- Institute for Molecular Microbiology and Biotechnology, University of Münster, Münster, Germany
The opportunistic pathogen Pseudomonas aeruginosa employs its complex quorum sensing (QS) network to regulate the expression of virulence factors such as pyocyanin. Besides cell density, QS in this bacterium is co-regulated by environmental cues. In this study, we employed a previously established co-culture model system to identify metabolic influences that are involved in the regulation of pyocyanin production in P. aeruginosa. In this co-culture consisting of P. aeruginosa and the chitinolytic bacterium Aeromonas hydrophila, parasitic growth of P. aeruginosa is strictly dependent on the production of pyocyanin. We could show that in this co-culture, pyocyanin production is likely induced by the stringent response mediated by SpoT in response to nutrient limitation. Pyocyanin production by stringent response mutants in the co-culture could not be complemented by overexpression of PqsE. Via transposon mutagenesis, several amino acid auxotrophic mutants were identified that were also unable to produce pyocyanin when PqsE was overexpressed or when complementing amino acids were present. The inability to produce pyocyanin even though PqsE was overexpressed was likely a general effect of amino acid auxotrophy. These results show the value of the co-culture approach to identify both extra- and intracellular metabolic influences on QS that might be important in infection processes as well.
Introduction
The Gram-negative Gammaproteobacterium Pseudomonas aeruginosa exhibits a broad metabolic versatility (Clarke, 1982; Alonso et al., 1999) and exists in a wide variety of environmental habitats (Ringen and Drake, 1952; Hardalo and Edberg, 1997; Mena and Gerba, 2009). As opportunistic pathogen, P. aeruginosa is able to infect different host organisms (Rahme et al., 1995; Hilbi et al., 2007; Morales et al., 2011), and it is a major agent of human infections posing a particular threat to immunocompromised persons and patients with cystic fibrosis (Driscoll et al., 2007; Harrison, 2007). Within a complex regulatory network that allows P. aeruginosa to sense and respond to different environmental cues with changes in gene expression, quorum sensing (QS) is a key regulatory system of this bacterium rendering P. aeruginosa a model organism for QS (Williams and Camara, 2009; Coggan and Wolfgang, 2012; Jimenez et al., 2012).
Quorum sensing (QS), a process of cell-to-cell communication, describes the regulation of gene expression in response to signal molecules, which are mostly membrane-diffusible N-acyl-homoserine lactones (AHLs) in Gram-negative bacteria. Usually, QS is defined to be cell density-dependent, and an increase in cell density is accompanied by an accumulation of signal molecules to a certain threshold concentration, at which the signals bind to the cognate receptor proteins, thereby eliciting changes in gene expression (Williams, 2007). In P. aeruginosa, however, QS is to a large degree co-regulated by environmental signals, mostly nutritional cues, resulting in the differential regulation of QS-controlled genes under different nutrient conditions (van Delden et al., 2001; Wagner et al., 2003; Bazire et al., 2005; Duan and Surette, 2007; Palmer et al., 2007; Oglesby et al., 2008). In addition to extracellular metabolic information, it is hypothesized that intracellular metabolic influences may be involved in QS regulation as well and that changing ratios of macronutrients may be monitored via intracellular metabolite fluxes (Mellbye and Schuster, 2014).
In this study, we aimed at investigating such extra- and intracellular metabolic influences that are involved in QS regulation in P. aeruginosa. For this, we employed a co-culture model system consisting of P. aeruginosa and the chitinolytic bacterium Aeromonas hydrophila. Chitin, which cannot be utilized by P. aeruginosa, is the sole growth substrate in this co-culture, and P. aeruginosa strictly depends on the production of virulence factors in order to grow by parasitically exploiting the chitinolytic properties of A. hydrophila (Jagmann et al., 2010, 2016). This is mediated by the redox-active virulence factor pyocyanin, which blocks the citric acid cycle in A. hydrophila by inhibiting the enzyme aconitase through the formation of reactive oxygen species, leading to a massive release of acetate by this bacterium. Acetate then serves as growth substrate for P. aeruginosa, while cells of A. hydrophila become inactivated. In a previous study employing the co-culture we identified the guanidinobutyrase GbuA that may take part in an intracellular metabolite flux involved in QS regulation in P. aeruginosa (Jagmann et al., 2016).
Pseudomonas aeruginosa possesses three QS system, which are closely interrelated and coordinate the expression of virulence factors. The Las and Rhl systems are mediated by AHLs as signal molecules and comprise the respective signal synthases (LasI and RhlI) and receptors (LasR and RhlR) (Williams, 2007). The PQS system is mediated by the 2-alkyl-4(1H)-quinolones (AQs) 2-heptyl-4-quinolone (HHQ) and 2-heptyl-3-hydroxy-4-quinolone (Pseudomonas Quinolone Signal: PQS) (Dubern and Diggle, 2008). HHQ is synthesized by the gene products of pqsABCDE and is further converted to PQS by the monooxygenase PqsH (Gallagher et al., 2002; Deziel et al., 2004; Drees and Fetzner, 2015). The pqsABCDE operon is regulated by the transcriptional regulator PqsR, which binds both HHQ and PQS (Xiao et al., 2006). PqsE mediates the cellular response to AQ signaling in a yet unknown way and is, thus, required for the production of virulence factors, such as pyocyanin (Rampioni et al., 2007). In the co-culture with A. hydrophila, the Rhl and PQS systems are required for pyocyanin production by P. aeruginosa (Jagmann et al., 2016), and it has been demonstrated that various mechanisms integrate nutrient limitation into QS activation mainly at the level of these two systems (Mellbye and Schuster, 2014; Welsh and Blackwell, 2016).
Phosphate limitation is integrated via the two component system PhoR-PhoB leading to an induction of both the Rhl and PQS systems (Jensen et al., 2006), which is mediated via a fourth QS signal that was termed IQS [“integrating QS and stress response”; (Lee et al., 2013)]. Transcription of both systems is enhanced under iron limitation as well (Jensen et al., 2006; Schmidberger et al., 2014).
Carbon limitation and the resulting shortage of amino acids is integrated via the stringent response, which is a conserved bacterial stress response enabling bacteria to adapt to growth-inhibiting environmental stresses (Magnusson et al., 2005; Potrykus and Cashel, 2008). It is mediated by the guanosine nucleotides pppGpp and ppGpp as regulatory components. Accumulation of (p)ppGpp is regulated by two enzymes, the monofunctional synthetase RelA and the bifunctional synthetase/hydrolase SpoT. In P. aeruginosa, (p)ppGpp is required for full expression of the Rhl and Las systems and negatively regulates the PQS system (van Delden et al., 2001; Schafhauser et al., 2014).
Nitrogen limitation leads to an induction of rhamnolipid biosynthesis via the small RNA NrsZ together with the two-component system NtrB/C and the alternative sigma factor RpoN without involvement of the Rhl system as it was previously suggested (Medina et al., 2003; Wenner et al., 2014). However, upstream regions of both rhlR and rhlI harbor RpoN-binding sites (Medina et al., 2003; Thompson et al., 2003), and RpoN has been shown to regulate PqsR (Cai et al., 2015), making a connection between nitrogen limitation and Rhl and PQS system induction possible as well.
To investigate metabolic influences that are involved in QS regulation, we used the production of pyocyanin by P. aeruginosa in the co-culture as an indicator for Rhl and PQS system activity. First, we wanted to elucidate which environmental cues induce QS in P. aeruginosa in the co-culture, and, second, we wanted to identify potential intracellular metabolic influences that are involved in the regulation of QS.
Materials and Methods
Bacterial Strains, Plasmids, and Growth Experiments
Bacterial strains and plasmids used in this study are shown in Supplementary Table S1 (Supporting Information). P. aeruginosa, A. hydrophila, and Escherichia coli strains were maintained on solid [1.5% (w/v) agar] lysogeny broth (LB) plates. Antibiotics were used at the following concentrations: ampicillin (100 μg ml-1 for E. coli), carbenicillin (400 μg ml-1 for P. aeruginosa), tetracycline (10 μg ml-1 for E. coli; 160 μg ml-1 for P. aeruginosa), chloramphenicol (30 μg ml-1 for E. coli; 350 μg ml-1 for P. aeruginosa), and gentamicin (20 μg ml-1 for E. coli and 120 μg ml-1 for P. aeruginosa).
Cells were cultivated in a volume of 4 ml in 15 ml glass tubes at 200 r.p.m. in a rotary shaker. For experiments with auxotrophic mutants, co-cultures with these mutants and the wild-type were incubated at 30°C, whereas co-cultures with all other strains were incubated at 37°C. Pre-cultures for co-culture growth experiments were incubated in medium B with ammonium and 0.1% tryptone (Jagmann et al., 2010) for 16 h. Cells were washed in medium B without ammonium and used to inoculate co-cultures at OD600 = 0.001 for both strains in case of cultivation at 30°C and at OD600 = 0.01 for A. hydrophila and OD600 = 0.001 for P. aeruginosa in case of cultivation at 37°C. Co-cultures were incubated in medium B without ammonium and with suspended chitin [0.5% (w/v)] for 5 days at 30°C and for 3 days at 37°C, respectively. At 37°C and with the given inoculation values, the course of the co-culture was accelerated, but the outcome of the co-culture, i.e., pyocyanin production by P. aeruginosa, acetate release by, and subsequent inactivation of A. hydrophila, was independent of temperature. Suspended chitin was prepared as described previously (Jagmann et al., 2010).
In chitin-containing cultures bacterial growth was measured as colony forming units (CFU) grown on Pseudomonas Isolation Agar plates containing 20 μg ml-1 tetracycline and on LB plates as described previously (Jagmann et al., 2010). In case of soluble substrates, bacterial growth was measured as OD600 in a spectrophotometer (Camspec; with test-tube holder).
Construction of Plasmids and Mutants of P. aeruginosa
DNA manipulations and plasmid preparations were performed according to standard methods. Genomic DNA of strain PAO1 was purified with the Puregene Tissue Core Kit B (Qiagen). Oligonucleotides used in this study are shown in Supplementary Table S2 (Supporting Information).
To construct insertional mutants of spoT in strains PAO1 and PAO1ΔrelA, a fragment containing the gene was amplified from genomic DNA of strain PAO1 using the primer pair I/J. The fragment was digested with XbaI/HindIII and ligated into the corresponding sites of pEX18Ap. The resulting plasmid was linearized with PstI cutting within the ORF of spoT and ligated with a res-cat-res cassette obtained from pKO2b through digestion with PstI. The resulting plasmid was mobilized into strains PAO1 and PAO1ΔrelA by triparental mating with E. coli strain DH5α as donor and strain HB101 pRK2013 as helper. Selection of mutants, excision of the vector by a second crossover, and excision of the res-cat-res cassette with pUCP24[parA] was performed as described previously (Jagmann et al., 2016).
To construct strain PAO1 deletion mutants of ambB, relA, lysA, argH, hisD, trpB, and a strain PAO1ΔpchEF deletion mutant of pchABCD two PCR products spanning parts of the up- and downstream regions of the respective genes were amplified from genomic DNA of strain PAO1 with primer pairs E/F and G/H for ambB, A/B and C/D for relA, T/U and V/W for lysA, AA/AB and AC/AD for argH, AE/AF and AG/AH for hisD, AI/AJ and AK/AL for trpB, and P/Q and R/S for pchABCD, and the resulting fragments were used as templates for a second overlapping PCR (SOE-PCR) (Ho et al., 1989) with the respective Up fw and Dn rev primer pairs. The resulting fragments were digested with XbaI/HindIII and ligated into the corresponding sites of the suicide vector pEX18Ap. Mobilization of the resulting plasmids into strains PAO1 and PAO1ΔpchEF, selection of mutants, and excision of the vector by a second crossover was done as described previously (Jagmann et al., 2016).
For complementation of strains PAO1ΔspoT and PAO1ΔrelAΔspoT with spoT, the gene was integrated chromosomally into the attTn7 site using pUC18T-mini-Tn7T-Gm. For this, a fragment containing the putative promoter region and ORF was amplified from genomic DNA of strain PAO1 with the primer pair M/N, digested with HindIII/BamHI, and ligated into the corresponding site of pUC18T-mini-Tn7T-Gm resulting in pUC18T[spoT]. The plasmid was introduced in strains PAO1ΔspoT and PAO1ΔrelAΔspoT, respectively, by four parental mating as described previously (Jagmann et al., 2016), and integration was confirmed by PCR with the primer pair glmSUp/Tn7L (Choi and Schweizer, 2006). For complementation of strain PAO1ΔrelAΔspoT[spoT] with relA, the gene was integrated chromosomally into the attB site using mini-CTX2. For this, a fragment containing the putative promoter region and ORF was amplified from genomic DNA of strain PAO1 with the primer pair K/L, digested with HindIII/BamHI and ligated into the corresponding site of mini-CTX2. The resulting plasmid was mobilized into strain PAO1ΔrelAΔspoT[spoT] by triparental mating as described above, and mutants were selected on PIA plates containing tetracycline. Parts of the vector containing the tetracycline resistance cassette were removed from the genome by introducing pFLP2 as described previously (Jagmann et al., 2016), and integration was confirmed by PCR with the primer pair Pser-up and Pser-down (Hoang et al., 2000).
For complementation of auxotrophic strains of strain PAO1 the ORF of the respective gene was amplified with primer pairs AM/AN for lysA, AO/AP for argH, and AQ/AR for hisD, the resulting fragments were digested with HindIII/XbaI and ligated into the corresponding sites of pBBR1MCS5. The resulting plamids were introduced into the respective auxotrophic strain by transformation (Chuanchuen et al., 2002).
To obtain chromosomal promoter-lacZ reporter gene fusions of rhlR and pqsA, mini-CTX-lacZ[PrhlR] and mini-CTX-lacZ[PpqsA] were mobilized into strain PAO1ΔrelAΔspoT as described previously (Jagmann et al., 2016).
β-Galactosidase Experiments
Expression of β-galactosidase from promoter-lacZ gene fusions of rhlR and pqsA was assessed in chitin-containing co-cultures of strain AH-1NΔlacZ with strains PAO1 and PAO1ΔrelAΔspoT. Co-cultures were incubated as described above, and β-galactosidase activities were determined with ONPG (4 mg ml-1) as substrate from 800 μl sample volumes after 48 h as described previously (Jagmann et al., 2016) and calculated as A405 nm × min-1 × cfu.
Quantification of Pyocyanin
Pyocyanin in culture supernatants was quantified at 314 nm with a high-performance liquid chromatography (HPLC) system (Shimadzu) using a C18-reversed-phase column as described previously (Jagmann et al., 2016). Pyocyanin in supernatants of LB-grown single cultures of auxotrophic mutants and the wild-type was quantified as described previously with slight modifications (Essar et al., 1990). Briefly, 1 ml of culture supernatant was mixed with 1 ml of chloroform, and the chloroform layer was then mixed with 1 ml of 0.2 M HCl. After centrifugation, the absorption of the HCl layer was measured at 520 nm. Pyocyanin standards in LB medium were treated accordingly.
Transposon Mutagenesis
Transposon mutagenesis of strain PAO1 with the plasmid pALMAR3 harboring a mariner transposon, screening of mutants in chitin-containing co-cultures in 96-well plates, and identification of transposon insertion sites was performed as described previously (Jagmann et al., 2016).
Oxidative Stress Experiments
Pre-cultures of strains PAO1 and PAO1ΔrelAΔspoT were cultivated in LB medium in a volume of 3 ml in 15 ml glass tubes at 200 r.p.m. at 37°C for 16 h in a rotary shaker. Pre-cultures were used to inoculate main cultures in LB medium in a volume of 20 ml in a 100 ml Erlenmeyer flask at an OD600 = 0.001. Main cultures were incubated at 200 r.p.m. at 37° C for 20 h. At this timepoint, cultures had reached stationary phase and were harvested by centrifugation for 5 min at 9300 × g, washed with 0.9% NaCl, and resuspended in 0.9% NaCl to an OD600 = 1. Cell suspensions were incubated in the wells of a 24-well-plate (Sarstedt; 500 μl suspension per well) in the presence or absence of pyocyanin. Methanol served as solvent control. At distinct timepoints, CFU numbers were determined as described above.
Results
QS-Regulated Pyocyanin Production by P. aeruginosa in the Co-culture Is Not Induced by IQS-Integrated Phosphate Limitation
We could show in a previous study that pyocyanin production by P. aeruginosa under co-culture conditions is not caused by iron limitation. However, increasing the concentration of phosphate in the co-culture medium from 150 to 600 μM abolishes pyocyanin production by P. aeruginosa (Jagmann et al., 2010). It has been shown that induction of pyocyanin production in response to phosphate limitation is mediated by the newly identified QS signal molecule IQS (Lee et al., 2013), which is suggested to be a byproduct from pyochelin synthesis via PchA-F (Ye et al., 2014; Murcia et al., 2015).
To test whether IQS-integrated phosphate limitation caused the production of pyocyanin by strain PAO1 in the co-culture, we generated a pchA-F and an ambB mutant, which does not produce IQS as well (Lee et al., 2013), and co-cultivated them with A. hydrophila strain AH-1N and chitin. Both strain PAO1ΔambB and PAO1ΔpchA-F produced pyocyanin in co-cultures. Whereas the amounts produced by strain PAO1ΔambB equaled those in wild-type co-cultures, strain PAO1ΔpchA-F produced even higher amounts than the wild-type (Figure 1). These results indicated that the IQS-mediated sensing of phosphate limitation is not inducing pyocyanin production by P. aeruginosa in the co-culture.
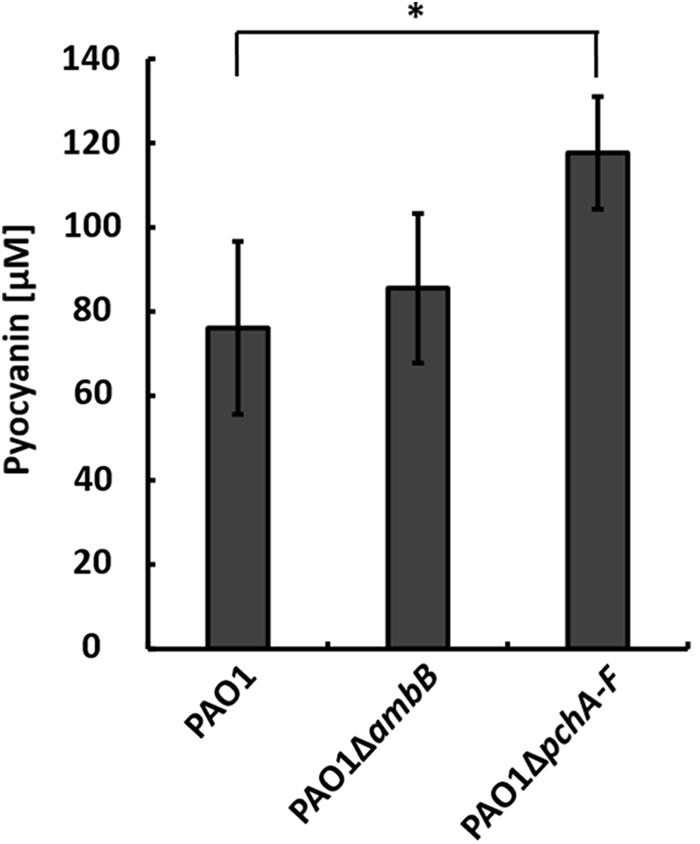
FIGURE 1. Production of pyocyanin in co-cultures with Aeromonas hydrophila strain AH-1N and chitin by Pseudomonas aeruginosa strains PAO1, PAO1ΔambB, and PAO1ΔpchA-F after 3 days of growth. Error bars indicate standard deviation (n = 3 for co-cultures with strains PAO1 and PAO1ΔambB, n = 5 for co-cultures with PAO1ΔpchA-F). Two-tailed Student’s t-test was performed for testing differences between groups. ∗Significant at P < 0.05.
QS-Regulated Pyocyanin Production in the Co-culture Is Induced by the Stringent Response
It is likely that P. aeruginosa faces general nutrient limitation in the first phase of the co-culture, because the cells depend on small amounts of acetate and ammonium and other exudates that are released by A. hydrophila (Jagmann et al., 2010).
To investigate, whether general nutrient limitation integrated via the stringent response is responsible for inducing QS and, in consequence, the production of pyocyanin in the co-culture, we generated the double mutant strain PAO1ΔrelAΔspoT and co-cultivated it with strain AH-1N. In these co-cultures, strain PAO1ΔrelAΔspoT reached about 10-fold lower CFU numbers compared to the wild-type and strain AH-1N was not inactivated (Figure 2A). No pyocyanin was produced by strain PAO1ΔrelAΔspoT (Figure 2B), which likely caused the survival of cells of strain AH-1N. Both pyocyanin production and inactivation of strain AH-1N by strain PAO1ΔrelAΔspoT could be restored by chromosomal integration of pUC18T[spoT] and mini-CTX2[relA], which contained the spoT and relA genes with the respective promoter regions (Figure 2B).
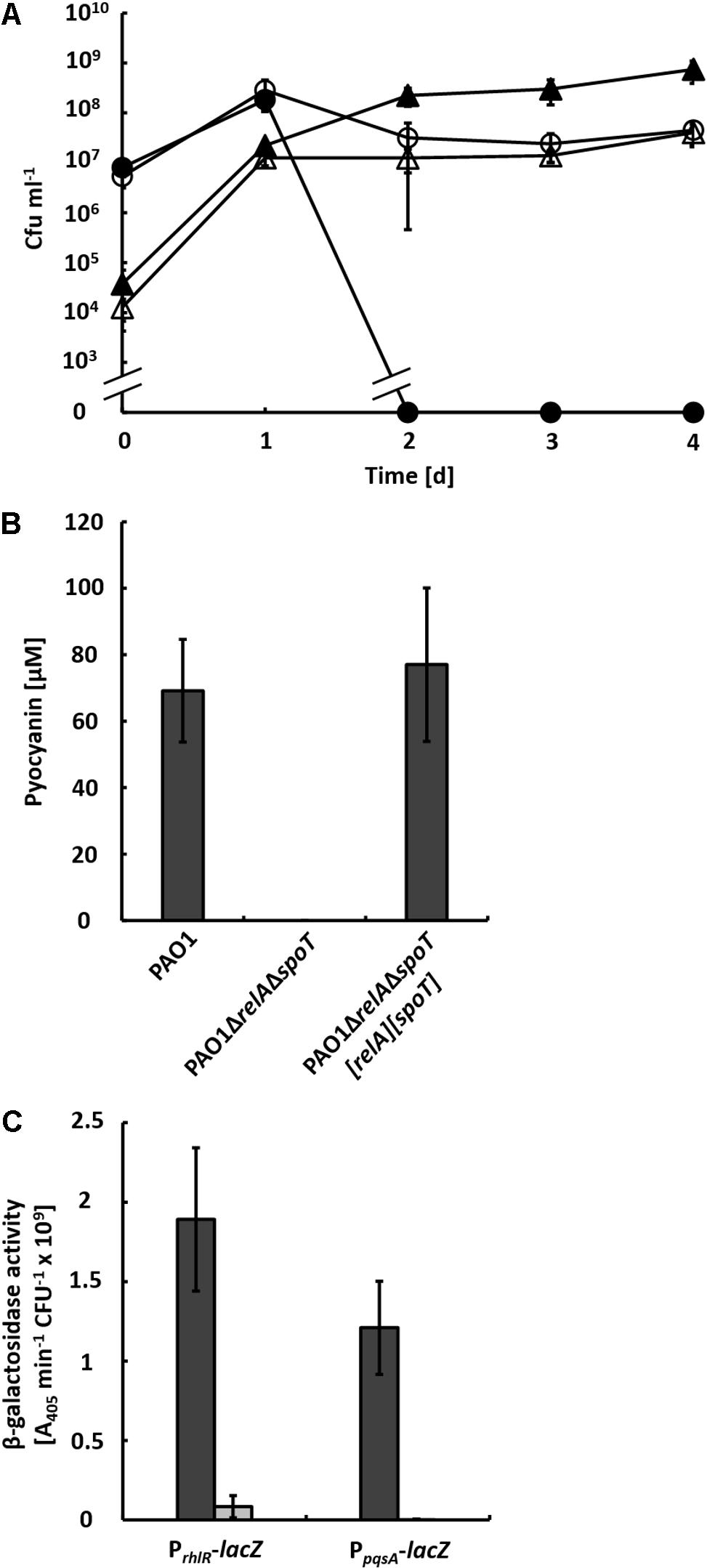
FIGURE 2. (A) Growth of A. hydrophila strain AH-1N with P. aeruginosa strains PAO1 and PAO1ΔrelAΔspoT in co-cultures with chitin. Colony-forming units (cfu) of strain AH-1N in co-cultures with strain PAO1 (closed circles), and with strain PAO1ΔrelAΔspoT (open circles); cfu of strain PAO1 (closed triangles); cfu of strain PAO1ΔrelAΔspoT (open triangles). (B) Production of pyocyanin in co-cultures with strain AH-1N and chitin by strains PAO1, PAO1ΔrelAΔspoT, and the complemented strain PAO1ΔrelAΔspoT[relA][spoT] after 3 days of growth. Error bars indicate standard deviation (n = 3 for co-cultures with strain PAO1, n = 7 for co-cultures with strains PAO1ΔrelAΔspoT and PAO1ΔrelAΔspoT[relA][spoT]). (C) β-Galactosidase activities of chromosomally encoded promoter-lacZ reporter gene fusions of rhlR and pqsA in strains PAO1 (dark gray bars) and PAO1ΔrelAΔspoT (light gray bars) in co-cultures with strain AH-1N and chitin after 2 days of growth. Error bars indicate standard deviation (n = 6).
It has been shown that stringent response mutants of E. coli have multiple amino acid requirements and, consequently, cannot grow in minimal medium (Xiao et al., 1991). To investigate whether incubation in co-culture minimal medium somehow contributed to the lack of pyocyanin production by strain PAO1ΔspoTΔrelA, we added 0.1% tryptone as source of amino acids to the co-culture. However, strain PAO1ΔrelAΔspoT did not produce pyocyanin under these co-culture conditions as well. Additionally, unlike the E. coli mutants, strain PAO1ΔrelAΔspoT could grow in minimal medium with succinate (data not shown), which has been observed previously (Vogt et al., 2011). These results indicated that the lack of pyocyanin production by strain PAO1ΔrelAΔspoT was not caused by a limitation of amino acids in the co-culture medium.
It has been shown previously that during growth in LB medium in single culture expression of the PQS system is upregulated in a ΔrelAΔspoT mutant of P. aeruginosa, while expression of both the Las and the Rhl system is reduced (Schafhauser et al., 2014). To test whether this expression pattern could also be observed in co-culture, we introduced transcriptional promoter-lacZ reporter gene fusions of pqsA and rhlR into strains PAO1 and PAO1ΔrelAΔspoT and monitored β-galactosidase activities in co-cultures with these strains and strain AH-1NΔlacZ (Figure 2C). According to β-galactosidase activities, transcription from the rhlR promoter was reduced in strain PAO1ΔrelAΔspoT (0.08 A405 nm min-1 cfu-1) compared to the wild-type (1.89 A405 nm min-1 cfu-1). However, no β-galactosidase activities of the PpqsA-lacZ reporter gene fusion could be measured in strain PAO1ΔrelAΔspoT. This indicated that there was no upregulation of expression of the PQS system in this mutant under co-culture conditions.
The Stringent Response in the Co-culture Is Mediated by SpoT
To further dissect the relative contributions of RelA and SpoT, respectively, to the phenotype of strain PAO1ΔrelAΔspoT in co-culture, we constructed the respective single mutant strains PAO1ΔrelA and PAO1ΔspoT. When strain PAO1ΔrelA was co-cultivated with strain AH-1N, the same amount of pyocyanin as in wild-type co-cultures was produced indicating that stressors integrated by RelA did not contribute to the induction of pyocyanin production in the co-culture (Figure 3).
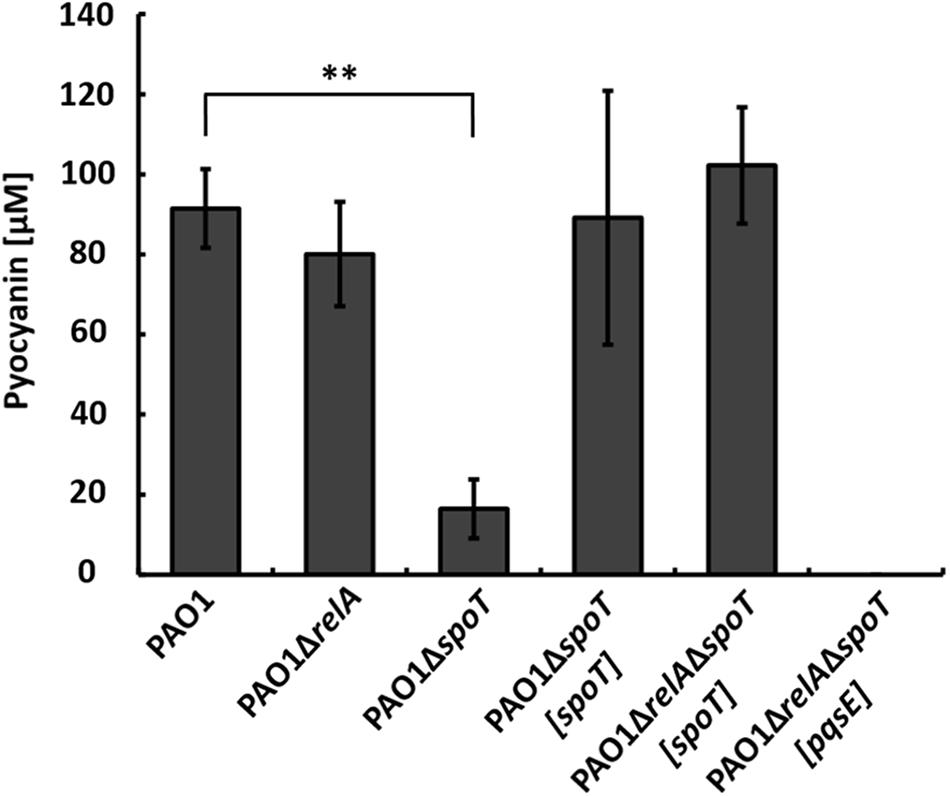
FIGURE 3. Production of pyocyanin in co-cultures with A. hydrophila strain AH-1N by P. aeruginosa strains PAO1, PAO1ΔrelA, PAO1ΔspoT, the complemented strains PAO1ΔspoT[spoT], PAO1ΔrelAΔspoT[spoT], and strain PAO1ΔrelAΔspoT[pqsE] after 3 days of growth. Error bars indicate standard deviation (n = 3 for co-cultures with strain PAO1, n = 6 for co-cultures with strain PAO1ΔrelAΔspoT[spoT] and n = 8 for all other co-cultures). Two-tailed Student’s t-test was performed for testing differences between groups. ∗∗Significant at P < 0.0001.
Despite repeated attempts, we could not obtain a ΔspoT single mutant when employing the gene deletion method using SOE-PCR (see “Materials and Methods” section). Therefore, we decided to generate a gene insertional mutant by inserting a res-cat-res-cassette conferring chloramphenicol resistance at position 1097 in the ORF of spoT. When mutants obtained on chloramphenicol selection plates were screened by PCR, a fragment with the correct size could only be detected in 2 of about 60 mutants. It has been discussed that such mutants may contain suppressor mutations that enable them to grow despite the lack of (p)ppGpp hydrolysis (Vogt et al., 2011; Hagmann, 2016). To investigate whether strain PAO1ΔspoT generated in this study contained such a mutation, we sequenced the relA gene of this mutant. Indeed, there was a single point mutation in the relA gene of strain PAO1ΔspoT leading to an amino acid exchange from glutamate to valine at position 359 located in the synthetase domain of RelA. When comparing the protein sequence of RelA from P. aeruginosa with the sequence of the catalytic fragment of the RelA/SpoT homolog Relseq of Streptococcus dysgalactiae subsp. equisimilis, for which the first crystal structure of the catalytic region was obtained (Hogg et al., 2004), this Glu359 residue was aligned to Glu336 of Relseq, which, along with other amino acids, forms H-bonds with GDP in the synthetase domain. We hypothesized that the substitution of Glu359 to a non-polar amino acid like valine would lead to a weaker binding of GDP and GTP, respectively, to the synthetase domain of RelA and, consequently, to a reduced formation of (p)ppGpp by RelA in strain PAO1ΔspoT, but not to a complete loss of (p)ppGpp formation as in strain PAO1ΔrelAΔspoT. To gain further insights into the role of spoT for induction of the stringent response in the co-culture, we tested strain PAO1ΔspoT in co-culture as well.
When strain PAO1ΔspoT was co-cultivated with strain AH-1N, pyocyanin production was reduced by about 80% compared to the wild-type, but was not completely abolished as in strain PAO1ΔrelAΔspoT. Pyocyanin production reaching wild-type levels could be restored in this mutant by complementation with chromosomally encoded spoT. This indicated that the stressors integrated by spoT were responsible for inducing QS and, thus, pyocyanin production in strain PAO1 in the co-culture. To verify this hypothesis, we complemented strain PAO1ΔspoTΔrelA with spoT only. When strain PAO1ΔrelAΔspoT[spoT] was co-cultivated with strain AH-1N, pyocyanin production was restored and reached amounts equally to those in co-cultures with the wild-type (Figure 3).
Overexpression of PqsE Does Not Restore Pyocyanin Production by Strain PAO1ΔrelAΔspoT in the Co-culture
PqsE is essential for the production of pyocyanin in P. aeruginosa, and expression of PqsE alone is sufficient to restore pyocyanin production in a pqsA mutant background (Rampioni et al., 2007; Farrow et al., 2008). As we could not measure transcription from the pqsA promoter in strain PAO1ΔrelAΔspoT in co-cultures (Figure 2C), we hypothesized that no or only small amounts of PqsE were produced in this strain. To test whether production of pyocyanin by strain PAO1ΔrelAΔspoT could be restored by overexpression of PqsE, we introduced pqsE on plasmid pUCP18 in this strain and co-cultivated it with strain AH-1N. However, overexpression of PqsE did not restore pyocyanin production by strain PAO1ΔrelAΔspoT in co-cultures (Figure 3).
It has been shown previously that a ΔrelAΔspoT mutant of P. aeruginosa faces increased endogenous oxidative stress during biofilm cultivation, which is caused by the prooxidant effects of AQs that are overproduced as a result of the enhanced expression of the PQS system. Additionally, activities of superoxide dismutase and catalase are reduced (Nguyen et al., 2011). It has been considered unlikely, however, that phenazines like pyocyanin, which also have prooxidant functions, cause the oxidative stress in this mutant, because it does not produce phenazines. Another possible explanation for the lack of phenazine production in a ΔrelAΔspoT mutant of P. aeruginosa may be that the susceptibility of this mutant to oxidative stress leads to the inhibition of phenazine production by unknown regulatory mechanisms, even if PqsE is overexpressed. If this was the case, a ΔrelAΔspoT mutant should likely show sensitivity toward pyocyanin. To test this hypothesis, we prepared cell suspensions of strains PAO1 and PAO1ΔrelAΔspoT harvested in stationary phase and incubated them in the presence of different concentrations of pyocyanin (Figure 4). After 50 h of incubation, CFUs of the wild-type had not significantly decreased in the presence of 100 μM or 200 μM pyocyanin. In contrast, CFUs of strain PAO1ΔrelAΔspoT decreased overtime and reached a 10-fold lower number after 50 h of incubation compared to the inoculation number. This decrease of CFUs, however, occurred both in cell suspensions with and without pyocyanin indicating a general defect in long-term survival of cells of strain PAO1ΔrelAΔspoT that was not influenced by the presence of pyocyanin and the resulting putative oxidative stress within the cells.
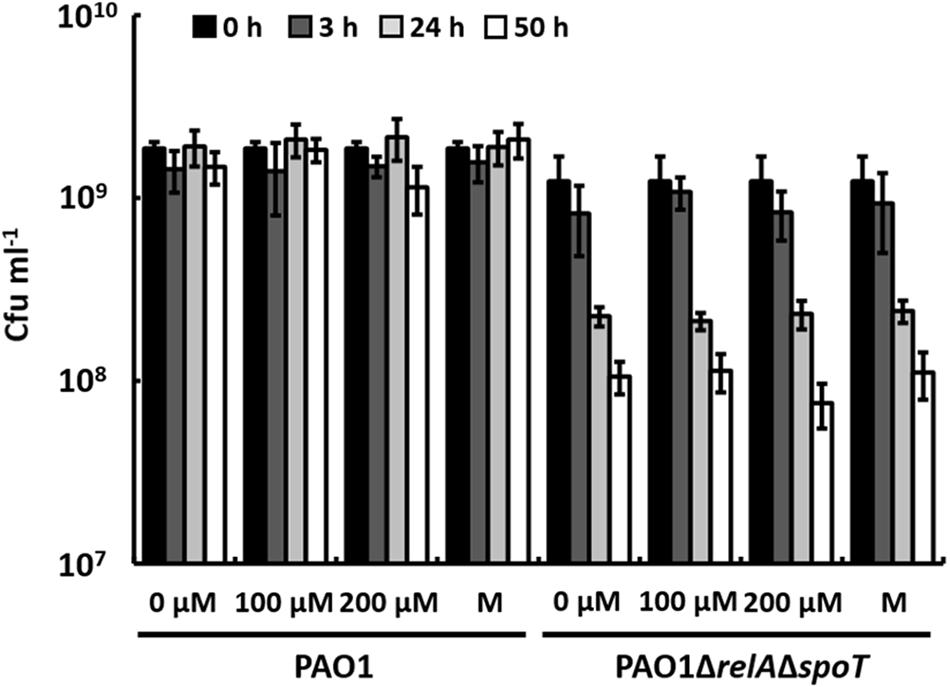
FIGURE 4. Effect of pyocyanin (0, 100, and 200 μM) and methanol (M; solvent control) on P. aeruginosa strains PAO1 and PAO1ΔrelAΔspoT after 0, 3, 24, and 50 h of incubation. Error bars indicate standard deviation (n = 3).
Genes Involved in Amino Acid Biosynthesis Are Essential for Pyocyanin Production in the Co-culture
To gain further insights into metabolic influences involved in regulation of pyocyanin production, we performed transposon mutagenesis and screened for mutants that did not produce pyocyanin in co-cultures anymore. To avoid the detection of auxotrophic mutants, we added 0.1% tryptone to the chitin-containing co-cultures, as this amount was sufficient to complement auxotrophic mutants in single cultures cultivated in minimal medium with succinate (data not shown). During these screenings, however, we again identified mutants with the transposon inserted in genes involved in amino acid biosynthesis that did not produce pyocyanin in co-culture. To study this effect further, we generated mutants with gene deletions in the amino acid biosynthesis pathways that were identified by transposon mutagenesis. We deleted genes encoding enzymes that catalyze the last step of biosynthesis of the respective amino acid, obtaining strains PAO1ΔlysA lacking diaminopimelate decarboxylase essential for lysine synthesis, PAO1ΔargH lacking argininosuccinate lyase essential for arginine synthesis, and PAO1ΔhisD lacking histidinol dehydrogenase essential for histidine synthesis.
When these mutants were incubated in co-cultures with strain AH-1N and chitin as sole carbon source, no pyocyanin was produced. Pyocyanin production could be restored by complementing the mutants with plasmid pBBR1MCS5 encoding the respective genes (Figure 5, dark gray bars).
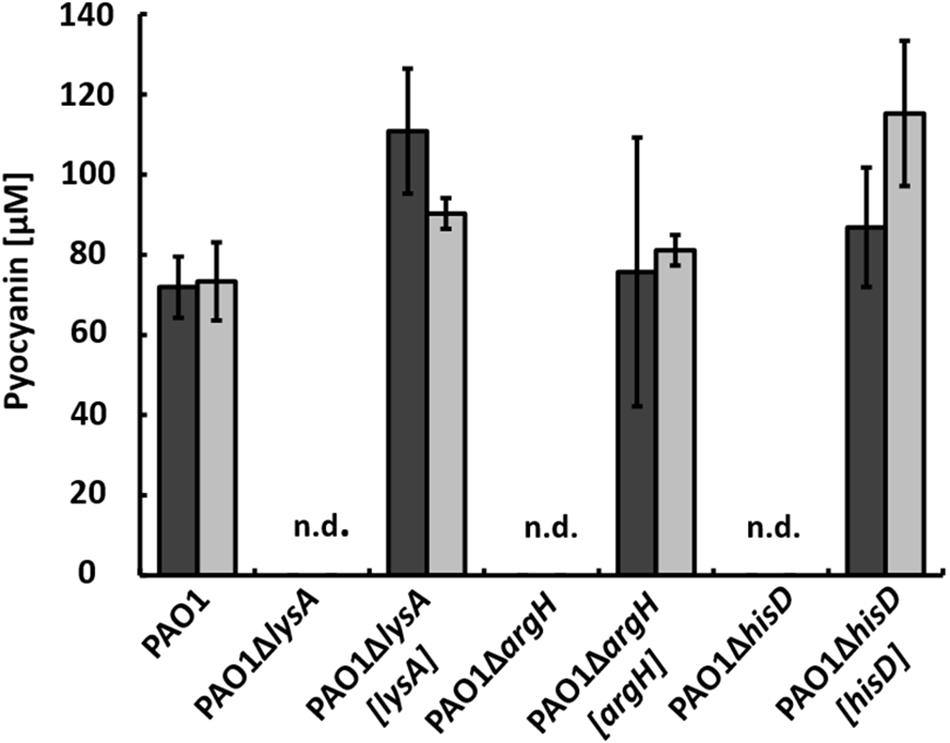
FIGURE 5. Production of pyocyanin in co-cultures with A. hydrophila strain AH-1N after 5 days of growth with chitin (dark gray bars) and with chitin and tryptone (light gray bars) by strain PAO1, the auxotrophic strains PAO1ΔlysA, PAO1ΔargH, PAO1ΔhisD, and the respective complemented strains PAO1ΔlysA[lysA], PAO1ΔargH[argH], and PAO1ΔhisD[hisD]. N.d., not detected; Error bars indicate standard deviation (n = 3).
When tryptone was added to the co-cultures, pyocyanin production by the wild-type was accelerated and cultures turned green after 24 h as described previously (Jagmann et al., 2010). Auxotrophic strains reached about the same CFU numbers compared to the wild-type in these co-cultures (data not shown), but again, no pyocyanin production could be observed despite the presence of tryptone as source of amino acids. Pyocyanin production in these co-cultures could again be restored by complementation with plasmid pBBR1MCS5 encoding the respective gene (Figure 5, light gray bars). To check whether auxotrophic mutants were generally unable to produce pyocyanin, we incubated strains PAO1ΔlysA, PAO1ΔargH, and PAO1ΔhisD in single cultures in LB medium. Under these conditions, all auxotrophic mutants produced pyocyanin in equal amounts as the wild-type (data not shown).
It might be possible that the phenotype of strains PAO1ΔlysA, PAO1ΔargH, and PAO1ΔhisD in co-cultures represented a general effect of amino acid auxotrophy. To support this hypothesis we generated an additional auxotrophic mutant strain PAO1ΔtrpB, which lacks the β-chain of the tryptophan synthase. Like the other auxotrophic strains, strain PAO1ΔtrpB did not produce pyocyanin either in co-cultures with chitin or with chitin and tryptone.
Overexpression of PqsE Does Not Restore Pyocyanin Production by Auxotrophic Strains in Co-culture
As mentioned above, PqsE is essential for the production of pyocyanin in P. aeruginosa (Rampioni et al., 2007). To test whether PqsE overexpression would restore pyocyanin production by auxotrophic strains in co-cultures as well, we introduced pqsE on plasmid pUCP18 into strains PAO1ΔlysA, PAO1ΔargH, PAO1ΔhisD, and PAO1ΔtrpB. These strains were co-cultivated with strain AH-1N in co-cultures with chitin and tryptone. However, overexpression of PqsE did not restore pyocyanin production by these strains in co-cultures.
These results indicated that amino acid auxotrophy in general was connected to the PqsE-mediated regulation of QS-controlled pyocyanin production under co-culture conditions.
Discussion
The parasitic exploitation of the chitinolytic properties of A. hydrophila by P. aeruginosa in the co-culture is strictly dependent on the QS-controlled production of pyocyanin, which renders the co-culture a suitable model system for studying QS regulation in P. aeruginosa. In this study, we aimed at identifying extra- and intracellular metabolic influences that are involved in this regulation. Our results showed that an intact starvation response as well as intact amino acid biosynthesis pathways are essential for inducing QS-controlled pyocyanin production under co-culture conditions.
We focused on starvation-related regulation as the trigger for QS activation in P. aeruginosa, because P. aeruginosa likely faces nutrient limitation at the beginning of the co-culture. Such a regulatory system is the stringent response, which employs (p)ppGpp as effector molecule, and which has been shown to regulate QS in P. aeruginosa (van Delden et al., 2001; Schafhauser et al., 2014). Stringent response mutants of P. aeruginosa are impaired in pyocyanin production, when cultivated in single culture in LB medium, and are less virulent in Drosophila melanogaster, rat lung, and mouse infection models (Nguyen et al., 2011; Vogt et al., 2011; Xu et al., 2016). (p)ppGpp is synthetized from GTP and ATP and leads to the upregulation of stress response processes and to the downregulation of proliferative processes (Potrykus and Cashel, 2008; Kanjee et al., 2012). Like E. coli and other Beta- and Gammaproteobacteria, P. aeruginosa harbors both the (p)ppGpp synthetase RelA and the bifunctional synthetase/hydrolase SpoT (Dalebroux et al., 2010; Hauryliuk et al., 2015). We could demonstrate that SpoT mediates the activation of QS and, thus, pyocyanin production by P. aeruginosa in the co-culture. This was shown by two observations: first, a relA mutant of P. aeruginosa produced the same amount of pyocyanin as the wild-type in co-culture, and, second, a relA spoT double mutant of P. aeruginosa could be fully complemented by SpoT. Studies on the stringent response in both E. coli and P. aeruginosa focused mainly on RelA, whose function is well-studied. RelA is associated with the ribosomes and responds to single amino acid limitation by sensing uncharged tRNAs (Hauryliuk et al., 2015; Arenz et al., 2016; Brown et al., 2016). SpoT, in contrast, responds to a variety of starvation stresses in E. coli, e.g., carbon, iron, and phosphate starvation, but the molecular details of SpoT regulation are still unknown (Hauryliuk et al., 2015). In P. aeruginosa, the stringent response mediated by SpoT has been shown to be essential for the detection of fatty acid starvation via activation of SpoT by the acyl carrier protein AcpP, for the expression of usp genes encoding universal stress proteins, and for full virulence in eukaryotic infection models (Boes et al., 2008; Battesti and Bouveret, 2009; Vogt et al., 2011). In these studies, a single environmental trigger has not been demonstrated, and besides binding to AcpP during fatty acid starvation the exact mechanisms by which these triggers are integrated into SpoT activation are unknown.
In our co-culture model system, the exact environmental stress signal that activates the SpoT-mediated stringent response is not known as well. However, it is very likely that the stringent response is activated in response to nutrient starvation. In the co-culture, P. aeruginosa faces both carbon and nitrogen starvation due to the lack of an utilizable substrate and of ammonium, and it is dependent on acetate and ammonium that are transiently released by A. hydrophila. We have shown that increasing the amount of phosphate in the co-culture abolishes pyocyanin production by P. aeruginosa (Jagmann et al., 2010), suggesting that the amounts of acetate and ammonium released by A. hydrophila are sufficient to suppress starvation signaling, and that P. aeruginosa rather faces phosphate starvation in the co-culture under normal cultivation conditions. In P. aeruginosa, phosphate limitation is integrated via the two component system PhoR-PhoB (Jensen et al., 2006). It has been demonstrated in E. coli, that phosphate starvation results in SpoT-dependent accumulation of (p)ppGpp, and that this accumulation is diminished in a phoB mutant showing a connection of phosphate sensing and the stringent response (Spira and Yagil, 1998). If phosphate limitation was the trigger for QS activation in P. aeruginosa in the co-culture, our results indicate, however, that IQS signaling is not involved in this regulation as an IQS negative mutant produced the same amount of pyocyanin compared to the wild-type. IQS has been shown to connect phosphate stress-response mechanisms to the Rhl and PQS systems (Lee et al., 2013). In this work, however, the stringent response was not addressed, and it may be possible that phosphate stress is integrated differently into QS under differential culture conditions. We cannot exclude, however, that activation of QS in and the production of pyocyanin by P. aeruginosa in the co-culture depended on the combination of several starvation signals that concertedly induced the SpoT-mediated stringent response.
In E. coli, SpoT exhibits a weak synthetic and strong hydrolytic activity for (p)ppGpp (An et al., 1979; Xiao et al., 1991). We assume that the balance of these activities was shifted to (p)ppGpp synthesis upon starvation in the co-culture, so that (p)ppGpp accumulation is independent of RelA. It has been hypothesized that the hydrolytic activity of SpoT is important for balancing the intracellular concentrations of (p)ppGpp produced by RelA during growth (An et al., 1979; Xiao et al., 1991; Hauryliuk et al., 2015). Therefore, a disruption of spoT is lethal in E. coli. The spoT gene has been designated as essential gene in P. aeruginosa as well (Hashimoto et al., 2005), and this is supported by the fact that we obtained spoT mutants of P. aeruginosa only, when there was a point mutation in relA. Failed attempts to generate a ΔspoT mutant of P. aeruginosa and other bacteria have been described earlier (Fisher et al., 2005; Manuel et al., 2011, 2012; Vogt et al., 2011; Holley et al., 2014; Hagmann, 2016). However, there are also studies that describe the generation of spoT mutants of P. aeruginosa (Viducic et al., 2006; Xu et al., 2016) and of other Pseudomonads (Takeuchi et al., 2012; Chatnaparat et al., 2015). To our knowledge, possible suppressor mutations, for example in relA, were not investigated in these studies.
We could show previously that both the Rhl and PQS system are crucial for pyocyanin production by P. aeruginosa in the co-culture (Jagmann et al., 2016). During cultivation of a relAspoT mutant of P. aeruginosa in LB medium in single culture, expression of the PQS system is upregulated, whereas the Rhl system is not fully expressed (Schafhauser et al., 2014). As shown by transcriptional lacZ fusion studies, expression of RhlR was downregulated in strain PAO1ΔrelAΔspoT in the co-culture as well. However, no upregulation of pqsA expression could be observed. Transcription of the pqsABCDE operon is negatively and positively regulated by RhlR and by PqsR, respectively, which bind to two distinctive start sites (Dötsch et al., 2012; Brouwer et al., 2014). Increased expression of the pqsABCDE operon would depend on transcription from the PqsR transcriptional start site. In our study we monitored expression from this PqsR-controlled promoter, indicating that the observed absence of β-galactosidase activity indeed originated from the absence of PqsR-induced pqsABCDE expression. It has been suggested that a third transcript comprising pqsD, pqsE, and the adjacent operon phnAB occurs during growth of P. aeruginosa in minimal medium, which would elevate the expression of PqsE and, in consequence, of PqsE-controlled virulence factors such as pyocyanin (Knoten et al., 2014). We cannot exclude that such a transcript occurs during growth under co-culture conditions as well. However, the relAspoT mutant produced no pyocyanin in the co-culture suggesting the absence of PqsE. In addition, it is known that QS induction in P. aeruginosa varies depending on environmental conditions, and the composition of the VGA minimal medium used in the study by Knoten et al. (2014) differs from the minimal medium B used in our experiments. Thus, it is feasible that under co-culture conditions, expression of both the PQS and the Rhl system is downregulated resulting in a lack of pyocyanin production.
While searching for intracellular metabolic influences on QS regulation, we identified amino acid auxotrophic strains of P. aeruginosa that did not produce pyocyanin in co-cultures even in the presence of tryptone as amino acid source. It has been hypothesized that intracellular metabolic fluxes play a role in starvation sensing in P. aeruginosa (Mellbye and Schuster, 2014), and amino acid biosynthesis pathways might be involved in such regulation.
In a pqsA mutant of P. aeruginosa, overexpression of PqsE restores pyocyanin production (Farrow et al., 2008). In contrast, overexpression of PqsE could not restore pyocyanin production by both strain PAO1 ΔrelAΔspoT and the auxotrophic mutants in co-cultures. Thus, we have identified two physiological conditions, under which P. aeruginosa is not responsive to PqsE overexpression. It has been observed before that a relAspoTpqsA triple mutant does not produce pyocyanin in the presence of PqsE (Nguyen et al., 2011). Concerning the auxotrophic mutants, it has been observed that in a pheA mutant of P. aeruginosa strain PA14 pyocyanin production could not be restored by PqsE overexpression as well (Brouwer, 2015). The chorismate mutase PheA catalyzes both the conversion of chorismate to prephenate and of prephenate to phenylpyruvate in the pathway of phenylalanine biosynthesis. The auxotrophic mutants investigated in our study were defect in the biosynthesis of amino acids that branch off different intermediates in the central metabolism. This suggests that the inability to produce pyocyanin even though PqsE is overexpressed might be a general effect of the inability to synthesize amino acids.
Constitutive expression of pqsE from plasmid pUCP18 is under the control of the lac promoter. Therefore, we hypothesize that a pqsE mRNA is at one point present in the cell, and that inhibition of PqsE-activated pyocyanin production is caused either by posttranscriptional regulation of PqsE or by regulation of pyocyanin biosynthesis genes. It has been suggested that the regulatory activity exerted by PqsE depends on the presence of the protein and is not caused by a pqsE regulatory RNA being involved in the expression of PqsE-controlled genes. However, regulatory mechanisms controlling gene expression that require the PqsE protein remain to be elucidated (Rampioni et al., 2016). It has been shown that PqsE represses the transcription of the pqsABCDE operon, and this effect is enhanced when PqsE is overexpressed (Rampioni et al., 2010). However, this negative autoregulatory role of PqsE should not be involved in the pyocyanin negative phenotype of the relAspoT mutant and the auxotrophic mutants overexpressing PqsE in the co-culture, because pyocyanin production caused by PqsE overexpression is independent of quinolone synthesis (Farrow et al., 2008), and because expression of pqsE from plasmid pUCP18 is independent of its native promoter.
Pyocyanin is synthesized via the products of the biosynthetic operons phzA1-G1 and phzA2-G2 that are expressed from differentially regulated promoter regions. It has been shown that the phz genes are upregulated when PqsE is overexpressed in the P. aeruginosa wild-type (Rampioni et al., 2010). However, knowledge about the connection between PqsE and metabolic influences, respectively, and the regulation of these operons is still scarce.
Taken together, by employing the co-culture model system, we have identified metabolic influences that are involved in QS regulation in P. aeruginosa. Both the stringent response and biosynthesis of amino acids have to be functional for QS-controlled pyocyanin production under these conditions. The previously discovered function of GbuA for the induction of pyocyanin biosynthesis in the co-culture might be linked to nutrient starvation and amino acid metabolism as well as this enzyme has been shown to be involved in an alternative arginine degradation pathway, which might be induced under starvation for the breakdown of endogenous proteins (Jagmann et al., 2016). As the co-culture represents conditions that do also prevail in human infections, that is nutrient limitation and competition with other bacteria (Harrison, 2007; Hogardt and Heesemann, 2010), regulatory mechanisms that play a role in the co-culture might also be important in infection processes.
Author Contributions
NJ conceived and performed the experiments, analyzed and interpreted the data, and wrote the manuscript. BP interpreted the data and commented on the manuscript.
Funding
This work was supported by the Deutsche Forschungs gemeinschaft (SFB 454 to BP) and by the Bundes ministerium für Wirtschaft und Energie (BMWI, ZIM program, KF3394402SB4 to BP).
Conflict of Interest Statement
The authors declare that the research was conducted in the absence of any commercial or financial relationships that could be construed as a potential conflict of interest.
Acknowledgments
The authors would like to thank Paul Williams (Nottingham) for providing plasmid pUCP18::pqsE and Pierre Cornelis for providing strain PAO1ΔpchEF. Experimental support by Stephan Pienkoß is acknowledged. Transposon mutagenesis was performed at the University of Konstanz in the laboratory of Bernhard Schink, who is gratefully thanked.
Supplementary Material
The Supplementary Material for this article can be found online at: https://www.frontiersin.org/articles/10.3389/fmicb.2018.00761/full#supplementary-material
References
Alonso, A., Rojo, F., and Martinez, J. L. (1999). Environmental and clinical isolates of Pseudomonas aeruginosa show pathogenic and biodegradative properties irrespective of their origin. Environ. Microbiol. 1, 421–430. doi: 10.1046/j.1462-2920.1999.00052.x
An, G., Justesen, J., Watson, R. J., and Friesen, J. D. (1979). Cloning the SpoT gene of Escherichia coli - Identification of the SpoT gene product. J. Bacteriol. 137, 1100–1110.
Arenz, S., Abdelshahid, M., Sohmen, D., Payoe, R., Starosta, A. L., Berninghausen, O., et al. (2016). The stringent factor RelA adopts an open conformation on the ribosome to stimulate ppGpp synthesis. Nucleic Acids Res. 44, 6471–6481. doi: 10.1093/nar/gkw470
Battesti, A., and Bouveret, E. (2009). Bacteria possessing two RelA/SpoT-like proteins have evolved a specific stringent response involving the Acyl Carrier Protein-SpoT interaction. J. Bacteriol. 191, 616–624. doi: 10.1128/JB.01195-08
Bazire, A., Dheilly, A., Diab, F., Morin, D., Jebbar, M., Haras, D., et al. (2005). Osmotic stress and phosphate limitation alter production of cell-to-cell signal molecules and rhamnolipid biosurfactant by Pseudomonas aeruginosa. FEMS Microbiol. Lett. 253, 125–131. doi: 10.1016/j.femsle.2005.09.029
Boes, N., Schreiber, K., and Schobert, M. (2008). SpoT-triggered stringent response controls usp gene expression in Pseudomonas aeruginosa. J. Bacteriol. 190, 7189–7199. doi: 10.1128/JB.00600-08
Brouwer, S. (2015). New Perspectives on Post-transcriptional Regulation Mechanisms in Pseudomonas aeruginosa. Doctoral dissertation, University of Braunschweig, Braunschweig.
Brouwer, S., Pustelny, C., Ritter, C., Klinkert, B., Narberhaus, F., and Haussler, S. (2014). The PqsR and RhlR transcriptional regulators determine the level of Pseudomonas quinolone signal synthesis in Pseudomonas aeruginosa by producing two different pqsABCDE mRNA isoforms. J. Bacteriol. 196, 4163–4171. doi: 10.1128/JB.02000-14
Brown, A., Fernandez, I. S., Gordiyenko, Y., and Ramakrishnan, V. (2016). Ribosome-dependent activation of stringent control. Nature 534, 277–280. doi: 10.1038/nature17675
Cai, Z., Liu, Y., Chen, Y. C., Yam, J. K. H., Chew, S. C., Chua, S. L., et al. (2015). RpoN regulates virulence factors of Pseudomonas aeruginosa via modulating the PqsR quorum sensing regulator. Int. J. Mol. Sci. 16, 28311–28319. doi: 10.3390/ijms161226103
Chatnaparat, T., Li, Z., Korban, S. S., and Zhao, Y. F. (2015). The bacterial alarmone (p)ppGpp is required for virulence and controls cell size and survival of Pseudomonas syringae on plants. Environ. Microbiol. 17, 4253–4270. doi: 10.1111/1462-2920.1274
Choi, K. H., and Schweizer, H. P. (2006). Mini-Tn7 insertion in bacteria with single attTn7 sites: example Pseudomonas aeruginosa. Nat. Protoc. 1, 153–161. doi: 10.1038/nprot.2006.24
Chuanchuen, R., Narasaki, C. T., and Schweizer, H. P. (2002). Benchtop and microcentrifuge preparation of Pseudomonas aeruginosa competent cells. Biotechniques 760, 762–763.
Clarke, P. H. (1982). The metabolic versatility of pseudomonads. Antonie Van Leeuwenhoek 48, 105–130. doi: 10.1007/BF00405197
Coggan, K. A., and Wolfgang, M. C. (2012). Global regulatory pathways and cross-talk control Pseudomonas aeruginosa environmental lifestyle and virulence phenotype. Curr. Issues Mol. Biol. 14, 47–70.
Dalebroux, Z. D., Svensson, S. L., Gaynor, E. C., and Swanson, M. S. (2010). ppGpp conjures bacterial virulence. Microbiol. Mol. Biol. Rev. 74, 171–199. doi: 10.1128/MMBR.00046-09
Deziel, E., Lepine, F., Milot, S., He, J., Mindrinos, M. N., Tompkins, R. G., et al. (2004). Analysis of Pseudomonas aeruginosa 4-hydroxy-2-alkylquinolines (HAQs) reveals a role for 4-hydroxy-2-heptylquinoline in cell-to-cell communication. Proc. Natl. Acad. Sci. U.S.A. 101, 1339–1344. doi: 10.1073/pnas.0307694100
Dötsch, A., Eckweiler, D., Schniederjans, M., Zimmermann, A., Jensen, V., Scharfe, M., et al. (2012). The Pseudomonas aeruginosa transcriptome in planktonic cultures and static biofilms using RNA sequencing. PLoS One 7:e31092. doi: 10.1371/journal.pone.0031092
Drees, S. L., and Fetzner, S. (2015). PqsE of Pseudomonas aeruginosa acts as pathway-specific thioesterase in the biosynthesis of alkylquinolone signaling molecules. Chem. Biol. 22, 611–618. doi: 10.1016/j.chembiol.2015.04.012
Driscoll, J. A., Brody, S. L., and Kollef, M. H. (2007). The epidemiology, pathogenesis and treatment of Pseudomonas aeruginosa infections. Drugs 67, 351–368. doi: 10.2165/00003495-200767030-00003
Duan, K., and Surette, M. G. (2007). Environmental regulation of Pseudomonas aeruginosa PAO1 las and rhl quorum-sensing systems. J. Bacteriol. 189, 4827–4836. doi: 10.1128/JB.00043-07
Dubern, J. F., and Diggle, S. P. (2008). Quorum sensing by 2-alkyl-4-quinolones in Pseudomonas aeruginosa and other bacterial species. Mol. Biosyst. 4, 882–888. doi: 10.1039/b803796p
Essar, D. W., Eberly, L., Hadero, A., and Crawford, I. (1990). Identification and characterization of genes for a second anthranilate synthase in Pseudomonas aeruginosa: interchangeability of the two anthranilate synthases and evolutionary implications. J. Bacteriol. 172, 884–900. doi: 10.1128/jb.172.2.884-900.1990
Farrow, J. M. III, Sund, Z. M., Ellison, M. L., Wade, D. S., Coleman, J. P., and Pesci, E. C. (2008). PqsE functions independently of PqsR-Pseudomonas quinolone signal and enhances the rhl quorum-sensing system. J. Bacteriol. 190, 7043–7051. doi: 10.1128/JB.00753-0
Fisher, S. D., Reger, A. D., Baum, A., and Hill, S. A. (2005). RelA alone appears essential for (p)ppGpp production when Neisseria gonorrhoeae encounters nutritional stress. FEMS Microbiol. Lett. 248, 1–8. doi: 10.1016/j.femsle.2005.05.014
Gallagher, L. A., Mcknight, S. L., Kuznetsova, M. S., Pesci, E. C., and Manoil, C. (2002). Functions required for extracellular quinolone signaling by Pseudomonas aeruginosa. J. Bacteriol. 184, 6472–6480. doi: 10.1128/JB.184.23.6472-6480.2002
Hagmann, L. V. (2016). Stringent Response Regulation and its Impact on ex Vivo Survival in the Commensal Pathogen Neisseria meningitidis. Doctoral dissertation, University of Würzburg, Würzburg.
Hardalo, C., and Edberg, S. C. (1997). Pseudomonas aeruginosa: assessment of risk from drinking water. Crit. Rev. Microbiol. 23, 47–75. doi: 10.3109/10408419709115130
Harrison, F. (2007). Microbial ecology of the cystic fibrosis lung. Microbiology 153, 917–923. doi: 10.1099/mic.0.2006/004077-0
Hashimoto, M., Ichimura, T., Mizoguchi, H., Tanaka, K., Fujimitsu, K., Keyamura, K., et al. (2005). Cell size and nucleoid organization of engineered Escherichia coli cells with a reduced genome. Mol. Microbiol. 55, 137–149. doi: 10.1111/j.1365-2958.2004.04386.x
Hauryliuk, V., Atkinson, G. C., Murakami, K. S., Tenson, T., and Gerdes, K. (2015). Recent functional insights into the role of (p)ppGpp in bacterial physiology. Nat. Rev. Microbiol. 13, 298–309. doi: 10.1038/nrmicro3448
Hilbi, H., Weber, S. S., Ragaz, C., Nyfeler, Y., and Urwyler, S. (2007). Environmental predators as models for bacterial pathogenesis. Environ. Microbiol. 9, 563–575. doi: 10.1111/j.1462-2920.2007.01238.x
Ho, S. N., Hunt, H. D., Horton, R. M., Pullen, J. K., and Pease, L. R. (1989). Site-directed mutagenesis by overlap extension using the polymerase chain reaction. Gene 77, 51–59. doi: 10.1016/0378-1119(89)90358-2
Hoang, T. T., Kutchma, A. J., Becher, A., and Schweizer, H. P. (2000). Integration-proficient plasmids for Pseudomonas aeruginosa: site-specific integration and use for engineering of reporter and expression strains. Plasmid 43, 59–72. doi: 10.1006/plas.1999.1441
Hogardt, M., and Heesemann, J. (2010). Adaptation of Pseudomonas aeruginosa during persistence in the cystic fibrosis lung. Int. J. Med. Microbiol. 300, 557–562. doi: 10.1016/j.ijmm.2010.08.008
Hogg, T., Mechold, U., Malke, H., Cashel, M., and Cell, R. H. (2004). Conformational antagonism between opposing active sites in a bifunctional RelA/SpoT homolog modulates (p)ppGpp metabolism during the stringent response. Cell 117, 415–415. doi: 10.1016/S0092-8674(04)00406-4
Holley, C., Gangaiah, D., Li, W., Fortney, K. R., Janowicz, D. M., Ellinger, S., et al. (2014). A (p)ppGpp-null mutant of Haemophilus ducreyi is partially attenuated in humans due to multiple conflicting phenotypes. Infect. Immun. 82, 3492–3502. doi: 10.1128/IAI.01994-14
Jagmann, N., Bleicher, V., Busche, T., Kalinowski, J., and Philipp, B. (2016). The guanidinobutyrase GbuA is essential for the alkylquinolone-regulated pyocyanin production during parasitic growth of Pseudomonas aeruginosa in co-culture with Aeromonas hydrophila. Environ. Microbiol. 18, 3550–3564. doi: 10.1111/1462-2920.13419
Jagmann, N., Brachvogel, H. P., and Philipp, B. (2010). Parasitic growth of Pseudomonas aeruginosa in co-culture with the chitinolytic bacterium Aeromonas hydrophila. Environ. Microbiol. 12, 1787–1802. doi: 10.1111/j.1462-2920.2010.02271.x
Jensen, V., Lons, D., Zaoui, C., Bredenbruch, F., Meissner, A., Dieterich, G., et al. (2006). RhlR expression in Pseudomonas aeruginosa is modulated by the Pseudomonas quinolone signal via PhoB-dependent and -independent pathways. J. Bacteriol. 188, 8601–8606. doi: 10.1128/JB.01378-06
Jimenez, P. N., Koch, G., Thompson, J. A., Xavier, K. B., Cool, R. H., and Quax, W. J. (2012). The multiple signaling systems regulating virulence in Pseudomonas aeruginosa. Microbiol. Mol. Biol. Rev. 76, 46–65. doi: 10.1128/MMBR.05007-11
Kanjee, U., Ogata, K., and Houry, W. A. (2012). Direct binding targets of the stringent response alarmone (p)ppGpp. Mol. Microbiol. 85, 1029–1043. doi: 10.1111/j.1365-2958.2012.08177.x
Knoten, C. A., Wells, G., Coleman, J. P., and Pesci, E. C. (2014). A conserved suppressor mutation in a tryptophan auxotroph results in dysregulation of Pseudomonas quinolone signal synthesis. J. Bacteriol. 196, 2413–2422. doi: 10.1128/JB.01635-14
Lee, J., Wu, J., Deng, Y. Y., Wang, J., Wang, C., Wang, J. H., et al. (2013). A cell-cell communication signal integrates quorum sensing and stress response. Nat. Chem. Biol. 9, 339–343. doi: 10.1038/nchembio.1225
Magnusson, L. U., Farewell, A., and Nystrom, T. (2005). ppGpp: a global regulator in Escherichia coli. Trends Microbiol. 13, 236–242. doi: 10.1016/j.tim.2005.03.008
Manuel, J., Berry, C., Selin, C., Fernando, W. G. D., and De Kievit, T. R. (2011). Repression of the antifungal activity of Pseudomonas sp strain DF41 by the stringent response. Appl. Environ. Microbiol. 77, 5635–5642. doi: 10.1128/AEM.02875-10
Manuel, J., Selin, C., Fernando, W. G. D., and De Kievit, T. (2012). Stringent response mutants of Pseudomonas chlororaphis PA23 exhibit enhanced antifungal activity against Sclerotinia sclerotiorum in vitro. Microbiology 158, 207–216. doi: 10.1099/mic.0.053082-0
Medina, G., Juarez, K., Diaz, R., and Soberon-Chavez, G. (2003). Transcriptional regulation of Pseudomonas aeruginosa rhlR, encoding a quorum-sensing regulatory protein. Microbiology 149, 3073–3081. doi: 10.1099/mic.0.26282-0
Mellbye, B., and Schuster, M. (2014). Physiological framework for the regulation of quorum sensing-dependent public goods in Pseudomonas aeruginosa. J. Bacteriol. 196, 1155–1164. doi: 10.1128/JB.01223-13
Mena, K. D., and Gerba, C. P. (2009). Risk assessment of Pseudomonas aeruginosa in water. Rev. Environ. Contam. Toxicol. 201, 71–115. doi: 10.1007/978-1-4419-0032-6_3
Morales, D. K., Jacobs, N. J., Rajamani, S., Krishnamurthy, M., Cubillos-Ruiz, J. R., and Hogan, D. A. (2011). Antifungal mechanisms by which a novel Pseudomonas aeruginosa phenazine toxin kills Candida albicans in biofilms. Mol. Microbiol. 78, 1379–1392. doi: 10.1111/j.1365-2958.2010.07414.x
Murcia, N. R., Lee, X. Y., Waridel, P., Maspoli, A., Linker, H. J., Chai, T. C., et al. (2015). The Pseudomonas aeruginosa antimetabolite L-2-amino-4-methoxy-trans-3-butenoic acid (AMB) is made from glutamate and two alanine residues via a thiotemplate-linked tripeptide precursor. Front. Microbiol. 6:170. doi: 10.3389/fmicb.2015.00170
Nguyen, D., Joshi-Datar, A., Lepine, F., Bauerle, E., Olakanmi, O., Beer, K., et al. (2011). Active starvation responses mediate antibiotic tolerance in biofilms and nutrient-limited bacteria. Science 334, 982–986. doi: 10.1126/science.1211037
Oglesby, A. G., Farrow, J. M. III, Lee, J. H., Tomaras, A. P., Greenberg, E. P., Pesci, E. C., et al. (2008). The influence of iron on Pseudomonas aeruginosa physiology: a regulatory link between iron and quorum sensing. J. Biol. Chem. 283, 15558–15567. doi: 10.1074/jbc.M707840200
Palmer, K. L., Aye, L. M., and Whiteley, M. (2007). Nutritional cues control Pseudomonas aeruginosa multicellular behavior in cystic fibrosis sputum. J. Bacteriol. 189, 8079–8087. doi: 10.1128/JB.01138-07
Potrykus, K., and Cashel, M. (2008). (p)ppGpp: still magical? Annu. Rev. Microbiol. 62, 35–51. doi: 10.1146/annurev.micro.62.081307.162903
Rahme, L. G., Stevens, E. J., Wolfort, S. F., Shao, J., Tompkins, R. G., and Ausubel, F. M. (1995). Common virulence factors for bacterial pathogenicity in plants and animals. Science 268, 1899–1902. doi: 10.1126/science.7604262
Rampioni, G., Falcone, M., Heeb, S., Frangipani, E., Fletcher, M. P., Dubern, J. F., et al. (2016). Unravelling the genome-wide contributions of specific 2-alkyl-4-quinolones and PqsE to quorum sensing in Pseudomonas aeruginosa. PLoS Pathog. 12:e1006029. doi: 10.1371/journal.ppat.1006029
Rampioni, G., Pustelny, C., Fletcher, M. P., Wright, V. J., Bruce, M., Rumbaugh, K. P., et al. (2010). Transcriptomic analysis reveals a global alkyl-quinolone-independent regulatory role for PqsE in facilitating the environmental adaptation of Pseudomonas aeruginosa to plant and animal hosts. Environ. Microbiol. 12, 1659–1673. doi: 10.1111/j.1462-2920.2010.02214.x
Rampioni, G., Schuster, M., Greenberg, E. P., Bertani, I., Grasso, M., Venturi, V., et al. (2007). RsaL provides quorum sensing homeostasis and functions as a global regulator of gene expression in Pseudomonas aeruginosa. Mol. Microbiol. 66, 1557–1565. doi: 10.1111/j.1365-2958.2007.06029.x
Ringen, L. M., and Drake, C. H. (1952). A study of the incidence of Pseudomonas aeruginosa from various natural sources. J. Bacteriol. 64, 841–845.
Schafhauser, J., Lepine, F., Mckay, G., Ahlgren, H. G., Khakimova, M., and Nguyen, D. (2014). The stringent response modulates 4-hydroxy-2-alkylquinoline biosynthesis and quorum-sensing hierarchy in Pseudomonas aeruginosa. J. Bacteriol. 196, 1641–1650. doi: 10.1128/JB.01086-13
Schmidberger, A., Henkel, M., Hausmann, R., and Schwartz, T. (2014). Influence of ferric iron on gene expression and rhamnolipid synthesis during batch cultivation of Pseudomonas aeruginosa PAO1. Appl. Microbiol. Biotechnol. 98, 6725–6737. doi: 10.1007/s00253-014-5747-y
Spira, B., and Yagil, E. (1998). The relation between ppGpp and the PHO regulon in Escherichia coli. Mol. Gen. Genet. 257, 469–477. doi: 10.1007/s004380050671
Takeuchi, K., Yamada, K., and Haas, D. (2012). ppGpp controlled by the Gac/Rsm regulatory pathway sustains biocontrol activity in Pseudomonas fluorescens CHA0. Mol. Plant Microbe Interact. 25, 1440–1449. doi: 10.1094/MPMI-02-12-0034-R
Thompson, L. S., Webb, J. S., Rice, S. A., and Kjelleberg, S. (2003). The alternative sigma factor RpoN regulates the quorum sensing gene rhlI in Pseudomonas aeruginosa. FEMS Microbiol. Lett. 220, 187–195. doi: 10.1016/S0378-1097(03)00097-1
van Delden, C., Comte, R., and Bally, A. M. (2001). Stringent response activates quorum sensing and modulates cell density-dependent gene expression in Pseudomonas aeruginosa. J. Bacteriol. 183, 5376–5384. doi: 10.1128/JB.183.18.5376-5384.2001
Viducic, D., Ono, T., Murakami, K., Susilowati, H., Kayama, S., Hirota, K., et al. (2006). Functional analysis of spoT, relA and dksA genes on quinolone tolerance in Pseudomonas aeruginosa under nongrowing condition. Microbiol. Immunol. 50, 349–357. doi: 10.1111/j.1348-0421.2006.tb03793.x
Vogt, S. L., Green, C., Stevens, K. M., Day, B., Erickson, D. L., Woods, D. E., et al. (2011). The stringent response is essential for Pseudomonas aeruginosa virulence in the rat lung agar bead and Drosophila melanogaster feeding models of infection. Infect. Immun. 79, 4094–4104. doi: 10.1128/IAI.00193-11
Wagner, V. E., Bushnell, D., Passador, L., Brooks, A. I., and Iglewski, B. H. (2003). Microarray analysis of Pseudomonas aeruginosa quorum-sensing regulons: effects of growth phase and environment. J. Bacteriol. 185, 2080–2095. doi: 10.1128/JB.185.7.2080-2095.2003
Welsh, M. A., and Blackwell, H. E. (2016). Chemical genetics reveals environment-specific roles for quorum sensing circuits in Pseudomonas aeruginosa. Cell Chem. Biol. 23, 361–369. doi: 10.1016/j.chembiol.2016.01.006
Wenner, N., Maes, A., Cotado-Sampayo, M., and Lapouge, K. (2014). NrsZ: a novel, processed, nitrogen-dependent, small non-coding RNA that regulates Pseudomonas aeruginosa PAO1 virulence. Environ. Microbiol. 16, 1053–1068. doi: 10.1111/1462-2920.12272
Williams, P. (2007). Quorum sensing, communication and cross-kingdom signalling in the bacterial world. Microbiology 153, 3923–3938. doi: 10.1099/mic.0.2007/012856-0
Williams, P., and Camara, M. (2009). Quorum sensing and environmental adaptation in Pseudomonas aeruginosa: a tale of regulatory networks and multifunctional signal molecules. Curr. Opin. Microbiol. 12, 182–191. doi: 10.1016/j.mib.2009.01.005
Xiao, G., Deziel, E., He, J., Lepine, F., Lesic, B., Castonguay, M. H., et al. (2006). MvfR, a key Pseudomonas aeruginosa pathogenicity LTTR-class regulatory protein, has dual ligands. Mol. Microbiol. 62, 1689–1699. doi: 10.1111/j.1365-2958.2006.05462.x
Xiao, H., Kalman, M., Ikehara, K., Zemel, S., Glaser, G., and Cashel, M. (1991). Residual guanosine 3′,5′-bispyrophosphate synthetic activity of relA null mutants can be eliminated by spoT null mutations. J. Biol. Chem. 266, 5980–5990.
Xu, X. H., Yu, H., Zhang, D., Xiong, J. Z., Qiu, J., Xin, R., et al. (2016). Role of ppGpp in Pseudomonas aeruginosa acute pulmonary infection and virulence regulation. Microbiol. Res. 192, 84–95. doi: 10.1016/j.micres.2016.06.005
Keywords: Pseudomonas aeruginosa, Aeromonas hydrophila, pyocyanin, quorum sensing, stringent response, amino acids, co-culture
Citation: Jagmann N and Philipp B (2018) SpoT-Mediated Regulation and Amino Acid Prototrophy Are Essential for Pyocyanin Production During Parasitic Growth of Pseudomonas aeruginosa in a Co-culture Model System With Aeromonas hydrophila. Front. Microbiol. 9:761. doi: 10.3389/fmicb.2018.00761
Received: 14 December 2017; Accepted: 04 April 2018;
Published: 18 April 2018.
Edited by:
Satoshi Tsuneda, Waseda University, JapanReviewed by:
Brett Mellbye, Oregon State University, United StatesSang Sun Yoon, Yonsei University, South Korea
Copyright © 2018 Jagmann and Philipp. This is an open-access article distributed under the terms of the Creative Commons Attribution License (CC BY). The use, distribution or reproduction in other forums is permitted, provided the original author(s) and the copyright owner are credited and that the original publication in this journal is cited, in accordance with accepted academic practice. No use, distribution or reproduction is permitted which does not comply with these terms.
*Correspondence: Nina Jagmann, bmluYS5qYWdtYW5uQHVuaS1tdWVuc3Rlci5kZQ==