
Traba, C., and Liang, J. F. (2011). Susceptibility of Staphylococcus aureus biofilms to reactive discharge gases. Biofouling 27, 763–772. doi: 10.1080/08927014.2011.602188
Tschowri, N., Busse, S., and Hengge, R. (2009). The BLUF-EAL protein YcgF acts as a direct anti-repressor in a blue-light response of Escherichia coli. Genes Dev. 23, 522–534. doi: 10.1101/gad.499409
Upadya, M. H., and Kishen, A. (2010). Influence of bacterial growth modes on the susceptibility to light-activated disinfection. Int. Endod. J. 43, 978–987. doi: 10.1111/j.1365-2591.2010.01717.x
van Alen, T., Claus, H., Zahedi, R. P., Groh, J., Blazyca, H., Lappann, M., et al. (2010). Comparative proteomic analysis of biofilm and planktonic cells of Neisseria meningitidis. Proteomics 10, 4512–4521. doi: 10.1002/pmic.201000267
van Houte, J., Lopman, J., and Kent, R. (1996). The final pH of bacteria comprising the predominant flora on sound and carious human root and enamel surfaces. J. Dent. Res. 75, 1008–1014. doi: 10.1177/00220345960750040201
van Ruyven, F. O., Lingström, P., van Houte, J., and Kent, R. (2000). Relationship among mutans streptococci, “low-pH” bacteria, and lodophilic polysaccharide-producing bacteria in dental plaque and early enamel caries in humans. J. Dent. Res. 79, 778–784. doi: 10.1177/00220345000790021201
Vassena, C., Fenu, S., Giuliani, F., Fantetti, L., Roncucci, G., Simonutti, G., et al. (2014). Photodynamic antibacterial and antibiofilm activity of RLP068/Cl against Staphylococcus aureus and Pseudomonas aeruginosa forming biofilms on prosthetic material. Int. J. Antimicrob. Agents 44, 47–55. doi: 10.1016/j.ijantimicag.2014.03.012
Vatansever, F., de Melo, W. C., Avci, P., Vecchio, D., Sadasivam, M., Gupta, A., et al. (2013). Antimicrobial strategies centered around reactive oxygen species–bactericidal antibiotics, photodynamic therapy, and beyond. FEMS Microbiol. Rev. 37, 955–989. doi: 10.1111/1574-6976.12026
Vaziri, S., Kangarlou, A., Shahbazi, R., Nazari Nasab, A., and Naseri, M. (2012). Comparison of the bactericidal efficacy of photodynamic therapy, 2.5% sodium hypochlorite, and 2% chlorhexidine against Enterococcous faecalis in root canals; an in vitro study. Dent. Res. J. 9, 613–618. doi: 10.4103/1735-3327.104882
Vickery, K., Deva, A., Jacombs, A., Allan, J., Valente, P., and Gosbell, I. B. (2012). Presence of biofilm containing viable multiresistant organisms despite terminal cleaning on clinical surfaces in an intensive care unit. J. Hosp. Infect. 80, 52–55. doi: 10.1016/j.jhin.2011.07.007
Voos, A. C., Kranz, S., Tonndorf-Martini, S., Voelpel, A., Sigusch, H., Staudte, H., et al. (2014). Photodynamic antimicrobial effect of safranine O on an ex vivo periodontal biofilm. Lasers Surg. Med. 46, 235–243. doi: 10.1002/lsm.22217
Vrouenraets, M. B., Visser, G. W., Snow, G. B., and van Dongen, G. A. (2003). Basic principles, applications in oncology and improved selectivity of photodynamic therapy. Anticancer Res. 23, 505–522.
Wang, L., Hu, X., Tao, G., and Wang, X. (2012). Outer membrane defect and stronger biofilm formation caused by inactivation of a gene encoding for heptosyltransferase I in Cronobacter sakazakii ATCC BAA-894. J. Appl. Microbiol. 112, 985–997. doi: 10.1111/j.1365-2672.2012.05263.x
Wang, Y., Zhou, Q., Wang, Y., Ren, J., Zhao, H., Wu, S., et al. (2015). In vitro photodynamic inactivation effects of Ru(II) complexes on clinical methicillin-resistant Staphylococcus aureus planktonic and biofilm cultures. Photochem. Photobiol. 91, 124–133. doi: 10.1111/php.12378
Wilder-Smith, C. H., Wilder-Smith, P., Grosjean, P., van den Bergh, H., Woodtli, A., Monnier, P., et al. (2002). Photoeradication of Helicobacter pylori using 5-aminolevulinic acid: preliminary human studies. Lasers Surg. Med. 31, 18–22. doi: 10.1002/lsm.10066
Wolcott, R. D., and Ehrlich, G. D. (2008). Biofilms and chronic infections. JAMA 299, 2682–2684. doi: 10.1001/jama.299.22.2682
Wong, T. W., Wang, Y. Y., Sheu, H. M., and Chuang, Y. C. (2005). Bactericidal effects of toluidine blue-mediated photodynamic action on Vibrio vulnificus. Antimicrob. Agents Chemother. 49, 895–902. doi: 10.1128/AAC.49.3.895-902.2005
Wood, A. J., Fraser, J., Swift, S., Amirapu, S., and Douglas, R. G. (2011). Are biofilms associated with an inflammatory response in chronic rhinosinusitis? Int. Forum Allergy Rhinol. 1, 335–339. doi: 10.1002/alr.20060
Wood, S., Metcalf, D., Devine, D., and Robinson, C. (2006). Erythrosine is a potential photosensitizer for the photodynamic therapy of oral plaque biofilms. J. Antimicrob. Chemother. 57, 680–684. doi: 10.1093/jac/dkl021
Wright, C. J., Wu, H., Melander, R. J., Melander, C., and Lamont, R. J. (2014). Disruption of heterotypic community development by Porphyromonas gingivalis with small molecule inhibitors. Mol. Oral Microbiol. 29, 185–193. doi: 10.1111/omi.12060
Wu, H., Song, Z., Hentzer, M., Andersen, J. B., Molin, S., Givskov, M., et al. (2004). Synthetic furanones inhibit quorum-sensing and enhance bacterial clearance in Pseudomonas aeruginosa lung infection in mice. J. Antimicrob. Chemother. 53, 1054–1061. doi: 10.1093/jac/dkh223
Xie, X., Mao, C., Liu, X., Zhang, Y., Cui, Z., Yang, X., et al. (2017). Synergistic bacteria killing through photodynamic and physical actions of graphene oxide/Ag/collagen coating. ACS Appl. Mater. Interfaces 9, 26417–26428. doi: 10.1021/acsami.7b06702
Yildirim, C., Karaarslan, E. S., Ozsevik, S., Zer, Y., Sari, T., and Usumez, A. (2013). Antimicrobial efficiency of photodynamic therapy with different irradiation durations. Eur. J. Dent. 7, 469–473. doi: 10.4103/1305-7456.120677
Yilmaz, C., Colak, M., Yilmaz, B. C., Ersoz, G., Kutateladze, M., and Gozlugol, M. (2013). Bacteriophage therapy in implant-related infections: an experimental study. J. Bone Joint Surg. Am. 95, 117–125. doi: 10.2106/JBJS.K.01135
Zand, V., Milani, A. S., Amini, M., Barhaghi, M. H., Lotfi, M., Rikhtegaran, S., et al. (2014). Antimicrobial efficacy of photodynamic therapy and sodium hypochlorite on monoculture biofilms of Enterococcus faecalis at different stages of development. Photomed. Laser Surg. 32, 245–251. doi: 10.1089/pho.2013.3557
Zaura, E., Keijser, B. J., Huse, S. M., and Crielaard, W. (2009). Defining the healthy “core microbiome” of oral microbial communities. BMC Microbiol. 9:259. doi: 10.1186/1471-2180-9-259
Zhou, Z., Peng, S., Sui, M., Chen, S., Huang, L., Xu, H., et al. (2017). Multifunctional nanocomplex for surface-enhanced Raman scattering imaging and near-infrared photodynamic antimicrobial therapy of vancomycin-resistant bacteria. Colloids Surf. B Biointerfaces 161, 394–402. doi: 10.1016/j.colsurfb.2017.11.005
Zimmerli, W., Trampuz, A., and Ochsner, P. E. (2004). Prosthetic-joint infections. N. Engl. J. Med. 351, 1645–1654. doi: 10.1056/NEJMra040181
Keywords: photodynamic therapy, microbial biofilms, photosensitizer structure, biofilm-related infections, photochemical mechanisms, reactive oxygen species
Citation: Hu X, Huang Y-Y, Wang Y, Wang X and Hamblin MR (2018) Antimicrobial Photodynamic Therapy to Control Clinically Relevant Biofilm Infections. Front. Microbiol. 9:1299. doi: 10.3389/fmicb.2018.01299
Received: 22 March 2018; Accepted: 28 May 2018;
Published: 27 June 2018.
Edited by:
Fabian Cieplik, University of Regensburg, GermanyReviewed by:
Mariusz Stanislaw Grinholc, Intercollegiate Faculty of Biotechnology of University of Gdansk and Medical University of Gdansk, PolandEliana Alves, University of Aveiro, Portugal
Ali Al-Ahmad, Universitätsklinikum Freiburg, Germany
Copyright © 2018 Hu, Huang, Wang, Wang and Hamblin. This is an open-access article distributed under the terms of the Creative Commons Attribution License (CC BY). The use, distribution or reproduction in other forums is permitted, provided the original author(s) and the copyright owner are credited and that the original publication in this journal is cited, in accordance with accepted academic practice. No use, distribution or reproduction is permitted which does not comply with these terms.
*Correspondence: Michael R. Hamblin, aGFtYmxpbkBoZWxpeC5tZ2guaGFydmFyZC5lZHU=