- 1Shandong Provincial Engineering and Technology Research Center for Wild Plant Resources Development and Application of Yellow River Delta, College of Biological and Environmental Engineering, Binzhou University, Binzhou, China
- 2Shandong Key Laboratory of Eco-Environmental Science for the Yellow River Delta, Binzhou University, Binzhou, China
- 3Department of Bioengineering, Binzhou Vocational College, Binzhou, China
- 4State Key Laboratory of Microbial Metabolism, School of Life Sciences and Biotechnology, Shanghai Jiao Tong University, Shanghai, China
Bacterial endophytes with the capacity to degrade petroleum hydrocarbons and promote plant growth may facilitate phytoremediation for the removal of petroleum hydrocarbons from contaminated soils. A hydrocarbon-degrading, biosurfactant-producing, and plant-growth-promoting endophytic bacterium, Pseudomonas aeruginosa L10, was isolated from the roots of a reed, Phragmites australis, in the Yellow River Delta, Shandong, China. P. aeruginosa L10 efficiently degraded C10–C26 n-alkanes from diesel oil, as well as common polycyclic aromatic hydrocarbons (PAHs) such as naphthalene, phenanthrene, and pyrene. In addition, P. aeruginosa L10 could produce biosurfactant, which was confirmed by the oil spreading method, and surface tension determination of inocula. Moreover, P. aeruginosa L10 had plant growth-stimulating attributes, including siderophore and indole-3-acetic acid (IAA) release, along with 1-aminocyclopropane-1-carboxylic (ACC) deaminase activity. To explore the mechanisms underlying the phenotypic traits of endophytic P. aeruginosa L10, we sequenced its complete genome. From the genome, we identified genes related to petroleum hydrocarbon degradation, such as putative genes encoding monooxygenase, dioxygenase, alcohol dehydrogenase, and aldehyde dehydrogenase. Genome annotation revealed that P. aeruginosa L10 contained a gene cluster involved in the biosynthesis of rhamnolipids, rhlABRI, which should be responsible for the observed biosurfactant activity. We also identified two clusters of genes involved in the biosynthesis of siderophore (pvcABCD and pchABCDREFG). The genome also harbored tryptophan biosynthetic genes (trpAB, trpDC, trpE, trpF, and trpG) that are responsible for IAA synthesis. Moreover, the genome contained the ACC deaminase gene essential for ACC deaminase activity. This study will facilitate applications of endophytic P. aeruginosa L10 to phytoremediation by advancing the understanding of hydrocarbon degradation, biosurfactant synthesis, and mutualistic interactions between endophytes and host plants.
Introduction
Soil contaminated by petroleum hydrocarbons has posed a serious threat to human health and ecological security, and represents a serious environmental problem (Bortey-Sam et al., 2014; Shi et al., 2015). Compared with the chemical and physical remediation technologies, bioremediation strategies are regarded as the cost-effective and sustainable alternatives for soil remediation (Chen et al., 2015). Among bioremediation strategies, phytoremediation, or utilizing growing plants to reduce the concentrations of organic and inorganic pollutants in soil, is a promising technology (Truu et al., 2015). However, the presence of organic pollutants including petroleum hydrocarbons in soil actually decreases plant growth (Gerhardt et al., 2009), and survival of plants becomes tough and then its overall health under pollutant stress is impaired (Mishra et al., 2017). The combined use of plants and bacteria, based on the synergistic activities of plants and their associated microbes, has been recently proposed to enhance the efficiency of remediation of soil contaminated with organic pollutants (Berg, 2009; Arslan et al., 2017). In the plant-microbe-based remediation system, plants provide residency and nutrients to their associated endophytic and rhizosphere bacteria (Arslan et al., 2017). In return, the bacteria support plant growth by the degradation and detoxification of hydrocarbons (Tara et al., 2014). Moreover, they improve plant growth and health due to their innate plant growth-promoting mechanisms (Pawlik et al., 2017).
Recently, plant–endophyte partnership has gained popularity compared to other mechanisms of bioremediation (Zhu et al., 2014). Host plants may take up hydrocarbons from the soils and translocate in their different tissues in which endophytic bacteria reside (Chhikara et al., 2010). A major advantage of endophytic bacteria over rhizobacteria or soil bacteria is that they reside in internal tissues of the host plant and hence have less competition for nutrients and space (Doty, 2008). Endophytic bacteria have a greater capacity to enhance petroleum hydrocarbon phytoremediation than rhizosphere or soil bacteria (Barac et al., 2004; Doty, 2008; Weyens et al., 2010; Balseiro-Romero et al., 2016). However, insufficient knowledge of the mechanism underlying the interactions between plants and hydrocarbon-degrading and/or plant-growth-promoting endophytic bacteria (Feng et al., 2017), along with a lack of genetic data, has hampered the application of endophytes in phytoremediation. The application of high-throughput sequencing techniques and functional genomics has been providing a new avenue for a deeper understanding of the interactions between microbes and their plant hosts.
Therefore, in the present study, a hydrocarbon-degrading, biosurfactant-producing, plant-growth-promoting, and endophytic Pseudomonas aeruginosa L10 was isolated from the roots of a reed (Phragmites australis) that grows in high-salinity oil-polluted areas of the Yellow River Delta, Shandong, China. To the best of our knowledge, there is no previous report of an endophytic P. aeruginosa isolated from a halophytic reed with the characteristics of hydrocarbon degradation, biosurfactant production, and plant growth promotion. In order to gain a deeper understanding of the genetic mechanisms of degrading petroleum hydrocarbons, producing biosurfactants, and promoting plant growth, we sequenced the complete genome of the endophytic P. aeruginosa L10. We identified genes related to petroleum hydrocarbon degradation, biosurfactant synthesis, and plant growth promotion.
Materials and Methods
Sampling and Isolation of Endophytic Bacteria
Healthy plant samples of reeds (Phragmites australis) were collected from region of Shengli Oilfield (the second largest oil-producing base of China), located in the Yellow River Delta, China (N 37°47′05.9″, E 118°39′28.3). This area is characterized by saline-alkaline soils (Gao et al., 2015) and the collected halobiotic reeds grow in salinity areas polluted by oil. Each reed sample was immediately placed in a sterile polyethylene bag, then transported to the laboratory in an ice cooler. Plant samples were treated immediately when they were transported to laboratory.
Reeds were washed with running water for 30 min, and then rinsed with distilled water three times (3 min per wash). After absorbing moisture from the plant surface, the plants were sectioned into roots, stems, and leaves. The plant parts were first surface-sterilized by soaking each plant component in 70% ethanol solution for 2 min, then washed with sterile water three times. Moreover, each plant part was immersed and shaken in 1.2% sodium hypochlorite solution (NaClO) for 30 min, and then washed with sterile water three times (2 min per wash). The last sterile water rinse (100 μL) was plated onto Luria-Bertani (LB) agar medium and incubated for 24 h (at 30°C) in order to test the efficacy of disinfection for each plant part surface (Dai et al., 2016).
Ten volumes of phosphate buffer were added to the sterilized plant tissue. After grinding the tissue completely and letting it stand for 5 min, the suspension was placed on mineral salts (MS) (Mueller et al., 1989) agar plates, supplemented with diesel oil (5 g/L) as the sole source of carbon and energy, and incubated at 30°C for 4 days. Diesel oil was chosen as carbon source because it is a mixture of different hydrocarbon pollutants that have been frequently reported as environmental contaminants (Gallego et al., 2001). The bacterial colonies were selected, sub-cultured, purified, and used for further studies.
Screening for Effective Hydrocarbon-Degrading and Plant-Growth-Promoting Endophytic Bacteria
Effective hydrocarbon-degrading bacteria were selected for their capacity to degrade diesel oil in liquid medium. Plant-growth-promoting bacteria were selected for their capacity to produce 1-aminocyclopropane-1-carboxylate (ACC) deaminase, siderophore, as well as indole-3-acetic acid (IAA).
Evaluation of Diesel Oil-Degrading Ability
Ability of isolates to degrade diesel oil was investigated according to a modified method described by Zhang et al. (2010). Diesel oil was sterilized using a 0.22 μm organic filter and added to MS liquid medium to a final concentration of 5 g/L. Bacterial suspensions (MS liquid medium, ∼1 × 108 CFU/mL) of a single strain were added at a 5% inoculum size to the medium, and incubated for 7 days at 30°C, with shaking at 200 revolutions/min (rpm). After incubation, the culture broth was extracted twice with n-hexane. All extracts were dehydrated with anhydrous sodium sulfate and evaporated to a final volume of 2 mL with a gentle nitrogen stream in a fume hood. The total petroleum hydrocarbons (TPHs) were quantified by a gas chromatographer with flame ionization detection (GC-FID, Agilent 7890A, United States). A control experiment was performed by inoculating with the boiled cells of isolate. The parameters for the chromatographer were as follows: an Agilent HP-5 GC column was used, with the dimensions 30 m × 0.25 mm × 0.25 μm; helium was used as the carrier gas; the inlet temperature and detector temperature were 290°C and 300°C, respectively; the column oven temperature was maintained at 50°C for 2 min, then increased to 300°C at a rate of 6°C per min, and held at 300°C for 16 min. The total area of detected hydrocarbon peaks was defined as the concentration of TPHs. The degradation ratio of TPHs of diesel oil was calculated according to the formula (1):
where D refers to the degradation rate of diesel in the medium; C0 refers to the content of residual diesel in the medium inoculated with the boiled cells of isolate; and Ci refers to the content of residual diesel in the medium inoculated with the strain. Three replicates were performed for these experiments, and the results are reported as means and standard deviations.
For identification of n-alkane peaks in the diesel oil gas chromatogram, a standard mixture of n-alkanes (n-C8 to n-C40) was used as the external standards.
Measurement of ACC Deaminase Activity
The ACC deaminase activity of bacterial endophytes was screened based on the ability to use ACC as a sole nitrogen source. The ACC deaminase activity was screened according to Penrose and Glick (2003) using the nitrogen-free DF salts minimal agar medium. The solid medium was supplemented with either 2 g/L (NH4)2SO4 or 3 mM ACC as sole nitrogen source, and incubated aerobically. ACC deaminase activity of cell-free extracts was quantitatively determined by measuring the production of alpha-ketobutyrate (alpha-KB) that is generated by the cleavage of ACC by ACC deaminase. After determining the amount of protein and alpha-KB, the enzyme activity was expressed as micromoles of alpha-KB per mg of protein per hour.
Assay for Siderophore Production
Bacterial endophytes were assayed for siderophores production on chrome azurol S (CAS) agar plate described by Machuca and Milagres (2003). Chrome azurol S agar plates were prepared and divided into equal sectors, spot inoculated with test organism (10 mL of 108 CFU/mL), and incubated at 30°C for 48 h. Development of yellow-orange halo around the growth was considered as positive for siderophore production. By measuring and comparing the diameters of yellow–orange halos, endophytes with the function of siderophore production were screened.
Assay of IAA Production
The IAA production of the bacterial endophyte was determined according to Sarwar and Kremer (1995) using a microplate method. Briefly, 150 μL of culture filtrate were dispensed into wells of 96-well microplates followed by addition of 100 μL of Salkowski reagent, allowed to react 30 min, and color intensity measured at 530 nm on a microplate reader. The amount of IAA produced was quantified by comparing with the standard curve prepared with known concentrations of IAA. Each experiment consisted of microplates prepared with newly dispensed reagents and filtrates. Comparative experiments were then repeated twice.
Detection of Biosurfactant Production
Biosurfactant production was detected by the oil spreading method (Anandaraj and Thivakaran, 2010) and surface tension determination of inocula (Zhu et al., 2014). The screened strains above were cultured in MS liquid medium (glucose as carbon source and urea as nitrogen source) on rotary shaker (200 rpm) at 30°C for 2 days. The supernatant was used to determine the diameter of oil spreading and its surface tension. Mineral salt medium without strains, under the same procedure, was used as the control sample (CK). Surface tension was measured in the cell free supernatant using a K100 tensiometer (Kruss GmbH, Hamburg, Germany), following the du Noüy ring method (Górna et al., 2011). Each experiment was repeated thrice, and interpretations were made using the averages of the triplicate measurements.
Assay for Polycyclic Aromatic Hydrocarbon (PAH) Biodegradation
The ability of endophytic isolates to degrade PAHs was also tested. Cell suspensions (MS liquid medium, ∼ 1 × 108 CFU/mL) of tested bacteria were added at a 5% inoculum volume in 100 mL MS (pH 7.4) supplemented with naphthalene, phenanthrene, or pyrene, respectively. The final concentration of PAH was 200 mg/L. The cultures were incubated at 30°C in a shaker at 200 rpm and extracted after 10 days of incubation. All samples were extracted and analyzed using gas chromatography, according to the methods described above (Zhang et al., 2010).
Pot Trial Experiment for Assessment of Plant Growth Promotion of Endophytes
The pot trial experiment had two treatments (with and without endophytic inoculation) each with six replicates having seven plantlets in each pot and arranged in a randomized manner. The soil used in the pot trial experiment was treated by the addition of crude oil with the final TPH concentration as 10,527 mg/kg. Overnight bacterial cultures were harvested by centrifugation (6000 × g, 5 min). Pellets were washed three times, and suspended in 1/2 strength Hoagland’s solution (Sionit et al., 1981) with cell density as 105–106 CFU/mL. For the colonization of tested endophytes in the plantlets, the plantlets were cultured in 1/2 strength Hoagland’s solution for 7 days. The uniform sized (plant height 10–13 cm) reed plantlets (Phragmites australis), both inoculated and uninoculated treatments, were transferred into pottery pots (diameter of 38 cm and depth of 29 cm). Each pottery pot contained 15 kg of sterilized soil. The plantlets were watered every other day. The plants were harvested after 120 days and biomasses (dry weights) of plants from different treatments were measured. Meanwhile, petroleum residues in soil samples were extracted with methylene chloride and the amount of residual TPH was determined gravimetrically (Wu et al., 2012).
Genome Sequencing, Assembly, and Annotation
In order to more pertinently understand the genetic mechanisms of endophytes to degrade petroleum hydrocarbons, produce biosurfactants, and promote plant growth, we selected one of the most promising endophytic strains. Subsequently, complete genome sequencing of the endophytic bacterium was performed. The genomic DNA of this endophytic strain was isolated from the overnight culture using the method described by Pitcher et al. (1989). The genome of the strain was sequenced using an Illumina HiSeq 4000 sequencing platform, and a PacBio RSII sequencing platform. Sequencing was performed at BGI-Shenzhen, Shenzhen, China. HiSeq raw and PacBio raw data were used to de novo assemble the bacterial genome. Gene prediction and annotation were performed on the genome by both the BGI-Shenzhen system and the NCBI Prokaryotic Genome Annotation Pipeline. Predicted proteins were assigned Clusters of Orthologous Groups numbers (Tatusov et al., 2000) and Protein Families domains by querying their sequences against the COG Database and the Pfam database, respectively.
Genomic Comparative Analysis
We retrieved 100 completely sequenced P. aeruginosa genomes from NCBI Genome (last accessed in March 2017) and compared them to the L10 genome sequenced in the present study. Single nucleotide polymorphism (SNP) calling and phylogenetic analysis was performed using kSNP3.0 (Gardner et al., 2015). The kSNP-generated maximum-likelihood tree was visualized using iTOL v3 (Letunic and Bork, 2016). A dot plot comparison of the genomes of P. aeruginosa L10 and the reference strain, PAO1, was performed. Nucleotide-based alignments were performed with MUMmer version 3.23 (Kurtz et al., 2004). The dot plots were generated with ‘mummerplot’ and ‘gnuplot’ from the MUMmer package, version 3.23.
Nucleotide Sequence Accession Number
The genome sequence of P. aeruginosa L10 has been submitted to GenBank under accession number CP019338.
Results
Isolation and Characterization of Endophytic Isolate L10
Nineteen endophytic isolates were obtained from the roots, leaves, and stems of P. australis. Isolate L10, which we refer to as P. aeruginosa L10, was selected for this study because of its excellent hydrocarbon degradation, biosurfactant synthesis, and plant growth-stimulating attributes. Pseudomonas aeruginosa L10 is a Gram-negative, rod-shaped bacterium (Figure 1A) that forms circular and smooth colonies (Figure 1B) on LB agar medium. Pseudomonas aeruginosa L10 had a strong ability to degrade TPHs in diesel oil, with a degradation rate of 79.3% after 7 days of incubation in MS liquid medium supplemented with 5 g/L of diesel oil. By comparing the area peak of individual n-alkane from gas chromatograms (Figure 2), we also found that endophytic isolate L10 could degrade the C10–C26 n-alkanes of the diesel oil. In addition, P. aeruginosa L10 had a strong ability to degrade the common PAHs naphthalene, phenanthrene, and pyrene, with degradation rates of 79.7, 71.6, and 34.7%, respectively, after 10 days of incubation in MS medium with 200 mg/L of PAH. Moreover, an oil spreading assay and surface tension measurements of inocula indicated that P. aeruginosa L10 had the ability to produce biosurfactant. Diameters of the clearing zones on the oil surface obtained from the oil spreading assay with cell free supernatant of P. aeruginosa L10 were measured up to ∼80 mm (Figure 3A). However, no clearing zone with only medium was observed. Pseudomonas aeruginosa L10 could decrease the surface tension from 70.7 mN/m to 29.5 mN/m (Figure 3B) in the cell free supernatant.
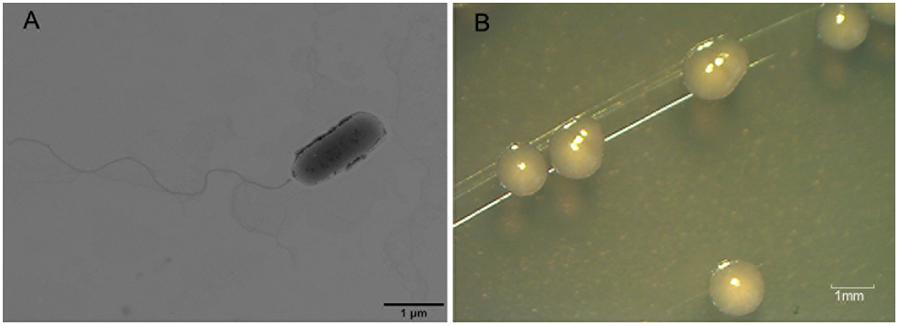
FIGURE 1. Transmission electron microscopy (TEM) image of endophytic bacterium L10 and its colonies on LB agar medium. (A) Transmission electron microscopy (TEM) image of rod-shaped endophytic isolate L10. (B) The circular and smooth colonies of endophytic bacterium L10 on LB agar medium.
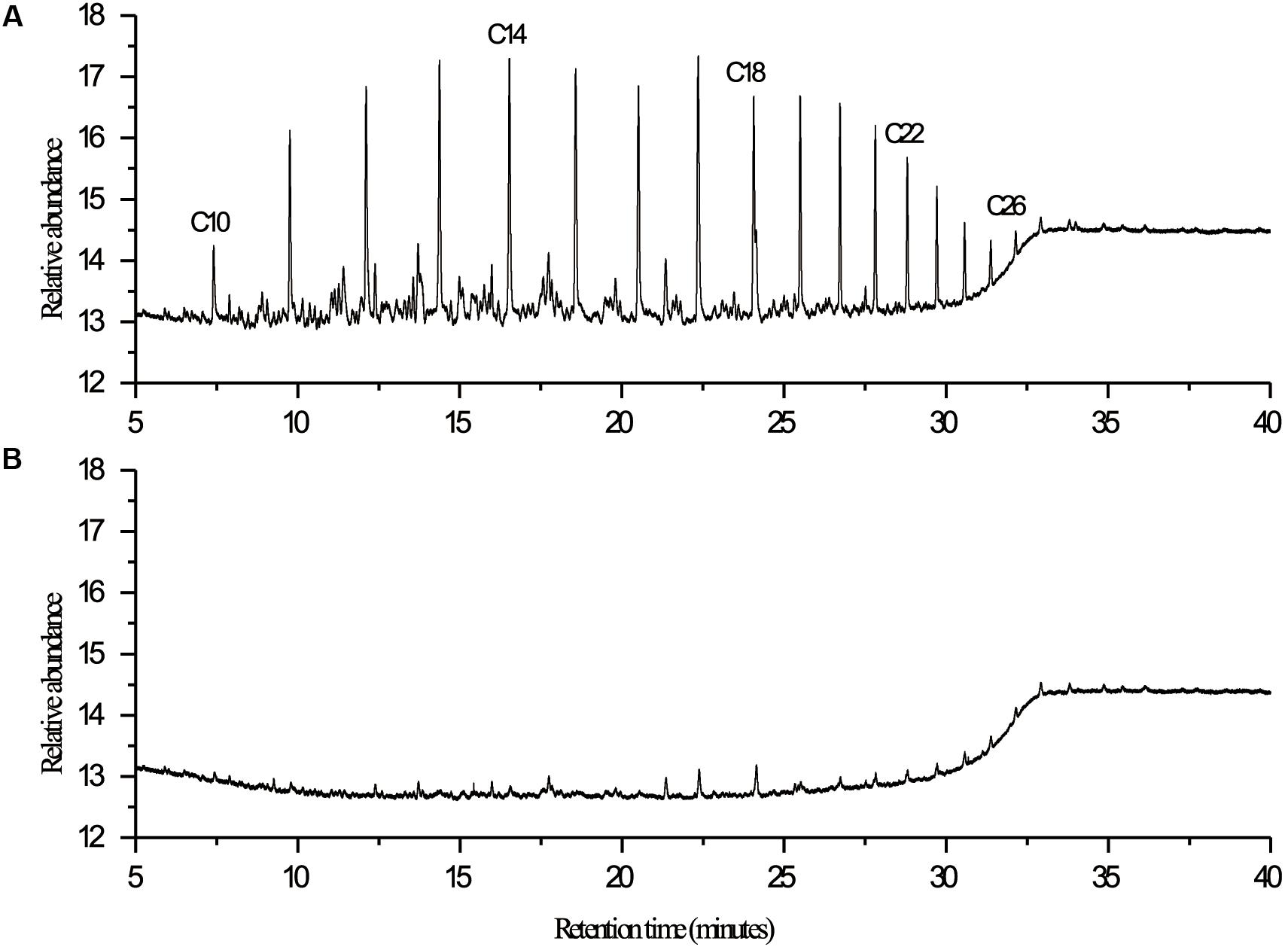
FIGURE 2. GC-FID chromatograms of diesel oil extracted at 7th day from (A) control and (B) experimental flask in biodegradation study with endophytic isolate L10. Degradation of diesel oil was carried out under aerobic conditions at 30°C in 300-mL Erlenmeyer flasks containing 100 mL of MS medium and 5 g/L of diesel oil. Control was performed by inoculating with the boiled cells of isolate. Hydrocarbons were extracted from the culture medium with an equal volume of n-hexane, analyzed using a gas chromatograph equipped with an FID detector (GC-FID, 7890A, Agilent, United States), and quantified in comparison to the n-alkane homologous series.
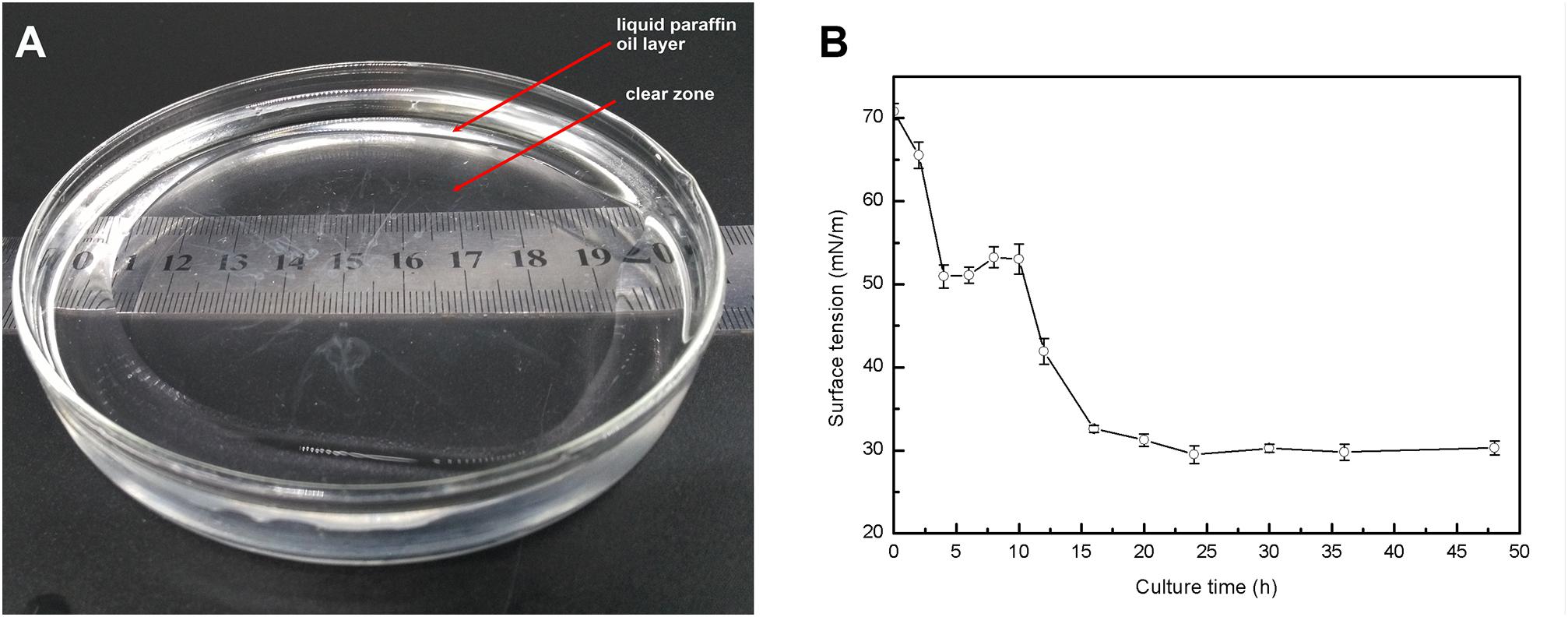
FIGURE 3. Production of biosurfactant by P. aeruginosa L10. (A) Oil displacement induced by the fermentation broth of a 48-h-old culture of strain L10 was measured using the oil spreading technique. (B) Surface tension of the fermentation broth as a function of time. Error bars indicate the standard deviations of three replicates.
We found that P. aeruginosa L10 has plant growth stimulating attributes, including siderophore and indole-3-acetic acid (IAA) production, along with 1-aminocyclopropane-1-carboxylic (ACC) deaminase activity. To quantify siderophore yield, we measured orange halo zone diameters in CAS medium 48 h post-inoculation. Siderophore zone diameters at 48 h were 4.1–4.5 cm. After a 72-h incubation period, the amount of IAA production by isolate L10 was 26.8 ± 7.4 mg/mL, as measured by the microplate method. Pseudomonas aeruginosa L10 could grow on DF minimal salt medium with ACC serving as the sole nitrogen source, indicating its ACC deaminase activity. The ACC deaminase enzyme activity of L10 was measured in bacterial extracts by quantifying the amount of alpha-KB produced during the deamination of ACC. The ACC deaminase activity of strain L10 was detected as 7496 ± 261 nmol alpha-KB/mg Pr⋅h.
Moreover, pot experiments were carried out to verify the effect of P. aeruginosa L10 on plant growth promotion. After 120 days of growth, the reed plants were harvested and the biomass above and below the ground (dry weight) was measured. We found that aerial and underground biomass of inoculated plants was significantly higher than that of non-inoculated plants (P < 0.01); aerial biomass was elevated by 77.9%, and underground biomass was elevated by 89.4% (Figure 4), indicating that P. aeruginosa L10 can significantly promote reed growth both above and below the ground. Moreover, the soil TPH degradation rate in phytoremediation incubated with P. aeruginosa L10 was 58.9%, elevated by 21.7% over phytoremediation without incubation (37.2%).
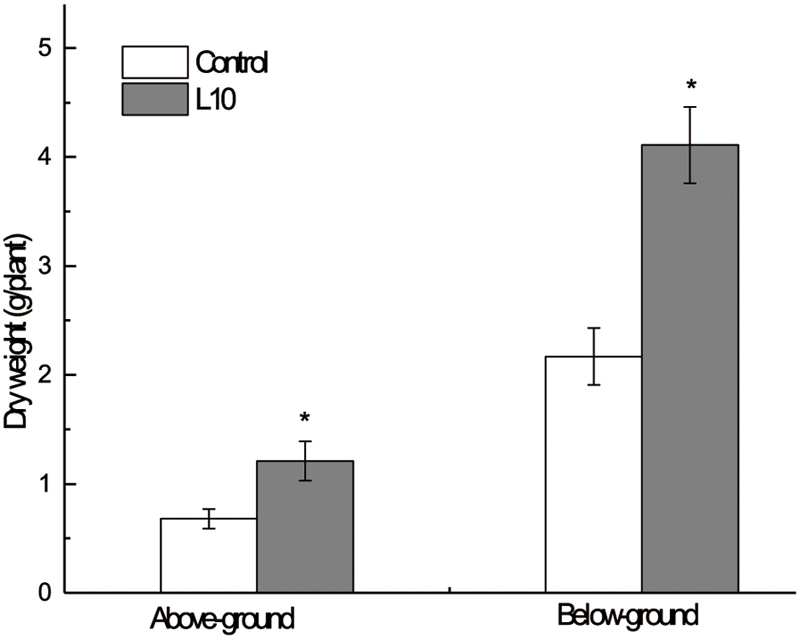
FIGURE 4. Effect of endophytic isolate L10 on the growth of the Phragmites australis. Bars indicate standard deviation (n = 6). Dried mass of inoculated plants was significantly greater (Student’s t-test; P < 0.05).
Complete Genome Sequencing of Endophytic P. aeruginosa L10
We sequenced the complete genome of isolate L10 and used this sequence to identify the isolate as Pseudomonas aeruginosa. The genome of P. aeruginosa L10 had one 6,661,962 bp circular chromosome (66.1% GC content; Table 1). NCBI’s Prokaryotic Genome Annotation Pipeline predicted 6,137 protein-coding genes, 12 rRNA genes (encoding four 23S rRNAs, four 16S rRNAs, and four 5S rRNAs), 62 tRNA genes, and 5 ncRNA genes, from a total of 6,307 genes (Figure 5 and Table 1). The rRNA genes were arranged in four operons with the typical 16S-23S-5S rRNA pattern. Genes were affiliated to COG- and Pfam-based functions were 79.66 and 83.57%, respectively. Supplementary Table S1 shows the distribution of genes across their COG functional categories.
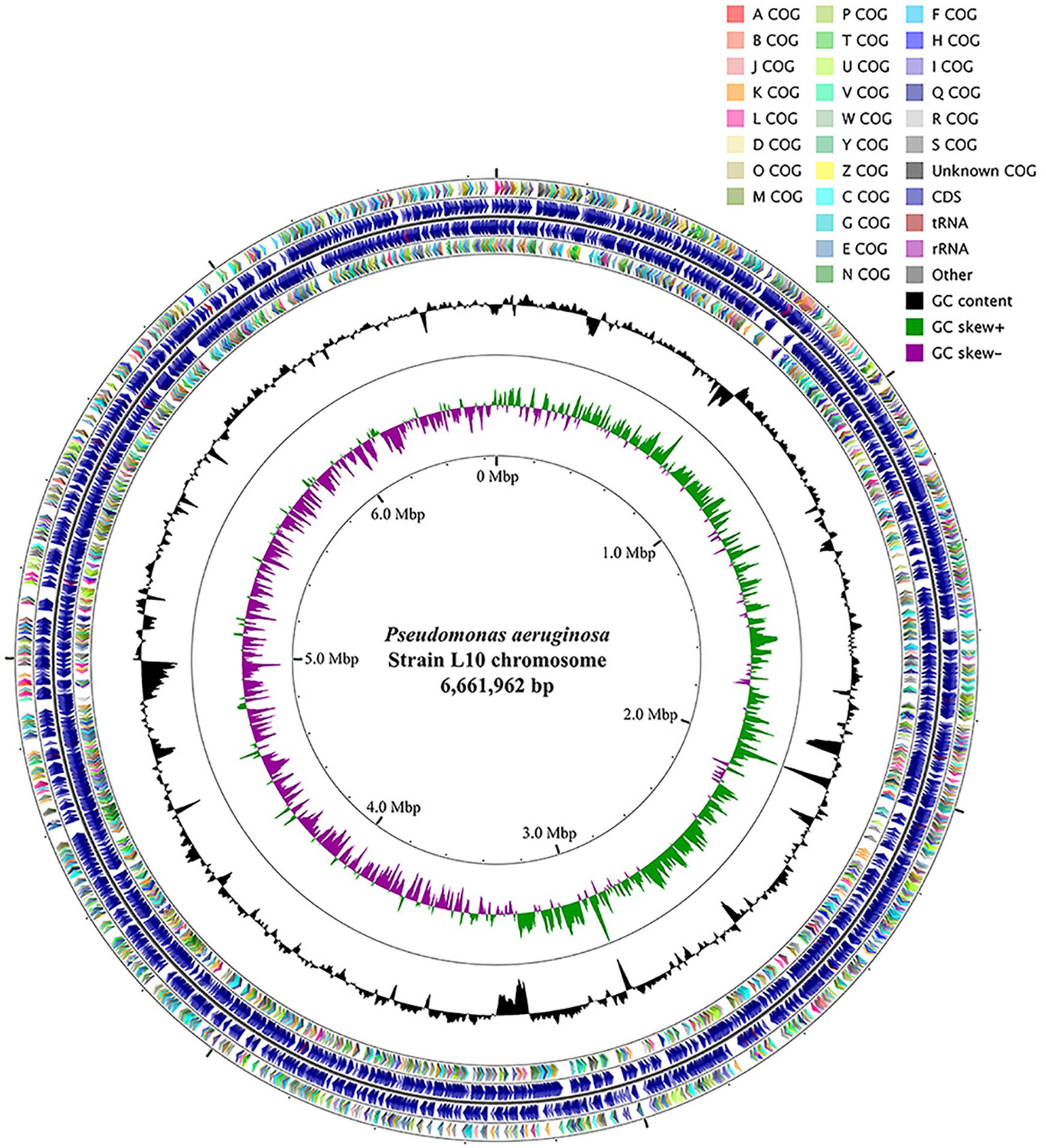
FIGURE 5. Circular genome map of P. aeruginosa L10. From outside to center, ring 1 and 4 show protein-coding genes oriented in the forward (colored by COG categories) and reverse (colored by COG categories) directions, respectively. ring 2 and 3 denote genes on forward/reverse strand; ring 5 shows G + C% content plot, and the inner-most ring shows GC skew, purple indicating negative values and olive, positive values. The circular map was generated with GCViewer (https://github.com/chewiebug/GCViewer, last accessed January 2017).
Functional Genomics of Endophytic P. aeruginosa L10
Genome annotation suggested the presence of genes related to hydrocarbon degradation, including genes encoding monooxygenase, dioxygenase, alcohol dehydrogenase, and aldehyde dehydrogenase. Genes related to the n-alkane degradation pathway were detected, such as genes encoding alkane 1-monooxygenase (BWR11_13240, BWR11_19485), 9 genes encoding aldehyde dehydrogenases, 5 genes encoding acyl-CoA synthetases, genes coding for rubredoxin reductase (BWR11_30355) and rubredoxin-NAD+ reductase (BWR11_30 < 360), genes coding for Rubredoxin-1 (BWR11_30370) and Rubredoxin-2 (BWR11_30365), 7 genes encoding methyl-accepting chemotaxis protein, and 7 genes coding for alcohol dehydrogenases (ADH). We also used AromaDeg1 (last accessed in May 2017) to detect genes putatively associated with the degradation of aromatic compounds. Five candidates were indicated: (1) three belonging to the benzoate family; (2) one belonging to the homoprotocatechuate family in the LigB superfamily; and (3) one belonging to the gentisate family.
A series of genes or gene clusters that may be involved in important biological functions were detected in the genome of endophytic P. aeruginosa L10. The genome annotation identified a potential rhamnolipid biosynthesis gene cluster, rhlABRI (BWR11_07880 to BWR11_07895). Genome annotation also indicated the presence of an ACC deaminase gene (BWR11_10155). In addition, we also found seven tryptophan biosynthetic genes clustered at various chromosomal locations, including trpAB (BWR11_00180 and BWR11_00185), trpDC (BWR11_03465 and BWR11_03470), trpE (two copies: BWR11_22230 and BWR11_03280), trpF (BWR11_10025), and trpG (two copies: BWR11_03460 and BWR11_22225). Moreover, we detected two clusters of genes involved in the biosynthesis of siderophore, pvcABCD (pyoverdine siderophore; BWR11_15175 to BWR11_15190) and pchABCDREFG (pyochelin siderophore; BWR11_03850 to BWR11_03885).
Phylogenetic Analysis of P. aeruginosa L10 and Other P. aeruginosa Strains
We used kSNP3.0 and the complete genomes of 101 P. aeruginosa strains (the genome of strain L10 and 100 publicly available genomes) to construct a phylogenetic tree; we visualized the tree using the iTOL software. Phylogenetic analysis of the 101 P. aeruginosa strains grouped strain L10 in a clade with seven other P. aeruginosa strains (B10W, Cu1510, IOMTU_133, M1608, M37351, UCBPP-PA14, and PA14OR; Figure 6). Alignment of the genomes of strain L10 and the reference strain PA01 (GenBank Accession No. NC_002516.2) using MUMmer 3.23 indicated relatively high sequence similarity (coverage 90%, similarity 99%; Supplementary Figure S1).
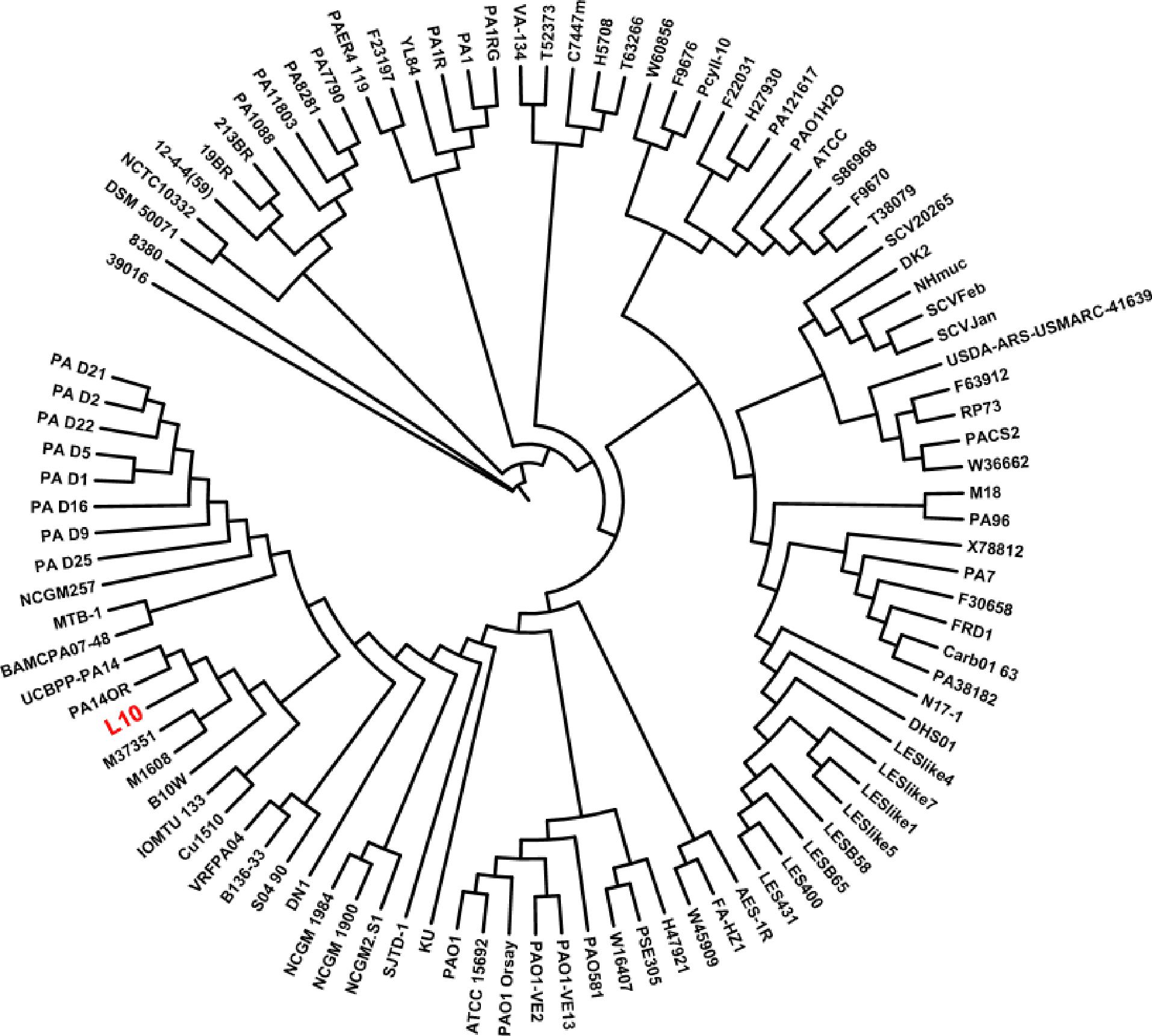
FIGURE 6. Phylogeny based on core SNPs of 101 strains of P. aeruginosa. We constructed the phylogenetic tree using kSNP3.0, and visualized it using iTOL. P. aeruginosa L10 is highlighted in red.
Discussion
Phytoremediation with endophytic microbes is an important new means of improving bioremediation efficacy (Andreolli et al., 2013; Zhang et al., 2014). Isolation and selection of hydrocarbon-degrading and plant-growth-promoting endophytic bacteria from plants growing in contaminated sites could be particularly important for exploiting the remediation potential of plant-endophyte systems. The present study isolated an endophytic P. aeruginosa L10 from reed roots, and found that the isolate degraded hydrocarbons, synthesized biosurfactant, and stimulated plant growth via IAA synthesis, siderophore production, and ACC deaminase activity. Pseudomonas sp. are ubiquitous in soil, water, plants, animals, and humans, and have been studied for their versatile metabolic capacity to induce systemic resistance, promote plant growth, and suppress microbial pathogens in plants (Ma et al., 2017). Until recently, over 20 endophytic Pseudomonas sp. strains (Supplementary Table S2) with some of these functions beneficial to their plant host were isolated from various herbaceous, vine, and woody plants. To the best of our knowledge, strain L10 is the first endophytic P. aeruginosa isolated from the halophytic reed of the Yellow River Delta with the characteristics of hydrocarbon degradation, biosurfactant production, and plant growth promotion.
Alkanes and PAHs are toxic compounds ubiquitous in petroleum (Bakker et al., 2000). Despite their poor water solubility, they can be absorbed and accumulated by plants (Palmroth et al., 2002; Meudec et al., 2006). This study demonstrates that endophytic P. aeruginosa L10 can effectively degrade n-alkanes and PAHs (naphthalene, phenanthrene, and pyrene). Therefore, in this case, endophytic P. aeruginosa L10 may directly detoxify hydrocarbons in plants. Some studies demonstrated that endophytes can improve biodegradation by reducing the amount of contaminants in the growth substrate, as well as through reduction of evapotranspiration of contaminants (Barac et al., 2004; Germaine et al., 2009). Conversely, halobiotic reed is one of the most dominant plant species in the saline area of the Yellow River Delta, China (Wang et al., 2012). This plant species has been chronically exposed to petroleum hydrocarbons in the Yellow River Delta since Shengli Oilfield (the second largest oil-producing base of China and lies in the Yellow River Delta) exploitation began in 1964 (Nie et al., 2009) and might have adapted itself to the petroleum-polluted saline soils. Early reports indicated that reed had high potential for phytoremediation of petroleum pollution in Yellow River Delta (Wang et al., 2011). This study demonstrates P. aeruginosa L10 could increase the biomass of its host reed; the association between halophytic reed and P. aeruginosa L10 exerted a significant effect on TPH removal from crude oil-contaminated soils, compared to uninoculated plants. These results suggest an immense bioremediation potential for P. aeruginosa L10.
An important limiting factor on hydrocarbon biodegradation is the low bioavailability of these compounds. Hydrocarbon-degrading microbes might produce biosurfactant to overcome this problem (Kukla et al., 2014). In the present study, evidence from both an oil spreading assay and surface tension measurements of inocula indicated that endophytic P. aeruginosa L10 has the ability to produce biosurfactant. Kukla et al. (2014) also observed a number of Pseudomonas sp. in the endophytic community of Lolium perenne. Biosurfactants released by bacteria can significantly increase solubility of hydrocarbons, it is likely that plant uptake of hydrocarbons at the phytoremediation contaminated soil was relatively high. High uptake of hydrocarbons by plants would result in high selective pressure on endophytic bacterial communities, and degrader communities would become well established (Phillips et al., 2008).
The endophyte P. aeruginosa L10 produces siderophore, IAA, and ACC deaminase. Microbes with these features may promote plant growth (Rodriguez et al., 2008). Siderophore and IAA were found capable of stimulating plant biomass (Zhang et al., 2011), and the ACC deaminase has been proposed to play a key role in microbe–plant association (Hontzeas et al., 2004).
We sequenced the complete genome of endophytic P. aeruginosa L10, using high-throughput sequencing, to better understand the genetic bases of its functions (hydrocarbon degradation, biosurfactant synthesis, and plant growth promotion). A deeper understanding of the interaction between endophytic P. aeruginosa L10 and its plant host (Phragmites australis) could be achieved through the functional genomic analysis of the endophyte; this understanding will benefit the application of endophytic bacteria to phytoremediation.
Endophytic P. aeruginosa L10 was an efficacious hydrocarbon-degrading bacterium, which was detected degrade the C10–C26 n-alkanes of the diesel oil effectively, and degradation rate of TPHs was measured up to 79.3% after 7 days of incubation. Complete genomic sequence of P. aeruginosa L10 was detected to be abundant in genes involved in n-alkane degradation, including genes encoding alkane 1-monooxygenase, rubredoxin, rubredoxin reductase, alcohol dehydrogenase, aldehyde dehydrogenase, and acyl-CoA synthetase. The medium- and long-chain n-alkanes could be oxidized by alkane 1-monooxygenase (alkane hydroxylase) with the help of two soluble electron transfer proteins (a dinuclear iron rubredoxin and a mononuclear iron rubredoxin reductase). The terminal oxidation of alkanes by alkane hydroxylases generated primary fatty alcohols (Smits et al., 2003), which are further oxidized to aldehydes by an alcohol dehydrogenases (ADH) (Rojo, 2009). The aldehydes then were converted to carboxylic acids by aldehyde dehydrogenases (Van Beilen and Funhoff, 2007). The carboxylic acids then served as substrates for acyl-CoA synthetase, and the resulting acyl-CoA entered the β-oxidation pathway (Wentzel et al., 2007). We found a substantial number of genes encoding putative enzymes characteristic of β-oxidation, including acyl-CoA dehydrogenase (12 genes) and enoyl-CoA hydratase (14 genes). Moreover, we also detected genes putatively associated with the degradation of aromatic compounds in the complete genome of endophytic P. aeruginosa L10. The aromatic compounds, particularly PAHs (e.g., naphthalene, phenanthrene, and pyrene), are more toxic than aliphatic compounds to organismal and ecological security (Harayama et al., 1999). Our PAH biodegradation assay showed that P. aeruginosa L10 had a strong ability to degrade naphthalene, phenanthrene, and pyrene. The effective degradation of aromatic compounds of endophytic P. aeruginosa L10 indicated its broad application prospect in practice of phytoremediation.
Genome annotation suggested a potential rhamnolipid biosynthesis gene cluster in the genome, rhlABRI (BWR11_07880 to BWR11_07895), which would be responsible for the biosurfactant synthesis. In the rhamnolipid pathway, the rhlAB genes (BWR11_07880 and BWR11_07885; functioning as one operon) encode rhamnosyltransferase 1, while rhlR (BWR11_07890) and rhlI (BWR11_07895) are arranged in sequence and act as regulators of rhlAB expression (Lang and Wullbrandt, 1999). Rhamnolipid biosurfactants are surface-active compounds, produced by P. aeruginosa, that enhance the emulsification of hydrocarbons, increasing their solubility and their availability for microbial degradation (Rahman et al., 2002). We suggest that, in P. aeruginosa L10, the rhamnolipid rhlABRI gene cluster produces biosurfactants that promote the degradation of hydrocarbons.
We find that the endophyte P. aeruginosa L10 has plant growth-stimulating attributes, including siderophore and IAA release, along with ACC deaminase activity. Genome annotation identified two clusters of genes involved in the biosynthesis of siderophore: pvcABCD (BWR11_15175 to BWR11_15190), and pchABCDREFG (BWR11_03850 to BWR11_03885). The pvcABCD operon of P. aeruginosa encoded four proteins (PA2254, PA2255, PA2256, and PA2257) which were identified as being responsible for a step in the maturation of the chromophore of the peptide siderophore pyoverdine (Drake and Gulick, 2008). Expression of the pchABCDREFG operon was required for the synthesis of the pyochelin siderophore (Lee et al., 2013). In addition, genome annotation indicated seven tryptophan biosynthetic genes clustered at various chromosomal locations, including trpAB (BWR11_00180 and BWR11_00185), trpDC (BWR11_03465 and BWR11_03470), trpE (two copies: BWR11_22230 and BWR11_03280), trpF (BWR11_10025), and trpG (two copies: BWR11_03460 and BWR11_22225). Tryptophan biosynthetic genes are involved in IAA synthesis (Ge et al., 2006), which should be the mechanism of IAA biosynthesis by the endophytic P. aeruginosa L10. Genome annotation also indicated an ACC deaminase gene (BWR11_10155) in the P. aeruginosa L10 genome. ACC deaminase activity in plant-associated bacteria significantly enhanced nutrient uptake (Safronova et al., 2006) and increased plant biomass (Belimov et al., 2009), consistent with our finding that P. aeruginosa L10 increased plant growth.
Conclusion
The endophyte P. aeruginosa L10 degrades petroleum hydrocarbons, produces biosurfactants, and promotes plant growth of Phragmites australis. This study used high-throughput sequencing and functional genomics to elucidate the mechanisms of hydrocarbon degradation, biosurfactant synthesis, and plant growth promotion by endophytic P. aeruginosa L10. The complete genome we have here presented will serve as a genetic basis for further work on this endophytic bacterial strain and will thus be of considerable value for further studies of the industrial and environmental applications of P. aeruginosa L10.
Author Contributions
TW designed the experiments. TW, JX, WX, and HY performed the experiments. TW and XL analyzed the data and wrote the manuscript. ZY and CS provided technical assistance to TW. All the authors read and approved the final manuscript.
Funding
The work was funded by the National Natural Science Foundation of China (41401359), a project of Shandong Province Higher Educational Science and Technology Program (J14LE05), Natural Science Foundation of Shandong Province (ZR2013DM001), and China Postdoctoral Science Foundation (2013M541506).
Conflict of Interest Statement
The authors declare that the research was conducted in the absence of any commercial or financial relationships that could be construed as a potential conflict of interest.
Acknowledgments
We would like to thank Editage (www.editage.cn) for English language editing.
Supplementary Material
The Supplementary Material for this article can be found online at: https://www.frontiersin.org/articles/10.3389/fmicb.2018.01087/full#supplementary-material
Footnotes
References
Anandaraj, B., and Thivakaran, P. (2010). Isolation and production of biosurfactant producing organism from oil spilled soil. J. Biosci. Technol. 1, 120–126.
Andreolli, M., Lampis, S., Poli, M., Gullner, G., Biró, B., and Vallini, G. (2013). Endophytic Burkholderia fungorum, DBT1 can improve phytoremediation efficiency of polycyclic aromatic hydrocarbons. Chemosphere 92, 688–694. doi: 10.1016/j.chemosphere.2013.04.033
Arslan, M., Imran, A., Khan, Q. M., and Afzal, M. (2017). Plant–bacteria partnerships for the remediation of persistent organic pollutants. Environ. Sci. Pollut. Res. Int. 24, 4322–4336. doi: 10.1007/s11356-015-4935-3
Bakker, M. I., Casado, B., Koerselman, J. W., Tolls, J., and Kollöffel, C. (2000). Polycyclic aromatic hydrocarbons in soil and plant samples from the vicinity of an oil refinery. Sci. Total Environ. 263, 91–100. doi: 10.1016/S0048-9697(00)00669-0
Balseiro-Romero, M., Gkorezis, P., Kidd, P. S., Vangronsveld, J., and Monterroso, C. (2016). Enhanced degradation of diesel in the rhizosphere of Lupinus luteus after inoculation with diesel-degrading and plant growth-promoting bacterial strains. J. Environ. Qual. 45, 924–932. doi: 10.2134/jeq2015.09.0465
Barac, T., Taghavi, S., Borremans, B., Provoost, A., Oeyen, L., Colpaert, J. V., et al. (2004). Engineered endophytic bacteria improve phytoremediation of water-soluble, volatile, organic pollutants. Nat. Biotechnol. 22, 583–588. doi: 10.1038/nbt960
Belimov, A. A., Dodd, I. C., Hontzeas, N., Theobald, J. C., Safronova, V. I., and Davies, W. J. (2009). Rhizosphere bacteria containing 1-aminocyclopropane-1-carboxylate deaminase increase yield of plants grown in drying soil via both local and systemic hormone signalling. New Phytol. 181, 413–423. doi: 10.1111/j.1469-8137.2008.02657.x
Berg, G. (2009). Plant–microbe interactions promoting plant growth and health: perspectives for controlled use of microorganisms in agriculture. Appl. Microbiol. Biotechnol. 84, 11–18. doi: 10.1007/s00253-009-2092-7
Bortey-Sam, N., Ikenaka, Y., Nakayama, S. M., Akoto, O., Yohannes, Y. B., Baidoo, E., et al. (2014). Occurrence, distribution, sources and toxic potential of polycyclic aromatic hydrocarbons (PAHs) in surface soils from the Kumasi Metropolis, Ghana. Sci. Total Environ. 496, 471–478. doi: 10.1016/j.scitotenv.2014.07.071
Chen, M., Xu, P., Zeng, G., Yang, C., Huang, D., and Zhang, J. (2015). Bioremediation of soils contaminated with polycyclic aromatic hydrocarbons, petroleum, pesticides, chlorophenols and heavy metals by composting: applications, microbes and future research needs. Biotechnol. Adv. 33, 745–755. doi: 10.1016/j.biotechadv.2015.05.003
Chhikara, S., Paulose, B., White, J. C., and Dhankher, O. P. (2010). Understanding the physiological and molecular mechanism of persistent organic pollutant uptake and detoxification in cucurbit species (zucchini and squash). Environ. Sci. Technol. 44, 7295–7301. doi: 10.1021/es100116t
Dai, Z.-C., Fu, W., Wan, L.-Y., Cai, H.-H., Wang, N., Qi, S.-S., et al. (2016). Different growth promoting effects of endophytic bacteria on invasive and native clonal plants. Front. Plant Sci. 7:706. doi: 10.3389/fpls.2016.00706
Doty, S. L. (2008). Enhancing phytoremediation through the use of transgenics and endophytes. New Phytol. 179, 318–333. doi: 10.1111/j.1469-8137.2008.02446.x
Drake, E. J., and Gulick, A. M. (2008). Three-dimensional structures of Pseudomonas aeruginosa PvcA and PvcB, two proteins involved in the synthesis of 2-isocyano-6, 7-dihydroxycoumarin. J. Mol. Biol. 384, 193–205. doi: 10.1016/j.jmb.2008.09.027
Feng, N.-X., Yu, J., Zhao, H.-M., Cheng, Y.-T., Mo, C.-H., Cai, Q.-Y., et al. (2017). Efficient phytoremediation of organic contaminants in soils using plant–endophyte partnerships. Sci. Total Environ. 583, 352–368. doi: 10.1016/j.scitotenv.2017.01.075
Gallego, J. L., Loredo, J., Llamas, J. F., Vázquez, F., and Sánchez, J. (2001). Bioremediation of diesel-contaminated soils: evaluation of potential in situ techniques by study of bacterial degradation. Biodegradation 12, 325–335. doi: 10.1023/A:1014397732435
Gao, Y.-C., Wang, J.-N., Guo, S.-H., Hu, Y.-L., Li, T.-T., Mao, R., et al. (2015). Effects of salinization and crude oil contamination on soil bacterial community structure in the Yellow River Delta region, China. Appl. Soil Ecol. 86, 165–173. doi: 10.1016/j.apsoil.2014.10.011
Gardner, S. N., Slezak, T., and Hall, B. G. (2015). kSNP3. 0: SNP detection and phylogenetic analysis of genomes without genome alignment or reference genome. Bioinformatics 31, 2877–2878. doi: 10.1093/bioinformatics/btv271
Ge, S.-M., Xie, B.-E., and Chen, S.-F. (2006). Characterization of two trpE genes encoding anthranilate synthase α-subunit in Azospirillum brasilense. Biochem. Biophys. Res. Commun. 341, 494–499. doi: 10.1016/j.bbrc.2006.01.009
Gerhardt, K. E., Huang, X.-D., Glick, B. R., and Greenberg, B. M. (2009). Phytoremediation and rhizoremediation of organic soil contaminants: potential and challenges. Plant Sci. 176, 20–30. doi: 10.1016/j.plantsci.2008.09.014
Germaine, K. J., Keogh, E., Ryan, D., and Dowling, D. N. (2009). Bacterial endophyte-mediated naphthalene phytoprotection and phytoremediation. FEMS Microbiol. Lett. 296, 226–234. doi: 10.1111/j.1574-6968.2009.01637.x
Górna, H., Ławniczak,Ł., Zgoła-Grześkowiak, A., and Kaczorek, E. (2011). Differences and dynamic changes in the cell surface properties of three Pseudomonas aeruginosa strains isolated from petroleum-polluted soil as a response to various carbon sources and the external addition of rhamnolipids. Bioresour. Technol. 102, 3028–3033. doi: 10.1016/j.biortech.2010.09.124
Harayama, S., Kasai, Y. K., and Kishira, H. (1999). Petroleum biodegradation in marine environments. J. Mol. Microbiol. Biotechnol. 1, 63–70.
Hontzeas, N., Zoidakis, J., Glick, B. R., and Abuomar, M. M. (2004). Expression and characterization of 1-aminocyclopropane-1-carboxylate deaminase from the rhizobacterium Pseudomonas putida uw4: a key enzyme in bacterial plant growth promotion. Biochim. Biophys. Acta 1703, 11–19. doi: 10.1016/j.bbapap.2004.09.015
Kukla, M., Płociniczak, T., and Piotrowska-Seget, Z. (2014). Diversity of endophytic bacteria in Lolium perenne, and their potential to degrade petroleum hydrocarbons and promote plant growth. Chemosphere 117, 40–46. doi: 10.1016/j.chemosphere.2014.05.055
Kurtz, S., Phillippy, A., Delcher, A. L., Smoot, M., Shumway, M., Antonescu, C., et al. (2004). Versatile and open software for comparing large genomes. Genome Biol. 5:R12. doi: 10.1186/gb-2004-5-2-r12
Lang, S., and Wullbrandt, D. (1999). Rhamnose lipids – biosynthesis, microbial production and application potential. Appl. Microbiol. Biotechnol. 51, 22–32. doi: 10.1007/s002530051358
Lee, J., Wu, J., Deng, Y., Wang, J., Wang, C., Wang, J., et al. (2013). A cell-cell communication signal integrates quorum sensing and stress response. Nat. Chem. Biol. 9, 339–343. doi: 10.1038/nchembio.1225
Letunic, I., and Bork, P. (2016). Interactive tree of life (iTOL) v3: an online tool for the display and annotation of phylogenetic and other trees. Nucleic Acids Res. 44, W242–W245. doi: 10.1093/nar/gkw290
Ma, R., Cao, Y., Cheng, Z., Lei, S., Huang, W., Li, X., et al. (2017). Identification and genomic analysis of antifungal property of a tomato root endophyte Pseudomonas sp. p21. Antonie Van Leeuwenhoek 110, 387–397. doi: 10.1007/s10482-016-0811-5
Machuca, A., and Milagres, A. (2003). Use of CAS-agar plate modified to study the effect of different variables on the siderophore production by Aspergillus. Lett. Appl. Microbiol. 36, 177–181. doi: 10.1046/j.1472-765X.2003.01290.x
Meudec, A., Dussauze, J., Deslandes, E., and Poupart, N. (2006). Evidence for bioaccumulation of PAHs within internal shoot tissues by a halophytic plant artificially exposed to petroleum-polluted sediments. Chemosphere 65, 474–481. doi: 10.1016/j.chemosphere.2006.01.058
Mishra, J., Singh, R., and Arora, N. K. (2017). Alleviation of heavy metal stress in plants and remediation of soil by rhizosphere microorganisms. Front. Microbiol. 8:1706. doi: 10.3389/fmicb.2017.01706
Mueller, J. G., Chapman, P., and Pritchard, P. (1989). Action of a fluoranthene-utilizing bacterial community on polycyclic aromatic hydrocarbon components of creosote. Appl. Environ. Microbiol. 55, 3085–3090.
Nie, M., Zhang, X., Wang, J., Jiang, L., Yang, J., Quan, Z., et al. (2009). Rhizosphere effects on soil bacterial abundance and diversity in the Yellow River Deltaic ecosystem as influenced by petroleum contamination and soil salinization. Soil Biol. Biochem. 41, 2535–2542. doi: 10.1016/j.soilbio.2009.09.012
Palmroth, M. R., Pichtel, J., and Puhakka, J. A. (2002). Phytoremediation of subarctic soil contaminated with diesel fuel. Bioresour. Technol. 84, 221–228. doi: 10.1016/S0960-8524(02)00055-X
Pawlik, M., Cania, B., Thijs, S., Vangronsveld, J., and Piotrowska-Seget, Z. (2017). Hydrocarbon degradation potential and plant growth-promoting activity of culturable endophytic bacteria of Lotus corniculatus and Oenothera biennis from a long-term polluted site. Environ. Sci. Pollut. Res. Int. 24, 19640–19652. doi: 10.1007/s11356-017-9496-1
Penrose, D. M., and Glick, B. R. (2003). Methods for isolating and characterizing ACC deaminase-containing plant growth-promoting rhizobacteria. Physiol. Plant. 118, 10–15. doi: 10.1034/j.1399-3054.2003.00086.x
Phillips, L. A., Germida, J. J., Farrell, R. E., and Greer, C. W. (2008). Hydrocarbon degradation potential and activity of endophytic bacteria associated with prairie plants. Soil Biol. Biochem. 40, 3054–3064. doi: 10.1016/j.soilbio.2008.09.006
Pitcher, D., Saunders, N., and Owen, R. (1989). Rapid extraction of bacterial genomic DNA with guanidium thiocyanate. Lett. Appl. Microbiol. 8, 151–156. doi: 10.1111/j.1472-765X.1989.tb00262.x
Rahman, K. S. M., Rahman, T. J., McClean, S., Marchant, R., and Banat, I. M. (2002). Rhamnolipid biosurfactant production by strains of Pseudomonas aeruginosa using low-cost raw materials. Biotechnol. Prog. 18, 1277–1281. doi: 10.1021/bp020071x
Rodriguez, H., Vessely, S., Shah, S., and Glick, B. R. (2008). Effect of a nickel-tolerant acc deaminase-producing Pseudomonas strain on growth of nontransformed and transgenic canola plants. Curr. Microbiol. 57, 170–174. doi: 10.1007/s00284-008-9181-1
Rojo, F. (2009). Degradation of alkanes by bacteria. Environ. Microbiol. 11, 2477–2490. doi: 10.1111/j.1462-2920.2009.01948.x
Safronova, V. I., Stepanok, V. V., Engqvist, G. L., Alekseyev, Y. V., and Belimov, A. A. (2006). Root-associated bacteria containing 1-aminocyclopropane-1-carboxylate deaminase improve growth and nutrient uptake by pea genotypes cultivated in cadmium supplemented soil. Biol. Fertil. Soils 42, 267–272. doi: 10.1007/s00374-005-0024-y
Sarwar, M., and Kremer, R. (1995). Determination of bacterially derived auxins using a microplate method. Lett. Appl. Microbiol. 20, 282–285. doi: 10.1111/j.1472-765X.1995.tb00446.x
Shi, Y., Xu, S., Xu, L., and Cai, Y. (2015). Distribution, elimination, and rearrangement of cyclic volatile methylsiloxanes in oil-contaminated soil of the Shengli oilfield, China. Environ. Sci. Technol. 49, 11527–11535. doi: 10.1021/acs.est.5b03197
Sionit, N., Mortensen, D., Strain, B., and Hellmers, H. (1981). Growth response of wheat to CO2 enrichment and different levels of mineral nutrition1. Agron. J. 73, 1023–1027. doi: 10.2134/agronj1981.00021962007300060027x
Smits, T. H., Witholt, B., and van Beilen, J. B. (2003). Functional characterization of genes involved in alkane oxidation by Pseudomonas aeruginosa. Antonie Van Leeuwenhoek 84, 193–200. doi: 10.1023/A:1026000622765
Tara, N., Afzal, M., Ansari, T. M., Tahseen, R., Iqbal, S., and Khan, Q. M. (2014). Combined use of alkane-degrading and plant growth-promoting bacteria enhanced phytoremediation of diesel contaminated soil. Int. J. Phytoremediation 16, 1268–1277. doi: 10.1080/15226514.2013.828013
Tatusov, R. L., Galperin, M. Y., Natale, D. A., and Koonin, E. V. (2000). The COG database: a tool for genome-scale analysis of protein functions and evolution. Nucleic Acids Res. 28, 33–36. doi: 10.1093/nar/28.1.33
Truu, J., Truu, M., Espenberg, M., Nõlvak, H., and Juhanson, J. (2015). Phytoremediation and plant-assisted bioremediation in soil and treatment wetlands: a review. Open Biotechnol. J. 9, 85–92. doi: 10.2174/1874070701509010085
Van Beilen, J. B., and Funhoff, E. G. (2007). Alkane hydroxylases involved in microbial alkane degradation. Appl. Microbiol. Biotechnol. 74, 13–21. doi: 10.1007/s00253-006-0748-0
Wang, X., Yu, J., Zhou, D., Dong, H., Li, Y., Lin, Q., et al. (2012). Vegetative ecological characteristics of restored reed (Phragmites australis) wetlands in the Yellow River Delta, China. Environ. Manage. 49, 325–333. doi: 10.1007/s00267-011-9757-6
Wang, Z., Xu, Y., Zhao, J., Li, F., Gao, D., and Xing, B. (2011). Remediation of petroleum contaminated soils through composting and rhizosphere degradation. J. Hazard. Mater. 190, 677–685. doi: 10.1016/j.jhazmat.2011.03.103
Wentzel, A., Ellingsen, T. E., Kotlar, H.-K., Zotchev, S. B., and Throne-Holst, M. (2007). Bacterial metabolism of long-chain n-alkanes. Appl. Microbiol. Biotechnol. 76, 1209–1221. doi: 10.1007/s00253-007-1119-1
Weyens, N., Croes, S., Dupae, J., Newman, L., Van Der Lelie, D., Carleer, R., et al. (2010). Endophytic bacteria improve phytoremediation of Ni and TCE co-contamination. Environ. Pollut. 158, 2422–2427. doi: 10.1016/j.envpol.2010.04.004
Wu, T., Xie, W., Yi, Y., Li, X., Yang, H., and Wang, J. (2012). Surface activity of salt-tolerant Serratia spp. and crude oil biodegradation in saline soil. Plant Soil Environ. 58, 412–416. doi: 10.17221/217/2012-PSE
Zhang, X., Liu, X., Wang, Q., Chen, X., Li, H., Wei, J., et al. (2014). Diesel degradation potential of endophytic bacteria isolated from Scirpus triqueter. Int. Biodeterior. Biodegradation 87, 99–105. doi: 10.1016/j.ibiod.2013.11.007
Zhang, Y. F., He, L. Y., Chen, Z. J., Zhang, W. H., Wang, Q. Y., Qian, M., et al. (2011). Characterization of lead-resistant and ACC deaminase-producing endophytic bacteria and their potential in promoting lead accumulation of rape. J. Hazard. Mater. 186, 1720–1725. doi: 10.1016/j.jhazmat.2010.12.069
Zhang, Z., Gai, L., Hou, Z., Yang, C., Ma, C., Wang, Z., et al. (2010). Characterization and biotechnological potential of petroleum-degrading bacteria isolated from oil-contaminated soils. Bioresour. Technol. 101, 8452–8456. doi: 10.1016/j.biortech.2010.05.060
Keywords: endophytic bacterium, Pseudomonas aeruginosa, complete genome, hydrocarbon degradation, biosurfactant synthesis, plant growth promotion
Citation: Wu T, Xu J, Xie W, Yao Z, Yang H, Sun C and Li X (2018) Pseudomonas aeruginosa L10: A Hydrocarbon-Degrading, Biosurfactant-Producing, and Plant-Growth-Promoting Endophytic Bacterium Isolated From a Reed (Phragmites australis). Front. Microbiol. 9:1087. doi: 10.3389/fmicb.2018.01087
Received: 31 March 2018; Accepted: 07 May 2018;
Published: 25 May 2018.
Edited by:
Pilar Martínez-Hidalgo, Universidad de Salamanca, SpainReviewed by:
Lars M. Blank, RWTH Aachen University, GermanyElena Perrin, Università degli Studi di Firenze, Italy
Copyright © 2018 Wu, Xu, Xie, Yao, Yang, Sun and Li. This is an open-access article distributed under the terms of the Creative Commons Attribution License (CC BY). The use, distribution or reproduction in other forums is permitted, provided the original author(s) and the copyright owner are credited and that the original publication in this journal is cited, in accordance with accepted academic practice. No use, distribution or reproduction is permitted which does not comply with these terms.
*Correspondence: Tao Wu, d3RzZGJ6QGhvdG1haWwuY29t; d3RzZGJ6QDE2My5jb20= Wenjun Xie, eHdqZXJpY0AxNjMuY29t