- 1Faculty of Science, University of South Bohemia, Ceske Budejovice, Czechia
- 2Biology Centre of ASCR, Institute of Parasitology, Ceske Budejovice, Czechia
- 3Department of Entomology, Southwestern Biological Institute, Tucson, AZ, United States
- 4US Army Public Health Command-Central, JBSA Fort Sam Houston, Houston, TX, United States
Insect microbiomes influence many fundamental host traits, including functions of practical significance such as their capacity as vectors to transmit parasites and pathogens. The knowledge on the diversity and development of the gut microbiomes in various blood feeding insects is thus crucial not only for theoretical purposes, but also for the development of better disease control strategies. In Triatominae (Heteroptera: Reduviidae), the blood feeding vectors of Chagas disease in South America and parts of North America, the investigation of the microbiomes is in its infancy. The few studies done on microbiomes of South American Triatominae species indicate a relatively low taxonomic diversity and a high host specificity. We designed a comparative survey to serve several purposes: (I) to obtain a better insight into the overall microbiome diversity in different species, (II) to check the long term stability of the interspecific differences, (III) to describe the ontogenetic changes of the microbiome, and (IV) to determine the potential correlation between microbiome composition and presence of Trypanosoma cruzi, the causative agent of Chagas disease. Using 16S amplicons of two abundant species from the southern US, and four laboratory reared colonies, we showed that the microbiome composition is determined by host species, rather than locality or environment. The OTUs (Operational Taxonomic Units) determination confirms a low microbiome diversity, with 12-17 main OTUs detected in wild populations of T. sanguisuga and T. protracta. Among the dominant bacterial taxa are Acinetobacter and Proteiniphilum but also the symbiotic bacterium Arsenophonus triatominarum, previously believed to only live intracellularly. The possibility of ontogenetic microbiome changes was evaluated in all six developmental stages and feces of the laboratory reared model Rhodnius prolixus. We detected considerable changes along the host's ontogeny, including clear trends in the abundance variation of the three dominant bacteria, namely Enterococcus, Acinetobacter, and Arsenophonus. Finally, we screened the samples for the presence of Trypanosoma cruzi. Comparing the parasite presence with the microbiome composition, we assessed the possible significance of the latter in the epidemiology of the disease. Particularly, we found a trend toward more diverse microbiomes in Trypanosoma cruzi positive T. protracta specimens.
Introduction
Insect microbiomes are recognized as a major factor determining host fitness and various phenotypic traits, including vectorial capacity in blood feeding species (Klepzig et al., 2009; Minard et al., 2013; Oliver and Martinez, 2014). While vector control strategies are now naturally venturing into the microbiome concept (Crotti et al., 2012; Saldaña et al., 2017), for some insect vectors and the allied pathogens the research focus has not yet fully passed the borders of epidemiological, surveillance, and medical studies. One such example are kissing bugs of the subfamily Triatominae (Hemiptera: Reduviidae) and Trypanosoma cruzi, the protozoan parasite responsible for Chagas disease.
Triatominae are hemimetabolous insects with five nymphal instars. Both adults and nymphs feed on vertebrate blood. There are over 140 extant Triatominae species with different biology, vectorial capacity and distribution, most of them found in South and Central America (Galvão et al., 2003; de Fuentes-Vicente et al., 2018). Those areas are the hot spots for Chagas disease, with nearly six million people affected (WHO, 2015). Among the seven main vector species of Trypanosoma cruzi in Latin America (Hernández et al., 2016), the most attention has traditionally been paid to Rhodnius prolixus colonizing domestic habitats (Gourbière et al., 2012). For many decades, R. prolixus has also served as a model organism to study insect physiology, immunity, metabolism, and development (Nunes-da-Fonseca et al., 2017). Such a status has promoted a high availability of information on this Triatominae species, including a whole genome sequence (Mesquita et al., 2015), and has contributed to unraveling its role as the vector for Trypanosoma cruzi.
The southern part of the United States is also endemic for this trypanosomiasis (Montgomery et al., 2014) and Chagas disease has recently been listed among the emerging diseases in this region (Edwards et al., 2017). Eleven Triatominae species are considered native to North America (Bern et al., 2011). The five species most frequently found are Triatoma gerstaeckeri, T. indictiva, T. lecticularia, T. sanguisuga, and T. rubida (Curtis-Robles et al., 2017a). So far, a modest number of both human and canine Trypanosoma cruzi infections has been documented in Texas (Wozniak et al., 2015; Curtis-Robles et al., 2017a). The latest surveillance data, however, reveal the presence of Triatominae adults and nymphs in domestic and peridomestic areas, which points at a potential increase of human infections (Curtis-Robles et al., 2017a). In fact, current studies are already describing a growing number of human subjects locally infected with Trypanosoma cruzi in the southern US (Garcia et al., 2017; Gunter et al., 2017).
Since Trypanosoma cruzi undergoes development exclusively in the intestinal tract of Triatominae vectors, it is exposed to interactions with gut microbes. Many studies to date have revealed an effect (either positive or negative) of microbiomes on the vectorial capacity of their insect hosts through different mechanisms such as resource competition, immunity modulation or antiparasitic secondary metabolite production (Cirimotich et al., 2011; Jupatanakul et al., 2014; Hegde et al., 2015). In Triatominae, the microbiomes interact with Trypanosoma cruzi by direct contact, including competition for resources (Garcia et al., 2010), or indirectly by increasing the expression of antiparasitic molecules and humoral immune defense factors of the host (Azambuja et al., 2005; Weiss and Aksoy, 2011). While there are two major threads to commonly used chemical control of Triatominae vectors, i.e., developing resistance and recurrent infestations by sylvatic species, symbiont manipulation has been proposed as a sustainable alternative (Gourbière et al., 2012). For instance, trypanolytic activity of some Triatominae associated Serratia marcescens strains (Azambuja et al., 2004) and gene silencing using transformed Rhodococcus rhodnii have been explored (Taracena et al., 2015). However, any advancement in microbiome-based vector control is hampered by the lack of a solid background on Triatominae symbiosis arising from the basic knowledge on the microbiome characteristics.
Until now, microbiome data and their basic characteristics have been available for a limited number of South American Triatominae species from wild and laboratory colonies (da Mota et al., 2012; Gumiel et al., 2015; Díaz et al., 2016). Based on these data, Triatominae possess low-complexity microbiomes, with one or few dominant bacterial genera, which appear to be specific to certain hosts (da Mota et al., 2012; Díaz et al., 2016). The complexity of lab-reared insect microbiomes is even lower, but maintains most of the bacterial groups found in their wild counterparts (da Mota et al., 2012). While for other insects the microbiomes are known to vary along the host ontogeny (Sudakaran et al., 2012; Gimonneau et al., 2014; Duguma et al., 2015), the development of Triatominae microbiomes is completely unknown. Considering the ability of all the instars to transmit Trypanosoma cruzi (Wozniak et al., 2015), possible changes in the microbiome composition along their ontogeny may be a key point toward the understanding of the disease transmission and its biological control. In this study, we extend the analysis of microbiome diversity and specificity to North American species from wild and laboratory colonies. We also take advantage of the model species R. prolixus and describe the microbiome variation in its different developmental stages. Finally, we present the first data on the correlation between the microbiome richness and presence of Trypanosoma cruzi in wild populations of two broadly found North American species, T. protracta and T. sanguisuga.
Materials and Methods
The Design of the Sample Set: Taxonomy and Origin
We used samples of four Triatominae species reared in long term laboratory colonies (R. prolixus, T. vitticeps, T. protracta, and T. recurva) and field collected samples of two species, T. protracta and T. sanguisuga. Due to this arrangement we could address the main hypothesis (i.e., the host specific microbiome composition) in two different ways. First, we compared field sampled individuals of two species from different localities to see whether the microbiome composition is determined by the host taxonomy or rather the geography. Second, we compared microbiomes of four species from laboratory colonies to determine whether the significant interspecific differences can be detected after a long term cohabitation in the same environment. Finally, since it has been shown in some other arthropods (the phylogenetically closest example being heteropterans of the genus Pyrrhocoris, see Discussion) that the microbiome composition can change during the host ontogeny, we used an available R. prolixus colony to directly characterize the ontogenetic development of the microbial communities across different developmental stages in a closely related and ecologically similar model.
Rearing Conditions and Microbiome Ontogeny
The microbiome ontogeny was assessed as follows. Eggs, all five nymphal instars (L1-L5), and adults of R. prolixus were analyzed in triplicates as described hereafter. Upon feces availability, feces from adults were collected on a filter paper within 24 h after deposition and processed in five replicates. The R. prolixus colony, established in 2010, originates from Brazil and has since been kept at the Faculty of Science, University of South Bohemia, Czech Republic. The colony is reared in plastic containers with mesh lids at a constant temperature of 27°C and 70% humidity under 16:8 h light/dark cycles. The blood meal, commercially purchased defibrinated sheep blood supplemented with egg yolk (Núñez and Lazzari, 1990), is supplied in 10 day intervals using artificial membrane feeding ad libitum.
Interspecific Diversity and Stability of the Microbiomes
The variability and host specificity of the microbial communities (i.e., the assumption that the specific microbiome profile is determined by the host species), was tested using laboratory reared colonies. In order to prevent downsizing of the colonies, only first and second instars were sampled in five replicates from four Triatominae species. The origins of the samples were as follows (see Table 1). The R. prolixus colony and T. vitticeps individuals were acquired from the above-described facility and were kept under the same breeding conditions. The colony of T. vitticeps originates from field-captured individuals sampled in Brazil and kept since 1995. The two other species, T. protracta and T. recurva, representing the North American (NA) Triatominae, were provided by the Southwestern Biological Institute, Tucson, AZ, USA. All the NA colonies were established by Justin Schmidt in 2010. T. protracta originated from Tucson, AZ, while T. recurva was sampled in Bisbee, AZ. The colonies are kept under simulated natural environmental conditions experienced by the insects in their native habitat. The temperature in the insectary is allowed to fluctuate with the weather, except with heating to maintain at least 4°C and cooling to maintain a temperature no higher than 32°C. Humidity is not being modified except during cooling conditions when maintained at 30%. Lighting is natural as provided by numerous windows of the insectary. Meals of rodent blood are provided every 14 days ad libitum as approved by the institutional review board of the Southwestern Biological Institute.
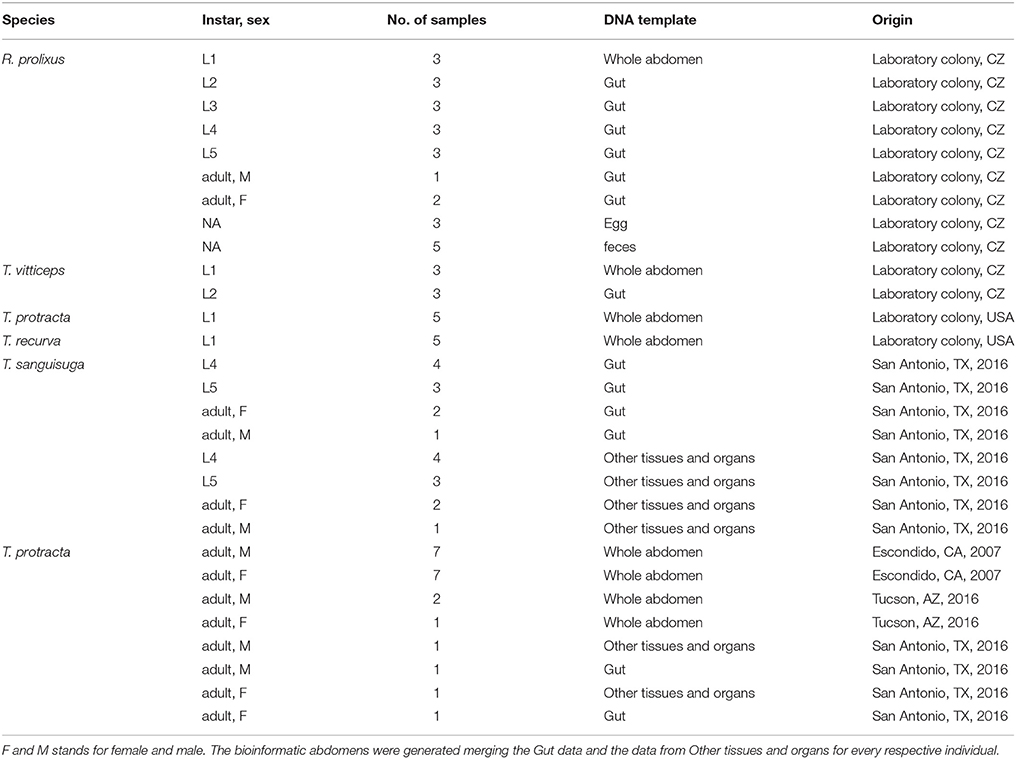
Table 1. List of all the DNA isolates for which the 16S rRNA gene amplicon data were generated in this study.
In addition to the laboratory-reared samples, we analyzed field collected samples to gain an initial insight into the diversity and ecology of the microbiome associated with the most widespread NA species. Triatominae sampled in the three most affected states, i.e., California, Arizona, and Texas, were provided by Christiane Weirauch (UC Riverside, California) and Walter D. Roachell (US Army Public Health Command Central, Texas). The samples were morphologically assigned to two species: T. sanguisuga and T. protracta, and the determination was further confirmed by molecular data. The complete information on laboratory bred and field collected individuals used in this study is provided in Table 1.
Template Extraction, Molecular Taxonomy and Trypanosoma cruzi Diagnostics
Colony individuals were sampled 4 days after feeding. Due to their size, the first instars were not dissected, and whole surface sterilized abdomens were used (see below for sterilization details). For the second and older instars in the ontogenetic survey, gut samples were acquired using a simple dissection protocol. The insects were surface sterilized by submersion in absolute ethanol for 5 s followed by a rinse in sterile deionizied water and dissected in sterile phosphate buffer saline (1X PBS; Sigma Life Science). The ventral cuticle was removed and the entire length of the gut was collected into individual tubes for each DNA extraction. The dissection ensured acquiring bacteria predominating the gut, where the main interactions with the host and possible parasites are expected to occur, and it also reduced any external contamination. To reduce possible contamination of the eggs, their environmental exposure was minimized collecting them immediately after deposition directly into tubes with the extraction buffer.
For the field sampled individuals, we optimized the extraction protocol so that comparable microbiome profiles could be generated from ethanol preserved abdomens (provided by some of our collaborators and available in numerous collections worldwide) and fresh dissected samples. Two separate templates were used from the dissected samples, i.e., the gut and the other abdominal tissues and organs (Table 1). This protocol, followed by merging the corresponding microbiome data into a “bioinformatic abdomen” (see section Data Processing and Statistical Analyses), ensures data comparability and provides gDNA gut specific templates for any future applications (i.e., qPCR and metagenomics). Total DNA was extracted from all the above described templates (Table 1) using the DNeasy Blood and Tissue (Qiagen, Hilden, Germany).
In order to verify the taxonomical determination of the 29 field collected individuals, we sequenced fragments of the COI gene amplified with the forward primers COIL6625 and/or COIL1490 and the reverse primer COIH7005 (Hafner et al., 1994). The reference sequence for T. sanguisuga was obtained from the adult individuals sampled by Walter Roachel in Lackland Air Force Base area, TX. The reference for T. protracta was sequenced from the laboratory colony (see above). A Maximum Likelihood (ML) analysis was performed with PhyML plugin in Geneious (Guindon et al., 2010; Kearse et al., 2012) to confirm the taxonomical assignment.
The field collected individuals were further screened for the presence of Trypanosoma cruzi. The diversity of Trypanosoma cruzi discrete typing units (DTUs), associated with geography, vector species, transmission and clinical outcomes (Hernández et al., 2016), spans five out of the six designated DTUs within United States (Garcia et al., 2017). Three sets of diagnostic primers were thus employed in this study: one generally amplifying all Trypanosoma cruzi DTUs (TCZ1/TCZ2; Moser et al., 1989) and the two other distinguishing for DTUI and the DTUII-VI group (ME/TC1 or TC2; Gumiel et al., 2015).
Library Preparation and Microbiome Sequencing
To generate data suitable for microbiome profiling, gDNA of 83 samples was amplified according to the EMP protocol (http://www.earthmicrobiome.org/protocols-and-standards/16s/), producing approximately 250 bp of the 16S rDNA V4 hypervariable region. Two negative controls of the extraction procedure and one blank control for PCR amplification were included in the sequencing. The libraries were then sequenced in a single run of Illumina MiSeq using the v2 chemistry (2 × 150 bp output mode).
Data Processing and Statistical Analyses
Forward and reverse paired-end reads were merged using USEARCH v7.0.1001 (fastq_mergepairs with fastq_minovlen set to 20; Edgar, 2013). Then the sequences were demultiplexed and their quality was checked using QIIME 1.8 (split_libraries_fastq.py with phred_quality_threshold set to 19; Caporaso et al., 2010b). The retained high quality sequences were aligned using the QIIME implementation of Pynast (Caporaso et al., 2010a) and trimmed to a final length of 251 bp using USEARCH (Edgar, 2013). Finally, the dataset was clustered at 100% identity to get a representative set for de novo OTU picking using the USEARCH global alignment option at 97% identity (Edgar, 2013). Each OTU was assigned to different taxonomic levels using the BLAST algorithm (Camacho et al., 2009) against the SILVA 123 database (Quast et al., 2013). The obtained OTU table was filtered to remove singletons and very low abundant OTUs using QIIME (following the recommendations of Bokulich et al., 2013), and all non-bacterial, chloroplast and mitochondrial OTUs were filtered out. After this final filtering step, the OTU table was rarefied at 1000 sequences per sample to normalize the dataset (as recommended in Weiss et al., 2017). The unrarefied data obtained for guts and other internal organs/tissues from each of the dissected wild sampled individuals were merged into “bioinformatic abdomens” using the QIIME script collapse_samples.py and normalized at the same rarefaction level of 1000 sequences per sample.
All statistical tests were performed in R environment (R Development Core Team, 2014), mainly using functions from the vegan package (Oksanen et al., 2013). The overall similarity among microbiomes was analyzed based on Bray-Curtis dissimilarities and visualized in two dimensional space using Non-Metric Dimensional Scaling (NMDS; Minchin, 1987). Using the vegan Adonis function, we tested the statistical significance of the differences among microbiomes from distinct host instars and host species. For T. protracta, differences between the distribution of diversity indices (Shannon index and richness) of males and females and between Trypanosoma cruzi positive and negative individuals were statistically evaluated using Kruskal-Wallis rank test (Kruskal and Wallis, 1952). All graphical outputs were generated using ggplot2 package (Wickham, 2009). In addition, 95% confident ellipses (Fox and Weisberg, 2011) were calculated with the stat_ellipse function of the same package.
Results
Sanger and Illumina Data
For molecular determination, we obtained 25 fragments 381 bp long and 4 fragments 652 bp long of the COI sequences. ML analysis of these fragments (not shown) determined 10 individuals as T. sanguisuga and 19 as T. protracta. The representative sequences for each species were deposited in GenBank under the following accession numbers: MH025901 and MH025902.
Illumina data were obtained for 42 samples from the laboratory reared colonies and 29 wild sampled individuals and 3 negative controls. The mean number of high quality merged reads passing the QC was 9279 (SD = 554). The extraction procedure controls resulted in low read numbers (988 and 666). The first extraction control was dominated by OTU161 (Segetibacter) that did not occur in any of the Triatominae microbiome data. The second extraction control sample as well as the blank PCR sample (253 reads) were composed of a mixture of low abundant OTUs that were excluded from further analysis. The raw data are available under ENA project no. PRJEB25175.
Microbiome Development Throughout the Host Ontogeny
The microbiome of R. prolixus undergoes major compositional changes along the host ontogeny (Figures 1, 2). Significant differences were found when comparing the microbiome of eggs, first four instars, fifth instar and adults, and feces of this species (R2 = 0.61335, significance level of 0.001, Figure 1B). When compared pairwise, all the microbiome pairs bore significant differences (four pairs at 0.01 level of significance and two at 0.05, see Supplementary Table 1). On average, the egg replicates showed the highest richness: 37 OTUs represented by numerous aerobes and facultative anaerobes. Two taxa equally dominated the microbial composition, namely Enterococcus and Bartonella (each accounting for 21.8%). The third well represented taxon was Methylobacterium (18.2%).
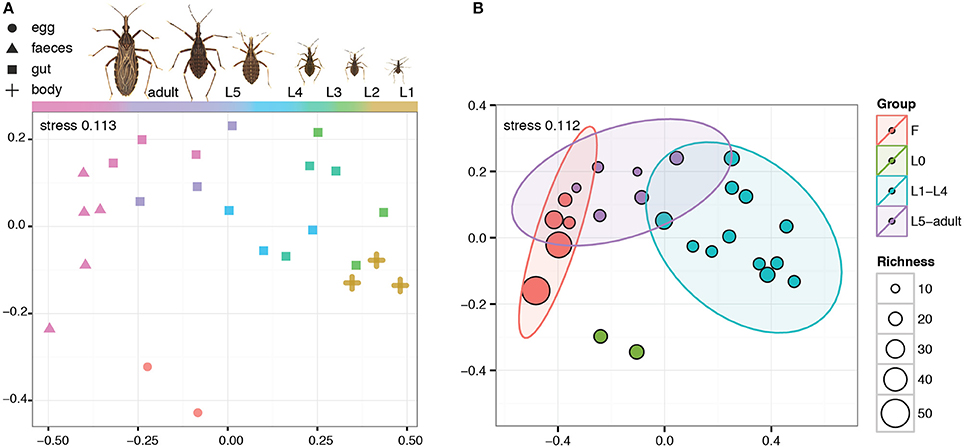
Figure 1. NMDS visualization of the ontogenetic shift of R. prolixus microbiome. F stands for feces, L0 for eggs, L1–L5 for nymphal instars. (A) Microbiome shift along the different developmental stages of R. prolixus. (B) NMDS including microbiome richness and 95% confident ellipses for each developmental stage. The ellipses were calculated with the stat_ellipse function of ggplot2 package.
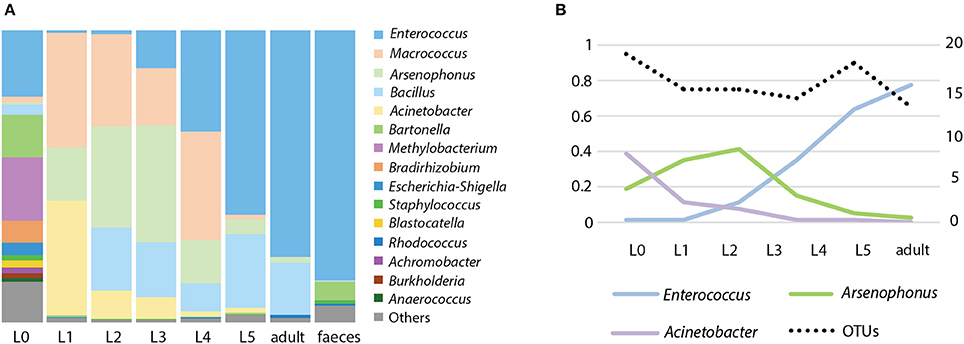
Figure 2. Microbiome of R. prolixus along its ontogeny. (A) Relative abundance of the main genera in different developmental stages. L0 stands for eggs, L1-L5 are nymphal instars. (B) Variation of the dominant OTUs; percentage of the total reads number (left axis) and number of OTUs in individual stages (right axis).
Starting with the first nymphal instar the composition of the bacterial community changes dramatically (Figure 2). The richness of the microbiome drops in the nymphal stages to 13-19 OTUs (Figure 2B), and in the feces it reaches 92 OTUs. During the ontogeny from L1 to an adult, the composition of the microbiome shows several consistent trends. The most pronounced of these trends are expressed in the quantities of the three dominant OTUs, Enterococcus, Acinetobacter, and Arsenophonus (Figure 2B). While the proportion of Enterococcus/Acinetobacter in the microbiome steadily increases/decreases during the host ontogeny, the number of Arsenophonus reaches its peaks in L3 and then starts decreasing (Figure 2). The only dominant OTU without clear consistent pattern was Macrococcus which varied in numbers among the nymphal stages and was absent in adults.
Microbiomes of T. protracta and T. sanguisuga
The two species from wild populations showed major differences between their microbiome profiles at the significance level of 0.001 (R2 = 0.289, Figure 3). Since the samples of T. protracta were mostly alcohol preserved, the two species were compared based on the microbial data retrieved from the entire abdomens and bioinformatically merged abdomen data. T. sanguisuga microbiomes retrieved from individuals of late nymphs (L4 and L5) and adults were composed of 17 OTUs with an averaged relative abundance higher than 1% (Supplementary Table 2). Among these, Arsenophonus and Acinetobacter bacteria clearly dominated. On average the relative abundance of Arsenophonus in all analyzed T. sanguisuga individuals was 39.5%, reaching 82.5% in the L4 nymphs, while for Acinetobacter the average was 14.3% with the highest relative abundance value of 34.1% in the last nymphal instar L5. Four other taxa exhibited an average relative abundance over 3%, i.e., Stenotrophomonas (6.2%), Rhodopseudomonas (4.6%), Pseudomonas (4.4%), and Sphingobacterium (3.4%) (Supplementary Table 2). T. protracta microbiome of 16 adult individuals was composed of 12 OTUs with an average relative abundance higher than 1% and the dominating OTU assigned to the genus Proteiniphilum (40.7%). Five OTUs showed an average relative abundance above 3%, i.e., Dietzia (11.6%), Salmonella (10.4%), Peptoniphilus (9.0%), Neisseriaceae (6.1%), Mycobacterium (3.7%). Dietzia was however recovered from a single individual of T. protracta reaching the abundance of 97% of all reads generated from this particular specimen (removed as an outlier from the Trypanosoma cruzi related analysis).
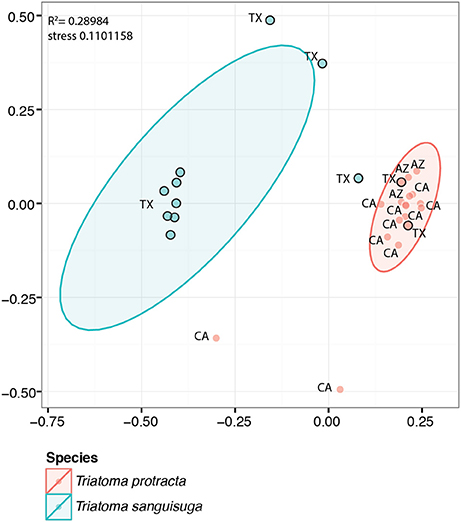
Figure 3. NMDS visualization of significant differences between the microbiomes of T. protracta and T. sanguisuga sampled from wild populations. The abbreviations stand for the states where the bugs were sampled. The black border circles designate samples for which the bioinformatic abdomen was generated (see Material and Methods section). The ellipses are 95% confident ellipses calculated with the stat_ellipse function of ggplot2 package.
When testing possible variability of T. protracta microbiome composition in relation to the sex of the host individuals, their geographical origin or the year of sampling, no significant differences were found. The only significant correlation was identified for the presence of Trypanosoma cruzi and the richness of T. protracta microbiome (Kruskal-Wallis chi-squared = 7.4558, df = 1, p-value = 0.006; Figure 4). The prevalence of Trypanosoma cruzi in 16 analyzed T. protracta adult individuals was 25%, with two cases of DTUI and the other two from DTUII-VI group.
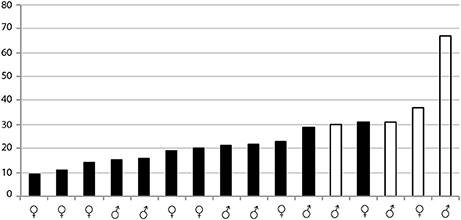
Figure 4. Significant differences in microbiome richness of Trypanosoma cruzi infected and uninfected T. protracta adult individuals measured by Kruskal-Wallis rank test (chi-squared = 7.4558, df = 1, p-value = 0.006323). The numbers stand for the richness values. The black bars represent Trypanosoma cruzi negative samples. The white bars are Trypanosoma cruzi positive samples.
Due to a single case of Trypanosoma cruzi infection in the tested set of T. sanguisuga, any correlation of microbiome profile with the presence of the parasite could not be evaluated for this species. It is however noteworthy that this case represented a double infection, with positive diagnostic PCR for both DTUI and DTUII-VI groups.
Microbiomes in the Laboratory Reared Colonies
The laboratory reared colonies also showed differential patterns in their microbiomes according to the Triatominae host species. Particularly, differences among the four species were evaluated as significant at the level of 0.001 (R2 = 0.784) using adonis (Figure 5). Colonies of T. protracta and T. recurva were dominated by Enterobacteriaceae (Pectobacterium sp.), while colonies of T. vitticeps were dominated mainly by Enterococcaceae (Enterococcus sp.) and also by Enterobacteriaceae (Arsenophonus sp.). R. prolixus did not show one single predominant OTU, but three with similar high abundance: Staphylococcaceae (Macrococcus sp.), Enterobacteriaceae (Arsenophonus sp.) and Moraxellaceae (Acinetobacter sp.). Bacillaceae (Bacillus sp.) were the next more predominant bacteria in R. prolixus and T. vitticeps.
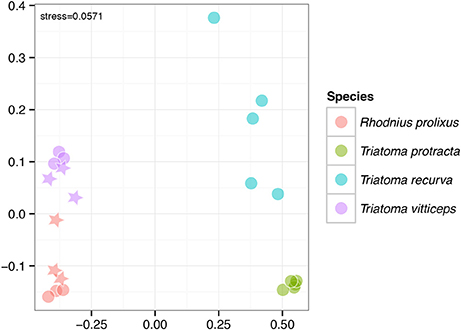
Figure 5. Significant differences among microbiomes of the lab-reared colonies depicted by the NMDS clustering (significance level of 0.001, R2 = 0.784). The circles stand for L1 instar, stars for L2.
Arsenophonus triatominarum Patterns
Both field collected and laboratory reared Triatominae showed distinct patterns for A. triatominarum presence. The averaged relative abundance of this bacterium in the gut of T. sanguisuga field-collected individuals was 39.76% (SD = 40.19%). However, the relative abundance showed an immense variance among the analyzed individuals spanning from as low as 0.02% found in L5 instars up to 99.03% in the adults. No reads for A. triatominarum were detected in T. protracta from wild populations. For laboratory-reared colonies, the patterns of A. triatominarum were more variable: R. prolixus harbored A. triatominarum in the gut (26.36 ± 20.43%), but this bacterium was absent in the samples from eggs or feces. T. vitticeps also harbored Arsenophonus in the gut (29.54 ± 28.21%). Arsenophonus was not found in T. recurva and T. protracta microbiomes of the sampled colonies.
Discussion
Microbiomes and Host Ontogeny
Given the current knowledge, it is difficult to make any general comparison of the pattern we found in Triatominae (i.e., the microbiome of early nymphs being different from the adults one) to other arthropods, since only few other groups have been analyzed to date. For example in ticks, the microbiome changes along the development toward higher diversity, probably due to off-host environmental bacterial acquisition (Zolnik et al., 2016). However, ticks feed on blood throughout their life, and the main component of the microbiome, Rickettsia, is present in all life stages, particularly in adult females and larvae, where it comprises most of the microbiome, possibly due to vertical transmission mechanisms (Zolnik et al., 2016). A different ontogenetic pattern was detected in mosquitoes, whose larval microbiome is more diverse and highly influenced by the environment (i.e., aquatic), while the adult microbiome shows a different, more stable pattern (Gimonneau et al., 2014; Duguma et al., 2015). As mosquitoes are hematophagous only in the adult stage, it has been suggested that selection toward functionally important bacteria in this context acts to shape the adult female mosquito microbiome (Gimonneau et al., 2014). Within Heteroptera, the bloodfeeding Cimicidae do not rely on gut microbiome, but possess so called “primary symbionts” dwelling in bacteriomes (Hypša and Aksoy, 2003; Nikoh et al., 2014), which make them difficult to compare to Triatominae. Perhaps the most similar pattern has been found in non-hematophagous heteropterans, the firebug Pyrrhocoris apterus. This species also show remarkable shifts in the microbiome profile when comparing the first instars with the latter instars and adults, but this difference is probably due to the change of feeding habits between stages (Sudakaran et al., 2012). There is currently too little data to make any conclusion on the phylogeny or feeding strategy as the main determinant of the microbiome ontogeny, but it is interesting to note that even in strictly hematophagous vectors, the blood meal effect seems to be quite limited (Zolnik et al., 2016).
Regarding the higher richness of bacteria in feces and eggs, the environmental component is worth discussion. The higher diversity in the egg microbiome compared to the nymphal instars could reflect a common contact of eggs and feces. In addition, the feces samples were exposed to possible external contamination and aerobic bacterial growth for a longer period (up to 24 h compared to the immediate collection of eggs after deposition). The three-fold increment in the richness of feces microbiome could thus be explained by this exposure time difference.
Triatominae can act as vectors of Trypanosoma cruzi at any of their developmental stages. The prevalence of Trypanosoma cruzi infection seems to be higher in adults and the latest instars compared to the younger nymphs, although this is based on a small number of unidentified nymph samples (McPhatter et al., 2012; Curtis-Robles et al., 2015; Wozniak et al., 2015). Different exposure to blood feeding on different hosts might be one of the possible factors behind these different infection rates. In our analyses of laboratory-reared individuals, we identify microbiome composition as another important trait which undergoes significant changes during the vector's ontogeny. This suggests that the microbiome may be another possible determinant of Trypanosoma cruzi prevalence. A further investigation on the microbiome ontogenetic patterns in wild populations of Triatominae and its correlation with Trypanosoma cruzi infection is needed to confirm this hypothesis.
Microbiomes in Wild Populations
Our results support previous findings on low-level complexity microbiomes of South American Triatominae. The most abundant bacterial taxa in T. sanguisuga and T. protracta comply with the diversity span previously described for South American species. T. sanguisuga microbiome profile dominated by two taxa, i.e., Arsenophonus and Acinetobacter, mirrors for instance that of T. brasiliensis complex (Díaz et al., 2016). T. protracta harbors distinct bacterial lineages. While Salmonella association with some Triatominae species was previously reported (Amino et al., 1998), additional taxa found here in T. protracta microbiome, i.e., Porphyromonadaceae (Proteiniphilum sp.), Clostridiales (Peptoniphilus sp.) and Neisseriaceae were identified as symbionts in other insects (e.g., Kwong et al., 2014; Husseneder et al., 2017; Zhang et al., 2017).
Microbiome species-specificity have been detected in several insect hosts to date (Aksoy et al., 2014; Leonhardt and Kaltenpoth, 2014; Novakova et al., 2017). The microbiome of Triatominae does not seem to be an exception, and species-specific patterns have been described as one of the main factors shaping the microbiome composition (da Mota et al., 2012; Díaz et al., 2016). While these studies were conducted mostly using laboratory-reared bugs, our results point out microbiome species-specific patterns in wild populations of NA Triatominae.
Apart from the species-specific patterns, we also checked for other factors possibly affecting the microbiome composition in Triatominae. T. protracta was collected from three remote locations in the southern US (San Antonio, TX; Tucson, AZ; Escondido, CA). However, neither the geographical origin of the samples nor the sex of the adult individuals showed an effect on the Triatominae microbiome. These results are in accordance with the characteristics described for another hemipteran, the firebug, where the microbiome is remarkably stable across different populations, sexes, and even diets (Sudakaran et al., 2012). However, since our data lack the information on blood meal source and originate from a complex sample set, including L4, L5 and adult individuals, the effects of these variables on the microbiome composition within wild populations will require further investigations.
Microbiome—Trypanosoma cruzi Interface
The prevalence of Trypanosoma cruzi in our T. protracta samples was 25%, lower than the prevalence found in previous studies of Triatominae from Texas (Curtis-Robles et al., 2017b). However, we still identified different Trypanosoma cruzi DTUs (here only differentiated as DTUI and non-DTUI). These findings are in accordance with recent studies, where at least four different DTUs have been found in Southern US (Garcia et al., 2017). Particularly, the main DTU found in Texas (DTUI) bears a high medical importance as causative agent of Chagasic cardiac disease, highlighting the necessity for further research on this topic in the US (Curtis-Robles et al., 2017b; Garcia et al., 2017).
Previous studies have found a significant change in the microbiome composition of different South American species of Triatominae when challenged with a Trypanosoma cruzi infected blood meal (Díaz et al., 2016). Working with wild populations, we collected Trypanosoma cruzi positive and negative individuals, which allowed us to assess the correlation between the microbiome and the infection status of T. protracta adults. We found that the diversity of the microbiomes in Trypanosoma cruzi positive individuals is significantly higher than in the negative ones. This result remarkably agrees with the findings on Trypanosoma cruzi challenged T. brasiliensis, T. sherlocki, and P. megistus (Díaz et al., 2016). However, due to the limited number of available samples, further studies will be necessary to confirm this correlation in natural Triatominae populations.
Arsenophonus triatominarum
A. triatominarum has been described as an intracellular symbiont with strict specificity to various organs and tissues in Triatoma infestans (Hypša, 1993). It is therefore interesting to see this bacterium as one of the dominant components of Triatominae gut microbiomes. The presence of A. triatominarum in the host guts, and feces, could provide an explanation for the intriguing host distribution of these bacteria. It has been noted that A. triatominarum is exclusively bound to Triatominae, but does not show phylogenetic concordance with the host (Sorfová et al., 2008). In fact, the extremely low molecular diversity of the Triatominae bound Arsenophonus suggests a recent acquisition and rapid horizontal spread rather than a long time coevolution. While the supposed intracellular location within internal organs was difficult to reconcile with such frequent and host specific horizontal transfers, the occurrence in gut content offers a new possible explanation, such as coprophagy or environmental contamination. Another interesting aspect of A. triatominarum being part of the gut microbiome is the possible role of this symbiont. While several members of the genus Arsenophonus are known for their profound effect on the host (reproduction distorters or nutritional mutualists; Wilkes et al., 2011; Nováková et al., 2015, 2016), no such phenomenon has so far been observed for A. triatomiarum. Considering a possible role of this symbiont, it might be interesting to note that unlike the other two major OTUs, Enterococcus and Acinetobacter, its relative abundance seems to grow from the first until the third nymphal stages and then drops afterwards. The study of this symbiotic bacterium in the context of the complex and dynamic gut microbiome thus offers a new opportunity to search for its possible effects on the host fitness.
Conclusion
The results presented here demonstrate that the composition of Triatominae microbiome is host-specific, maintaining its identity across large geographic areas. Compared to many other insect microbiomes, its overall diversity is low, with 12-17 main bacterial OTUs identified in wild populations of Triatoma sanguisuga and T. protracta. Surprisingly, one of the dominant taxa is the symbiotic bacterium Arsenophonus triatominarum, previously known as an exclusively intracellular symbiont from several Triatominae species. Based on the observations of a laboratory reared colony of Rhodnius prolixus, the frequency of the most abundant bacterial genera (Enterococcus, Acinetobacter, and Arsenophonus in this Triatominae species) undergoes significant and consistent changes during the host ontogeny. Finally, a screening of T. protracta indicates possible relation between the microbiomes diversity and the occurrence of Trypanosoma cruzi in the host gut. This finding highlights the significance of further studies on the microbiomes of NA Triatominae species as a background for developing efficient vector control strategies.
Author Contributions
EN and SR-R designed the study. RR, JS, and WR managed the field collections and colony rearing. VS, SR-R and EN generated the data and performed the data analyses. EN, SR-R, and VH drafted the manuscript. All the authors read and contributed to the final text.
Conflict of Interest Statement
The authors declare that the research was conducted in the absence of any commercial or financial relationships that could be construed as a potential conflict of interest.
Acknowledgments
We would like to thank Christiane Weirauch who provided us with T. protracta samples, Roman Hrdlicka for the care he pays to the Triatominae colonies, and Noah Fierer's laboratory for conducting the sequencing. The work was supported by the Ministry of Education, Youth and Sports of the Czech Republic (grant LH-15161 under the program for international collaboration KONTAKT II), and the Grant Agency of the Czech Republic (project no. 18-24707Y).
Supplementary Material
The Supplementary Material for this article can be found online at: https://www.frontiersin.org/articles/10.3389/fmicb.2018.01167/full#supplementary-material
References
Aksoy, E., Telleria, E. L., Echodu, R., Wu, Y., Okedi, L. M., Weiss, B. L., et al. (2014). Analysis of multiple Tsetse fly populations in Uganda reveals limited diversity and species-specific gut microbiota. Appl. Environ. Microbiol. 80, 4301–4312. doi: 10.1128/AEM.00079-14
Amino, R., Porto, R. M., Chammas, R., Egami, M. I., and Schenkman, S. (1998). Identification and characterization of a sialidase released by the salivary gland of the hematophagous insect Triatoma infestans. J. Biol. Chem. 273, 24575–24582. doi: 10.1074/jbc.273.38.24575
Azambuja, P., Feder, D., and Garcia, E. S. (2004). Isolation of Serratia marcescens in the midgut of Rhodnius prolixus: impact on the establishment of the parasite Trypanosoma cruzi in the vector. Exp. Parasitol. 107, 89–96. doi: 10.1016/j.exppara.2004.04.007
Azambuja, P., Garcia, E. S., and Ratcliffe, N. A. (2005). Gut microbiota and parasite transmission by insect vectors. Trends Parasitol. 21, 568–572. doi: 10.1016/j.pt.2005.09.011
Bern, C., Kjos, S., Yabsley, M. J., and Montgomery, S. P. (2011). Trypanosoma cruzi and Chagas' disease in the United States. Clin. Microbiol. Rev. 24, 655–681. doi: 10.1128/CMR.00005-11
Bokulich, N. A., Subramanian, S., Faith, J. J., Gevers, D., Gordon, J. I., Knight, R., et al. (2013). Quality-filtering vastly improves diversity estimates from Illumina amplicon sequencing. Nat. Methods 10, 57–59. doi: 10.1038/nmeth.2276
Camacho, C., Coulouris, G., Avagyan, V., Ma, N., Papadopoulos, J., Bealer, K., et al. (2009). BLAST+: architecture and applications. BMC Bioinformatics 10:421. doi: 10.1186/1471-2105-10-421
Caporaso, J. G., Bittinger, K., Bushman, F. D., DeSantis, T. Z., Andersen, G. L., and Knight, R. (2010a). PyNAST: a flexible tool for aligning sequences to a template alignment. Bioinformatics 26, 266–267. doi: 10.1093/bioinformatics/btp636
Caporaso, J. G., Kuczynski, J., Stombaugh, J., Bittinger, K., Bushman, F. D., Costello, E. K., et al. (2010b). QIIME allows analysis of high-throughput community sequencing data. Nat. Methods 7, 335–336. doi: 10.1038/nmeth.f.303
Cirimotich, C. M., Ramirez, J. L., and Dimopoulos, G. (2011). Native microbiota shape insect vector competence for human pathogens. Cell Host Microbe 10, 307–310. doi: 10.1016/j.chom.2011.09.006
Crotti, E., Balloi, A., Hamdi, C., Sansonno, L., Marzorati, M., Gonella, E., et al. (2012). Microbial symbionts: a resource for the management of insect-related problems. Microb. Biotechnol. 5, 307–317. doi: 10.1111/j.1751-7915.2011.00312.x
Curtis-Robles, R., Hamer, S. A., Lane, S., Levy, M. Z., and Hamer, G. L. (2017a). Bionomics and spatial distribution of triatomine vectors of Trypanosoma cruzi in Texas and other southern states, USA. Am. J. Trop. Med. Hyg. 98, 113–121. doi: 10.4269/ajtmh.17-0526
Curtis-Robles, R., Snowden, K. F., Dominguez, B., Dinges, L., Rodgers, S., Mays, G., et al. (2017b). Epidemiology and molecular typing of Trypanosoma cruzi in naturally-infected hound dogs and associated triatomine vectors in Texas, USA. PLoS Negl. Trop. Dis. 11:e0005298. doi: 10.1371/journal.pntd.0005298
Curtis-Robles, R., Wozniak, E. J., Auckland, L. D., Hamer, G. L., and Hamer, S. A. (2015). Combining public health education and disease ecology research: using citizen science to assess Chagas disease entomological risk in Texas. PLoS Negl. Trop. Dis. 9:e0004235. doi: 10.1371/journal.pntd.0004235
da Mota, F. F., Marinho, L. P., Moreira, C. J., Lima, M. M., Mello, C. B., Garcia, E. S., et al. (2012). Cultivation-independent methods reveal differences among bacterial gut microbiota in triatomine vectors of Chagas disease. PLoS Negl. Trop. Dis. 6:e1631. doi: 10.1371/journal.pntd.0001631
de Fuentes-Vicente, J. A., Gutiérrez-Cabrera, A. E., Flores-Villegas, A. L., Lowenberger, C., Benelli, G., Salazar-Schettino, P. M., et al. (2018). What makes an effective Chagas disease vector? Factors underlying Trypanosoma cruzi-triatomine interactions. Acta Trop. 183, 23–31. doi: 10.1016/j.actatropica.2018.04.008
Díaz, S., Villavicencio, B., Correia, N., Costa, J., and Haag, K. L. (2016). Triatomine bugs, their microbiota and Trypanosoma cruzi: asymmetric responses of bacteria to an infected blood meal. Parasit. Vectors 9:636. doi: 10.1186/s13071-016-1926-2
Duguma, D., Hall, M. W., Rugman-Jones, P., Stouthamer, R., Terenius, O., Neufeld, J. D., et al. (2015). Developmental succession of the microbiome of Culex mosquitoes. BMC Microbiol. 15:140. doi: 10.1186/s12866-015-0475-8
Edgar, R. C. (2013). UPARSE: highly accurate OTU sequences from microbial amplicon reads. Nat. Methods 10, 996–998. doi: 10.1038/nmeth.2604
Edwards, M. S., Stimpert, K. K., and Montgomery, S. P. (2017). Addressing the challenges of Chagas disease: an emerging health concern in the United States. Infect. Dis. Clin. Pract. 25:118. doi: 10.1097/IPC.0000000000000512
Fox, J., and Weisberg, S. (2011). An R Companion to Applied Regression, 2nd Edn. Thousand Oaks, CA: Sage Publications. Available online at: http://socserv.socsci.mcmaster.ca/jfox/Books/Companion.
Galvão, C., Carcavallo, R., Rocha, D. D. S., and Jurberg, J. (2003). A checklist of the current valid species of the subfamily Triatominae Jeannel, 1919 (Hemiptera, Reduviidae) and their geographical distribution, with nomenclatural and taxonomic notes. Zootaxa 202, 1–36. doi: 10.11646/zootaxa.202.1.1
Garcia, E. S., Castro, D. P., Figueiredo, M. B., and Azambuja, P. (2010). Immune homeostasis to microorganisms in the guts of triatomines (Reduviidae): a review. Mem. Inst. Oswaldo Cruz 105, 605–610. doi: 10.1590/S0074-02762010000500001
Garcia, M. N., Burroughs, H., Gorchakov, R., Gunter, S. M., Dumonteil, E., Murray, K. O., et al. (2017). Molecular identification and genotyping of Trypanosoma cruzi DNA in autochthonous Chagas disease patients from Texas, USA. Infect. Genet. Evol. 49, 151–156. doi: 10.1016/j.meegid.2017.01.016
Gimonneau, G., Tchioffo, M. T., Abate, L., Boissière, A., Awono-Ambéné, P. H., Nsango, S. E., et al. (2014). Composition of Anopheles coluzzii and Anopheles gambiae microbiota from larval to adult stages. Infect. Genet. Evol. 28, 715–724. doi: 10.1016/j.meegid.2014.09.029
Gourbière, S., Dorn, P., Tripet, F., and Dumonteil, E. (2012). Genetics and evolution of triatomines: from phylogeny to vector control. Heredity 108, 190–202. doi: 10.1038/hdy.2011.71
Guindon, S., Dufayard, J. F., Lefort, V., Anisimova, M., Hordijk, W., and Gascuel, O. (2010). New Algorithms and methods to estimate maximum-likelihood phylogenies: assessing the performance of PhyML 3.0. Syst. Biol. 59, 307–321. doi: 10.1093/sysbio/syq010
Gumiel, M., da Mota, F. F., Rizzo Vde, S., Sarquis, O., de Castro, D. P., Lima, M. M., et al. (2015). Characterization of the microbiota in the guts of Triatoma brasiliensis and Triatoma pseudomaculata infected by Trypanosoma cruzi in natural conditions using culture independent methods. Parasit. Vectors 8:245. doi: 10.1186/s13071-015-0836-z
Gunter, S. M., Murray, K. O., Gorchakov, R., Beddard, R., Rossmann, S. N., Montgomery, S. P., et al. (2017). Likely autochthonous transmission of Trypanosoma cruzi to humans, south central Texas, USA. Emerg. Infect. Dis. 23, 500–503. doi: 10.3201/eid2303.161157
Hafner, M. S., Sudman, P. D., Villablanca, F. X., Spradling, T. A., Demastes, J. W., and Nadler, S. A. (1994). Disparate rates of molecular evolution in cospeciating hosts and parasites. Science 265, 1087–1090. doi: 10.1126/science.8066445
Hegde, S., Rasgon, J. L., and Hughes, G. L. (2015). The microbiome modulates arbovirus transmission in mosquitoes. Curr. Opin. Virol. 15, 97–102. doi: 10.1016/j.coviro.2015.08.011
Hernández, C., Salazar, C., Brochero, H., Teherán, A., Buitrago, L. S., Vera, M., et al. (2016). Untangling the transmission dynamics of primary and secondary vectors of Trypanosoma cruzi in Colombia: parasite infection, feeding sources and discrete typing units. Parasit. Vectors 9:620. doi: 10.1186/s13071-016-1907-5
Husseneder, C., Park, J. S., Howells, A., Tikhe, C. V., and Davis, J. A. (2017). Bacteria associated with Piezodorus guildinii (Hemiptera: Pentatomidae), with special reference to those transmitted by feeding. Environ. Entomol. 46, 159–166. doi: 10.1093/ee/nvw112
Hypša, V. (1993). Endosytobionts of Triatoma infestans: distribution and transmission. J. Invertebr. Pathol. 61, 32–38. doi: 10.1006/jipa.1993.1006
Hypša, V., and Aksoy, S. (2003). Phylogenetic characterization of two transovarially transmitted endosymbionts of the bedbug Cimex lectularius (Heteroptera: Cimicidae). Insect Mol. Biol. 6, 301–304. doi: 10.1046/j.1365-2583.1997.00178.x
Jupatanakul, N., Sim, S., and Dimopoulos, G. (2014). The insect microbiome modulates vector competence for arboviruses. Viruses 6, 4294–4313. doi: 10.3390/v6114294
Kearse, M., Moir, R., Wilson, A., Stones-Havas, S., Cheung, M., Sturrock, S., et al. (2012). Geneious Basic: an integrated and extendable desktop software platform for the organization and analysis of sequence data. Bioinformatics 28, 1647–1649. doi: 10.1093/bioinformatics/bts199
Klepzig, K. D., Adams, A. S., Handelsman, J., and Raffa, K. F. (2009). Symbioses: a key driver of insect physiological processes, ecological interactions, evolutionary diversification, and impacts on humans. Environ. Entomol. 38, 67–77. doi: 10.1603/022.038.0109
Kruskal, W. H., and Wallis, W. A. (1952). Use of ranks in one-criterion variance analysis. J. Am. Stat. Assoc. 47, 583–621. doi: 10.1080/01621459.1952.10483441
Kwong, W. K., Engel, P., Koch, H., and Moran, N. A. (2014). Genomics and host specialization of honey bee and bumble bee gut symbionts. Proc. Natl. Acad. Sci. U.S.A. 111, 11509–11514. doi: 10.1073/pnas.1405838111
Leonhardt, S. D., and Kaltenpoth, M. (2014). Microbial communities of three sympatric Australian stingless bee species. PLoS ONE 9:e10571 doi: 10.1371/journal.pone.0105718
McPhatter, L., Roachell, W., Mahmood, F., Hoffman, L., Lockwood, N., Osuna, A., et al. (2012). Vector surveillance to determine species composition and occurrence of Trypanosoma cruzi at three military installations in San Antonio, Texas. US Army Med. Dep. J. 3–12, 12–21.
Mesquita, R. D., Vionette-Amaral, R. J., Lowenberger, C., Rivera-Pomar, R., Monteiro, F. A., Minx, P., et al. (2015). Genome of Rhodnius prolixus, an insect vector of Chagas disease, reveals unique adaptations to hematophagy and parasite infection. Proc. Natl. Acad. Sci. U.S.A. 112, 14936–14941. doi: 10.1073/pnas.1506226112
Minard, G., Mavingui, P., and Moro, C. V. (2013). Diversity and function of bacterial microbiota in the mosquito holobiont. Parasit. Vectors 6:146. doi: 10.1186/1756-3305-6-146
Minchin, P. R. (1987). An evaluation of the relative robustness of techniques for ecological ordination. Vegetatio 69, 89–107. doi: 10.1007/BF00038690
Montgomery, S. P., Starr, M. C., Cantey, P. T., Edwards, M. S., and Meymandi, S. K. (2014). Neglected parasitic infections in the United States: Chagas disease. Am. J. Trop. Med. Hyg. 90, 814–818. doi: 10.4269/ajtmh.13-0726
Moser, D. R., Kirchhoff, L. V., and Donelson, J. E. (1989). Detection of Trypanosoma cruzi by DNA amplification using the polymerase chain reaction. J. Clin. Microbiol. 27, 1477–1482.
Nikoh, N., Hosokawa, T., Moriyama, M., Oshima, K., Hattori, M., and Fukatsu, T. (2014). Evolutionary origin of insect–Wolbachia nutritional mutualism. Proc. Nat. Acad. Sci. U.S.A. 111, 10257–10262. doi: 10.1073/pnas.1409284111
Nováková, E., Husník, F., Šochová, E., and Hypša, V. (2015). Arsenophonus and Sodalis symbionts in louse flies: an analogy to the Wigglesworthia and Sodalis system in tsetse flies. Appl. Environ. Microbiol. 81, 6189–6199. doi: 10.1128/AEM.01487-15
Nováková, E., Hypša, V., Nguyen, P., Husník, F., and Darby, A. C. (2016). Genome sequence of Candidatus Arsenophonus lipopteni, the exclusive symbiont of a blood sucking fly Lipoptena cervi (Diptera: Hippoboscidae). Stand. Genomic Sci. 11:72. doi: 10.1186/s40793-016-0195-1
Novakova, E., Woodhams, D. C., Rodríguez-Ruano, S. M., Brucker, R. M., Leff, J. W., Maharaj, A., et al. (2017). Mosquito microbiome dynamics, a background for prevalence and seasonality of West Nile Virus. Front. Microbiol. 8:526. doi: 10.3389/fmicb.2017.00526
Nunes-da-Fonseca, R., Berni, M., Tobias-Santos, V., Pane, A., and Araujo, H. M. (2017). Rhodnius prolixus: from classical physiology to modern developmental biology. Genesis 55:e22995. doi: 10.1002/dvg.22995
Núñez, J. A., and Lazzari, C. R. (1990). Rearing of Triatoma infestans Klug (Het., Reduviidae) in the absence of a live host. J. Appl. Entomol. 109, 87–92. doi: 10.1111/j.1439-0418.1990.tb00023.x
Oksanen, J., Blanchet, F. G., Kindt, R., Legendre, P., Minchin, P. R., O'Hara, R. B., et al. (2013). Vegan: Community Ecology Package. The Comprehensive R Archive Network (CRAN) Available online at: http://CRAN.R-project.org/package=vegan.
Oliver, K. M., and Martinez, A. J. (2014). How resident microbes modulate ecologically-important traits of insects. Curr. Opin. Insect Sci. 4, 1–7. doi: 10.1016/j.cois.2014.08.001
Quast, C., Pruesse, E., Yilmaz, P., Gerken, J., Schweer, T., Yarza, P., et al. (2013). The SILVA ribosomal RNA gene database project: improved data processing and web-based tools. Nucleic Acids Res. 41, D590–D596. doi: 10.1093/nar/gks1219
R Development Core Team (2014). R: A Language and Environment for Statistical Computing. R Foundation for Statistical Computing Vienna. Available online at: http://www.R-project.org/
Saldaña, M. A., Hegde, S., and Hughes, G. L. (2017). Microbial control of arthropod-borne disease. Mem. Inst. Oswaldo Cruz 112, 81–93. doi: 10.1590/0074-02760160373
Sorfová, P., Skeríková, A., and Hypsa, V. (2008). An effect of 16S rRNA intercistronic variability on coevolutionary analysis in symbiotic bacteria: molecular phylogeny of Arsenophonus triatominarum. Syst. Appl. Microbiol. 31, 88–100. doi: 10.1016/j.syapm.2008.02.004
Sudakaran, S., Salem, H., Kost, C., and Kaltenpoth, M. (2012). Geographical and ecological stability of the symbiotic mid-gut microbiota in European firebugs, Pyrrhocoris apterus (Hemiptera, Pyrrhocoridae). Mol. Ecol. 21, 6134–6151. doi: 10.1111/mec.12027
Taracena, M. L., Oliveira, P. L., Almendares, O., Umaña, C., Lowenberger, C., Dotson, E. M., et al. (2015). Genetically modifying the insect gut microbiota to control Chagas disease vectors through systemic RNAi. PLoS Negl. Trop. Dis. 9:e0003358. doi: 10.1371/journal.pntd.0003358
Weiss, B., and Aksoy, S. (2011). Microbiome influences on insect host vector competence. Trends Parasitol. 27, 514–522. doi: 10.1016/j.pt.2011.05.001
Weiss, S., Xu, Z. Z., Peddada, S., Amir, A., Bittinger, K., Gonzalez, A., et al. (2017). Normalization and microbial differential abundance strategies depend upon data characteristics. Microbiome 5:27. doi: 10.1186/s40168-017-0237-y
WHO (2015). Chagas disease in Latin America: an epidemiological update based on 2010 estimates. Releve Epidemiol. Hebd. 90, 33–43.
Wickham, H. (2009). Ggplot2: Elegant Graphics for Data Analysis. New York, NY: Springer-Verlag Available online at: http://ggplot2.org.
Wilkes, T. E., Duron, O., Darby, A. C., Hypša, V., Nováková, E., and Hurst, G. D. D. (2011). “The genus Arsenophonus,” in Manipulative Tenants: Bacteria Associated With Arthropods, eds E. Zchori-Fein and K. Bourtzis, (Boca Raton, FL: CRC Press), 520–528.
Wozniak, E. J., Lawrence, G., Gorchakov, R., Alamgir, H., Dotson, E., Sissel, B., et al. (2015). The biology of the Triatomine bugs native to south central Texas and assessment of the risk they pose for autochthonous Chagas disease exposure. J. Parasitol. 101, 520–528. doi: 10.1645/15-748
Zhang, G., Browne, P., Zhen, G., Johnston, A., Cadillo-Quiroz, H., and Franz, N. (2017). Endosymbiont diversity and evolution across the weevil tree of life. bioRxiv, 171181. doi: 10.1101/171181
Keywords: Triatominae, microbiome, ontogeny, Trypanosoma cruzi, Rhodnius prolixus
Citation: Rodríguez-Ruano SM, Škochová V, Rego ROM, Schmidt JO, Roachell W, Hypša V and Nováková E (2018) Microbiomes of North American Triatominae: The Grounds for Chagas Disease Epidemiology. Front. Microbiol. 9:1167. doi: 10.3389/fmicb.2018.01167
Received: 09 March 2018; Accepted: 14 May 2018;
Published: 13 June 2018.
Edited by:
Francesca Turroni, Università degli Studi di Parma, ItalyReviewed by:
Guillaume Henri Tetreau, UMR5075 Institut de Biologie Structurale (IBS), FranceAlex Cordoba-Aguilar, Universidad Nacional Autónoma de México, Mexico
Copyright © 2018 Rodríguez-Ruano, Škochová, Rego, Schmidt, Roachell, Hypša and Nováková. This is an open-access article distributed under the terms of the Creative Commons Attribution License (CC BY). The use, distribution or reproduction in other forums is permitted, provided the original author(s) and the copyright owner are credited and that the original publication in this journal is cited, in accordance with accepted academic practice. No use, distribution or reproduction is permitted which does not comply with these terms.
*Correspondence: Eva Nováková, bm92YWV2YUBwYXJ1LmNhcy5jeg==