- 1Key Laboratory of Experimental Marine Biology, Institute of Oceanology, Chinese Academy of Sciences, Qingdao, China
- 2Laboratory for Marine Biology and Biotechnology, Qingdao National Laboratory for Marine Science and Technology, Qingdao, China
Edwardsiella tarda is a broad-host pathogen that can infect mammals, reptiles, and fish. E. tarda exhibits a remarkable ability to survive in host serum and replicate in host phagocytes, but the underlining mechanism is unclear. In this study, in order to identify E. tarda proteins involved in serum resistance, iTRAQ proteomic analysis was performed to examine the whole-cell protein profiles of TX01, a pathogenic E. tarda isolate, in response to serum treatment. Of the differentially expressed proteins identified, one (named Sip2) possesses a conserved hydrogenase domain and is homologous to the putative hydrogenase accessory protein HypB. When Sip2 was expressed in Escherichia coli, it significantly enhanced the survival of the host cells in serum. Compared to TX01, the sip2 knockout, TX01Δsip2, was dramatically reduced in the ability of hydrogenase activity, serum resistance, intracellular replication, dissemination in fish tissues, and causing mortality in infected fish. The lost virulence capacities of TX01Δsip2 were restored by complementation with the sip2 gene. Furthermore, TX01Δsip2 was significantly reduced in the capacity to grow under low pHs and iron-depleted conditions, and was unable to maintain its internal pH in acidic environment. Taken together, these results indicate that Sip2 is a novel serum-induced protein that is essential to serum resistance, cellular and tissue infection, and coping with acidic stress via its ability to modulate intracellular pH.
Introduction
Edwardsiella tarda is a Gram-negative bacterium of the family Enterobacteriaceae. It is a zoonotic pathogen with a broad host range that includes mammals, reptiles, and fish (Mohanty and Sahoo, 2007; Leung et al., 2012). In aquaculture, E. tarda is known to infect a large number of freshwater and marine fish including flounder, turbot, tongue sole, and tilapia (Matsuyama et al., 2005; Katharios et al., 2015; Wang and Sun, 2015; Zeng et al., 2017). As a result, E. tarda is counted one of the most severe fish pathogens. In addition to fish, E. tarda is also a human pathogen and has been reported to cause acute gastroenteritis, meningitis, septicemia, and wound infections in humans (Nelson et al., 2009; Park et al., 2012; Crosby et al., 2013; Suezawa et al., 2016).
Previous studies have shown that E. tarda is able to evade the bactericidal effect of host serum (Leung et al., 2012; Li et al., 2015a; Zhou et al., 2015; Dubytska et al., 2016). In serum, the complement system plays a key role in host defense against infection via mechanisms involving both innate and adaptive immunity (Walport, 2001; Merle et al., 2015). The complement system consists of three pathways of activation: the classical pathway, the alternative pathway, and the lectin pathway (Ricklin et al., 2010). Activation of the complement system leads to the formation of membrane attack complex (MAC) that lyses bacteria by inserting into bacterial membrane and forming large pores (Endo et al., 2006; Sarma and Ward, 2011). In recent studies, it has been observed that E. tarda resists serum killing by preventing complement activation via the alternative pathway (Li et al., 2015a), and that a zinc metalloprotease, Sip1, of E. tarda is essential for serum survival and host infection (Zhou et al., 2015). Moreover, serum enhances the tricarboxylic acid cycle of E. tarda, which increases membrane potential and decreases the formation of MAC at cell surface, resulting in serum resistance (Cheng et al., 2017). However, the key factors that are involved in serum resistance still remain to be discovered.
Hydrogenases are bacterial enzymes that catalyze the bidirectional oxidation of hydrogen according to the following reaction: (Wang et al., 2015). Hydrogenases are found in diverse organisms including anaerobic and aerobic prokaryotes (Sawers et al., 1985; Sawers, 2005; Wang et al., 2015). The physiological function of most prokaryotic hydrogenases is to oxidize hydrogen gas and reduce electron acceptors (Kwan et al., 2015). The production and consumption of hydrogen gas by hydrogenases have critical roles in the global hydrogen cycle and are intimately connected to the nitrogen and carbon cycles (Tamagnini et al., 2007; Thauer, 2010). Another function of prokaryotic hydrogenases is to maintain the intracellular pH and redox potential at suitable levels (Adams et al., 1980; Ueno et al., 1999). The hydrogenase of Escherichia coli has been suggested to decrease cytoplasmic acid stress and contribute to acid resistance (Yoshida et al., 2005; Hayes et al., 2006). In E. coli, Hya and Hyb are hydrogen-oxidizing hydrogenases, and Hyc and Hyf are hydrogen evolving hydrogenases (Zbell and Maier, 2009). Hya may be used to recycle Hyc-produced H2, since the hya operon is expressed at high levels during fermentative growth, or it may play a role in acid stress resistance (King and Przybyla, 1999). In Salmonella enterica, it has been shown that hydrogenase is expressed during infection and involved in the virulence of the bacterium by facilitating tissue invasion (Maier et al., 2004; Zbell et al., 2008; Lamichhane-Khadka et al., 2015).
In this study, with an aim to identify E. tarda proteins associated with serum resistance, we employed proteomic approach to examine the whole proteins of E. tarda that were induced in expression by tongue sole serum. Of the proteins thus identified, one was further investigated for biological activity and involvement in pathogenicity including serum survival.
Materials and Methods
Ethics Statement
Experiments involving live animals conducted in this study were approved by the Ethics Committee of Institute of Oceanology, Chinese Academy of Sciences. All methods were carried out in accordance with the relevant guidelines, including any relevant details.
Fish
Clinically healthy tongue sole were purchased from a commercial fish farm in Shandong Province, China. Fish were maintained at 20°C in aerated seawater and fed daily with commercial dry pellets. Before experiment, the fish were verified to be clinically healthy by examining bacterial presence in some tissues as reported previously (Zhou and Sun, 2015). Fish were euthanized with an overdose of tricaine methanesulfonate (Sigma, St. Louis, MO, United States) before tissue collection.
Bacterial Culture Conditions
Edwardsiella tarda TX01, a fish isolate, was cultured in Luria-Bertani broth (LB) at 28°C as reported previously (Zhang et al., 2008b). E. coli BL21 (DE3) and DH5α were purchased from TransGen Biotech (Beijing, China); E. coli S17-1λpir was purchased from Biomedal (Sevilla, Spain). The E. coli strains were cultured in LB medium at 37°C. Where indicated, polymyxin B, tetracycline, and chloramphenicol were supplemented at the concentrations of 100, 20, and 50 μg/ml, respectively.
Quantitative iTRAQ-LC–MS/MS Proteomic Analysis
Edwardsiella tarda TX01 was cultured in LB medium to an OD600 of 0.8. The cells were washed with PBS and mixed with tongue sole serum or PBS (control). After incubation with mild agitation at 28°C for 1 h, the cells were collected by centrifugation, washed with PBS, and immediately frozen in liquid nitrogen. iTRAQ-LC–MS/MS proteomic analysis and data analysis were performed as reported previously (Hu and Sun, 2016).
Sequence Analysis
Sequence analysis was performed using the BLAST program at the National Center for Biotechnology Information (NCBI) and the Expert Protein Analysis System. Domain search was performed with the conserved domain search program of NCBI. Theoretical molecular mass and isoelectric point were predicted using EditSeq in the DNASTAR software package (Madison, WI, United States). Multiple sequence alignment was created with DNAMAN. Subcellular localization prediction was performed with the PSORTb v.3.0 server.
Plasmid Construction
To construct pETSip2, the coding sequence of Sip2 was amplified by PCR with primers Sip2F (5′- GATATCATGTGTACCACCTGCGGCTG -3′, underlined sequence, EcoRV site) and Sip2R (5′-GATATCTTGATTTTCTCCCAGCGTGG -3′, underlined sequence, EcoRV site). The PCR product was ligated with the T-A cloning vector T-Simple (TransGen Biotech., Beijing, China), and the recombinant plasmid was digested with EcoRV to retrieve the sip2-containing fragment, which was inserted into pET259 (Zhou and Sun, 2015) at the SwaI site, resulting in pETSip2. E. coli BL21 (DE3) was transformed with pETSip2 or pET259 (control), and the transformants were named BL21/pETSip2 or BL21/pET259.
To construct the low copy-number plasmid pJTSip2 that expresses sip2, sip2 was amplified by PCR as above; the PCR product was ligated with the TA cloning vector T-Simple, and the recombinant plasmid was digested with EcoRV. The fragment containing sip2 was retrieved and inserted into plasmid pBT3 (Zhang et al., 2008a) at the EcoRV site, resulting in pBT3Sip2. pBT3Sip2 was digested with SwaI, and the fragment carrying sip2 was inserted into plasmid pJT (Sun et al., 2009) at the SwaI site, resulting in pJTSip2. All PCR products were verified by sequence analysis.
Construction of TX01Δsip2 and TX01Δsip2/sip2
To construct E. tarda TX01Δsip2, in-frame deletion of a 300 bp segment of sip2 (residues 275 to 374) was performed by overlap extension PCR as follows: the first overlap PCR was performed with primers F1 (5′- GGATCCCACCACTATCACTATGTCTTC -3′, underlined sequence, BamHI site) and R1 (5′- ACACATTAGTTTTCAATGAACAGTAGGC -3′), the second overlap PCR was performed with primers F2 (5′- TTGAAAACTAATGTGTCTGGGAATTCCG -3′) and R2 (5′- GGATCCGTCACCAAAGGTGCAGAACA -3′, underlined sequence, BamHI site), and the fusion PCR was performed with the primer pair F1/R2. The PCR product was inserted into the suicide plasmid pDM4 (Milton et al., 1996) at the BglII site, resulting in pDMSip2. S17-1λpir was transformed with pDMSip2, and the transformants were conjugated with TX01 as reported previously (Sun et al., 2012). Briefly, the donor and recipient strains were cultured in LB medium to OD600 of 0.8 and mixed at a ratio of 3:1. The mixture was dropped onto a LB agar plate, and the plate was incubated at 28°C for 24 h. After incubation, the bacteria on the plate were resuspended in 2 ml LB, from which 100 μl was taken and plated on a LB agar plate supplemented with polymyxin B and chloramphenicol and then on LB plates containing 10% sucrose. One of the colonies resistant to sucrose and sensitive to chloramphenicol (marker of pDM4) was analyzed by PCR, and the PCR product was subjected to DNA sequencing to confirm in-frame deletion. This strain was named TX01Δsip2.
To construct the sip2 complement strain TX01Δsip2/sip2, S17-1λpir was transformed with pJTSip2, and the transformants were conjugated with TX01Δsip2. The transconjugants were selected on LB agar plates supplemented with tetracycline (marker of pJT) and polymyxin B (marker of TX01 and its derivatives). One of the transformants was named TX01Δsip2/sip2.
Serum Survival Assay
Serum survival assay was performed as reported previously (Li et al., 2015a). Briefly, to examine the serum survival of E. tarda, E. tarda strains (TX01, TX01Δsip2, and TX01Δsip2/sip2) were cultured in LB medium to an OD600 of 0.8. The cells were washed with PBS and resuspended in PBS. Approximately 105 bacterial cells were mixed with 50 μl untreated tongue sole serum or heat-inactivated tongue sole serum (control). After incubation with mild agitation at 22°C for 1 h, the mixture was serially diluted and plated in triplicate on LB agar plates. The plates were incubated at 28°C for 48 h, and the colonies that appeared on the plates were enumerated. The genetic identity of the colonies was verified as above. The survival rate was calculated as follows: (number of cells surviving serum treatment/number of cells surviving heat-inactivated serum treatment) × 100%.
To examine the serum survival of E. coli BL21/pETSip2 and BL21/pET259, the bacteria were cultured in LB medium to mid-logarithmic phase, and isopropyl-β-D-thiogalactopyranoside (1 mM) was added to the culture to induce Sip2 expression. After growth at 28°C for an additional 4 h, the cells were washed and harvested by centrifugation, and resuspended to 2 × 106 colony-forming units (CFU)/ml in PBS. The bacterial suspension was mixed with heated or unheated tongue sole serum (1/8 dilution), followed by incubation at 22°C for 1 h. the mixture was serially diluted and plated in triplicate in LB agar plates. The plates were incubated at 37°C for 24 h, and the colonies that appeared on the plates were enumerated. The genetic nature of the colonies was verified by PCR. The survival rate was calculated as above. All experiments were performed three times.
Electron Microscopy
E. tarda TX01, TX01Δsip2, and TX01Δsip2/sip2 were cultured as above and resuspended in PBS to 108 CFU/ml. The cells were incubated with normal or heat-inactivated (control) tongue sole serum at 22°C for 1 h. After incubation, the cells were observed with a transmission electron microscope (HT7700, Hitachi, Japan) or a scanning electron microscope (S-3400N, Hitachi, Japan).
In Vivo Infection Analysis
Bacterial in vivo infection analysis was performed as reported previously (Li et al., 2015b). Briefly, E. tarda TX01, TX01Δsip2, and TX01Δsip2/sip2 were cultured as above. The cells were washed with PBS and resuspended in PBS to 5 × 106 CFU/ml. Tongue sole (average 15.7 g) were randomly divided into three groups (20 fish/group) and infected via intramuscular injection with 100 μl TX01, TXΔsip2, or TXΔsip2/sip2. At 12, 24, and 48 h post-infection, kidney, spleen, and blood were collected from the fish (five at each time point). The tissues were homogenized in PBS. The homogenates was serially diluted and plated in triplicate on LB agar plates. The plates were incubated at 28°C for 48 h, and the colonies that appeared on the plates were enumerated. The genetic identity of the colonies was verified as above. For mortality analysis, three groups (20 fish/group) of tongue sole were infected as above with TX01, TX01Δsip2, or TX01Δsip2/sip2, and the fish were monitored daily for mortality for 15 days. The experiments were performed three times.
Bacterial Replication in Peripheral Blood Leukocytes (PBL)
Tongue sole PBL were prepared with Percoll as reported previously (Zhang and Sun, 2015). The cells were cultured in L-15 medium (Thermo Scientific HyClone, Beijing, China) in 96-well culture plates (105 cells/well). TX01, TX01Δsip2, and TX01Δsip2/sip2 were prepared as above and added to PBL (106 CFU/well). The cells were incubated at 28°C for 1 h and washed three times with PBS. Fresh L-15 medium containing 100 μg/ml gentamicin (Solarbio, Beijing, China) was added to the cells, and the cells were incubated at 28°C for 1 h to kill extracellular bacteria. The plates were then washed three times with PBS and incubated at 28°C for 0, 1, 2, 4, and 8 h. After incubation, the plates were washed with PBS, and the cells were lysed with 100 μl 1% Triton X-100. The cell lysate was diluted and plated in triplicate on LB agar plates. The plates were incubated at 28°C for 48 h, and the colonies that emerged on the plates were counted. The identity of the colonies was verified as described above. The experiment was performed three times.
Bacterial Growth Under Different Conditions
TX01, TX01Δsip2, and TX01Δsip2/sip2 were cultured in normal LB medium or in LB medium adjusted to different pH or depleted of iron by adding 2,2′-dipyridyl (100 μM) (Sigma, St. Louis, United States). After culturing at 28°C for 0 to 12 h, cell density was measured using a spectrophotometer (Thermo Scientific, Beijing, China). The assay was performed three times.
Hydrogenase Activity Assay
Hydrogenase activity was assayed as reported previously (Lamichhane-Khadka et al., 2010). Briefly, E. tarda TX01, TX01Δsip2, and TX01Δsip2/sip2 were cultured as above. The cells were collected and resuspended in LB medium adjusted to different pH or depleted of iron. The cells were grown at 28°C for 8 h under anaerobic condition with 10% H2. After growth, 109 cells were resuspended in PBS and permeabilized by adding 10% Triton X-100, followed by incubation for 30 min at room temperature. After incubation, 1 ml suspension was transferred to a sealed glass cuvette pre-flushed with H2. Sodium dithionite was then injected to a final concentration of 200 μM, followed by the injection of methylene blue to a final concentration of 400 μM. Hydrogen uptake activity was determined by measuring the reduction of methylene blue at 570 nm and is expressed as μmol H2 taken up/min/109 cells.
Bacterial Survival Under Acidic Condition
PBS buffer was adjusted to pH 7, pH 5, or pH 4.5 with hydrochloric acid. TX01, TX01Δsip2, and TX01Δsip2/sip2 (105 CFU/ml) were cultured as above and resuspended in PBS of different pH. The cells were incubated at 28°C for 2 h. After incubation, the cells were diluted in PBS and plated on LB agar plates. The plates were incubated at 28°C for 24 h, and the colonies emerged on the plates were counted. To examine the effect of the acidic environment on the internal pH of E. tarda, TX01, TX01Δsip2, and TX01Δsip2/sip2 (1010 CFU/ml) were incubated at 28°C in PBS (pH 5) for 2 h. The cells were then harvested by centrifugation and resuspended in 1 ml PBS (pH 7) and then boiling for 5 min at 100°C. The cells were then subjected to sonication in an ice-water bath, and the pH of the cell lysate was measured using a pH meter (Sartorius, Beijing, China).
Statistical Analysis
All experiments were repeated three times. Statistical analyses were carried out with SPSS 17.0 software (SPSS Inc., Chicago, IL, United States). Data were analyzed with analysis of variance (ANOVA), and statistical significance was defined as P < 0.05.
Results
Identification of Sip2 and Characterization of Its Effect on Serum Resistance
iTraq analysis indicated that compared to the untreated E. tarda TX01, TX01 treated with serum exhibited differential expression in 124 proteins (Supplementary Table S1). Of the differentially expressed proteins, 16 were up-regulated by more than twofold, and one of these proteins was named Sip2 (Serum Induced Protein 2) and used in this study. Sip2 contains 374 amino acid residues and shares the highest sequence identity (98%) with the putative hydrogenase accessory protein HypB of E. tarda. When the sip2 gene was introduced into and expressed in E. coli, it mildly but significantly increased the survival of the host strain in tongue sole serum (Figure 1A). When the sip2 of TX01 was knocked out via markerless in-frame deletion, the resulting mutant, TX01Δsip2, displayed a serum survival rate that was 54.5% lower than that of the wild type TX01 (Figure 1B). However, when the sip2 gene was introduced back into TX01Δsip2, the resulting complement strain TX01Δsip2/sip2 exhibited a serum survival rate comparable to that of the wild type (Figure 1B). Transmission and scanning microscopy showed that serum treatment caused severe damage to the cellular structure of TX01Δsip2, which, however, was not observed in TX01Δsip2/sip2 or the wild type (Figure 2).
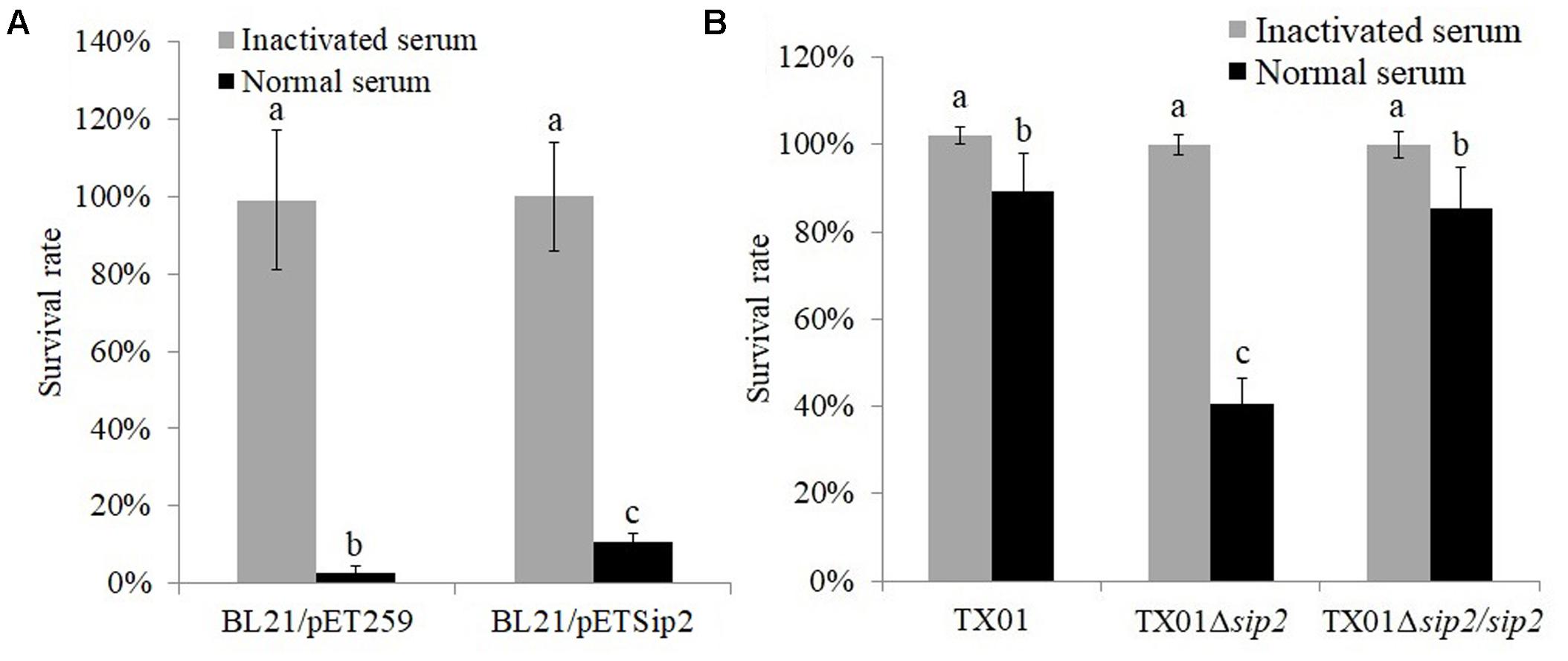
FIGURE 1. Effect of Sip2 on bacterial resistance against serum damage. (A) Escherichia coli BL21/pETSip2 (expressing Sip2) and BL21/pET259 (control) were treated with normal or inactivated tongue sole serum for 1 h, and bacterial survival was determined. (B) Edwardsiella tarda TX01, TX01Δsip2, and TX01Δsip2/sip2 were treated with tongue sole serum as above, and bacterial survival rate was determined. Data are the means of three independent experiments and presented as means ± SEM. Values with different letters indicate significantly different (P < 0.05).
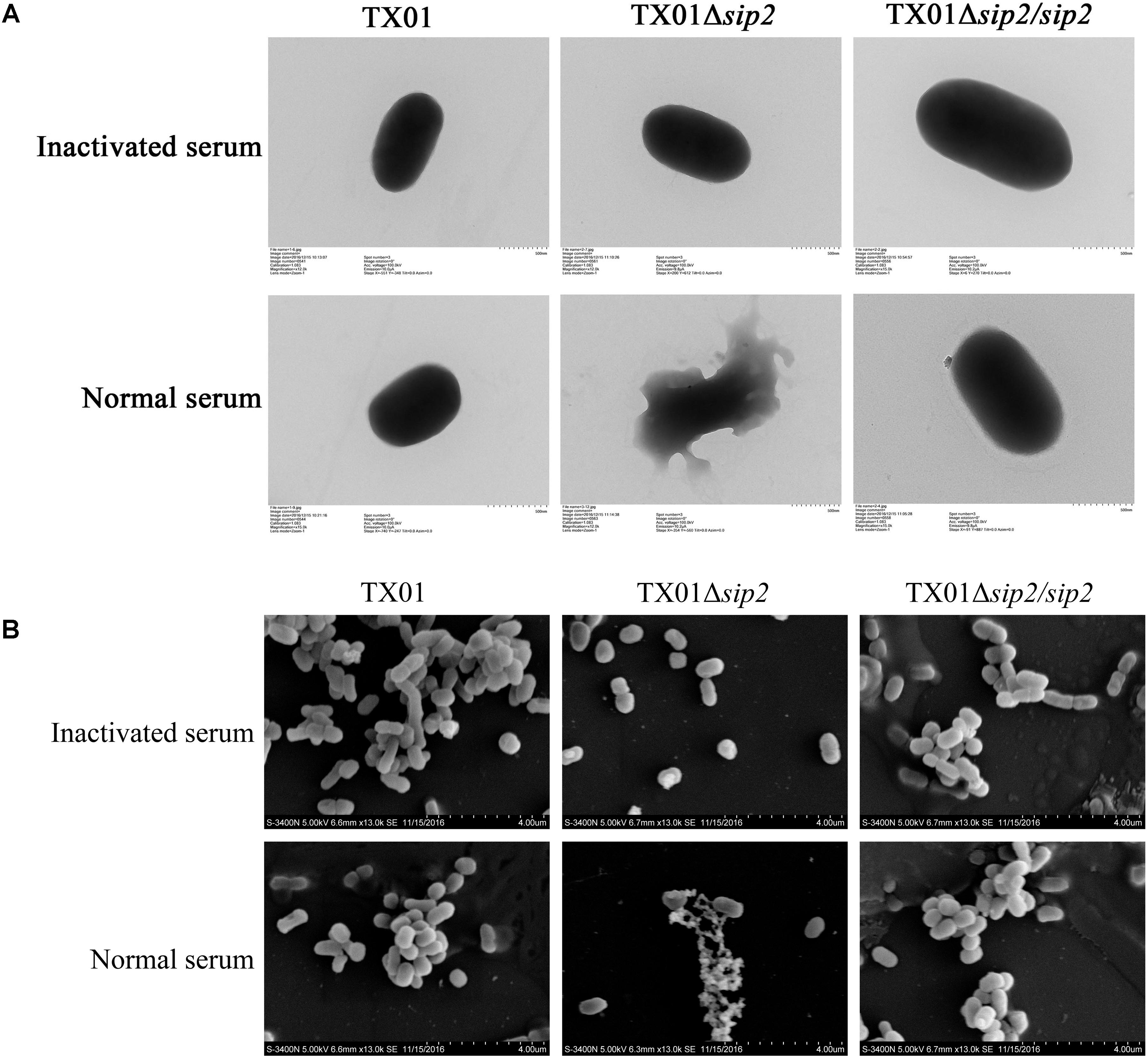
FIGURE 2. Microscopic examination of the effect of serum treatment on Edwardsiella tarda. TX01, TX01Δsip2, and TX01Δsip2/sip2 were treated with normal or heat-inactivated (control) tongue sole serum for 1 h and then subjected to transmission (A) and scanning microscopy (B).
Effect of Sip2 on E. tarda Infectivity
In vivo study showed that when inoculated into tongue sole, TX01Δsip2 exhibited dramatically reduced bacterial disseminations in kidney, spleen, and blood in comparison with the wild type TX01, whereas the tissue dissemination capacity of TX01Δsip2/sip2 was similar to that of the wild type (Figure 3A). Consistently, fish mortality induced by TX01Δsip2 was significantly lower than that induced by TX01 or TX01Δsip2/sip2 (Figure 3B). Cellular infection study indicated that following incubation with tongue sole PBL, TX01 and TX01Δsip2/sip2 replicated steadily inside the cells as shown by the time-dependent increase in intracellular bacterial numbers, whereas TX01Δsip2 replication was barely detectable (Figure 3C).
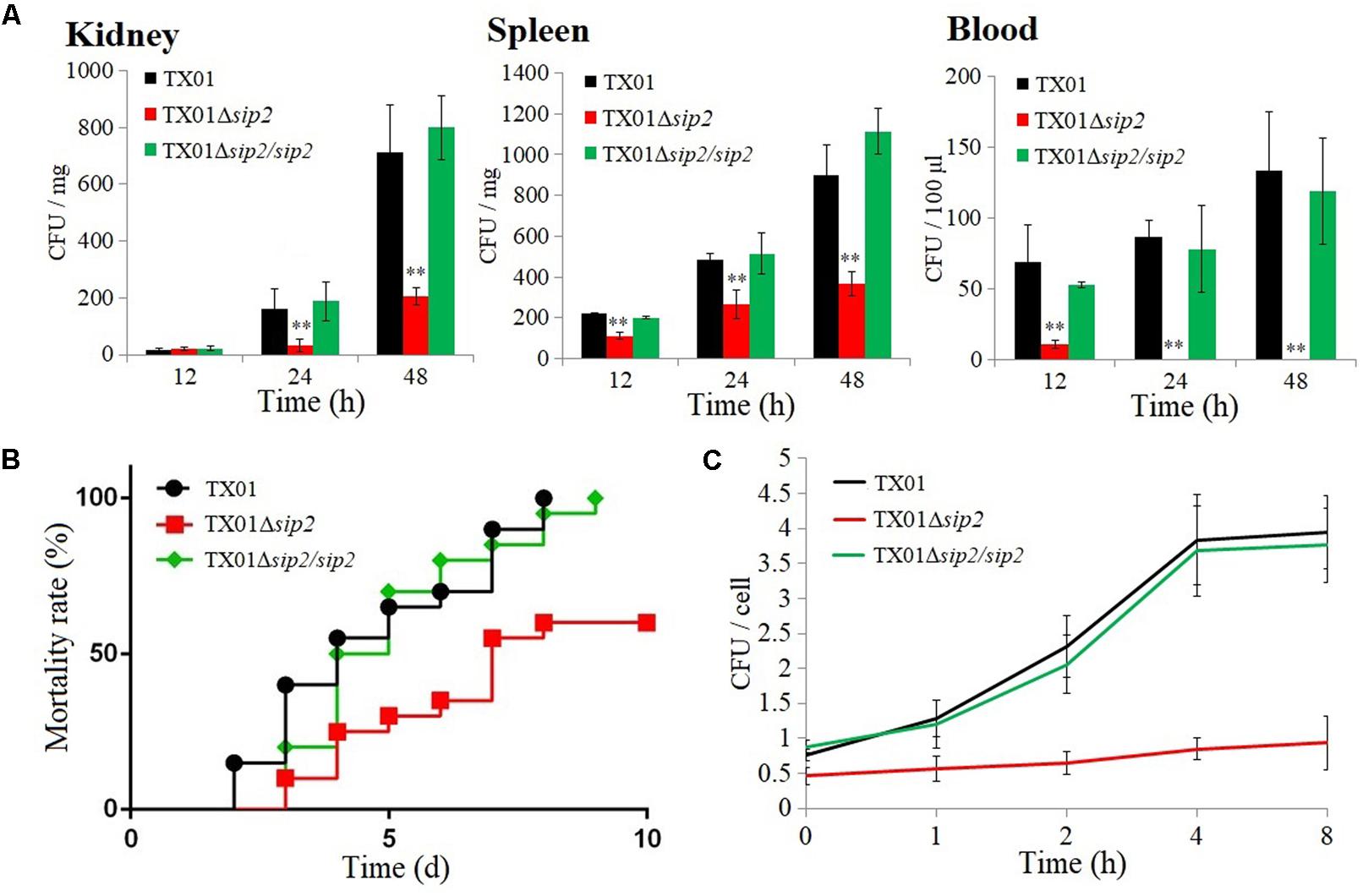
FIGURE 3. Virulence of Edwardsiella tarda. (A) Tongue sole were inoculated with E. tarda TX01, TX01Δsip2, or TX01Δsip2/sip2, and bacterial dissemination in kidney, spleen, and blood was determined by examining bacterial recoveries from the tissues at different time points. Data are the means of three independent assays and presented as means ± SEM. ∗∗P < 0.01, ∗P < 0.05. (B) Tongue sole were infected with E. tarda as above, and mortality of the fish was calculated at different time points. (C) Tongue sole peripheral blood leukocytes (PBL) were infected with TX01, TX01Δsip2, or TX01Δsip2/sip2 for 1 h, and extracellular bacteria were killed; intracellular bacterial number was determined at different time points. Data are the means of three independent assays and presented as means ± SEM.
Effect of Sip2 on Bacterial Growth Under Different Conditions
When cultured in LB medium at pH 7, TX01Δsip2 displayed a growth profile similar to that of TX01 and TX01Δsip2/sip2 (Figure 4A); however, when the culture was performed at pH 6, TX01Δsip2 grew apparently slower than TX01 or TX01Δsip2/sip2 and reached much lower cell densities (Figure 4B). When iron was depleted from the LB medium, the wild type and TX01Δsip2/sip2 exhibited comparable growth profiles, but no growth of TX01Δsip2 was observed (Figure 4C).
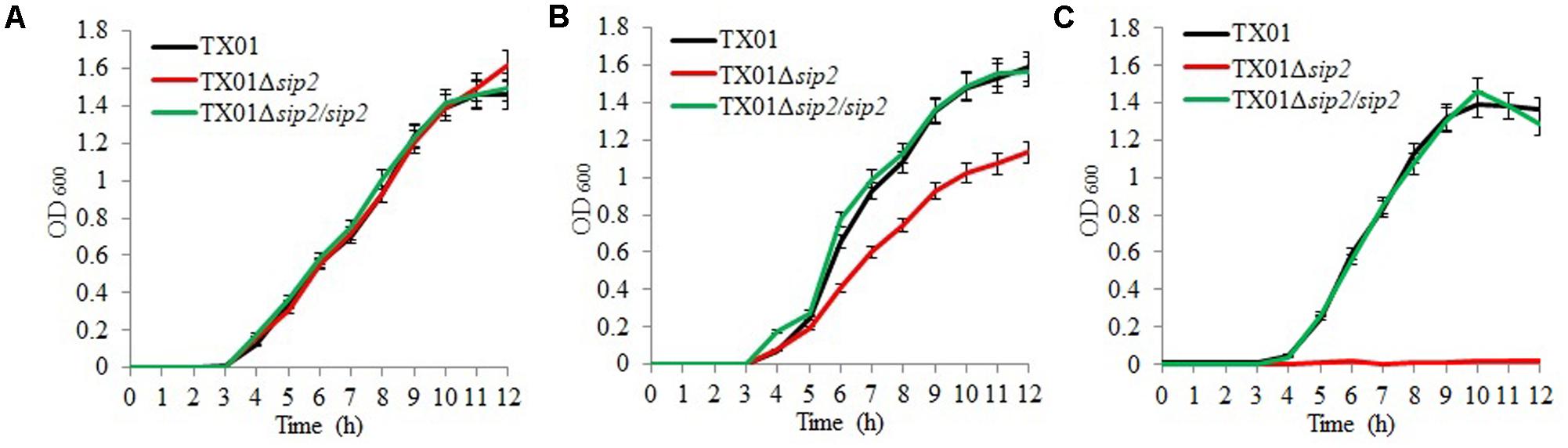
FIGURE 4. Effect of pH and iron on the growth of Edwardsiella tarda. (A,B) E. tarda TX01, TX01Δsip2, and TX01Δsip2/sip2 were cultured in LB medium at pH 7 (A) or pH 6 (B), and bacterial growth was monitored at different time points. (C) E. tarda TX01, TX01Δsip2, and TX01Δsip2/sip2 were cultured in iron-depleted LB medium at pH 7, and bacterial growth was monitored as above. For all panels, data are the means of three independent assays and presented as means ± SEM.
Effect of Sip2 on the Hydrogenase Activity of E. tarda Under Different Conditions
Hydrogenase assay showed that compared to TX01, TX01Δsip2 exhibited significantly lower hydrogenase activity at pH 7, while the hydrogenase activity of TX01Δsip2/sip2 was comparable to that of TX01 (Table 1). Similarly, at pH 6 or when iron was depleted, the hydrogenase activity of TX01Δsip2 was significantly reduced compared to wild type or TX01Δsip2/sip2 (Table 1).
Effect of Sip2 on the Survival of E. tarda Under Acidic Condition
Since, as shown above, TX01Δsip2 was defective when grown under low pH, we examined its capacity to survive under different acidic conditions. The results showed that when incubated in PBS buffer at pH 7, pH 5, and pH 4.5, the survival rates of TX01Δsip2 were 100, 32.9, and 1.6%, respectively, while the survival rates of the wild type were 99.1, 83.6, and 37.9%, respectively (Figure 5A). In contrast to TX01Δsip2, the survival rates of TX01Δsip2/sip2 were largely similar to that of the wild type, especially at lower pH (Figure 5A). To examine whether the acidic environment affected the internal pH of the bacteria, the pH of the cell lysate were measured. The results showed that following incubation at pH 5, the pH of TX01Δsip2 lysate was 6.35, which was significantly lower than that of the wild type (pH 6.95) or TX01Δsip2/sip2 (pH 7.1) (Figure 5B).
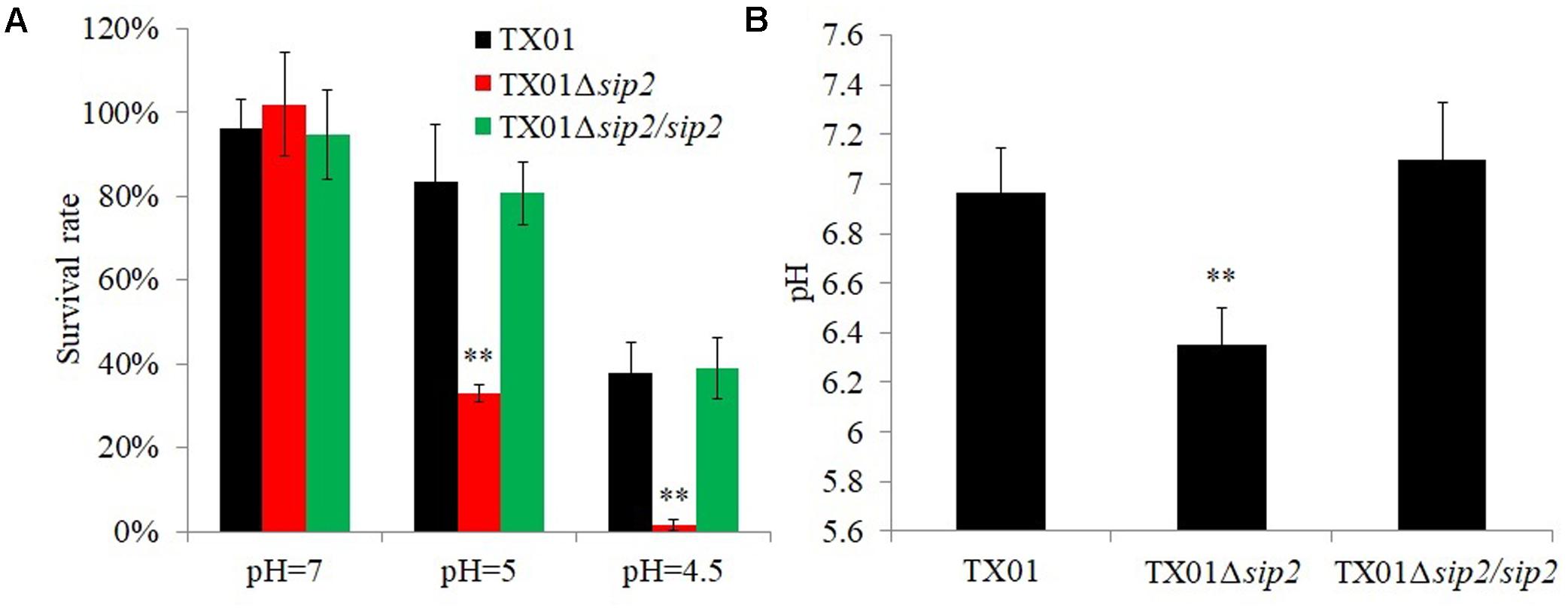
FIGURE 5. Survival of Edwardsiella tarda against acidic stress. (A) TX01, TX01Δsip2, and TX01Δsip2/sip2 were incubated at different pH for 2 h, and bacterial survival was determined. (B) TX01, TX01Δsip2, and TX01Δsip2/sip2 were incubated at pH 5 for 2 h and lysed, and the pH of the cell lysate was determined. Data are the means of three independent assays and presented as means ± SEM. ∗∗P < 0.01, ∗P < 0.05.
Discussion
Serum bactericidal activity mediated by complement plays an important role in the clearance of bacterial infection (Boshra et al., 2006). However, some bacterial pathogens can escape from complement-mediated killing (Berends et al., 2014; Holers, 2014). Studies have shown that pathogens like Streptococcus agalactiae, S. enterica, E. coli, and E. tarda regulate protein expression in response to host serum (Li et al., 2012; Zhou et al., 2015; Dudek et al., 2016; Wang et al., 2016). Despite recent advances in the understanding of the pathogenesis of E. tarda, the mechanism of E. tarda serum resistance is still to be elucidated (Li et al., 2015a). In the present study, we found that contact with fish serum significantly altered the expression of 124 proteins of E. tarda, suggesting that serum stress had a global effect on the expression of E. tarda proteins, which is consistent with the concept that complement-mediated immunity is a dire challenge that has to be coped with by the pathogen. One of the proteins identified in our study, Sip2, was upregulated by serum, suggesting a possible role in serum survival. Sip2 shares the highest sequence identity with a putative HypB protein of E. tarda, however, since no study has been reported with this protein, the function of the putative HypB is unclear.
In bacteria, three mechanisms associated with serum resistance have been observed: lipopolysaccharide and capsular polysaccharide-mediated suppression of complement activation, secretion of proteases that directly inactivate complement, and inhibition of complement activation through recruitment of factors such as factor H and C4BP to bacterial cell surface (Rooijakkers and van Strijp, 2007; Hovingh et al., 2016; Abreu and Barbosa, 2017). In our study, we found that the recombinant E. coli BL21 that expresses Sip2 (i.e., E. coli BL21/pETSip2) exhibited significantly increased survival in tongue sole serum, suggesting that recombinant Sip2 was able to confer protection against serum on the host cells. In line with this observation, TX01Δsip2 displayed dramatically reduced survival rate in tongue sole serum, and the reduced surviving ability of TX01Δsip2 was restored by introduction of the sip2 gene into the bacteria. These results indicated that Sip2 was essential to the serum resistance of E. tarda. Since Sip2 is a homologue of a putative intracellular hydrogenase, Sip2-mediated serum resistance mechanism may possibly differ from the three known mechanisms mentioned above. Future studies will require physiological analysis of whole cells.
The importance of membrane integrity has been demonstrated in many bacteria (Marshall and Gunn, 2015; Putrins et al., 2015; Xie et al., 2016). Activation of complement cascades leads to the formation of the key component C3b on the bacterial surface, resulting in the formation of the MAC that causes cell lysis (Sarma and Ward, 2011). In our study, microscopy revealed severe structural damage and lysis of the cells of TX01Δsip2 following serum treatment, which were not observed in the wild type TX01 or in the sip2 complement strain TX01Δsip2/sip2. In contrast, treatment with heat-inactivated serum failed to cause apparent change in the structure of TX01Δsip2, suggesting that it was the complement in the serum that was responsible for the destruction of TX01Δsip2. These results support a vital role of Sip2 in the protection of E. tarda against complement-mediated killing.
Several reports have shown that hydrogenases are implicated in the virulence of S. enterica (Maier et al., 2004; Zbell et al., 2008; Lamichhane-Khadka et al., 2015). In Helicobacter pylori, hydrogenases participate in the process of energy generation, which is important for efficient bacterial colonization within the acid environment of mouse stomach (Olson and Maier, 2002). In Edwardsiella ictaluri, it has been shown that replication of the bacteria in macrophages required modulation of the pH of the bacteria-containing vacuole through an acid-activated urease (Booth et al., 2009; Baumgartner et al., 2014). In our study, we found that compared to the wild type, TX01Δsip2 completely lost the capacity to replicate in fish PBL, and was significantly impaired in the ability of tissue dissemination and inducing mortality in the host. These results indicate that Sip2 is a virulence factor that is essential to the ability of E. tarda to multiply intracellularly and cause lethal infection.
In S. enterica, hydrogenase Hyb contributes to energy conservation and oxidizes H2 and generates electrons, which are passed through the electron transport chain to terminal acceptors (Zbell et al., 2007). Hyb is important for recycling Hyc-produced H2 during fermentative growth (Redwood et al., 2008). In S. enterica, Hyc and formate dehydrogenases constitute the formate hydrogen lyase complex, which oxidizes formate to produce CO2 and H2 (Rossmann et al., 1991; Sawers, 2005); oxidizing hydrogenases, such as Hyb, are important for bacterial virulence, as the host colonic flora produces highly diffusible H2 (Maier et al., 2004). In our study, we found that compared to the wild type, TX01Δsip2 was significantly impaired in H2-uptake hydrogenase activity, regardless of the pH and iron conditions. These results indicated that Sip2 is associated with H2 uptake in E. tarda.
Previous studies showed that E. ictaluri can survive at low pH but replicates poorly at pHs below 6 (Booth et al., 2009; Baumgartner et al., 2014). Hydrogenases are an important mechanism of bacterial adaption to acidic pH (Ogata et al., 2015). Currently, hydrogenases are grouped into three classes based on the metal cofactor present at the active site, namely [Fe-Fe], [Ni-Fe], and [Fe] hydrogenases (Mangayil et al., 2016), all which possess the iron active site and are involved in adapting to acidic environment (Ogata et al., 2015). In E. coli, S. enterica, and Shigella flexneri, hydrogenase mutants display impaired acid resistance (Hayes et al., 2006; Zbell et al., 2008; Noguchi et al., 2010; McNorton and Maier, 2012; Sui et al., 2017). In our study, we found that the growth of TX01Δsip2 was apparently retarded in acidic medium, and was barely detectable when cultured in iron-deplete medium, suggesting that Sip2 was required for E. tarda growth under acidic conditions. Since iron active site is highly conserved in all hydrogenases, iron depletion likely abolishes the activity of the enzyme. Consistent with these observations, incubation in acidic medium significantly reduced the pH of TX01Δsip2 lysates, suggesting an importance of Sip2 in the regulation of internal pH in E. tarda. These results also suggest that the inability of TX01Δsip2 to replicate in PBL is likely due to the impaired capacity of this mutant to regulate intracellular pH under the highly acidic environment inside the vacuoles such as endolysosomes where E. tarda has been reported to reside (Blum et al., 2017; Sui et al., 2017).
Conclusion
In conclusion, we in this study identified a serum-induced protein of E. tarda that is essential to hydrogenase activity, serum survival, intracellular replication, host infection, and acid resistance. However, the underlining mechanisms of these observations remain unclear. Our results revealed a strong connection between Sip2 and intracellular pH, which raises for future studies questions such as whether Sip2 facilitates intracellular survival of E. tarda by regulating intracellular pH homeostasis.
Author Contributions
LS and M-fL conceived and designed the experiments and wrote the paper. M-fL performed the experiments and analyzed the data.
Funding
This work was supported by the grants from the National Natural Science Foundation of China (31602197 and 31330081), the Youth Innovation Promotion Association of the Chinese Academy of Sciences (2017249), the Huiquan Young Scholar Program of Institute of Oceanology, CAS, and the Taishan Scholar Program of Shandong Province.
Conflict of Interest Statement
The authors declare that the research was conducted in the absence of any commercial or financial relationships that could be construed as a potential conflict of interest.
Supplementary Material
The Supplementary Material for this article can be found online at: https://www.frontiersin.org/articles/10.3389/fmicb.2018.01084/full#supplementary-material
References
Abreu, A. G., and Barbosa, A. S. (2017). How Escherichia coli circumvent complement-mediated killing. Front. Immunol. 8:452. doi: 10.3389/fimmu.2017.00452
Adams, M. W., Mortenson, L. E., and Chen, J. S. (1980). Hydrogenase. Biochim. Biophys. Acta 594, 105–176. doi: 10.1016/0304-4173(80).90007-5
Baumgartner, W. A., Dubytska, L., Rogge, M. L., Mottram, P. J., and Thune, R. L. (2014). Modulation of vacuolar pH is required for replication of Edwardsiella ictaluri in channel catfish macrophages. Infect. Immun. 82, 2329–2336. doi: 10.1128/IAI.01616-13
Berends, E. T., Kuipers, A., Ravesloot, M. M., Urbanus, R. T., and Rooijakkers, S. H. (2014). Bacteria under stress by complement and coagulation. FEMS Microbiol. Rev. 38, 1146–1171. doi: 10.1111/1574-6976.12080
Blum, F. C., Hu, H. Q., Servetas, S. L., Benoit, S. L., Maier, R. J., Maroney, M. J., et al. (2017). Structure-function analyses of metal-binding sites of HypA reveal residues important for hydrogenase maturation in Helicobacter pylori. PLoS One 12:e0183260. doi: 10.1371/journal.pone.0183260
Booth, N. J., Beekman, J. B., and Thune, R. L. (2009). Edwardsiella ictaluri encodes an acid- activated urease that is required for intracellular replication in channel catfish (Ictalurus punctatus). macrophages. Appl. Environ. Microbiol. 75, 6712–6720. doi: 10.1128/AEM.01670-09
Boshra, H., Li, J., and Sunyer, J. O. (2006). Recent advances on the complement system of teleost fish. Fish Shellfish Immunol. 20, 239–262. doi: 10.1016/j.fsi.2005.04.004
Cheng, Z. X., Gong, Q. Y., Wang, Z., Chen, Z. G., Ye, J. Z., Li, J., et al. (2017). Edwardsiella tarda tunes tricarboxylic acid cycle to evade complement-mediated killing. Front. Immunol. 8:1706. doi: 10.3389/fimmu.2017.01706
Crosby, S. N., Snoddy, M. C., Atkinson, C. T., Lee, D. H., and Weikert, D. R. (2013). Upper extremity myonecrosis caused by Edwardsiella tarda resulting in transhumeral amputation: case report. J. Hand Surg. Am. 38, 129–132. doi: 10.1016/j.jhsa.2012.10.009
Dubytska, L. P., Rogge, M. L., and Thune, R. L. (2016). Identification and characterization of putative translocated effector proteins of the Edwardsiella ictaluri type III secretion system. mSphere 1, e00039-16. doi: 10.1128/mSphere.00039-16
Dudek, B., Krzyżewska, E., Kapczyñska, K., Rybka, J., Pawlak, A., Korzekwa, K., et al. (2016). Proteomic analysis of outer membrane proteins from Salmonella enteritidis strains with different sensitivity to human serum. PLoS One 11:e0164069. doi: 10.1371/journal.pone.0164069
Endo, Y., Takahashi, M., and Fujita, T. (2006). Lectin complement system and pattern recognition. Immunobiology 211, 283–293. doi: 10.1016/j.imbio.2006.01.003
Hayes, E. T., Wilks, J. C., Sanfilippo, P., Yohannes, E., Tate, D. P., Jones, B. D., et al. (2006). Oxygen limitation modulates pH regulation of catabolism and hydrogenases, multidrug transporters, and envelope composition in Escherichia coli K-12. BMC Microbiol. 6:89. doi: 10.1186/1471-2180-6-89
Holers, V. M. (2014). Complement and its receptors: new insights into human disease. Annu. Rev. Immunol. 32, 433–459. doi: 10.1146/annurev-immunol-032713-120154
Hovingh, E. S., van den Broek, B., and Jongerius, I. (2016). Hijacking complement regulatory proteins for bacterial immune evasion. Front. Microbiol. 7:2004. doi: 10.3389/fmicb.2016.02004
Hu, Y. H., and Sun, L. (2016). The global regulatory effect of Edwardsiella tarda Fur on iron acquisition, stress resistance, and host infection: a proteomics-based interpretation. J. Proteom. 140, 100–110. doi: 10.1016/j.jprot.2016.04.005
Katharios, P., Kokkari, C., Dourala, N., and Smyrli, M. (2015). First report of Edwardsiellosis in cage-cultured sharpsnout sea bream, Diplodus puntazzo from the Mediterranean. BMC Vet. Res. 11:155. doi: 10.1186/s12917-015-0482-x
King, P. W., and Przybyla, A. E. (1999). Response of hya expression to external pH in Escherichia coli. J. Bacteriol. 181, 5250–5256.
Kwan, P., McIntosh, C. L., Jennings, D. P., Hopkins, R. C., Chandrayan, S. K., Wu, C. H., et al. (2015). The [NiFe]-hydrogenase of Pyrococcus furiosus exhibits a new type of oxygen tolerance. J. Am. Chem. Soc. 137, 13556–13565. doi: 10.1021/jacs.5b07680
Lamichhane-Khadka, R., Benoit, S. L., Miller-Parks, E. F., and Maier, R. J. (2015). Host hydrogen rather than that produced by the pathogen is important for Salmonella enterica serovar Typhimurium virulence. Infect. Immun. 83, 311–316. doi: 10.1128/IAI.02611-14
Lamichhane-Khadka, R., Kwiatkowski, A., and Maier, R. J. (2010). The Hyb hydrogenase permits hydrogen-dependent respiratory growth of Salmonella enterica Serovar Typhimurium. mBio 1:e00284-10. doi: 10.1128/mBio.00284-10
Leung, K. Y., Siame, B. A., Tenkink, B. J., Noort, R. J., and Mok, Y. K. (2012). Edwardsiella tarda virulence mechanisms of an emerging gastroenteritis pathogen. Microbes Infect. 14, 26–34. doi: 10.1016/j.micinf.2011.08.005
Li, G. W., Cai, W. T., Hussein, A., Wannemuehler, Y. M., Logue, C. M., and Nolan, L. K. (2012). Proteome response of an extraintestinal pathogenic Escherichia coli strain with zoonotic potential to human and chicken sera. J. Proteom. 75, 4853–4862. doi: 10.1016/j.jprot.2012.05.044
Li, M. F., Sun, L., and Li, J. (2015a). Edwardsiella tarda evades serum killing by preventing complement activation via the alternative pathway. Fish Shellfish Immunol. 43, 325–329. doi: 10.1016/j.fsi.2014.12.037
Li, M. F., Wang, C., and Sun, L. (2015b). Edwardsiella tarda MliC, a lysozyme inhibitor that participates in pathogenesis in a manner that parallels ivy. Infect. Immun. 83, 583–590. doi: 10.1128/IAI.02473-14
Maier, R. J., Olczak, A., Maier, S., Soni, S., and Gunn, J. (2004). Respiratory hydrogen use by Salmonella enterica serovar Typhimurium is essential for virulence. Infect. Immun. 72, 6294–6299. doi: 10.1128/IAI.72.11.6294-6299.2004
Mangayil, R., Karp, M., Lamminmäki, U., and Santala, V. (2016). Recombinant antibodies for specific detection of clostridial [Fe-Fe] hydrogenases. Sci. Rep. 6:36034. doi: 10.1038/srep36034
Marshall, J. M., and Gunn, J. S. (2015). The O-antigen capsule of Salmonella enterica serovar Typhimurium facilitates serum resistance and surface expression of FliC. Infect. Immun. 83, 3946–3959. doi: 10.1128/IAI.00634-15
Matsuyama, T., Kamaishi, T., Ooseko, N., Kurohara, K., and Iida, T. (2005). Pathogenicity of motile and non-motile Edwardsiella tarda to some marine fish. Fish Pathol. 40, 133–135. doi: 10.3354/dao03051
McNorton, M. M., and Maier, R. J. (2012). Roles of H2 uptake hydrogenases in Shigella flexneri acid tolerance. Microbiology 158, 2204–2212. doi: 10.1099/mic.0.058248-0
Merle, N. S., Church, S. E., Fremeaux-Bacchi, V., and Roumenina, L. T. (2015). Complement system part I- molecular mechanisms of activation and regulation. Front. Immunol. 6:262. doi: 10.3389/fimmu.2015.00262
Milton, D. L., O’Toole, R., Hörstedt, P., and Wolf-Watz, H. (1996). Flagellin A is essential for the virulence of Vibrio anguillarum. J. Bacteriol. 178, 1310–1319. doi: 10.1128/jb.178.5.1310-1319.1996
Mohanty, B. R., and Sahoo, P. K. (2007). Edwardsiellosis in fish: a brief review. J. Biosci. 32, 1331–1344. doi: 10.1007/s12038-007-0143-8
Nelson, J. J., Nelson, C. A., and Carter, J. E. (2009). Extraintestinal manifestations of Edwardsiella tarda infection: a 10-year retrospective review. J. La. State Med. Soc. 161, 103–106.
Noguchi, K., Riggins, D. P., Eldahan, K. C., Kitko, R. D., and Slonczewski, J. L. (2010). Hydrogenase-3 contributes to anaerobic acid resistance of Escherichia coli. PLoS One 5:e10132. doi: 10.1371/journal.pone.0010132
Ogata, H., Krämer, T., Wang, H., Schilter, D., Pelmenschikov, V., van Gastel, M., et al. (2015). Hydride bridge in [NiFe]-hydrogenase observed by nuclear resonance vibrational spectroscopy. Nat. Commun. 6:7890. doi: 10.1038/ncomms8890
Olson, J. W., and Maier, R. J. (2002). Molecular hydrogen as an energy source for Helicobacter pylori. Science 298, 1788–1790. doi: 10.1126/science.1077123
Park, S. B., Aoki, T., and Jung, T. S. (2012). Pathogenesis of and strategies for preventing Edwardsiella tarda infection in fish. Vet. Res. 43:67. doi: 10.1186/1297-9716-43-67
Putrins, M., Kogermann, K., Lukk, E., Lippus, M., Varik, V., and Tenson, T. (2015). Phenotypic heterogeneity enables uropathogenic Escherichia coli to evade killing by antibiotics and serum complement. Infect. Immun. 83, 1056–1067. doi: 10.1128/IAI.02725-14
Redwood, M. D., Mikheenko, I. P., Sargent, F., and Macaskie, L. E. (2008). Dissecting the roles of Escherichia coli hydrogenases in biohydrogen production. FEMS Microbiol. Lett. 278, 48–55. doi: 10.1111/j.1574-6968.2007.00966.x
Ricklin, D., Hajishengallis, G., Yang, K., and Lambris, J. D. (2010). Complement: a key system for immune surveillance and homeostasis. Nat. Immunol. 11, 785–797. doi: 10.1038/ni.1923
Rooijakkers, S. H., and van Strijp, J. A. (2007). Bacterial complement evasion. Mol. Immunol. 44, 23–32. doi: 10.1016/j.molimm.2006.06.011
Rossmann, R., Sawers, G., and Bock, A. (1991). Mechanism of regulation of the formate-hydrogenlyase pathway by oxygen, nitrate, and pH: definition of the formate regulon. Mol. Microbiol. 5, 2807–2814. doi: 10.1111/j.1365-2958.1991.tb01989.x
Sarma, J. V., and Ward, P. A. (2011). The complement system. Cell Tissue Res. 343, 227–235. doi: 10.1007/s00441-010-1034-0
Sawers, R. G. (2005). Formate and its role in hydrogen production in Escherichia coli. Biochem. Soc. Trans. 33, 42–46. doi: 10.1042/BST0330042
Sawers, R. G., Ballantine, S. P., and Boxer, D. H. (1985). Differential expression of hydrogenase isoenzymes in Escherichia coli K-12: evidence for a third isoenzyme. J. Bacteriol. 164, 1324–1331.
Suezawa, C., Yasuda, M., Negayama, K., Kameyama, T., Hirauchi, M., Nakai, T., et al. (2016). Identification of genes associated with the penetration activity of the human type of Edwardsiella tarda EdwGII through human colon epithelial cell monolayers. Microb. Pathog. 95, 148–156. doi: 10.1016/j.micpath.2016.04.007
Sui, Z. H., Xu, H. J., Wang, H. D., Jiang, S., Chi, H., and Sun, L. (2017). Intracellular trafficking pathways of Edwardsiella tarda: from clathrin- and caveolin- mediated endocytosis to endosome and lysosome. Front. Cell. Infect. Microbiol. 7:400. doi: 10.3389/fcimb.2017.00400
Sun, K., Zhang, W., Hou, J., and Sun, L. (2009). Immunoprotective analysis of VhhP2, a Vibrio harveyi vaccine candidate. Vaccine 27, 2733–2740. doi: 10.1016/j.vaccine.2009.03.012
Sun, Y., Zheng, W. J., Hu, Y. H., Sun, B. G., and Sun, L. (2012). Edwardsiella tarda Eta1, an in vivo induced antigen that is involved in host infection. Infect. Immun. 80, 2948–2955. doi: 10.1128/IAI.00063-12
Tamagnini, P., Leitao, E., Oliveira, P., Ferreira, D., Pinto, F., Harris, D. J., et al. (2007). Cyanobacterial hydrogenases: diversity, regulation and applications. FEMS Microbiol. Rev. 31, 692–720. doi: 10.1111/j.1574-6976.2007.00085.x
Thauer, R. K. (2010). Functionalization of methane in anaerobic microorganisms. Angew. Chem. Int. Ed. 49, 6712–6713. doi: 10.1002/anie.201002967
Ueno, Y., Kurano, N., and Miyachi, S. (1999). Purification and characterization of hydrogenase from the marine green alga, Chlorococcum littorale. FEBS Lett. 443, 144–148. doi: 10.1016/S0014-5793(98).01699-8
Walport, M. J. (2001). Complement. First of two parts. N. Engl. J. Med. 344, 1058–1066. doi: 10.1056/NEJM200104053441406
Wang, H., Yoda, Y., Ogata, H., Tanaka, Y., and Lubitz, W. (2015). A strenuous experimental journey searching for spectroscopic evidence of a bridging nickel-iron-hydride in [NiFe] hydrogenase. J. Synchrotron Radiat. 6, 1334–1344. doi: 10.1107/S1600577515017816
Wang, J. J., and Sun, L. (2015). Edwardsiella tarda-regulated proteins in Japanese flounder (Paralichthys olivaceus): identification and evaluation of antibacterial potentials. J. Proteom. 124, 1–10. doi: 10.1016/j.jprot.2015.04.011
Wang, Z., Li, M. Y., Peng, B., Cheng, Z. X., Li, H., and Peng, X. X. (2016). GC-MS-based metabolome and metabolite regulation in serum-resistant Streptococcus agalactiae. J. Proteome Res. 15, 2246–2253. doi: 10.1021/acs.jproteome.6b00215
Xie, F., Li, G., Zhang, W., Zhang, Y., Zhou, L., Liu, S., et al. (2016). Outer membrane lipoprotein VacJ is required for the membrane integrity, serum resistance and biofilm formation of Actinobacillus pleuropneumoniae. Vet. Microbiol. 183, 1–8. doi: 10.1016/j.vetmic.2015.11.021
Yoshida, A., Nishimura, T., Kawaguchi, H., Inui, M., and Yukawa, H. (2005). Enhanced hydrogen production from formic acid by formate hydrogen lyase-overexpressing Escherichia coli strains. Appl. Environ. Microbiol. 71, 6762–6768. doi: 10.1128/AEM.71.11.6762-6768.2005
Zbell, A. L., Benoit, S. L., and Maier, R. J. (2007). Differential expression of NiFe uptake-type hydrogenase genes in Salmonella enterica serovar Typhimurium. Microbiology 153, 3508–3516. doi: 10.1099/mic.0.2007/009027-0
Zbell, A. L., and Maier, R. J. (2009). Role of the Hya hydrogenase in recycling of anaerobically produced H2 in Salmonella enterica serovar Typhimurium. Appl. Environ. Microbiol. 75, 1456–1459. doi: 10.1128/AEM.02064-08
Zbell, A. L., Maier, S. E., and Maier, R. J. (2008). Salmonella enterica serovar Typhimurium NiFe uptake-type hydrogenases are differentially expressed in vivo. Infect. Immun. 76, 4445–4454. doi: 10.1128/IAI.00741-08
Zeng, Z. H., Du, C. C., Liu, S. R., Li, H., Peng, X. X., and Peng, B. (2017). Glucose enhances tilapia against Edwardsiella tarda infection through metabolome reprogramming. Fish Shellfish Immunol. 61, 34–43. doi: 10.1016/j.fsi.2016.12.010
Zhang, B. C., and Sun, L. (2015). Tongue sole (Cynoglossus semilaevis). prothymosin alpha: cytokine-like activities associated with the intact protein and the C-terminal region that lead to antiviral immunity via Myd88-dependent and –independent pathways respectively. Dev. Comp. Immunol. 53, 96–104. doi: 10.1016/j.dci.2015.07.004
Zhang, W., Sun, K., Cheng, S., and Sun, L. (2008a). Characterization of DegQVh, a serine protease and a protective immunogen from a pathogenic Vibrio harveyi strain. Appl. Environ. Microbiol. 74, 6254–6262. doi: 10.1128/AEM.00109-08
Zhang, M., Sun, K., and Sun, L. (2008b). Regulation of autoinducer 2 production and luxS expression in a pathogenic Edwardsiella tarda strain. Microbiology 154, 2060–2069. doi: 10.1099/mic.0.2008/017343-0
Zhou, Z. J., Sun, B. G., and Sun, L. (2015). Edwardsiella tarda Sip1: a serum-induced zinc metalloprotease that is essential to serum resistance and host infection. Vet. Microbiol. 177, 332–340. doi: 10.1016/j.vetmic.2015.03.030
Keywords: Edwardsiella tarda, hydrogenase, serum resistance, acid resistance, virulence
Citation: Li M-f and Sun L (2018) Edwardsiella tarda Sip2: A Serum-Induced Protein That Is Essential to Serum Survival, Acid Resistance, Intracellular Replication, and Host Infection. Front. Microbiol. 9:1084. doi: 10.3389/fmicb.2018.01084
Received: 25 February 2018; Accepted: 07 May 2018;
Published: 25 May 2018.
Edited by:
Alexandre Morrot, Universidade Federal do Rio de Janeiro, BrazilReviewed by:
Robert Maier, University of Georgia, United StatesXuanxian Peng, Sun Yat-sen University, China
Copyright © 2018 Li and Sun. This is an open-access article distributed under the terms of the Creative Commons Attribution License (CC BY). The use, distribution or reproduction in other forums is permitted, provided the original author(s) and the copyright owner are credited and that the original publication in this journal is cited, in accordance with accepted academic practice. No use, distribution or reproduction is permitted which does not comply with these terms.
*Correspondence: Li Sun, bHN1bkBxZGlvLmFjLmNu