- 1Department of Environmental Biology, Centro de Investigaciones Biológicas, Consejo Superior de Investigaciones Científicas, Madrid, Spain
- 2Novo Nordisk Foundation Center for Biosustainability, Technical University of Denmark, Lyngby, Denmark
Nowadays steroid manufacturing occupies a prominent place in the pharmaceutical industry with an annual global market over $10 billion. The synthesis of steroidal active pharmaceutical ingredients (APIs) such as sex hormones (estrogens, androgens, and progestogens) and corticosteroids is currently performed by a combination of microbiological and chemical processes. Several mycobacterial strains capable of naturally metabolizing sterols (e.g., cholesterol, phytosterols) are used as biocatalysts to transform phytosterols into steroidal intermediates (synthons), which are subsequently used as key precursors to produce steroidal APIs in chemical processes. These synthons can also be modified by other microbial strains capable of introducing regio- and/or stereospecific modifications (functionalization) into steroidal molecules. Most of the industrial microbial strains currently available have been improved through traditional technologies based on physicochemical mutagenesis and selection processes. Surprisingly, Synthetic Biology and Systems Biology approaches have hardly been applied for this purpose. This review attempts to highlight the most relevant research on Steroid Biotechnology carried out in last decades, focusing specially on those works based on recombinant DNA technologies, as well as outlining trends and future perspectives. In addition, the need to construct new microbial cell factories (MCF) to design more robust and bio-sustainable bioprocesses with the ultimate aim of producing steroids à la carte is discussed.
Introduction
Steroids are a family of terpenoid lipids widely distributed in nature that present a relatively rigid common structure named gonane formed by four fused alicyclic rings (Figure 1). The oxidation state of the steroid nucleus rings and the presence of different attached functional groups determine the particular biological properties of each steroidic molecule, i.e., its biological function (Lednicer, 2011). Steroidal compounds play important biological roles in different organisms, including cell membrane stabilization and regulation of relevant cellular processes such as cell proliferation and tissue differentiation. For instance, sterols having a hydroxyl group at C-3 and a side-chain of eight or more carbon atoms at C-17 act as stabilizing agents in cell membranes in animals (cholesterol), plants (phytosterols), yeasts and fungi (ergosterol), and certain bacteria (e.g., lanosterol) (Figure 1; Pearson et al., 2003; Lamb et al., 2007; Nelson and Cox, 2012; Wei et al., 2016). Steroidal compounds are also relevant carbon and energy sources for different bacteria (Galán et al., 2017a) and appear participate in various cellular signaling mechanisms.
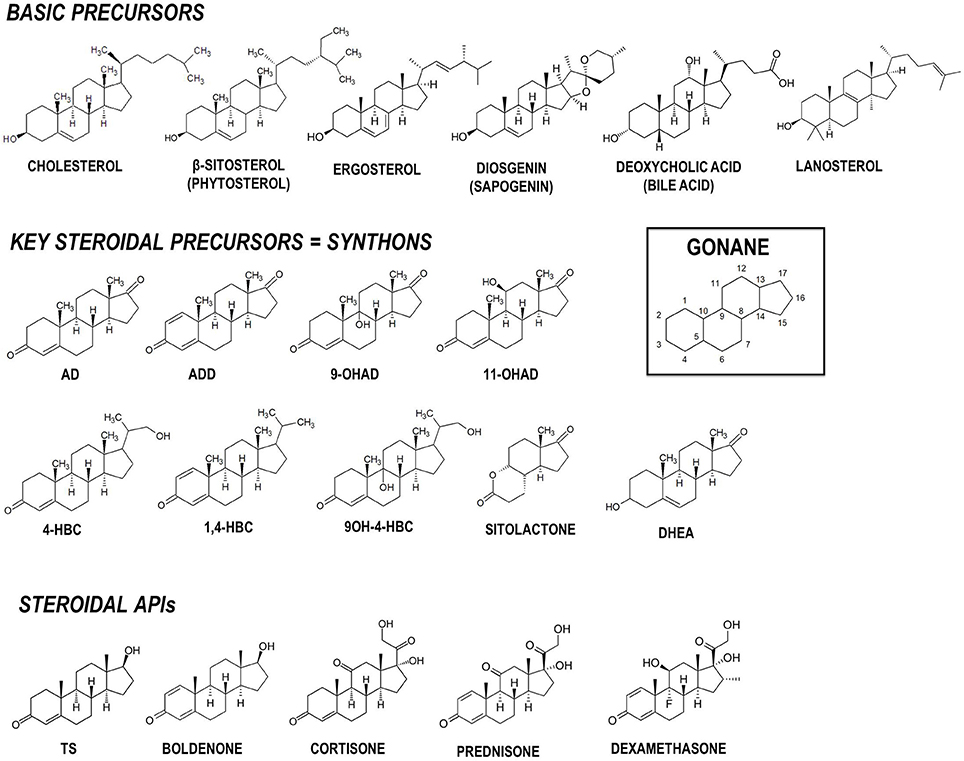
Figure 1. Chemical structures of relevant steroidal compounds containing the gonane tetracyclic skeleton. Structures of basic precursors, key intermediates (synthons) and active pharmaceutical ingredients (APIs) are shown. AD, 4-androstene-3,17-dione; ADD, 1,4-androstadiene-3,17-dione; 9OH-AD, 9α-hydroxy-4-androstene-3,17-dione; 4-HBC, 22-hydroxy-23,24-bisnorchol-4-ene-3-one; 1,4-HBC, 22-hydroxy-23,24-bisnorchol-1,4-diene-3-one; 9OH-4-HBC, 9,22-dihydroxy-23,24-bisnorchol-4-ene-3-one; 11OH-AD, 11α-hydroxy-4-androstene-3,17-dione; DHEA, 3β-hydroxy-5-androstene-7-one, dehydroepiandrosterone; TS, testosterone.
Steroid-based drugs present a broad range of therapeutic applications and represent the highest marketed category of pharmaceuticals after antibiotics with an annual production of more than one million tons. According to Tong and Dong (2009) the annual global market for steroid-containing products exceeds $10 billion with about 300 steroid drugs approved to date (Sedlaczek and Leland, 1988; Demain, 1992; Bureik and Bernhardt, 2007; Donova, 2017). Nevertheless the recent Global Steroids-Corticosteroid Market Research Report 2017 estimates a market size of 3,840 million $ in 2016 and it will reach 4,680 million $ by 2021 (Reports and Market, India). First studies on steroid synthesis took place in the early twentieth century and were notably increased in the 1950s due to the discovery of the pharmacological properties of progesterone and hydrocortisone. Steroidal active pharmaceutical ingredients (APIs) have been classically synthesized by chemical processes (Herráiz, 2017). However, the potential of microbial steroid biotransformation is known since several decades since its application offer a number of advantages over chemical synthesis: (i) regio- and/or stereospecific functionalization of molecules at positions not always available for chemical agents, (ii) multiple consecutive reactions carried out in a single operation step, (iii) more eco-friendly processes (i.e., mild reaction conditions, aqueous media). One of the first demonstrations of this potential was described with the production of cortisone. Traditionally, cortisone was synthesized from deoxycholic acid in a multi-step chemical process (31 steps) characterized by low mass yields (0.16%) and high economic costs (200 $/g, 1949). The incorporation of a biotransformation step with Rhizopus arrhizus ATCC 11145 and Aspergillus niger ATCC 9142 markedly reduced the number of required chemical steps (11 steps) and production costs (1$/g, 1979) of the industrial process (Carballeira et al., 2009). Similarly, other chemical steps have been replaced by microbial bioconversions in steroid synthesis processes in the last decades, leading to more competitive and robust industrial processes. For example, the steroid hormone testosterone (TS) is chemically synthesized from the steroidal intermediate 4-androstene-3,17-dione (AD), which is previously obtained from natural sterols by microbial biotransformation (Fernández-Cabezón et al., 2017a).
In this context, this review aims to compile the most relevant microbial bioprocesses of synthesis and/or functionalization of steroidal compounds described to date, focusing mainly on those designed in the light of the new metabolic engineering approaches. These bioprocesses, conventionally classified within the scope of White or Industrial Biotechnology, have been designed primarily by traditional approaches based on the isolation of microorganisms that produce a molecule of interest or that biocatalyze a particular process and their subsequent improvement through tedious processes of physical or chemical mutagenesis and selection. Nevertheless, in recent years, new bioprocesses have been also designed by recombinant DNA technology approaches, that open up new opportunities for the construction of more robust and versatile microbial cell factories (MCF) for the production of steroids à la carte.
Regardless of the aforementioned approaches, steroid synthesis bioprocesses can be classified into three groups: (i) bioprocesses for production of steroidal intermediates from natural sterols, (ii) bioprocesses for modification and/or functionalization of steroidal molecules, (iii) de novo biosynthesis of steroids (Figure 2). A more detailed description of these bioprocesses is presented below, highlighting the most relevant achievements in the last decades, as well as outlining the trends and perspectives of research into this field. In no case, this review intends to carry out a comprehensive analysis of all the publications, patents and microbial strains described so far.
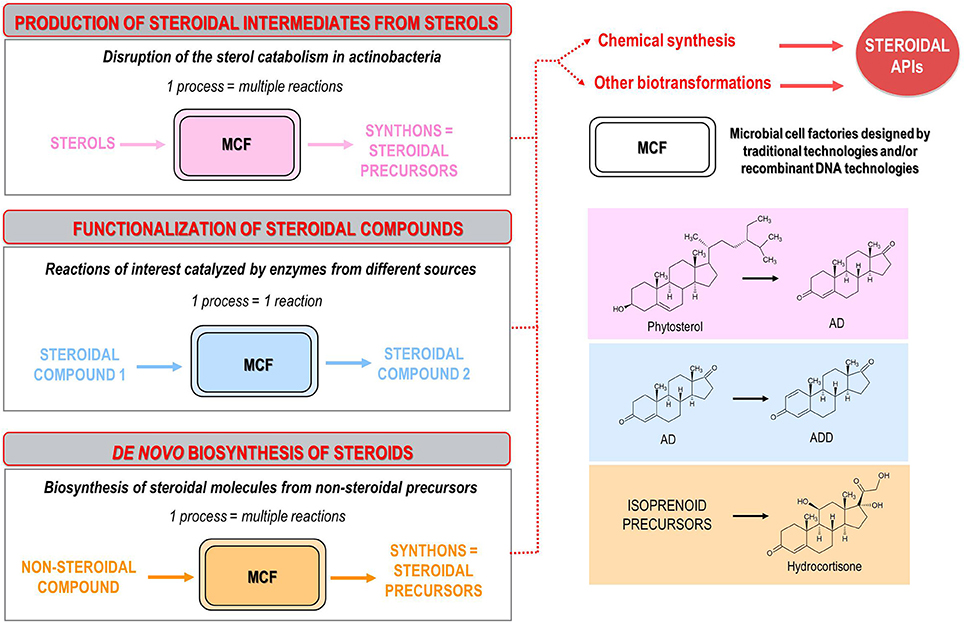
Figure 2. Classification of the microbial bioprocesses for steroid synthesis described to date: (i) production of steroidal intermediates from natural sterols (e.g., bioconversion of phytosterols into ADD); (ii) functionalization of steroidal molecules (e.g., bioconversion of AD into ADD); (iii) de novo biosynthesis of steroids (e.g., biosynthesis of hydrocortisone from non-steroidal substrates). Several microbial strains, isolated from natural sources and improved by conventional mutagenesis or designed by using recombinant DNA technologies, are used as microbial cell factories (MCF) to produce key steroidal intermediates (synthons). The resulting synthons are subsequently modified by chemical steps or additional bioconversion processes to synthetize final steroidal active pharmaceutical ingredients (APIs).
Current Achievements of Steroid Biotechnology
Microbial Production of Steroidal Intermediates From Sterols
Natural sapogenins such as diosgenin have been used as basic precursors in the steroid chemistry for decades (Figure 1; Herráiz, 2017). For example, 16-dehydropregnenolone acetate derived from diosgenin can be converted to valuable steroids by chemical synthesis processes (Hanson, 2005; Laveaga, 2005). Diosgenin can be also transformed into steroidal intermediates with therapeutic activities by microbial biotransformation (Wang et al., 2007; Wang F. Q. et al., 2009; Wang W. et al., 2009). Nevertheless, the utilization of sapogenins as feedstock has been significantly reduced since it presents several drawbacks: high costs, multiple steps, low yields, and wild plant resources depletion (Tong and Dong, 2009; Wang et al., 2011). Therefore, sapogenins have been progressively replaced by several natural sterols (e.g., phytosterols, cholesterol) that can also be biotransformed into steroidal derivatives with properties similar to certain sex hormones (Figure 1). These steroidal derivatives, which are actually intermediates or byproducts of the sterol catabolic pathway (e.g., AD) (see below), are currently used as key precursors (synthons) for the chemical synthesis of steroid-based drugs such as corticosteroids, mineralocorticoids and oral contraceptives. Several types of phytosterols (e.g., soybean, pine, paper industry wastes) are used generally as industrial feedstock instead of cholesterol (obtained from animal fats and oils), due to the exhaustive quality controls required for the use of any type of animal basic precursor (Fernandes et al., 2003; Malaviya and Gomes, 2008).
In general, actinobacteria producing mycolic acids such as those belonging to the genera Gordonia, Mycobacterium, Nocardia, and Rhodococcus, are able to metabolize cholesterol and other natural sterols (Donova, 2010; García et al., 2012; Galán et al., 2017a). In last decades numerous members of these genera have been isolated from different natural sources (e.g., soil) and subsequently optimized by conventional mutagenesis to select bacterial strains that produce steroidal intermediates of interest. For example, the C-19 steroids (e.g., AD, ADD, 9OH-AD, and TS), the C-22 steroids (e.g., 4-HBC, 1,4-HBC, 9OH-4-HBC) and the sitolactone intermediate are currently produced from sterols by microbial bioconversion (Figure 1). Interestingly, only strains of the genus Mycobacterium are used for production purposes at an industrial scale (Marsheck et al., 1972; Imada and Takahashi, 1980; Knight and Wovcha, 1981; Liu and Meng, 1981; Liu et al., 1994; Lo et al., 2002; Donova et al., 2005a,b; Wei et al., 2010a; Supplementary Table 1). New steroid-producing strains have also been rationally designed by metabolic engineering approaches in recent years (Table 1). Since certain C-19 steroids (e.g., AD, ADD, 9OH-AD) have been postulated as intermediates of the (chole)sterol catabolic pathway in actinobacteria (García et al., 2012; Galán et al., 2017a), redirecting metabolic fluxes toward their accumulation can be addressed by gene deletion and/or overexpression (Figure 3). For instance, the mutant strains of Mycobacterium smegmatis named MS6039 (ΔMSMEG_6039, ΔkshB1) and MS6039-5941 (ΔMSMEG_6039 ΔMSMEG_5941, ΔkshB1 ΔkstD1) mainly produced ADD and AD from sterols, respectively (Galán et al., 2017b). Several multiple-gene-deletion mutants of M. neoaurum ATCC 25795 were developed to produce 9OH-AD or C-22 derivatives from sterols (Yao et al., 2014; Xu et al., 2016). Furthermore, the overepression of the kshA gene in the 9OH-AD-producing mutant of M. neoaurum contributed greatly to improve the process selectivity by reducing the accumulation of undesirable byproducts (e.g., AD) that impair downstream purification processes (Yao et al., 2014). Wei et al. (2010b) also engineered an AD-producing mutant by deleting the kstD gene of M. neoaurum NwlB-01, which had been previously isolated from soil samples and selected because of its ability to accumulate mixtures of AD/ADD from phytosterols. Alternatively, Wei et al. (2014) improved the production of ADD in the strain NwlB-01 by the overexpression of various kstD genes from different sources. Similarly, Yuan et al. (2015) constructed a 9OH-AD-producing strain by overexpressing the kshA and kshB genes from M. smegmatis mc2155 and Gordonia neofelifaecis, respectively, in the strain Mycobacterium sp. NRRL B-3805 lacking 9α-hydroxylase activity. Finally, Fernández-Cabezón et al. (2017a) overexpressed two 17β-HSD genes from different sources in M. smegmatis MS6039-59410 with the objective of producing testosterone from sterols in a single fermentation step.
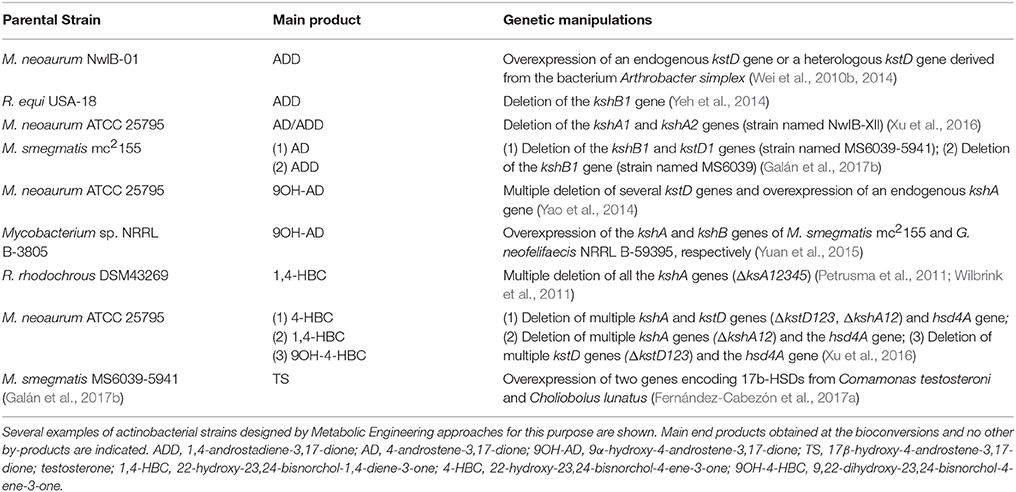
Table 1. Microbial production of steroidal intermediates from natural sterols by recombinant DNA technology approaches.
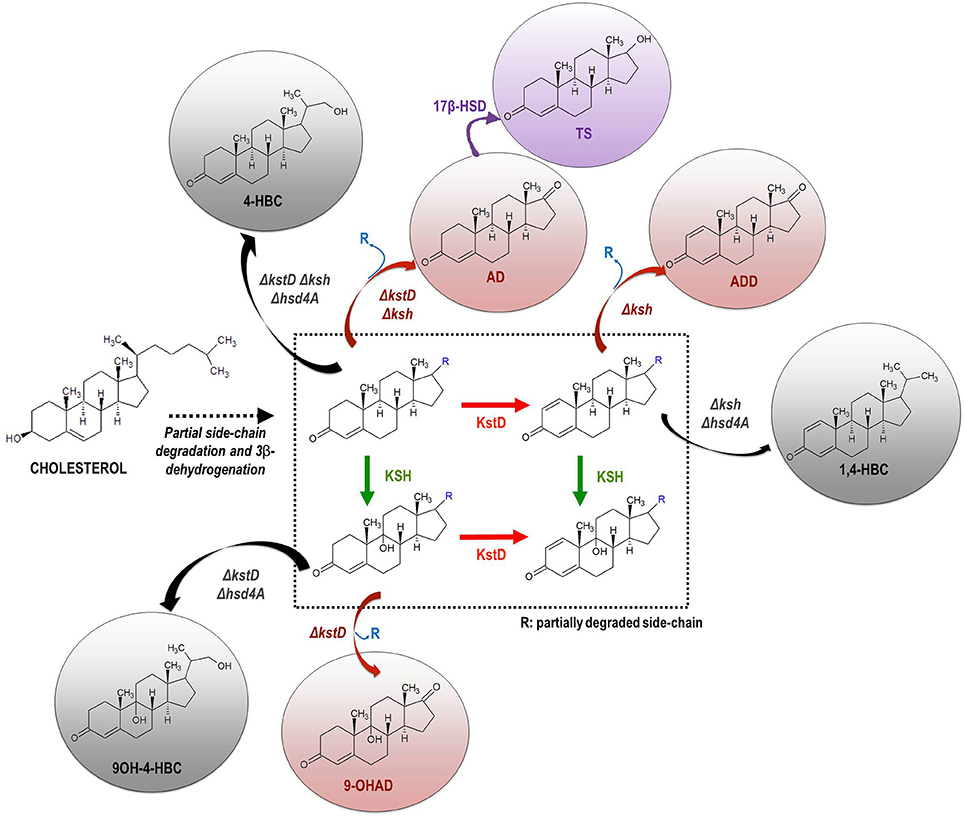
Figure 3. Scheme of the rational construction of mycobacterial strains producing steroidal intermediates from natural sterols by metabolic engineering approaches. Based on the knowledge of the catabolic pathway of sterols in actinobacteria, the redirection of metabolic flux toward the accumulation/synthesis of C-19 (red) or C-22 (gray) steroidal intermediates can be addressed via single/multiple gene deletions. The metabolic flux can be also redirected to the synthesis of new steroidal compounds by incorporating heterologous enzymatic activities (pink) (e.g., 17β-hydroxysteroid dehydrogenase, 17β-HSD). kstD, 3-ketosteroid-Δ1-dehydrogenase; ksh, 3-ketosteroid-9α-hydroxylase; hsd4A, hydroxysteroid dehydrogenase. Abbreviations of metabolites: ADD, 1,4-androstadiene-3,17-dione; AD, 4-androstene-3,17-dione; 9OH-AD, 9α-hydroxy-4-androstene-3,17-dione; TS, 17β-hydroxy-4-androstene-3,17-dione; testosterone; 1,4-HBC, 22-hydroxy-23,24-bisnorchol-1,4-diene-3-one; 4-HBC, 22-hydroxy-23,24-bisnorchol-4-ene-3-one; 9OH-4-HBC, 9,22-dihydroxy- 23,24-bisnorchol-4-en-3-one.
Other authors have attempted to construct mutant strains producing steroidal intermediates from sterols in species of the genus Rhodococcus. However, the presence of multiple steroid catabolic pathways apparently convergent and the existence of basal levels of certain isoenzymes (e.g., KstD, KshA, or KshB) have hindered the development of stable producers in these species (van der Geize et al., 2000, 2001, 2002a,b, 2008; Petrusma et al., 2011; Fernández de las Heras et al., 2012; Liu et al., 2016; Guevara et al., 2017a,b). In certain members of the genus Mycobacterium such as M. smegmatis, M. tuberculosis or M. neoaurum, the deletion of a single key gene (kshA, kshB, or kstD) appears to be sufficient to prevent an efficient mineralization of sterols (Brzostek et al., 2005; Andor et al., 2006; Capyk et al., 2009; Brzezinska et al., 2013; Yao et al., 2014; Galán et al., 2017b). In contrast, the deletion of five kshA genes was needed to construct a mutant strain of Rhodococcus rhodochrous DSM43269 incapable of mineralizing sterols (Petrusma et al., 2011). Similarly, two kstD genes were deleted into R. ruber str. Chol-4 to avoid cholesterol mineralization (Fernández de las Heras et al., 2012). So far, only Yeh et al. (2014) were able to design an ADD-producing strain of Rhodococcus equi by deleting only one kshB gene. Moreover, the equivalent mutants of the genus Mycobacterium and Rhodococcus accumulate different types and/or amounts of steroidal intermediates from sterols. For instance, AD/ADD-producing mycobacterial strains accumulate small amounts of 4-HBC/1,4-HBC alcohols, whereas the respective mutants of Rhodococcus accumulate notable amounts of their acid derivatives 3-oxo-23,24-bisnorchol-4-en-22-oic acid (4-BNC) and 3-oxo-23,24-bisnorchol-1,4-dien-22-oic acid (1,4-BNC) (Marsheck et al., 1972; Wilbrink et al., 2011; Yeh et al., 2014; Xu et al., 2016; Galán et al., 2017b). For example, Wilbrink et al. (2011) constructed a mutant strain of R. rhodochrous DSM 43269 (strain RG32) devoid of 9α-hydroxylase activity, which accumulated mostly 1,4-BNC and only small amounts of ADD from sterols.
The successful implementation of the aforementioned metabolic engineering approaches would not have been possible without the numerous studies of catabolic pathways of steroids developed in various model actinobacteria (e.g., M. smegmatis mc2155, R. jostii RHA1, M. tuberculosis H37Rv), the development of new molecular biology tools for genetic manipulation of these non-model bacteria and the sequencing and annotation of their genomes (Kendall et al., 2007, 2010; van der Geize et al., 2007; Mohn et al., 2012; Uhía et al., 2012; Haußmann et al., 2013; Li et al., 2014; Bergstrand et al., 2016; Crowe et al., 2017).
Microbial Functionalization of Steroidal Molecules
The chemical modification of steroidal molecules has attracted considerable attention in last decades. Baeyer-Villiger oxidations, hydroxylations, and reduction-oxidation (redox) reactions are some of the most relevant modifications (functionalizations) performed on steroidal compounds by chemical synthesis processes. These and other functionalizations of steroids can alternatively be performed by biocatalytic procedures. For example, redox reactions such as oxidations of alcohols, reductions of carbonyl groups, dehydrogenations of C-C bonds or hydrogenations of C-C double bonds, can be catalyzed by specific enzymes. The Δ1,2-dehydrogenation and the 17β-reduction of 3-ketosteroids are two examples of enzymatic redox reactions of relevance for steroid synthesis (Figure 4). Hydroxylations of steroidal molecules, in which a hydrogen atom is replaced by a hydroxyl group (e.g., 7α, 9α-, 11α-, 11β-, 16α-, and 17α-hydroxylation), can also be catalyzed by several families of hydroxylase enzymes such as multicomponent cytochromes P450 and monooxygenases (Figure 4). Even the classical Baeyer-Villiger oxidations, in which an ester/lactone is generated from a ketone/cyclic ketone (e.g., transformation of ADD into testolactone, an aromatase inhibitor) (Kołek et al., 2008), can be catalyzed by enzymes called Baeyer-Villiger monooxygenases (BVMOs) that use molecular oxygen and NADPH (Figure 4; Leisch et al., 2011). To perform these chemical functionalizations on steroidal molecules, several wild-type microorganisms that possess the steroid-modifying enzymatic activities of interest are frequently used at an industrial scale (Supplementary Table 2; Zhang et al., 1998; El-Kadi and Mostafa, 2004; Li et al., 2005, 2017; Reese, 2007; Roglič et al., 2007; Manosroi et al., 2008; Yildirim et al., 2010; Peart et al., 2011; Bhatti and Khera, 2012; Donova and Egorova, 2012; Prakash and Bajaj, 2017). Since these bioconversions often exhibit various drawbacks such as low selectivity and substrate conversion yields, and may even require the culture of opportunistic pathogenic microorganisms (e.g., Rhizopus oryzae), it has been proposed the design of alternative MCFs by using recombinant DNA technologies in recent years. Several model microorganisms such as Escherichia coli, Bacillus subtilis, or Saccharomyces cervisiae have been chosen to heterologously express one or more genes encoding the proteins involved in the single enzymatic steps of interest mentioned above (i.e., 9α-hydroxylation and Δ1,2-dehydrogenation of 3-ketosteroids) (Figure 4, Table 2; Andor et al., 2006; Arnell et al., 2007; Li et al., 2007; Petrusma et al., 2009; Zhang W. et al., 2013; Zhang et al., 2016; Shao et al., 2017). Non-conventional yeasts and bacteria (e.g., Schizosaccharomyces pombe, Pseudomonas putida) have been also used for this purpose (Ruijssenaars et al., 2007; Hakki et al., 2008; Petrič et al., 2010 Table 2). To our knowledge, none of the bioprocesses designed by using recombinant DNA technologies has been industrially implemented due to the low achieved substrate conversions that seem to be the consequence of an inefficient transport of steroids. For this reason, the rational genetic improvement of microorganisms capable of efficiently transporting and modifying steroids may be a challenging approach to follow. Zhang W. et al. (2013) increased successfully the 3-ketosteroid-Δ1-dehydrogenase activity of an Arthrobacter simplex strain by genomic integration of multiple copies of the endogenous ksdD gene under the control of a constitutive promoter, reaching with the recombinant strain a conversion yield of substrate 32.9% higher than that of wild-type strain. Similarly, Kiss et al. (2015) increased the endogenous 15β-hydroxylase activity in B. megaterium by overexpressing the cytochrome CYP106A2.
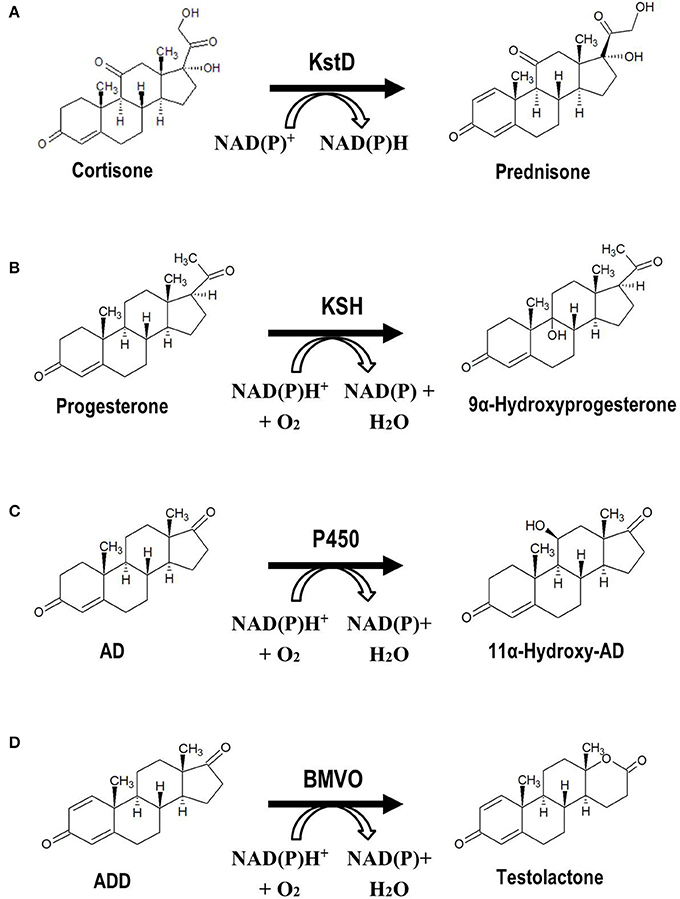
Figure 4. Microbial functionalization of steroidal molecules of industrial relevance. (A) Δ1-Dehydrogenation of 3-ketosteroids: conversion of cortisone into prednisone. (B) 9α-Hydroxylation of 3-ketosteroids: conversion of progesterone into 9α-hydroxy-progesterone. (C) 11α-Hydroxylation of 3-ketosteroids: conversion de AD into 11α-hydroxy-AD. (D) Baeyer-Villiger oxidation: conversion of ADD into testolactone. KstD, 3-ketosteroid-Δ1-dehydrogenase, KSH, 3-ketosteroid-9α-hydroxylase, P450, 11α-hydroxylase, cytochrome P450, BMVO, Baeyer-Villiger monooxygenase.
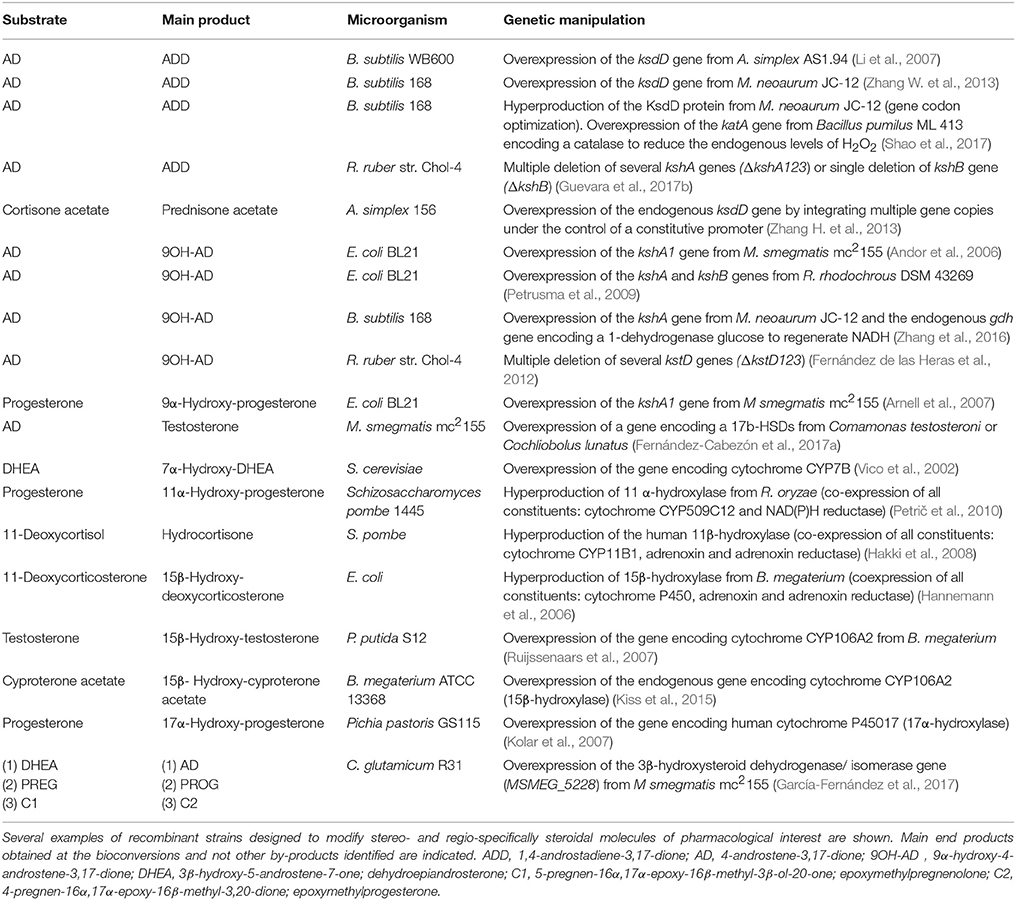
Table 2. Microbial functionalization of steroidal molecules by recombinant DNA technology approaches.
Alternatively, several authors have developed high-yield bioconversions of steroids based on in vitro enzymatic processes that use recombinant proteins purified or obtained as cleared cell lysate and that frequently incorporate a couple enzyme to recycle the cofactor consumed in the reaction (i.e., NAD(P)/H) (Fogal et al., 2013; Hoebenreich et al., 2017). None of these bioprocesses has been implemented industrially to date due to the high production cost of active recombinant enzymes.
De novo Biosynthesis of Steroids in Yeasts
Several microbial bioprocesses designed by synthetic biology and system biology approaches have been successfully implemented for the industrial production of high-added value compounds (Lee et al., 2016; Nielsen and Keasling, 2016; Silber et al., 2016). However, steroid industry has hardly explored this path, and there are only two examples that highlight the potential of these disciplines. De novo biosynthesis of progesterone and hydrocortisone from simple carbon sources (e.g., galactose, ethanol) was successfully achieved in recombinant strains of Saccharomyces cerevisiae, by engineering the endogenous sterol biosynthesis pathway to generate a cholesterol-like molecule that served as a precursor to a multi-enzymatic heterologous route mimicking human steroid biosynthesis (Duport et al., 1998; Szczebara et al., 2003).
Current Challenges of Steroid Biotechnology
Constructing a Second Generation of Mycobacterial Strains
The economic feasibility of certain industrial bioprocesses for steroid production is limited by low sterol conversion yields and low product selectivity achieved with the currently available Mycobacterium strains. Consequently, the construction of a second generation of MCFs with improved properties is one of the most relevant short-term challenges for the production of steroidal intermediates from sterols. By implementing the knowledge related to the bacterial steroid catabolism acquired in recent years and a combination of empirical methodologies proposed below, new more robust industrial bioprocesses may be designed.
In last years the genome sequencing and annotation of several industrial mycobacterial strains obtained by traditional approaches has been reported (e.g., Mycobacterium sp. NRRL B-3805, Mycobacterium sp. NRRL B-3683, Mycobacterium sp. VKM Ac-1815D) (Bragin et al., 2013; Shtratnikova et al., 2014, 2015; Rodríguez-García et al., 2016). The mutations present in these industrial strains have also been determined with the aim of identifying potential genetic targets for a rational design of new highly-producer MCFs. Complementary approaches to conventional mutagenesis such as the Adaptive Laboratory Evolution (ALE) can be applied for this same purpose. The ALE methodology has already been implemented to optimize numerous bioprocesses over the last 25 years (Dragosits and Mattanovich, 2013). Taking into account that overproduction of compounds and high stress tolerances are determined by interaction of multiple parameters/factors, that are not always known and predictable and therefore cannot be easily addressed through rational approaches, the ALE methodology based on long-term adaptation of microbial strains can be an interesting alternative. By progressively increasing the sterol concentration of the medium in a chemostat or in several consecutive batch cultures, ALE evolved strains that efficiently biotransform sterols into steroidal intermediates could be selected (Mondaca et al., 2017). In addition, ALE methodology could be combined with reverse engineering strategies, which seek to “rationalize” the selected phenotypes by identifying the mutations present in the evolved strains and improve them, if possible, in new rounds of evolution.
A better understanding of the steroid catabolic complexity may be also key to optimizing several current industrial bioprocesses in actinobacteria. The partial degradation of products described in many mycobacterial strains could be avoided through the elimination of undesirable enzymatic activities via rational gene deletion. Yao et al. (2014) demonstrated that two residual enzymatic activities KstD (KstD2 and KstD3) present in the mutant MutMN−KSTD1 (ΔkstD1) of M. neoaurum ATCC 25795 markedly decreased the conversion yield of 9OH-AD from sterols. Interestingly, KstD2 and KstD3 enzymes are encoded by genes located in a second steroid gene cluster that is homologous to the C-19+ cluster recently described in M. smegmatis, which has apparently evolved independently from the upper cholesterol kstR-regulon and appears to be necessary for the efficient metabolization of C-19 steroids in this bacterium (i.e., AD, ADD, 9OH-AD) (Fernández-Cabezón et al., 2017b). Certain industrial mycobacterial strains such as M. neoaurum VKM Ac-1815D and Mycobacterium sp. NRRL B-3805 do not have the C-19+ gene cluster. In fact, a genomic deletion of this cluster is found when their genomes are compared to the genome of the close phylogenetic strain M. neoaurum DSM 44074 (ATCC 25795). Therefore, the deletion of the C-19+ gene cluster or of certain genes located therein (e.g., kstD, kshA, kshB), may increase the bioconversion yields of those producer strains derived from M. smegmatis, M. neoaurum or other mycobacteria carrying the C-19+ cluster. On the other hand, the identification of enzymes involved in each of the steps of sterol catabolism may be useful for the construction of new strains producing new steroidal intermediates of industrial interest (e.g., 4-HBC, 1,4-HBC, 9-OH-4-HBC) (Xu et al., 2016), as well as in the determination of limiting enzymatic steps leading to low conversion yields and accumulation of undesirable by-products. For example, a deep understanding of the enzymatic steps involved into the sterol side-chain degradation could be useful for the design of new AD/ADD-producing strains that do not accumulate the intermediates 4-HBC/1,4-HBC. In order to further understand the regulatory mechanisms of sterol catabolism and steroid production, several transcriptomic and proteomic studies have been also done in recent years, increasingly focused on higher-producer strains (Liu et al., 2016a,b; Xiong et al., 2017a,b).
The characterization of the C-19+ gene cluster above mentioned, as well as of other steroid degradation gene clusters in bacteria, has allowed to identify multiple steroid-modifying isoenzymes with different catalytic properties (e.g., substrate specificity, cellular localization) (Knol et al., 2008; Petrusma et al., 2011, 2012; Penfield et al., 2014; Guevara et al., 2017a,b; Wang et al., 2017), that should be considered to design more efficient industrial bioprocesses. For example, the selective production of ADD from sterols in a single fermentation step remains a challenge at an industrial scale. To date, all available mycobacterial strains accumulate mixtures AD/ADD that hamper the subsequent purification processes (Marsheck et al., 1972; Wei et al., 2010b; Galán et al., 2017b). Several hypotheses have been raised about the impossibility of achieving bioconversions close to 100%: (i) existence of certain enzymatic activities 1-en reductase that catalyze the reverse reaction (Goren et al., 1983; Egorova et al., 2002); (ii) phenomena of inhibition and/or toxicity because of the ADD accumulation (Smith et al., 1993); (iii) insufficient levels of enzymatic activity KstD (Wei et al., 2014). On the basis of this latter hypothesis, several authors have overexpressed endogenous or heterologous kstD genes (involved into the sterol catabolism) in ADD-producing strains that have led to remarkable improvements in bioconversion selectivity but still insufficient (Wei et al., 2010b, 2014). Surprisingly, the utilization of alternative kstD genes involved into different steroid catabolic pathways or even a combination of kstD genes have not been explored yet. For instance, the simultaneous overexpression of the kstD1 (MSMEG_5941) and kstD2 (MSMEG_2869) genes from M. smegmatis involved into the cholesterol and C-19 steroids catabolism, respectively, could be investigated in ADD-producing strains with the aim of favoring the Δ1,2-dehydrogenation reactions in different steps (on different substrates) during the sterol bioconversion (Supplementary Figure 1).
Finally, the construction of new MCFs is necessary for the direct conversion of sterols into steroidal compounds that are not part of the steroid catabolism in bacteria (e.g., 11α-OHAD, 11β-OHAD, 17β-OHAD). As mentioned above, most of these compounds are currently synthesized in a second fermentation step from C-19 or C-22 steroidal intermediates. The need for a second fermentation involves further purification steps of intermediate products and a significant increase in the time and cost of the overall process. Therefore, the development of one-step bioprocesses by incorporating new steroid-modifying enzymatic activities in certain mycobacterial strains may be a convenient approach. For example, the overexpression of several heterologous genes encoding 11α-hydroxylases in the AD-producing mutant M. smegmatis MS6039-5941 have been already proposed to synthesize 11α-OHAD from (phyto)sterols in a one-step fermentation (Ríos et al., 2017). However, given that most of these enzymatic reactions are catalyzed by membrane-associated multicomponent cytochromes P450 which are very abundant in their native microorganisms (e.g., certain species of fungi have among several tens to a hundred of cytochromes P450), significant efforts will be required to identify the genes encoding the enzymatic activities of interest, as well as to achieve an adequate production of active enzyme that allows redirecting fully (or mostly) the metabolic flow toward the formation of steroidal product.
Microbial Functionalization of Steroids
The challenges proposed above are based on the utilization of mycobacterial species as MCFs. However, it is likely that the biosynthesis of a broad portfolio of steroids cannot be achieved by using a single microbial chassis as MCF. Therefore, the identification of alternative microbial chassis, i.e., alternative microorganisms with desirable properties (e.g., efficient steroid transport, high stress tolerance, non-pathogenicity, absence of undesired steroid-modifying enzymatic activities, availability of multiple genetic modification tools), must be investigated. For example, the utilization of the well-known industrial actinobacteria Corynebacterium glutamicum has been recently applied for designing new functionalization bioprocesses of steroids (García-Fernández et al., 2017).
On the other hand, the identification of novel steroid-modifying enzymes is also required for the design of new bioprocesses. To do this, several approaches such as the exploration of alternative ecological niches (e.g., marine, anoxic and thermopile environments) by means of metagenomic approaches, the annotation of genomes available in database, the development of new proteins by protein engineering and the implementation of high-throughput assays for strain screening can be applied. For example, Ribeiro et al. (2017) have investigated the identification of thermostable enzymes for developing in vitro bioconversions of steroids, since thermostability can increase not only the enzyme half-life but also the solubility of steroids notably improving reaction yields.
De novo Biosynthesis of Steroids in Actinobacteria
Most of the limitations observed in current industrial bioprocesses are directly related to the intrinsic properties of steroidal molecules (e.g., low solubility in aqueous media, high cell toxicity). With the aim of overcoming these limitations, different technological approaches such as micronization or emulsification with surfactants of steroidal substrates, utilization of solvent organics in two-phase systems, cell immobilization and development of in continuous or in situ production systems have been investigated in recent years (de Carvalho et al., 2004; Donova et al., 2007; Egorova et al., 2009; Lam, 2010; Marques et al., 2010; Donova and Egorova, 2012; Galán et al., 2017a).
Alternatively, the development of MCFs capable of de novo synthesizing steroids from conventional feedstock such as glucose has been proposed. In this sense, it was mentioned previously that the de novo biosynthesis of progesterone and hydrocortisone was successfully achieved in recombinant strains of S. cerevisiae (Duport et al., 1998; Szczebara et al., 2003). Similarly, de novo biosynthesis of steroids in mycobacteria might be also achieved (Supplementary Figure 2). Briefly, taking account that steroids are part of the isoprenoid family that are synthesized in all organisms through a modular synthesis pattern, it is possible to channel the metabolic flux of the universal isoprenoid precursors (i.e., isopentenyl pyrophosphate, IPP; dimethylallyl pyrophosphate, DMAPP) toward the synthesis of squalene through a limited number of heterologous enzymatic steps and, from this compound, to generate by cyclization the steroidal molecule lanosterol. Thus, it is possible to assemble an artificial biosynthetic route of sterols with a partially-interrupted cholesterol catabolic pathway to synthesize steroidal intermediates of interest. The bioconversion of lanosterol into steroidal intermediates of interest by Mycobacterium sp. NRRL B-3805 has been already described (Wang et al., 1995). Nevertheless, for a successful implementation on an industrial scale of the approaches detailed here, new genetic tools must be designed. In recent years significant advances have been made in the development of genome-editing tools (e.g., recombineering-assisted by CRISPR-Cas9), which facilitate the construction of microbial strains for different purposes such as the industrial applications of White Biotechnology (Pines et al., 2015; Jakočiunas et al., 2016). These tools allow the simultaneous introduction of several types of genomic modifications, shortening the time of strain development and avoiding the use of selection markers. At present, a recombineering system for the genomic modification of mycobacteria is available (Murphy et al., 2015) and a CRISPR-Cas9 interference system (CRISPRi) has been successfully implemented (Choudhary et al., 2015; Rock et al., 2017), which opens the possibility of designing even more versatile genome editing systems in mycobacteria (e.g., recombineering assisted by CRISPR-Cas9). For instance, Yan et al. (2017) have recently developed a recombineering assisted by CRISPR-Cas12a (Cpf1) (i.e., a new type of CRISPR-Cas system), that allows an efficient editing of M. smegmatis genome. Moreover, in the next few years, additional efforts must be also made to construct promoter libraries of different strength for fine-tuned gene expression in mycobacteria (Kanno et al., 2016), as well as the engineering of regulatory circuits for an optimal control of the heterologous gene expression (Bradley et al., 2016).
Concluding Remarks
The biotechnological production of steroids as other industrial bioprocesses, such as the production of amino acids or antibiotics, has been traditionally based on the isolation of microorganisms of interest and their subsequent improvement through physicochemical mutagenesis and selection. Although in recent years the optimization of several bioprocesses has been addressed through recombinant DNA technology approaches, these techniques have not been hardly applied for steroid synthesis (Lee et al., 2016; Nielsen and Keasling, 2016; Silber et al., 2016). This fact could be in part explained by the intrinsic difficulties associated with the utilization of steroids (e.g., low solubility in aqueous media, high cell toxicity) and the difficult genetic manipulation and bad reputation of the MCFs commonly used in these bioprocesses (i.e., mycobacterial species). Nevertheless, this trend is changing as we have showed in this review highlighting the most relevant research carried out on steroid biotechnology based on recombinant DNA technologies. In our opinion, the main challenge to become competitive with the current industrial chemical processes is to design more robust bioprocesses with higher substrate conversion yields and product selectivity. An additional challenge will be to offer a new portfolio of steroids with the ultimate goal of producing steroids à la carte. To achieve all these challenges, it will be necessary to construct new MCFs based on the implementation of Synthetic Biology and Systems Biology approaches (Figure 5). The long road that must be undertaken to design bio-sustainable processes such as those proposed here is justified by the growing demand for steroidal pharmaceuticals and the commitment increasingly needed with the environment.
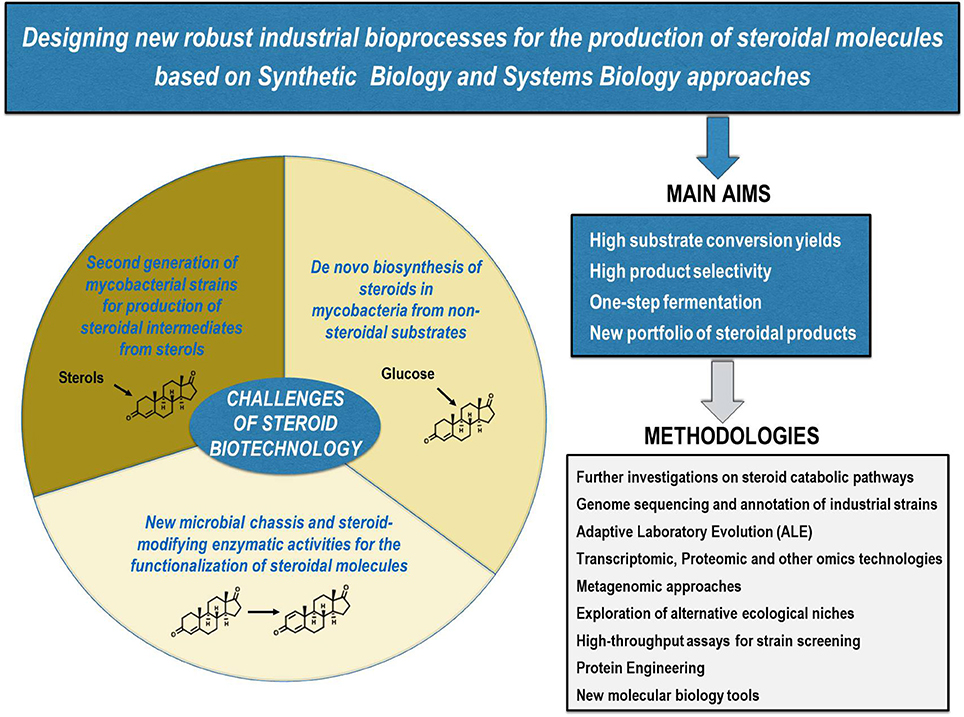
Figure 5. Current challenges of steroid biotechnology. New microbial cell factories are needed to design new industrial bioprocesses for the production of steroids à la carte, i.e., more robust one-step biotechnological processes with higher substrate conversion yields and product selectivity. Using multiple currently available methodologies, at least the following challenges should be addressed: (i) construction of a second generation of mycobacterial strains for the production of steroidal intermediates from sterols; (ii) identification of new microbial chassis and steroid-modifying enzymatic activities for the functionalization of steroidal molecules; (iii) de novo biosynthesis of steroids from non-steroidal substrates in mycobacteria.
Author Contributions
LF-C and JG: Conceived and designed the work; LF-C, JG, and BG: prepared the figures, and wrote the manuscript; JG: Ensured the financial and material resources; LF-C, BG, and JG: Reviewed the final draft.
Funding
This work was supported by grants from the Ministry of Science and Innovation (BFU2006-15214-C03-01, BFU2009-11545-C03-03) and Ministry of Economy and Competitiveness (BIO2012-39695-C02-01, BIO2015-66960-C3-3-R, RTC-2014-2249-1). Project RTC-2014-2249-1 was co-financed with FEDER funds. LF-C was supported by an FPU fellowship from the Spanish Ministry of Education, Culture and Sports.
Conflict of Interest Statement
The authors declare that the research was conducted in the absence of any commercial or financial relationships that could be construed as a potential conflict of interest.
Acknowledgments
We thank the Spanish Research Council (CSIC), and the Ministry of Economy and Competitiveness for supporting this work.
Supplementary Material
The Supplementary Material for this article can be found online at: https://www.frontiersin.org/articles/10.3389/fmicb.2018.00958/full#supplementary-material
References
Andor, A., Jekkel, A., Hopwood, D. A., Jeanplong, F., Ilkoy, E., Kónya, A., et al. (2006). Generation of useful insertionally blocked sterol degradation pathway mutants of fast-growing mycobacteria and cloning, characterization, and expression of the terminal oxygenase of the 3- ketosteroid 9alpha-hydroxylase in Mycobacterium smegmatis mc(2)155. Appl. Environ. Microbiol. 72, 6554–6559. doi: 10.1128/AEM.00941-06
Arnell, R., Johannisson, R., Lindholm, J., Fornstedt, T., Ersson, B., Ballagi, A., et al. (2007). Biotechnological approach to the synthesis of 9alpha-hydroxylated steroids. Prep. Biochem. Biotechnol. 3, 309–321. doi: 10.1080/10826060701593209
Bergstrand, L. H., Cardenas, E., Holert, J., Van Hamme, J. D., and Mohn, W. W. (2016). Delineation of steroid-degrading microorganisms through comparative genomic analysis. MBio 7:e00166. doi: 10.1128/mBio.00166-16
Bhatti, H. N., and Khera, R. A. (2012). Biological transformations of steroidal compounds: a review. Steroids 77, 1267–1290. doi: 10.1016/j.steroids.2012.07.018
Bradley, R. W., Buck, M., and Wang, B. (2016). Tools and principles for microbial gene circuit engineering. J. Mol. Biol. 428, 862–888. doi: 10.1016/j.jmb.2015.10.004
Bragin, E. Y., Shtratnikova, V. Y., Dovbnya, D. V., Schelkunov, M. I., Pekov, Y. A., Malakho, S. G., et al. (2013). Comparative analysis of genes encoding key steroid core oxidation enzymes in fast-growing Mycobacterium spp. strains. J. Steroid Biochem. Mol. Biol. 138, 41–53. doi: 10.1016/j.jsbmb.2013.02.016
Brzezinska, M., Szulc, I., Brzostek, A., Klink, M., Kielbik, M., Sulowska, Z., et al. (2013). The role of 3-ketosteroid 1(2)-dehydrogenase in the pathogenicity of Mycobacterium tuberculosis. BMC Microbiol. 13:43. doi: 10.1186/1471-2180-13-43
Brzostek, A., Sliwinski, T., Rumijowska-Galewicz, A., Korycka-Machała, M., and Dziadek, J. (2005). Identification and targeted disruption of the gene encoding the main 3-ketosteroid dehydrogenase in Mycobacterium smegmatis. Microbiology 151, 2393–2402. doi: 10.1099/mic.0.27953-0
Bureik, M., and Bernhardt, R. (2007). “Steroid hydroxylation: microbial steroid biotransformations using cytochrome P450 enzymes,” in Modern Biooxidation: Enzymes, Reactions and Applications, eds R. D. Schmid and V. B. y Urlacher (Weinheim: Wiley-VCH Verlag GmbH and Co. KGaA), 155–176.
Capyk, J. K., D'Angelo, I., Strynadka, N. C., and Eltis, L. D. (2009). Characterization of 3-ketosteroid 9α-hydroxylase, a rieske oxygenase in the cholesterol degradation pathway of Mycobacterium tuberculosis. J. Biol. Chem. 284, 9937–9946. doi: 10.1074/jbc.M900719200
Carballeira, J. D., Quezada, M. A., Hoyos, P., Simeó, Y., Hernaiz, M. J., Alcantara, A. R., et al. (2009). Microbial cells as catalysts for stereo selective red-ox reactions. Biotechnol. Adv. 27, 686–714. doi: 10.1016/j.biotechadv.2009.05.001
Choudhary, E., Thakur, P., Pareek, M., and Agarwal, N. (2015). Gene silencing by CRISPR interference in mycobacteria. Nat. Commun. 6:6267. doi: 10.1038/ncomms7267
Crowe, A. M., Casabon, I., Brown, K. L., Liu, J., Lian, J., Rogalski, J. C., et al. (2017). Catabolism of the last two steroid rings in Mycobacterium tuberculosis and other bacteria. MBio 8:e00321–17. doi: 10.1128/mBio.00321-17
de Carvalho, C. C., Cruz, A., Angelova, B., Fernandez, P., Pons, M. N., Pinheiro, H. M., et al. (2004). Behaviour of Mycobacterium sp. NRRL B-3805 whole cells in aqueous, organic-aqueous and organic media studied by fluorescence microscopy. Appl. Microbiol. Biotechnol. 64, 695–701. doi: 10.1007/s00253-003-1508-z
Demain, A. L. (1992). Microbial secondary metabolism: a new theoretical frontier for academia, a new opportunity for industry. Ciba Found. Symp. 171, 3–16.
Donova, M. V., Dovbnya, D. V., Sukhodolskaya, G. V., Khomutov, S. M., Nikolayeva, V. M., Kwon, I., et al. (2005a). Microbial conversion of sterol-containing soybean oil production waste. J. Chem. Technol. Biotechnol. 80, 55–60. doi: 10.1002/jctb.1156
Donova, M. V., and Egorova, O. V. (2012). Microbial steroid transformations: current state and prospects. Appl. Microbiol. Biotechnol. 94, 1423–1447. doi: 10.1007/s00253-012-4078-0
Donova, M. V., Gulevskaya, S. A., Dovbnya, D. V., and Puntus, I. F. (2005b). Mycobacterium sp. mutant strain producing 9alpha-hydroxyandrostenedione from sitosterol. Appl. Microbiol. Biotechnol. 67, 671–678. doi: 10.1007/s00253-004-1808-y
Donova, M. V. (2017). Steroid bioconversions. Methods Mol. Biol. 1645, 1–13. doi: 10.1007/978-1-4939-7183-1_1
Donova, M. V., Nikolayeva, V. M., Dovbnya, D. V., Gulevskaya, S. A., and Suzina, N. E. (2007). Methyl-beta-cyclodextrin alters growth, activity and cell envelope features of sterol-transforming mycobacteria. Microbiology 153, 1981–1992. doi: 10.1099/mic.0.2006/001636-0
Dragosits, M., and Mattanovich, D. (2013). Adaptative laboratory evolution- principles and applications for biotechnology. Microb. Cell Fact. 1:64. doi: 10.1186/1475-2859-12-64
Duport, C., Spagnoli, R., Degryse, E., and Pompon, D. (1998). Self-sufficient biosynthesis of pregnenolone and progesterone in engineered yeast. Nat. Biotechnol. 16, 186–189. doi: 10.1038/nbt0298-186
Egorova, O. V., Gulevskaya, S. A., Puntus, I. F., Filonov, A. E., and Donova, M. V. (2002). Production of androstenedione using mutants of Mycobacterium sp. J. Chem. Tech. Biotech. 77, 141–147. doi: 10.1002/jctb.536
Egorova, O. V., Nikolayeva, V. M., Sukhodolskaya, G. V., and Donova, M. V. (2009). Transformation of C19-steroids and testosterone production by sterol-transforming strains of Mycobacterium sp. J. Mol. Catal. B Enzym. 57, 198–203. doi: 10.1016/j.molcatb.2008.09.003
El-Kadi, I. A., and Mostafa, M. E. (2004). Hydroxylation of progesterone by some Trichoderma species. Folia Microbiol. 49, 285–290. doi: 10.1007/BF02931044
Fernandes, P., Cruz, A., Angelova, B., Pinheiro, H. M., and Cabral, J. M. S. (2003). Microbial conversion of steroid com-pounds: recent developments. Enzyme Microb. Technol. 32, 688–705. doi: 10.1016/S0141-0229(03)00029-2
Fernández-Cabezón, L., Galán, B., and García, J. L. (2017a). Engineering Mycobacterium smegmatis for testosterone production. Microb. Biotechnol. 10, 151–161. doi: 10.1111/1751-7915.12433
Fernández-Cabezón, L., Galán, B., and García, J. L. (2017b). Molecular characterization of a new gene cluster for steroid degradation in Mycobacterium smegmatis. Environ. Microbiol. 19, 2546–2563. doi: 10.1111/1462-2920.13704
Fernández de las Heras, L., van der Geize, R., Drzyzga, O., Perera, J., and Navarro-Llorens, M. J. (2012). Molecular characterization of three 3-ketosteroid-Δ(1)-dehydrogenase isoenzymes of Rhodococcus ruber strain Chol-4. J. Steroid Biochem. Mol. Biol. 132, 271–281. doi: 10.1016/j.jsbmb.2012.06.005
Fogal, S., Bergantino, E., Motterle, R., Castellin, A., and Arvotti, A. (2013). Process for the Preparation of Testosterone. U.S. Patent No 8592178B2.
Galán, B., García-Fernández, J., Felpeto-Santero, C., Fernández-Cabezón, L., and García, J. L. (2017a). “Bacterial metabolism of Steroids,” in Handbook of Hydrocarbon and Lipid Microbiology Series. Aerobic Utilization of Hydrocarbons, Oils and Lipids, ed F. Rojo (Cham: Springer), 1–22.
Galán, B., Uhía, I., García-Fernández, E., Martínez, I., Bahíllo, E., de la Fuente, J. L., et al. (2017b). Mycobacterium smegmatis is a suitable cell factory for the production of steroidic synthons. Microb. Biotechnol. 10, 138–150. doi: 10.1111/1751-7915.12429
García-Fernández, J., Galán, B., Felpeto-Santero, C., Barredo, J. L., and García, J. L. (2017). Production of 4-ene-3-ketosteroids in Corynebacterium glutamicum. Catalysts 7:316. doi: 10.3390/catal7110316
García, J. L., Uhía, I., and Galán, B. (2012). Catabolism and biotechnological applications of cholesterol degrading bacteria. Microb. Biotechnol. 5, 679–699. doi: 10.1111/j.1751-7915.2012.00331.x
Goren, T., Harnik, M., Rimon, S., and Aharonowitz, Y. (1983). 1-Ene-steroid reductase of Mycobacterium sp. NRRL B-3805. J. Steroid Biochem. 19, 1789–1797.
Guevara, G., Fernández de las Heras, L., Perera, J., and Navarro Llorens, J. M. (2017a). Functional differentiation of 3-ketosteroid Δ1-dehydrogenase isozymes in Rhodococcus ruber strain Chol-4. Microb. Cell Fact. 16:42. doi: 10.1186/s12934-017-0657-1
Guevara, G., Heras, L. F. L., Perera, J., and Llorens, J. M. N. (2017b). Functional differentiation of 3-ketosteroid 9α-hydroxylases in Rhodococcus ruber strain Chol-4. J. Steroid Biochem. Mol. Biol. 7, 176–187. doi: 10.1016/j.jsbmb.2017.06.011
Hakki, T., Zearo, S., Drăagan, C. A., Bureik, M., and Bernhardt, R. (2008). Coexpression of redox partners increases the hydrocortisone (cortisol) production efficiency in CYP11B1expressing fission yeast Schizosaccharomyces pombe. J. Biotechnol. 133, 351–359. doi: 10.1016/j.jbiotec.2007.06.022
Hannemann, F., Virus, C., and Bernhardt, R. (2006). Design of an Escherichia coli system for whole cell mediated steroid synthesis and molecular evolution of steroid hydroxylases. J. Biotechnol. 124, 172–181. doi: 10.1016/j.jbiotec.2006.01.009
Hanson, J. R. (2005). Steroids: reactions and partial synthesis. Nat. Prod. Rep. 22, 104–110. doi: 10.1039/b415086b
Haußmann, U., Wolters, D. A., Fränzel, B., Eltis, L. D., and Poetsch, A. (2013). Physiological adaptation of the Rhodococcus jostii RHA1 membrane proteome to steroids as growth substrates. J. Proteome Res. 12, 1188–1198. doi: 10.1021/pr300816n
Herráiz, I. (2017). Chemical pathways of corticosteroids, industrial synthesis from sapogenins. Methods Mol. Biol. 1645, 15–27. doi: 10.1007/978-1-4939-7183-1_2
Hoebenreich, S., Spinck, M., and Nett, N. (2017). Laboratory-scale hydroxylation of steroids by P450BM3 variants. Methods Mol. Biol. 1645, 239–257. doi: 10.1007/978-1-4939-7183-1_17
Imada, Y., and Takahashi, K. (1980). Process for Producing Steroidal Alcohols. U.S. Patent No/ 4223091.
Jakočiunas, T., Jensen, M. K., and Keasling, J. D. (2016). CRISPR/Cas9 advances engineering of microbial cell factories. Metab. Eng. 34, 44–59. doi: 10.1016/j.ymben.2015.12.003
Kanno, A. I., Goulart, C., Rofatto, H. K., Oliveira, S. C., Leite, L. C. C., and McFadden, J. (2016). New recombinant Mycobacterium bovis BCG expression vectors: improving genetic control over mycobacterial promoters. Appl. Environ. Microbiol. 82, 2240–2246. doi: 10.1128/AEM.03677-15
Kendall, S. L., Burgess, P., Balhana, R., Withers, M., Ten Bokum, A., Lott, J. S., et al. (2010). Cholesterol utilization in mycobacteria is controlled by two TetR-type transcriptional regulators: kstR and kstR2. Microbiology 156, 1362–1371. doi: 10.1099/mic.0.034538-0
Kendall, S. L., Withers, M., Soffair, C. N., Moreland, N. J., Gurcha, S., Sidders, B., et al. (2007). A highly conserved transcriptional repressor controls a large regulon involved in lipid degradation in Mycobacterium smegmatis and Mycobacterium tuberculosis. Mol. Microbiol. 65, 684–699. doi: 10.1111/j.1365-2958.2007.05827.x
Kiss, F. M., Lundemo, M. T., Zapp, J., Woodley, J. M., and Bernhardt, R. (2015). Process development for the production of 15β-hydroxycyproterone acetate using Bacillus megaterium expressing CYP106A2 as whole-cell biocatalyst. Microb. Cell Fact. 14:28. doi: 10.1186/s12934-015-0210-z
Knight, J. C., and Wovcha, M. G. (1981). Process for the Microbial Transformation of Steroids. U.S. Patent No/4304860A.
Knol, J., Bodewits, K., Hessels, G. I., Dijkhuizen, L., and van der Geize, R. (2008). 3-Keto-5alpha-steroid delta(1)-dehydrogenase from Rhodococcus erythropolis SQ1 and its orthologue in Mycobacterium tuberculosis H37Rv are highly specific enzymes that function in cholesterol catabolism. Biochem. J. 410, 339–346. doi: 10.1042/BJ20071130
Kolar, N. W., Swart, A. C., Mason, J. I., and Swart, P. (2007). Functional expression and characterization of human cytochrome P45017alpha in Pichia pastoris. J. Biotechnol. 129, 635–644. doi: 10.1016/j.jbiotec.2007.02.003
Kołek, T., Szpineter, A., and Swizdor, A. (2008). Baeyer-Villiger oxidation of DHEA, pregnenolone, and androstenedione by Penicillium lilacimum AM111. Steroids 73, 1441–1445. doi: 10.1016/j.steroids.2008.07.008
Lamb, D. C., Jackson, C. J., Warrilow, A. G., Manning, N. J., Kelly, D. E., and Kelly, S. L. (2007). Lanosterol biosynthesis in the prokaryote Methylococcus capsulatus: insight into the evolution of sterol biosynthesis. Mol. Biol. Evol. 24, 1714–1721. doi: 10.1093/molbev/msm090
Lam, K. S. (2010). “Application of whole-cell biotransformation in the pharmaceutical industry,” in Biocatalysis for the Pharmaceutical Industry: Discovery, Development, and Manufacturing, eds J. Tao, G. Q. Lin, and A. y Liese (New York, NY: Wiley), 213–227.
Laveaga, G. S. (2005). Uncommon trajectories: steroid hormones, Mexican peasants, and the search for a wild yam. Stud. Hist. Phil. Biol. Biomed. Sci. 36, 743–760. doi: 10.1016/j.shpsc.2005.09.007
Lee, J. Y., Na, Y. A., Kim, E., Lee, H. S., and Kim, P. (2016). The actinobacterium Corynebacterium glutamicum, an industrial workhorse. J. Microbiol. Biotechnol. 26, 807–822. doi: 10.4014/jmb.1601.01053
Leisch, H., Morley, K., and Lau, P. C. (2011). Baeyer-Villiger monooxygenases: more than just green chemistry. Chem. Rev. 111, 4165–4222. doi: 10.1021/cr1003437
Li, H., Liu, H. M., Ge, W., Huang, L., and Shan, L. (2005). Synthesis of 7alpha-hydroxy- dehydroepiandrosterone and 7beta-hydroxy-dehydroepiandrosterone. Steroids 70, 970–973. doi: 10.1016/j.steroids.2005.07.006
Li, H., Sun, J., and Xu, Z. (2017). Biotransformation of DHEA into 7α,15α-diOH-DHEA. Methods Mol. Biol. 1645, 289–295. doi: 10.1007/978-1-4939-7183-1_20
Liu, M., Zhu, Z. T., Tao, X. Y., Wang, F. Q., and Wei, D. Z. (2016a). Single nucleotide polymorphism analysis for the production of valuable steroid intermediates in Mycobacterium neoaurum. Biotechnol. Lett. 38, 1881–1892. doi: 10.1007/s10529-016-2187-z
Liu, M., Zhu, Z. T., Tao, X. Y., Wang, F. Q., and Wei, D. Z. (2016b). RNA-Seq analysis uncovers non-coding small RNA system of Mycobacterium neoaurum in the metabolism of sterols to accumulate steroid intermediates. Microb. Cell Fact. 15:64. doi: 10.1186/s12934-016-0462-2
Liu, W. H., Kuo, C. W., Wu, K. L., Lee, C. Y., and Hsu, W. Y. (1994). Transformation of cholesterol to testosterone by Mycobacterium sp. J. Ind. Microbiol. 13, 167–171. doi: 10.1007/BF01584002
Liu, X., and Meng, H. (1981). Mycobacterium Fortuitum and Application Thereof in Fermentation Production of Delta-Lactone. C.N. Patent No/103756940B.
Liu, Y., Shen, Y., Qiao, Y., Su, L., Li, C., and Wang, M. (2016). The effect of 3-ketosteroid-Δ(1)-dehydrogenase isoenzymes on the transformation of AD to 9α-OH-AD by Rhodococcus rhodochrous DSM 43269. J. Ind. Microbiol. Biotechnol. 43, 1303–1311. doi: 10.1007/s10295-016-1804-0
Li, W., Ge, F., Zhang, Q., Ren, Y., Yuan, J., He, J., et al. (2014). Identification of gene expression profiles in the actinomycete Gordonia neofelifaecis grown with different steroids. Genome 57, 345–353. doi: 10.1139/gen-2014-0030
Li, Y., Lu, F., Sun, T., and Du, L. (2007). Expression of ksdD gene encoding 3-ketosteroid-Delta1- dehydrogenase from Arthrobacter simplex in Bacillus subtilis. Lett. Appl. Microbiol. 44, 563–568. doi: 10.1111/j.1472-765X.2007.02134.x
Lo, C. K., Pan, C. P., and Liu, W. H. (2002). Production of testosterone from phytosterol using a single-step microbial transformation by a mutant of Mycobacterium sp. J. Ind. Microbiol. Biotechnol. 28, 280–283. doi: 10.1038/sj.jim.7000243
Malaviya, A., and Gomes, J. (2008). Androstenedione production by biotransformation of phytosterols. Bioresour. Technol. 99, 6725–6737. doi: 10.1016/j.biortech.2008.01.039
Manosroi, J., Saowakhon, S., and Manosroi, A. (2008). Enhancement of 17alpha-hydroxyprogesterone production from progesterone by biotransformation using hydroxypropyl-beta-cyclodextrin complexation technique. J. Steroid Biochem. Mol. Biol. 112, 201–204. doi: 10.1016/j.jsbmb.2008.10.003
Marques, M. P. C., Carvalho, F., de Carvalho, C. C. C. R., Cabral, J. M. S., and Fernandes, P. (2010). Steroid bioconversion: toward green processes. Food Bioprod. Proc. 88, 12–20. doi: 10.1016/j.fbp.2010.01.009
Marsheck, W. J., Kraychy, S., and Muir, R. D. (1972). Microbial degradation of sterols. Appl. Microbiol. 23, 72–77.
Mohn, W. W., Wilbrink, M. H., Casabon, I., Stewart, G. R., Liu, J., van der Geize, R., et al. (2012). Gene cluster encoding cholate catabolism in Rhodococcus spp. J. Bacteriol. 194, 6712–6719. doi: 10.1128/JB.01169-12
Mondaca, M. A., Vidal, M., Chamorro, S., and Vidal, G. (2017). Selection of biodegrading phytosterol strains. Methods Mol. Biol. 1645, 143–150. doi: 10.1007/978-1-4939-7183-1_9
Murphy, K. C., Papavinasasundaram, K., and Sassetti, C. M. (2015). Mycobacterial recombineering. Methods Mol. Biol. 1285, 177–199. doi: 10.1007/978-1-4939-2450-9_10
Nelson, D. L., and Cox, M. M. (2012). Lehninger Principles of Biochemistry. 6th Edn. New York, NY: WH Freeman.
Nielsen, J., and Keasling, J. D. (2016). Engineering cellular metabolism. Cell 164, 1185–1197. doi: 10.1016/j.cell.2016.02.004
Pearson, A., Budin, M., and Brocks, J. J. (2003). Phylogenetic and biochemical evidence for sterol synthesis in the bacterium Gemmata obscuriglobus. Proc. Natl. Acad. Sci. U.S.A. 100, 15352–15357. doi: 10.1073/pnas.2536559100
Peart, P. C., McCook, K. P., Russell, F. A., Reynolds, W. F., and Reese, P. B. (2011). Hydroxylation of steroids by Fusarium oxysporum, Exophiala jeanselmei and Ceratocystis paradoxa. Steroids 76, 1317–1330. doi: 10.1016/j.steroids.2011.06.010
Penfield, J. S., Worrall, L. J., Strynadka, N. C., and Eltis, L. D. (2014). Substrate specificities and conformational flexibility of 3-ketosteroid 9α-hydroxylases. J. Biol. Chem. 289, 25523–25536. doi: 10.1074/jbc.M114.575886
Petrič, Š., Hakki, T., Bernhardt, R., Žigon, D., and Črešnar, B. (2010). Discovery of a steroid 11α- hydroxylase from Rhizopus oryzae and its biotechnological application. J. Biotechnol. 150, 428–437. doi: 10.1016/j.jbiotec.2010.09.928
Petrusma, M., Dijkhuizen, L., and van der Geize, R. (2009). Rhodococcus rhodochrous DSM 43269 3- ketosteroid 9alpha-hydroxylase, a two-component iron-sulfur-containing monooxygenase with subtle steroid substrate specificity. Appl. Environ. Microbiol. 75, 5300–5307. doi: 10.1128/AEM.00066-09
Petrusma, M., Dijkhuizen, L., and van der Geize, R. (2012). Structural features in the KshA terminal oxygenase protein that determine substrate preference of 3-ketosteroid 9α-hydroxylase enzymes. J. Bacteriol. 194, 115–121. doi: 10.1128/JB.05838-11
Petrusma, M., Hessels, G., Dijkhuizen, L., and van der Geize, R. (2011). Multiplicity of 3-ketosteroid-9α-hydroxylase enzymes in Rhodococcus rhodochrous DSM 43269 for specific degradation of different classes of steroids. J. Bacteriol. 193, 3931–3940. doi: 10.1128/JB.00274-11
Pines, G., Freed, E. F., Winkler, J. D., and Gill, R. T. (2015). Bacterial recombineering: genome engineering via phage-based homologous recombination. ACS Synth. Biol. 4, 1176–1185. doi: 10.1021/acssynbio.5b00009
Prakash, S., and Bajaj, A. (2017). Process for biotransformation of androsta-4-ene-3,17-dione (4-AD) to androsta-1,4-diene-3,17-dione (ADD). Methods Mol. Biol. 1645, 227–238. doi: 10.1007/978-1-4939-7183-1_16
Reese, P. (2007). “Biotransformation of terpenes and steroids by fungi,” in Natural Products: Essential Resources for Human Survival, eds Y. Z. Zhu, B. K. H. Tan, B. H. Bay, and C. H. Liu (Singapore: Word Scientific Publishing Co Pte Ltd), 71–76. doi: 10.1142/9789812707444_0006
Ribeiro, A. L., Sánchez, M., Hidalgo, A., and Berenguer, J. (2017). Stabilization of enzymes by using thermophiles. Methods Mol. Biol. 1645, 297–312. doi: 10.1007/978-1-4939-7183-1_21
Ríos, L. O. L., Luengo, J. M., and Fernández-Cañón, J. M. (2017). Steroid 11-alpha-hydroxylation by the fungi Aspergillus nidulans and Aspergillus ochraceus. Methods Mol. Biol. 1645, 271–287. doi: 10.1007/978-1-4939-7183-1_19
Rock, J. M., Hopkins, F. F., Chavez, A., Diallo, M., Chase, M. R., Gerrick, E. R., et al. (2017). Programmable transcriptional repression in mycobacteria using an orthogonal CRISPR interference platform. Nat. Microbiol. 2:16274. doi: 10.1038/nmicrobiol.2016.274
Rodríguez-García, A., Fernández-Alegre, E., Morales, A., Sola-Landa, A., Lorraine, J., Macdonald, S., et al. (2016). Complete genome sequence of ‘Mycobacterium neoaurum’ NRRL B-3805, an androstenedione (AD) producer for industrial biotransformation of sterols. J. Biotechnol. 224, 64–65. doi: 10.1016/j.jbiotec.2016.03.021
Roglič, U., and Plazl, I, Žnidarši, C. -P. (2007). Batch and continuous transformation of progesterone by Rhizopus nigricans pellets in the presence of β-cyclodextrin. Biocat. Biotrans. 25, 16–23. doi: 10.1080/10242420601060954
Ruijssenaars, H. J., Sperling, E. M., Wiegerinck, P. H., Brands, F. T., Wery, J., and de Bont, J. A. (2007). Testosterone 15beta-hydroxylation by solvent tolerant Pseudomonas putida S12. J. Biotechnol. 131, 205–208. doi: 10.1016/j.jbiotec.2007.06.007
Sedlaczek, L., and Leland, L. S. (1988). Biotransformations of steroids. Crit. Rev. Biotechnol. 7, 187–236. doi: 10.3109/07388558809146602
Shao, M., Sha, Z., Zhang, X., Rao, Z., Xu, M., Yang, T., et al. (2017). Efficient androst-1,4-diene-3,17-dione production by co-expressing 3-ketosteroid-Δ1-dehydrogenase and catalase in Bacillus subtilis. J. Appl. Microbiol. 122, 119–128. doi: 10.1111/jam.13336
Shtratnikova, V. Y., Bragin, E. Y., Dovbnya, D. V., Pekov, Y. A., Schelkunov, M. I., Strizhov, N., et al. (2014). Complete genome sequence of sterol- transforming Mycobacterium neoaurum strain VKM Ac-1815D. Genome Announc. 2:e01177–13. doi: 10.1128/genomeA.01177-13
Shtratnikova, V. Y., Schelkunov, M. I., Dovbnya, D. V., Pekov, Y. A., Bragin, E. Y., Ashaphin, V. V., et al. (2015). Complete genome sequence of Mycobacterium sp. strain VKM Ac-1817D, capable of producing 9α-hydroxy-androst-4-ene-3,17-dione from phytosterol. Genome Announc. 3:e01447–14. doi: 10.1128/genomeA.01447-14
Silber, J., Kramer, A., Labes, A., and Tasdemir, D. (2016). From discovery to production: biotechnology of marine fungi for the production of new antibiotics. Mar. Drugs 14:E137. doi: 10.3390/md14070137
Smith, M., Zahnley, J., Pfeifer, D., and Goff, D. (1993). Growth and cholesterol oxidation by Mycobacterium species in Tween 80 medium. Appl. Environ. Microbiol. 59, 1425–1429.
Szczebara, F. M., Chandelier, C., Villeret, C., Masurel, A., Bourot, S., Duport, C., et al. (2003). Total biosynthesis of hydrocortisone from a simple carbon source in yeast. Nat. Biotechnol. 21, 143–149. doi: 10.1038/nbt775
Tong, W. Y., and Dong, X. (2009). Microbial biotransformation: recent developments on steroid drugs. Recent Pat. Biotechnol. 3, 141–153. doi: 10.2174/187220809788700157
Uhía, I., Galán, B., Kendall, S. L., Stoker, N. G., and García, J. L. (2012). Cholesterol metabolism in Mycobacterium smegmatis. Environ. Microbiol. Rep. 4, 168–182. doi: 10.1111/j.1758-2229.2011.00314.x
van der Geize, R., Hessels, G. I., and Dijkhuizen, L. (2002a). Molecular and functional characterization of the kstD2 gene of Rhodococcus erythropolis SQ1 encoding a second 3-ketosteroid Δ1- dehydrogenase isoenzyme. Microbiology 148, 3285–3292. doi: 10.1099/00221287-148-10-3285
van der Geize, R., Hessels, G. I., Gerwen, R. V., Meijden, P. V. D., and Dijkhuizen, L. (2002b). Molecular and functional characterization of kshA and kshB, encoding two components of 3-ketosteroid 9α- hydroxylase, a class IA monooxygenase, in Rhodococcus erythropolis strain SQ1. Mol. Microbiol. 45, 1007–1018. doi: 10.1046/j.1365-2958.2002.03069.x
van der Geize, R., Hessels, G. I., Nienhuis-Kuiper, M., and Dijkhuizen, L. (2008). Characterization of a second Rhodococcus erythropolis SQ1 3-ketosteroid 9alpha-hydroxylase activity comprising a terminal oxygenase homologue, KshA2, active with oxygenase-reductase component KshB. Appl. Environ. Microbiol. 74, 7197–7203. doi: 10.1128/AEM.00888-08
van der Geize, R., Hessels, G. I., van Gerwen, R., van der Meijden, P., and Dijkhuizen, L. (2001). Unmarked gene deletion mutagenesis of kstD, encoding 3-ketosteroid delta1-dehydrogenase, in Rhodococcus erythropolis SQ1 using sacB as counter-selectable marker. FEMS Microbiol. Lett. 205, 197–202. doi: 10.1016/S0378-1097(01)00464-5
van der Geize, R., Hessels, G. I., van Gerwen, R., Vrijbloed, J. W., van Der Meijden, P., and Dijkhuizen, L. (2000). Targeted disruption of the kstD gene encoding a 3-kestosteroid Δ(1)-dehydrogenase isoenzyme of Rhodococcus erythropolis strain SQ1. Appl. Environ. Microbiol. 66, 2029–2036. doi: 10.1128/AEM.66.5.2029-2036.2000
van der Geize, R., Yam, K., Heuser, T., Wilbrink, M. H., Hara, H., Anderton, M. C., et al. (2007). A gene cluster encoding cholesterol catabolism in a soil actinomycete provides insight into Mycobacterium tuberculosis survival in macrophages. Proc. Natl. Acad. Sci. U.S.A. 104, 1947–1952. doi: 10.1073/pnas.0605728104
Vico, P., Cauet, G., Rose, K., Lathe, R., and Degryse, E. (2002). Dehydroepiandrosterone (DHEA) metabolism in Saccharomyces cerevisiae expressing mammalian steroid hydroxylase CYP7B: Ayr1p and Fox2p display 17beta-hydroxysteroid dehydrogenase activity. Yeast 19, 873–886. doi: 10.1002/yea.882
Wang, F. Q., Li, B., Wang, W., Zhang, C. G., and Wei, D. Z. (2007). Biotransformation of diosgenin to nuatigenin-type steroid by a newly isolated strain, Streptomyces virginiae IBL-14. Appl. Microbiol. Biotechnol. 77, 771–777. doi: 10.1007/s00253-007-1216-1
Wang, F. Q., Yao, K., and Wei, D. Z. (2011). “From soybean phytosterols to steroid hormones,” in Soybean and Health, ed H. El-Shemy (Rijeka InTech—Open Access Publisher), 232–252.
Wang, F. Q., Zhang, C. G., Li, B., Wei, D. Z., and Tong, W. Y. (2009). New microbiological transformations of steroids by Streptomyces virginiae IBL-14. Environ. Sci. Technol. 43, 5967–5974. doi: 10.1021/es900585w
Wang, K. C., You, B. J., Jan, J. L., and Lee, S. S. (1995). Microbial transformation of lanosterol derivates with Mycobacterium sp. (NRRL B-3805). J. Nat. Prod. 58, 1222–1227. doi: 10.1021/np50122a010
Wang, W., Wang, F. Q., and Wei, D. Z. (2009). Characterization of P450 FcpC, the enzyme responsible for bioconversion of diosgenone to isonuatigenone in Streptomyces virginiae IBL-14. Appl. Environ. Microbiol. 75, 4202–4425. doi: 10.1128/AEM.02606-08
Wang, X., Feng, J., Zhang, D., Wu, Q., Zhu, D., and Ma, Y. (2017). Characterization of new recombinant 3-ketosteroid-Δ1-dehydrogenases for the biotransformation of steroids. Appl. Microbiol. Biotechnol. 101, 6049–6060. doi: 10.1007/s00253-017-8378-2
Wei, J. H., Yin, X., and Welander, P. V. (2016). Sterol synthesis in diverse bacteria. Front. Microbiol. 7:990. doi: 10.3389/fmicb.2016.00990
Wei, W., Fan, S., Wang, F., and Wei, D. (2010a). A new steroid-transforming strain of Mycobacterium neoaurum and cloning of 3-ketosteroid 9alpha-hydroxylase in NwIB-01. Appl. Biochem. Biotechnol. 162, 1446–1456. doi: 10.1007/s12010-010-8919-y
Wei, W., Fan, S. Y., Wang, F. Q., and Wei, D. Z. (2014). Accumulation of androstadiene-dione by overexpression of heterologous 3-ketosteroid Δ1-dehydrogenase in Mycobacterium neoaurum NwIB-01. World J. Microbiol. Biotechnol. 30, 1947–1954. doi: 10.1007/s11274-014-1614-3
Wei, W., Wang, F. Q., Fan, S. Y., and Wei, D. Z. (2010b). Inactivation and augmentation of the primary 3-ketosteroid-{delta}1-dehydrogenase in Mycobacterium neoaurum NwIB-01: biotransformation of soybean phytosterols to 4-androstene-3,17-dione or 1,4-androstadiene-3,17-dione. Appl. Environ. Microbiol. 76, 4578–4582. doi: 10.1128/AEM.00448-10
Wilbrink, M. H., Petrusma, M., Dijkhuizen, L., and van der Geize, R. (2011). FadD19 of Rhodococcus rhodochrous DSM 43269, a steroid-coenzymeA ligase essential for degradation of C-24 branched sterol side chains. Appl. Environ. Microbiol. 77, 4455–4464. doi: 10.1128/AEM.00380-11
Xiong, L. B., Liu, H. H., Xu, L. Q., Sun, W. J., Wang, F. Q., and Wei, D. Z. (2017a). Improving the production of 22-hydroxy-23,24-bisnorchol-4-ene-3-one from sterols in Mycobacterium neoaurum by increasing cell permeability and modifying multiple genes. Microb. Cell Fact. 16:89. doi: 10.1186/s12934-017-0705-x
Xiong, L. B., Liu, H. H., Xu, L. Q., Wei, D. Z., and Wang, F. Q. (2017b). Role identification and application of SigD in the transformation of soybean phytosterol to 9α-hydroxy-4-androstene-3,17-dione in Mycobacterium neoaurum. J. Agric. Food Chem. 65, 626–631. doi: 10.1021/acs.jafc.6b05314
Xu, L. Q., Liu, Y. J., Yao, K., Liu, H. H., Tao, X. Y., Wang, F. Q., et al. (2016). Unraveling and engineering the production of 23,24-bisnorcholenic steroids in sterol metabolism. Sci. Rep. 6:21928. doi: 10.1038/srep21928
Yan, M. Y., Yan, H. Q., Ren, G. X., Zhao, J. P., Guo, X. P., and Sun, Y. C. (2017). CRISPR-Cas12a-assisted recombineering in bacteria. Appl. Environ. Microbiol. 83:e00947–17. doi: 10.1128/AEM.00947-17
Yao, K., Xu, L. Q., Wang, F. Q., and Wei, D. Z. (2014). Characterization and engineering of 3-ketosteroid- Δ1-dehydrogenase and 3-ketosteroid-9α-hydroxylase in Mycobacterium neoaurum ATCC 25795 to produce 9α-hydroxy-4-androstene-3,17-dione through the catabolism of sterols. Metab. Eng. 24, 181–191. doi: 10.1016/j.ymben.2014.05.005
Yeh, C. H., Kuo, Y. S., Chang, C. M., Liu, W. H., Sheu, M. L., and Meng, M. (2014). Deletion of the gene encoding the reductase component of 3-ketosteroid 9α-hydroxylase in Rhodococcus equi USA-18 disrupts sterol catabolism, leading to the accumulation of 3-oxo-23,24-bisnorchola-1,4-dien-22-oic acid and 1,4- androstadiene-3,17-dione. Microb. Cell Fact. 13:130. doi: 10.1186/s12934-014-0130-3
Yildirim, K., Kupcu, I., and Gulsan, F. (2010). Biotransformation of some steroids by Aspergillus wentii. Z Naturforsch C. 65, 688–692. doi: 10.1515/znc-2010-11-1209
Yuan, J., Chen, G., Cheng, S., Ge, F., Qiong, W., Li, W., et al. (2015). Accumulation of 9α-hydroxy-4-androstene-3,17-dione by co-expressing kshA and kshB encoding component of 3-ketosteroid-9α- hydroxylase in Mycobacterium sp. NRRL B-3805. Sheng Wu Gong Cheng Xue Bao 31, 523–533. doi: 10.13345/j.cjb.140411
Zhang, H., Tian, Y., Wang, J., Li, Y., Wang, H., Mao, S., et al. (2013). Construction of engineered Arthrobacter simplex with improved performance for cortisone acetate biotransformation. Appl. Microbiol. Biotechnol. 97, 9503–9514. doi: 10.1007/s00253-013-5172-7
Zhang, W., Shao, M., Rao, Z., Xu, M., Zhang, X., Yang, T., et al. (2013). Bioconversion of 4-androstene-3,17-dione to androst-1,4-diene-3,17-dione by recombinant Bacillus subtilis expressing ksdd gene encoding 3-ketosteroid-Δ1-dehydrogenase from Mycobacterium neoaurum JC-12. J. Steroid Biochem. Mol. Biol. 135, 36–42. doi: 10.1016/j.jsbmb.2012.12.016
Zhang, X., Rao, Z., Zhang, L., Xu, M., and Yang, T. (2016). Efficient 9α-hydroxy-4-androstene-3,17-dione production by engineered Bacillus subtilis co-expressing Mycobacterium neoaurum 3-ketosteroid 9α- hydroxylase and B. subtilis glucose 1-dehydrogenase with NADH regeneration. Springerplus 5:1207. doi: 10.1186/s40064-016-2871-4
Keywords: steroid, pharmaceuticals, actinobacteria, microbial cell factory, metabolic engineering, systems biology
Citation: Fernández-Cabezón L, Galán B and García JL (2018) New Insights on Steroid Biotechnology. Front. Microbiol. 9:958. doi: 10.3389/fmicb.2018.00958
Received: 25 December 2017; Accepted: 24 April 2018;
Published: 15 May 2018.
Edited by:
Qiang Wang, Institute of Hydrobiology (CAS), ChinaReviewed by:
Pablo Ivan Nikel, The Novo Nordisk Foundation Center for Biosustainability (DTU Biosustain), DenmarkTaisen Iguchi, National Institute for Basic Biology, Japan
Copyright © 2018 Fernández-Cabezón, Galán and García. This is an open-access article distributed under the terms of the Creative Commons Attribution License (CC BY). The use, distribution or reproduction in other forums is permitted, provided the original author(s) and the copyright owner are credited and that the original publication in this journal is cited, in accordance with accepted academic practice. No use, distribution or reproduction is permitted which does not comply with these terms.
*Correspondence: José L. García, amxnYXJjaWFAY2liLmNzaWMuZXM=