- 1Key Laboratory of Experimental Marine Biology, Institute of Oceanology, Chinese Academy of Sciences, Qingdao, China
- 2Laboratory for Marine Biology and Biotechnology, Qingdao National Laboratory for Marine Science and Technology, Qingdao, China
- 3College of Earth Sciences, University of Chinese Academy of Sciences, Beijing, China
- 4College of Life Sciences, Qingdao University, Qingdao, China
Mercury-mediated toxicity remains one of the greatest barriers against microbial survival, even though bacterial resistance to mercury compounds can occur. However, the genetic and physiological adaptations of bacteria to mercury stress still remains unclear. Here, we show that the marine bacterium Pseudomonas stutzeri 273 is resistant to 50 μM Hg2+ and removes up to 94% Hg2+ from culture. Using gene homologous recombination and complementation, we show that genes encoding Hg2+-transport proteins MerT, MerP, the mercuric reductase MerA and the regulatory protein MerD are essential for bacterial mercuric resistance when challenged with Hg2+. Further, mercury stress inhibits flagellar development, motility, chemotaxis and biofilm formation of P. stutzeri 273, which are verified by transcriptomic and physiological analyses. Surprisingly, we discover that MerF, a previously reported Hg2+-transporter, determines flagellar development, motility and biofilm formation in P. stutzeri 273 by genetic and physiological analyses. Our results strongly indicate that MerF plays an integral role in P. stutzeri 273 to develop physiological responses to mercury stress. Notably, MerF homologs are also prevalent in different human pathogens. Using this unique target may provide novel strategies to control these pathogenic bacteria, given the role of MerF in flagella and biofilm formation. In summary, our data provide an original report on MerF in bacterial physiological development and suggest that the mer in marine bacteria has evolved through progressive, sequential recruitment of novel functions over time.
Introduction
Mercury, as one of the most toxic heavy metals naturally present in the earth, endangers the environment and causes a variety of diseases in humans and animals (Dash and Das, 2012). Specifically, exposure to mercury stress can dramatically decrease reproduction like fertilization capability, hatchability, viability and sperm motility (Dietrich et al., 2010). In the aquatic environment including the ocean, increasing evidence indicates that sublethal mercury pollution may cause a long-term decline and eventual extinction of species by adversely affecting fertilization and limiting propagation of a species (Kime, 1995).
Since mercury is ubiquitous in the earth, microorganisms inevitably encounter this heavy metal in their natural environment (Singh et al., 2014). Responding to environmental changes is a fundamental property of unicellular organisms who directly interact with an ever-changing microenvironment (Singh et al., 2014). Mercuric effects on live microorganisms impact their physiology (Dietrich et al., 2010). Through evolution, motility and chemotaxis are remarkably evolved features of bacterial physiology and strengthen bacterial adaptive capabilities to mercuric stress (Gadd, 2010). Chemotaxis of a bacterium toward metal stress largely depends on its physiological capability to utilize or resist the metallic environment or bacterial swarming powered by rotating helical flagella, a universal movement patterns (Kearns, 2010). In addition, bacteria within a developing biofilm may require chemotaxis and/or motility to move along the surface, thereby facilitating the growth and spread of the biofilm (Stelmack et al., 1999). With extensive evolution, mercury-resistant bacteria obtain the mer (mercuric ion resistance) operon in their genome to respond to stress from toxic mercury compounds. The mer operon enables bacteria to survive in the presence of mercury and reduce it to volatile, less-toxic Hg0, which diffuses out of the cell (Mathema et al., 2011).
Of the known bacterial heavy metal resistance systems, the mer operon is an intensively studied mercurial resistance system. Typically, the mer operon consists of a series of structural genes that transport and transform inorganic and organic mercury, such as mercuric reductase (MerA), organomercury lyase (MerB), periplasmic Hg2+ scavenging protein (MerP), one or more inner membrane spanning proteins (MerT, MerC, MerE, MerF, and MerG) that transport Hg2+ to the cytoplasm for reduction by MerA and regulatory proteins (MerR, MerD). Expression of the mer operon is tightly regulated by the dual function transcriptional regulator, MerR, which binds to the mer operator/promoter (O/P) region and acts as a transcriptional repressor or activator in the absence or presence of Hg2+ (Mathema et al., 2011). MerD, the other proposed regulatory protein of mer operon, may function as either an activator (Nucifora et al., 1989) or a repressor of the mer operon (Mukhopadhyay et al., 1991). MerD can form a ternary complex in association with O/P region and MerR to co-regulate the expression of mer operon (Champier et al., 2004). Following exposure to ionic Hg2+, the toxic metal firstly binds to two cysteine residues at positions 14 and 17 of MerP (Steele and Opella, 1997), which directly transfers the Hg2+ to the mercury-specific transporter MerT (Schue et al., 2008). MerT transfers Hg2+ from its two cysteine residues located on the periplasmic side of the membrane to two cysteine residues located on the cytosolic side (Morby et al., 1995). Once bound on the cytosolic side of MerT, the Hg2+ is transferred directly to two cysteine residues within the amino-terminal domain of MerA (NMerA) (Schue et al., 2008) which then transfers the Hg2+ to two cysteine residues in the active site of MerA for NAD(P)H-dependent reduction to Hg0 (Hong et al., 2014). The mercuric reductase MerA is the central enzyme in the microbial mercury detoxification system and merA is a suitable biomarker for examining the functional diversity of Hg detoxification (Boyd and Barkay, 2012). The merE gene is a predicted small ORF immediately following merD in many Gram-negative mer operon sequences, and it is proposed to encode a transporter of Hg2+ or methylmercury (Sone et al., 2017). MerF could transport ionic mercury from the periplasmic protein MerP across the bilayer to MerA and it plays a similar function as that of MerT (Barkay et al., 2003). However, the other functions of MerF toward mercury resistance are still unclear given that MerT is considered as the main transporter of mercury (Wahba et al., 2017).
Despite being the most studied bacterial toxic metal resistance loci, significant issues remain unknown to elucidate the genetic and physiological adaptations of bacteria to mercury stress, such as the relationship between each gene in the mer operon and bacterial physiology toward mercury resistance. While the role of the mer operon is well understood in terrestrial bacteria, however, it is not conclusive how mer works to cope with mercury stress in marine bacteria (Barkay et al., 2003). Notably, increasingly serious mercuric pollution in the ocean not only leads to food chain contamination, but also hastens ocean acidification (Wang et al., 2017). So, we must quickly develop cost-effective, sustainable and environmentally friendly remediation methods that facilitate the removal of mercury from contaminated ocean sites (Sone et al., 2013). But, we can only develop novel bioremediation strategies with a comprehensive understanding how mer systems function in response to the ever-increasing challenges of co-existing with mercury.
In this study, we show that the mercury resistant marine bacterium P. stutzeri 273 contains a mer gene cluster consisting of putative regulatory proteins (MerR, MerD), transporters (MerT, MerP, MerE, and MerF) and the mercuric reductase (MerA). Using genetic and transcriptomic assays, we demonstrate that merT, merA, merP, merD, and merR confer mercury resistance in P. stutzeri 273. Importantly, we identify novel functions of MerF to promote bacterial mercury resistance, such as the determination of flagellar development, motility, chemotaxis and biofilm formation. Finally, we propose a model for the mercury-adapted lifestyle of the bacterium P. stutzeri 273.
Materials and Methods
Bacterial Strains and Media
Pseudomonas stutzeri 273 was isolated from the sediment samples collected by RV KEXUE during a cruise in the East China Sea in the year of 2014 (Wu et al., 2016). P. stutzeri 273 and its mutants were cultured in marine broth 2216E (5 g/L tryptone, 1 g/L yeast extract, one liter filtered seawater, pH adjusted to 7.4–7.6) or Luria Bertani (LB) medium (10 g/L peptone, 5 g/L yeast extract, 10 g/L NaCl, pH adjusted to 7.0) and incubated at 28°C under vigorous agitation at speed of 150 rpm. Escherichia coli DH5α was used as the host for plasmid construction, and E. coli S17-1 was used as a vector donor in conjugation. E. coli DH5α, E. coli SY327, and E. coli S17-1 were grown in LB medium at 37°C with shaking at speed of 150 rpm. When necessary, antibiotics were used at the following concentrations: chloramphenicol (Cm) and gentamicin (Gm) with a final concentration of 25 and 25 μg/mL, respectively (Wu et al., 2017).
Bioinformatic Analysis
The sequences of genes within the mer gene cluster of P. stutzeri 273, MerD and MerF protein sequences of other bacteria used in our study were obtained from GenBank. All of the bacterial MerF sequences were aligned using ClustalW2 (Larkin et al., 2007), and the phylogenetic tree was constructed with MEGA6.0 (Tamura et al., 2013). The complete genome sequence of P. stutzeri 273 has been deposited at GenBank under the accession number CP015641, and all the genome information of P. stutzeri 273 available in GenBank.
Determination of Mercury Minimal Inhibitory Concentrations (MIC), Growth Curves and Mercury Removal Rate in P. stutzeri 273 or P. aeruginosa PAO1
To determine the mercury MIC against P. stutzeri 273, fifty-microliter of mid-log-phase cultures was inoculated in 5 mL LB medium containing 0, 20, 40, 60, 80, 100, and 120 μM HgCl2, respectively. Cultures were incubated at 28°C with the speed of 150 rpm for 24 h for each strain tested until confluent growth was observed in control (no HgCl2) tubes, followed by examination of growth on the HgCl2-supplemented groups. The MIC value was the lowest concentration of Hg2+ at which growth in LB medium was inhibited (Vetriani et al., 2005). Each treatment was performed in triplicate and the MIC was defined as the average number of three repeats. To determine the mercury MIC against P. aeruginosa PAO1, fifty-microliter of mid-log-phase cultures was inoculated in 5 mL LB medium containing 0, 0.1, 0.2, 0.3, 0.4, 0.5, 0.6, 0.7, 0.8, 0.9, and 1.0 μM HgCl2, respectively. Other operations were performed as described above. To check the growth of P. stutzeri 273 under HgCl2 stress, 1 mL overnight bacterial culture was inoculated in 100 mL flask at 28°C with the speed at 150 rpm in LB medium in the absence or presence of 20 μM or 50 μM HgCl2. Bacterial growth status was monitored by measuring the OD600 value every 4 h until cell growth reached stationary phase. To determine the Hg2+ removal rate of P. stutzeri 273, P. stutzeri 273 was incubated at 28°C with speed of 150 rpm in LB medium supplemented with 20 μM or 50 μM HgCl2 to OD600 value of 1.5. The supernatant of culture was collected by centrifugation (13,400 × g, 2 min). After which, the supernatant was thoroughly digested with nitric acid and perchloric acid, and diluted with Milli-Q water for Hg2+ concentration detection. The dissolved Hg2+ concentrations were measured with an inductively coupled plasma source mass spectrometer (Optima 7300 DV, PerkinElmer) (Han et al., 2014).
Construction of Deletion Mutants of Genes Within mer Gene Cluster and Complementation of Mutants for Mercury Sensitivity Assays
The P. stutzeri 273 derivatives (ΔmerA, ΔmerP, ΔmerR, ΔmerT, ΔmerD, ΔmerE, ΔmerF, and ΔfliC) were constructed by allelic exchange as previously described (Wu et al., 2017). Briefly, fragments for mutant construction were amplified from the chromosome of P. stutzeri 273 by primers shown in Supplementary Table S1. Then, PCR fragments were purified, digested and ligated into the suicide vector pEX18Gm containing an oriT for conjugation. The resulting plasmid was transformed successively into E. coli SY327 and E. coli S17-1 by the CaCl2 method. Mating between P. stutzeri 273 and E. coli S17-1 containing different plasmids was performed at 30°C for 24 h. Colonies growing on LB agar amended with Cm (25 μg/mL) and Gm (25 μg/mL) were single-event positive recombinant strains. The individual colony was picked and incubated overnight at 30°C with shaking in LB broth with Cm (25 μg/mL) and Gm (25 μg/mL), then diluted 1:1000 into fresh LB broth and plated onto LB agar plate amended with 10% sucrose and incubated for 48 h at 30°C. A single colony was re-streaked several times before being replicated onto a LB agar plate amended with Gm (25 μg/mL) to confirm sensitivity to gentamicin and loss of the pEX18GM vector. All double recombination mutants candidates were verified by PCR amplification and sequencing.
To construct complementary strains, pUCP18 was used as the mother plasmid, and E. coli DH5a was used as the host strain. Briefly, genes (merT, merP, merF, merA, and merD) derived from P. stutzeri 273 together with their native promoters were amplified from the wild-type strain by primers listed in Supplementary Table S2. Then, the corresponding PCR products were digested with HindIII and BamHI and ligated into pUCP18 to produce pUCP18-merT, pUCP18-merP, pUCP18-meF, pUCP18-merA, and pUCP18-merD, respectively. The above resulting plasmids were separately transformed into the corresponding mutants ΔmerT, ΔmerP, ΔmerF, ΔmerA, and ΔmerD with the CaCl2 method (Lee et al., 2013; Weller-Stuart et al., 2017). The final complementary strains (ΔmerT/cmerT, ΔmerP/cmerP, ΔmerF/cmerF, ΔmerA/cmerA, and ΔmerD/cmerD) were verified by PCR amplification and sequencing.
Mercury sensitivity assays of P. stutzeri 273 wild type, different mutants and the corresponding complementations were performed on LB agar plates with different concentrations of HgCl2. Cells were grown to the exponential phase in LB liquid medium and then diluted to an OD600 of 0.1. Five 10-fold dilutions were carried out and spotted on agar plates incubated at 28°C for 48 h. Each experiment was repeated three times.
Motility, Chemotaxis and Biofilm Formation Assays
For the motility assay, P. stutzeri 273 and correlative mutants were cultured in LB medium at 28°C with speed of 150 rpm to the OD600 of 1.2. Then 20 μL different culture was inoculated onto the center of 1% agar plate without or with 20 or 50 μM HgCl2 and incubated for 5 days at 28°C (Radin et al., 2013). For the chemotaxis assay, wild type P. stutzeri 273 was cultured in LB medium at 28°C with speed of 150 rpm to the OD600 of 1.2. Then 20 μL culture was inoculated onto the center of 1% agar plate, and the filter paper containing 20 μL water or 20 or 50 μM HgCl2 was loaded 2-cm away from the culture spot. Plates were incubated for 5 days at 28°C for further examination. For the biofilm formation assay, 50 μL of P. stutzeri 273 culture (OD600 about 0.8) was inoculated in a borosilicate tube containing 1 mL liquid LB medium without or with 20 and 50 μM HgCl2, respectively. The tubes were incubated at 28°C without shaking for 3 days for further biofilm assays. To quantify the amount of biofilm, the liquid LB medium and planktonic cells were removed from the borosilicate tubes, and the remaining biofilms were washed off with phosphate-buffered saline (PBS, pH 7.4) and stained with crystal violet. The stained biofilms were eluted in 100% ethanol and monitored for biofilm formation as determined by spectrophotometry at 595 nm (Lee et al., 2013). The biofilm formation assays for P. stutzeri 273 ΔmerF and its complement were performed as described above.
Western Blotting
Bacterial lysates of wild type P. stutzeri 273 and merF deletion mutant were harvested and lysed in the sample buffer containing sodium dodecyl sulphate (SDS) and β-mercaptoethanol, then boiled for 10 min at 100°C. Same amount proteins from wild type and mutant were separated on 12% SDS-page gels, electro-transferred to nitrocellulose membranes and incubated with FliC primary antibody (BioLegend) and secondary antibody (Proteintech Group).
Quantitative Reverse Transcription-PCR (qRT-PCR)
For qRT-PCR, cells of P. stutzeri 273 or corresponding mutants incubated in LB medium amended without or with 20 or 50 μM Hg2+ to OD600 of 1.2. Cells were centrifuged at 6,000 × g for 10 min, total RNAs were extracted using the RNApure Bacteria Kit (DNase I) (CWBio, China). Total RNAs were reverse transcribed into cDNA, and the transcriptional levels of different genes were determined by qRT-PCR with Sybr Green Premix Low rox (MDbio, China) and the QuantStudioTM 6 Flex (Thermo Fisher Scientific, United States). RNA degradation was examined on 1% agarose gels. RNA purity was verified with NanoPhotometer R spectrophotometer (IMPLEN, Westlake Village, CA, United States). RNA concentration was determined by QubitR RNA Assay Kit in QubitR 2.0 Fluorometer (Life Technologies, Carlsbad, CA, United States). RNA integrity was assessed by RNA Nano6000 Assay Kit for the Bioanalyzer 2100 system (Agilent Technologies, Santa Clara, CA, United States). 16S rDNA was used as an internal reference. The relative gene expression was calculated using the 2-ΔΔCt method with each transcript signal normalized to 16S rDNA (Petrus et al., 2015; Zhu et al., 2017). Transcript signals for each treatment were compared to the transcript signals from the control group. Specific primers for the genes within mer gene cluster and 16S rDNA were designed using Primer 5.0 as shown in Supplementary Table S3. All qRT-PCR runs were conducted with three biological and three technical replicates.
Transcriptional Profiling of P. stutzeri 273 Challenged With Different Concentrations of Hg2+
Total RNAs of P. stutzeri 273 incubated in LB medium in the absence or presence of 20 or 50 μM of HgCl2 with OD600 about 1.2 were extracted and checked as described above. Detailed protocols of RNA-seq are described in the Supplementary Information. qRT-PCR verification of transcriptional profiling data was performed as described above. Five genes (PS273GM-RS08590, flgG; PS273GM-RS08570, flgC; PS273GM-RS08525, motA; PS273GM-RS02905, cheY; PS273GM-RS08565, flgB) were chosen for verification of transcriptional profiling data by qRT-PCR. The specific primers were listed in Supplementary Table S3. The heat map was made by HemI 1.0 based on the KEGG enrichment results.
Transmission Electron Microscopy (TEM)
To examine the flagella formation, wild-type and relative mutants of P. stutzeri 273 were all examined using TEM with a JEOL JEM 12000 EX (equipped with a field emission gun) at 100 kV. The cell suspension was washed with sterile nutrient solution or Milli-Q water and centrifuged at 5,000 × g for 3 min. Finally, these samples were taken by immersing copper grids coated with a carbon film for 1 min in bacterial suspensions and washed for several minutes in distilled water and dried several minutes at room temperature (Bundeleva et al., 2014; Han et al., 2014).
Statistical Analysis
Tests for significance of the differences among groups were subjected to one-way analysis of variance (one-way ANOVA) and multiple comparisons by using the GraphPad Prism 5. Statistical significance was defined in our study by P < 0.05 (indicated by∗ in all figures), P < 0.01 (indicated by ∗∗ in all figures) or P < 0.001 (indicated by ∗∗∗ in all figures).
Results
Characterization of Mercury Resistance Conferred by the mer Gene Cluster in P. stutzeri 273
Pseudomonas stutzeri 273 has its entire genome sequenced (NCBI accession number CP015641) (Wu et al., 2017). When analyzing the genome sequence of this bacterium, a candidate mer gene cluster between nucleotide 2193317 to nucleotide 2196970 was found in the chromosome of P. stutzeri 273. Notably, the mer gene cluster is located on a genomic island flanked by a transposase (WP_046622512.1) and a resolvase (WP_003089068.1) (Figure 1A). This mer gene cluster consists of two potential mercury-responsive regulatory proteins, MerR and MerD, the mercury transporters MerP, MerT, MerE, and MerF, and the mercuric ion reductase MerA, which reduces toxic Hg2+ to non-toxic Hg0 (Table 1). To measure the potential mercury resistance conferred by the mer gene cluster, we examined the resistance of P. stutzeri 273 against Hg2+. Our results showed that Hg2+ has a 60 μM minimum inhibition concentration against P. stutzeri 273 (Figure 1B). Notably, the Hg2+ MIC against P. aeruginosa PAO1 without mer gene cluster in the chromosome is 0.7 μM (Supplementary Figure S1), which is much lower than that of P. stutzeri 273. The bacteria could grow to a similar density in the presence of 20 μM Hg2+ or 50 μM Hg2+ compared to growth with no mercury, however, the time reaching stationary phase is 10 and 20 h later than that without mercury stress, respectively (Figure 1C). We then assessed the mercury removal property of P. stutzeri 273 by measuring metal depletion in culture supernatants by inductively coupled plasma-optical emission spectroscopy (ICP-OES). We found that up to 94% Hg2+ could be removed in both 20 and 50 μM Hg2+ conditions (Figure 1D). Taken together, these results indicate that P. stutzeri 273 is a good candidate to develop bioremediation products toward mercuric pollution in the marine environment.
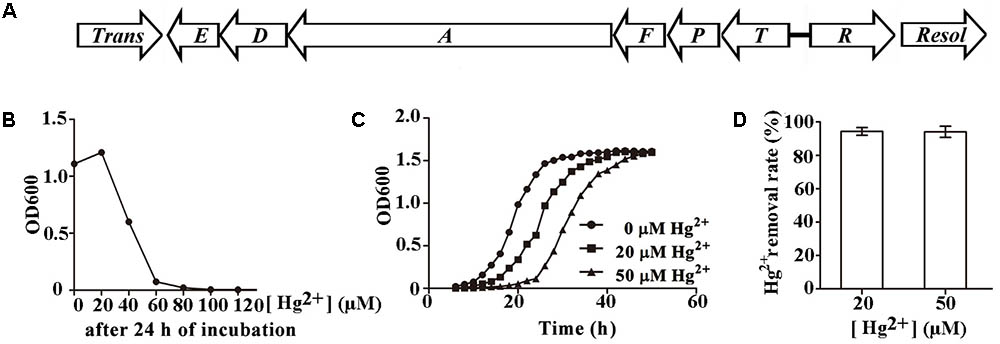
FIGURE 1. Characterization of mercury resistance by Pseudomonas stutzeri 273. (A) Arrangement of potential mer gene cluster in P. stutzeri 273. Trans, transposase (PS273GM_RS09950); E, merE (PS273GM_RS09955); D, merD (PS273GM_RS09960); A, merA (PS273GM_RS09965); F, merF (PS273GM_RS09970); P, merP (PS273GM_RS09975); T, merT (PS273GM_RS09980); R, merR (PS273GM_RS09985); Resol, resolvase (PS273GM_RS09990). Locus tags indicated in the bracket. (B) Hg2+ MIC determination in P. stutzeri 273. (C) Growth style of P. stutzeri 273 in the absence or presence of Hg2+ (20 μM or 50 μM). (D) Hg2+ removal by P. stutzeri 273 with different concentrations of Hg2+.
Genetic and Biochemical Determination of Key Genes in the mer Gene Cluster of P. stutzeri 273 Conferring Mercury Resistance
Mer gene cluster is widely accepted to determine mercurial resistance of most bacteria (Barkay et al., 2003). We sought to elucidate which genes in the mer gene cluster are essential for mercury resistance in P. stutzeri 273. So, we constructed different deletion mutants (ΔmerA, ΔmerP, ΔmerR, ΔmerT, ΔmerD, ΔmerE, ΔmerF) and tested their growth status in LB agar plates supplemented with different concentrations of Hg2+. As expected, the wild type showed normal growth in the concentration of 50 μM Hg2+. However, the mutants with deletion of merA (encoding the mercuric reductase) or merT (encoding the Hg2+-transporter) exhibited the greatest sensitivity to the low concentration of Hg2+, such that the cells could not even grow in the agar plate containing 20 μM Hg2+ (Figure 2A, middle panel). The deletion of merD (encoding the regulatory protein of mer gene cluster) affected bacterial growth to a certain extent in the presence of 20 μM Hg2+ (Figure 2A, middle panel), but cellular growth was completely inhibited by 50 μM Hg2+ (Figure 2A, right panel). The deletion of merP (encoding the Hg2+-transporter) only affected bacterial growth under the 50 μM Hg2+ condition but not the low level Hg2+ condition (Figure 2A, middle and right panels). On the other hand, deletions of merR (encoding the regulatory protein of mer gene cluster), merE and merF (encoding Hg2+-transporter) did not affect the mercury resistance of P. stutzeri 273, as the cells grew as well as the wild type in the presence of 50 μM Hg2+ (Figure 2A, right panel). To better understand how merA, merP, merT, and merD confer mercury resistance to P. stutzeri 273, we constructed complements (ΔmerA/cmerA, ΔmerP/cmerP, ΔmerT/cmerT, and ΔmerD/cmerD) of the above deletion strains and examined their growth in the presence of both low (20 μM) and high (50 μM) levels of Hg2+. As expected, merA, merP, merT, or merD could complement the mercury sensitive phenotype of corresponding P. stutzeri 273 gene deletion strain (Figure 2B). These results clearly demonstrate that merA, merP, merT, and merD determine mercury resistance in P. stutzeri 273.
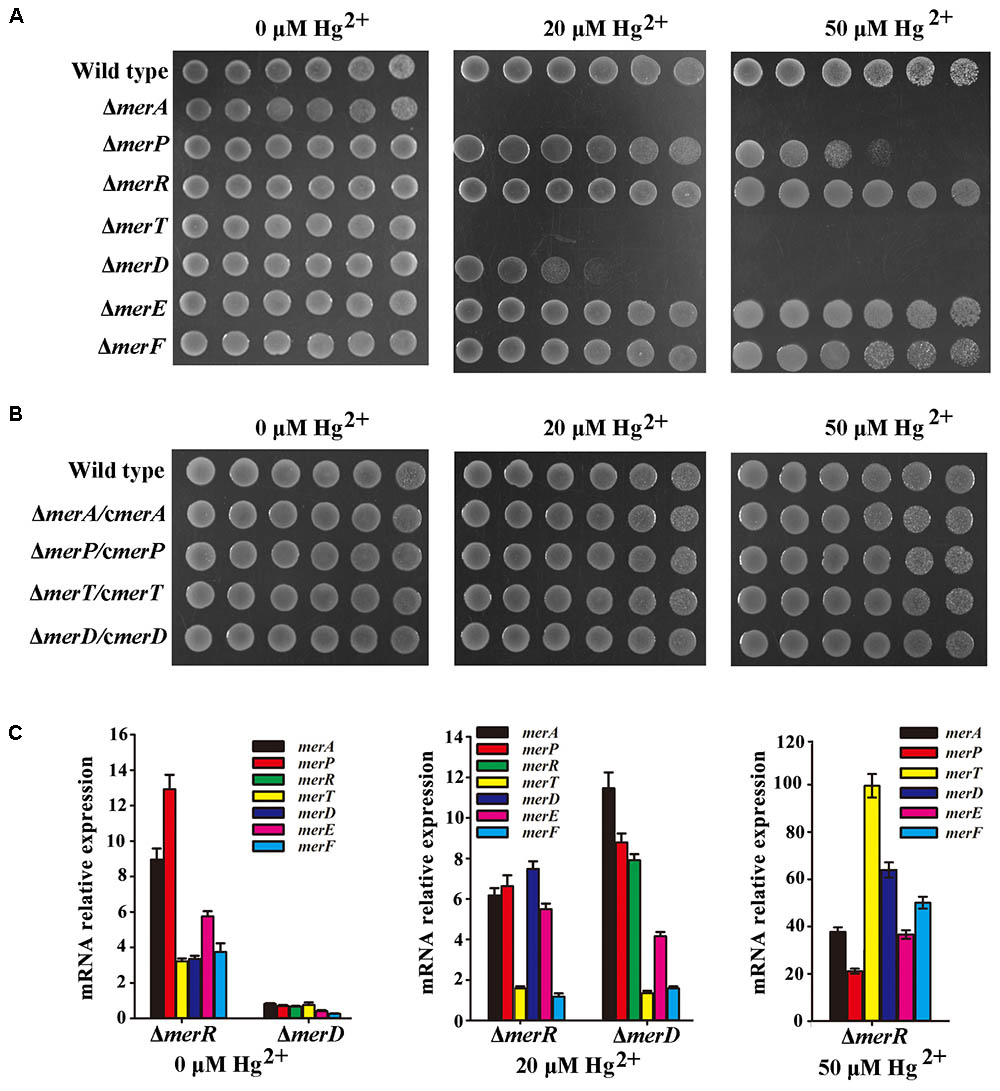
FIGURE 2. Genetic and biochemical analyses of mer gene cluster function for mercury resistance in P. stutzeri 273. (A) Growth in LB solid media of wild type, mutant strains (ΔmerA, ΔmerP, ΔmerR, ΔmerT, ΔmerD, ΔmerE, and ΔmerF) and (B) complemented strains (ΔmerA/cΔmerA, ΔmerP/cΔmerP, ΔmerT/cΔmerT, and ΔmerD/cΔmerD) of P. stutzeri 273. Five 10-fold dilutions spotted from left to right with the indicated concentration of Hg2+. (C) Expression of each gene in the mer gene cluster of P. stutzeri 273 mutant with merR or merD deletion without Hg2+ (left panel), in the presence of 20 μM Hg2+ (middle panel). Expression of each gene in the mer gene cluster of P. stutzeri 273 mutant with merR deletion in the presence of 50 μM Hg2+ (right panel).
The metalloregulatory protein MerR acts as both a repressor and an activator of the transcription of the mer operon depending on the absence or presence of mercuric ions, respectively (Champier et al., 2004). MerR is the predominant regulator for mercury resistance, however, the function of the other potential regulator MerD remains speculative. To further clarify the function of MerD and MerR for mercury resistance in P. stutzeri 273, we detected the expression of other mer genes in the mutant ΔmerR or ΔmerD of P. stutzeri 273 by qRT-PCR in the presence of different concentrations of Hg2+. Interestingly, in the absence of Hg2+, the expression of all mer genes was up-regulated in the merR deletion mutant (Figure 2C, left panel), which indicates that merR is a repressor in P. stutzeri 273 in the absence of Hg2+. However, the expression of all mer genes was down-regulated (Figure 2C, left panel) in the merD deletion mutant, which indicates that merD is an activator in P. stutzeri 273 in the absence of Hg2+. When challenged with 20 μM Hg2+, in the merR or merD deletion mutant, the expression of most mer genes was up-regulated from 4 to 12 times except for merT and merF, whose expression almost kept the same level when challenged with 20 μM Hg2+ (Figure 2C, middle panel). Notably, the expression of merD or merR was up-regulated about eight times in the merR or merD deletion mutant (Figure 2C, middle panel), which suggests that MerD or MerR might regulate the expression of the mer gene cluster when the other is absent. In the presence of 50 μM Hg2+, the growth of the merR deletion mutant was completely inhibited, however, the merR deletion mutant grew very well with the expression of merD increased more than 60-fold (Figure 2C, right panel). Moreover, the expression of all other mer genes in merR deletion mutant was up-regulated from 20 to 100 times in the presence of 50 μM Hg2+ (Figure 2C, right panel). Together with the results of gene deletion and complement shown in Figures 2A,B, we propose that MerR is a repressor and MerD is an activator in the absence of Hg2+, MerD acts as an activator in both low (20 μM) and high (50 μM) concentrations of Hg2+, and MerR is only functional as an activator in the presence of low (20 μM) but not high (50 μM) concentration of Hg2+.
Transcriptome Profiles of P. stutzeri 273 Challenged With Different Concentrations of Hg2+
Mercury and its compounds exert inhibitory effects on the functioning of bacterial enzymes and proteins and render them useless. However, bacterial genetic and morphological flexibility along with immense physiological variability enable them to survive in extreme environmental conditions (Mathema et al., 2011). To reveal the overall responses when exposed to mercury stress, we performed transcriptome analyses of P. stutzeri 273 challenged with different concentrations of Hg2+. As expected, expression of merT, merA, merD, and merP was significantly up-regulated with increasing Hg2+ concentrations (Figure 3A). These results are consistent with the genetic results (Figure 2) and confirm the importance of these genes to confer mercury resistance in P. stutzeri 273. KEGG enrichment analysis showed that flagellar assembly, bacterial chemotaxis and two-component system were enriched among the two-way comparison of Hg0 (absence of Hg2+), Hg20 (presence of 20 μM Hg2+), and Hg50 (presence of 50 μM Hg2+) (Figure 3B). It is noteworthy that all of the genes expressed related to these three pathways were significantly down-regulated with increasing concentrations of Hg2+ (Figures 3C–E). There are 18 differentially expressed genes (DEGs), 15 DEGs or 24 DEGs under the terms “flagellar assembly” (Figure 3C), “bacterial chemotaxis” (Figure 3D) and “two-component system involved in the regulation of flagella and chemotaxis” (Figure 3E), respectively. Down-regulation of chemotaxis and two-component system implies that signal transduction of P. stutzeri 273 was strictly controlled in response to mercury stress as described previously (Liu et al., 2015). Notably, many down-regulated genes overlapped with different terms, such as PS273GM_RS08465 and PS273GM_RS08525 were classified as both “flagellar assembly” and “bacterial chemotaxis,” and PS273GM_RS08525 belongs to all three pathways, which strongly suggests that these three pathways are closely correlated. We then verified the reliability of the transcriptomic data by qRT-PCR analysis. In total, we selected five genes (flgG, fliC, motA, cheY, and flgB) that belong to “flagellar assembly,” “bacterial chemotaxis” or “two-component system” for validation. We consistently observed similar trends between qRT-PCR and RNA-seq results, which support the validity of our RNA-seq data (Supplementary Figure S2).
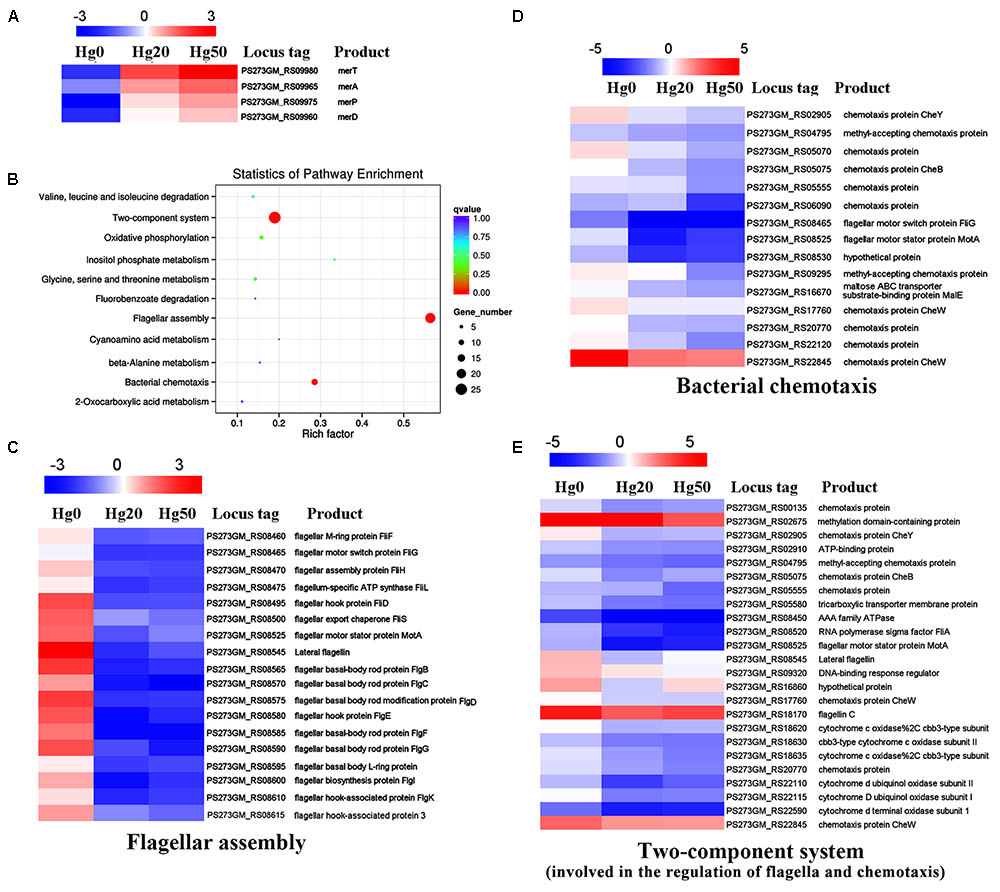
FIGURE 3. Transcriptome profiles of P. stutzeri 273 challenged with different Hg2+ concentrations. (A) Heat map shows relatively up-regulated and differentially expressed Hg2+ stress-responsive genes (DEGs) within mer gene cluster in P. stutzeri 273 among Hg0, Hg20, and Hg50 enriched by KEGG analysis. (B) DEGs enriched KEGG pathway scatterplot of P. stutzeri 273 challenged with different Hg2+ concentrations. The Rich factor is the ratio of differentially expressed gene numbers annotated in this pathway term to all gene numbers annotated in this pathway term. The greater the Rich factor, the greater the degree of pathway enrichment. A Q-value is the corrected p-value ranging from 0 to 1, and a lower value indicates greater pathway enrichment. Heat map shows relatively down-regulated Hg2+ stress responsive DEGs that contribute to flagellar assembly (C), bacterial chemotaxis (D) and two-component system involved in the regulation of flagella and chemotaxis (E) among Hg0, Hg20, and Hg50 enriched by KEGG analysis. The locus tag and its corresponding encoding product shown with the heat map. Heat map made using Heml 1.0.3.3.
Different Mercury Concentrations on Physiological Development in P. stutzeri 273
Because many DEGs involved in “bacterial chemotaxis” or “two-component system” are associated with flagellar formation, we examined the effects of different mercury concentrations on flagella formation in P. stutzeri 273 by TEM. Consistent with our transcriptome profile results, flagellar formation in P. stutzeri 273 was dramatically inhibited with increased Hg2+ concentration (Figure 4A). In the condition without mercury stress, we could observe one intact polar flagellum in all cells (Figure 4A, left panel). However, most cells formed only abnormal flagella when exposed to Hg2+, and the flagellar abnormal ratio and severity were Hg2+-concentration dependent (Figure 4A, middle and right panels). So, we then examined the protein expression of flagellin (FliC), the typical symbol protein for detecting flagella formation, using western blot (Ammendola et al., 2016). The results showed that FliC expression gradually decreased with increased Hg2+ concentration (Figure 4B). These results confirm that Hg2+ affects bacterial flagella development as shown in Figure 4A. Because flagella formation is closely related to bacterial motility, chemotaxis and biofilm formation (Stelmack et al., 1999; Kearns, 2010), we then examined these features of P. stutzeri 273 in the absence or presence of Hg2+. P. stutzeri 273 is a strong motile bacterium, and it swarms in 0.5–1.0% agar plate with a similar speed (Supplementary Figure S3). However, cell motility was significantly inhibited when amended with 20 μM Hg2+. This inhibition became more evident when the concentration of Hg2+ increased to 50 μM (Figure 4C, row a). The bacterial chemotaxis system, which helps bacteria find optimal conditions for growth and survival, decreased toward the Hg2+ gradient (Figure 4C, row b). Consistent with the result of Figure 4B, the bacteria totally lost the motility ability when removing fliC from the chromosome regardless the absence or presence of mercury stress (Figure 4C, row c). Considering the close relationship among bacterial flagellar formation, motility, chemotaxis, and biofilm formation (Dressaire et al., 2015), we examined biofilm formation of P. stutzeri 273 when exposed to Hg2+ environment. As expected, the biofilm formation of P. stutzeri 273 was dramatically inhibited in the presence of 20 μM or 50 μM Hg2+ (Figure 4D). This result indicates that P. stutzeri 273 physiologically adapted to the mercury stress by down-regulating motility and positive chemotaxis.
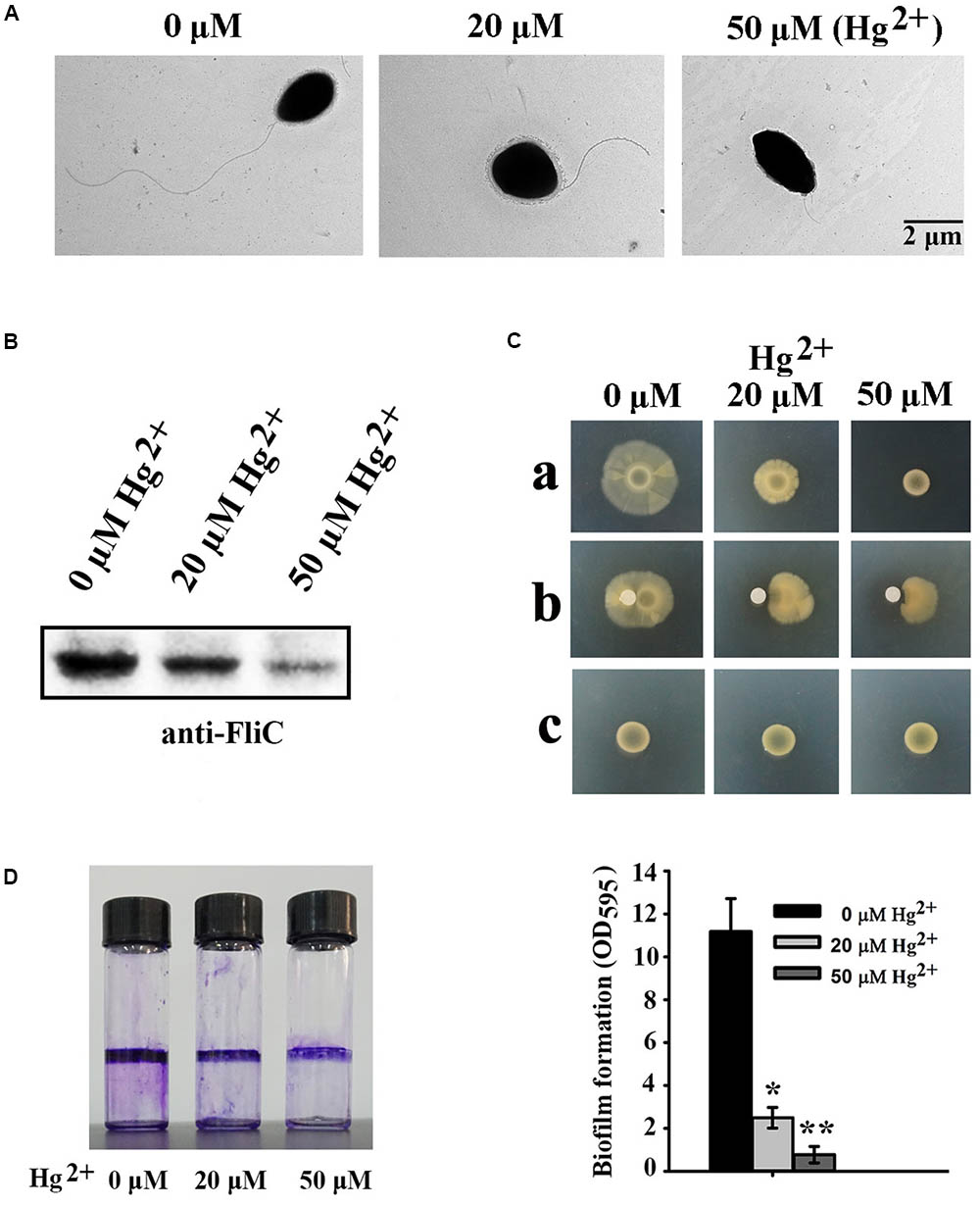
FIGURE 4. Effects of different Hg2+ concentrations on P. stutzeri 273 physiology. (A) Effects of different Hg2+ concentrations on flagella formation in P. stutzeri 273 analyzed by TEM. Representative pictures are shown. (B) Western blot analysis of protein expression of the typical flagellar related gene fliC. (C) Effects of different concentrations of Hg2+ on motility (row a) and chemotaxis (row b) in P. stutzeri 273. Motility assays of fliC deletion mutant of P. stutzeri 273 in the absence or presence of Hg2+ (row c). For the motility assay, the medium contained different Hg2+ concentrations. The chemotaxis assay loaded different Hg2+ concentrations on the filter paper. (D) Effects of different Hg2+ concentrations on biofilm formation in P. stutzeri 273 analyzed by crystal violet staining (left panel). Quantification shown in right panel.
MerF Determines Flagellar Formation and Motility of P. stutzeri 273
Mer operon plays key roles in mercury resistance. But, our results suggest that mercury stress correlates with bacterial physiology. We next examined the possible relationship between mer genes and bacterial physiological development. First, we checked the morphology of wild type and mer-related gene deletion mutants of P. stutzeri 273 via TEM. Surprisingly, the mutant with merF deletion (ΔmerF) could not form flagella compared with wild type and other mutants (Figure 5A). The mutant ΔmerF completely lost motile capability on the agar plate, while wild type and other mutants showed normal motile phenotype (Figure 5B). To further elucidate these novel functions of MerF, we constructed a complement of ΔmerF (ΔmerF/cmerF). We detected bacterial motility and flagella/biofilm formation. As expected, the complementation of merF restored the capabilities of motility (Figure 6A), flagella formation (Figures 6B,C) and biofilm formation (Figure 6D). These results demonstrate that MerF is a key factor to determine flagella formation in P. stutzeri 273.
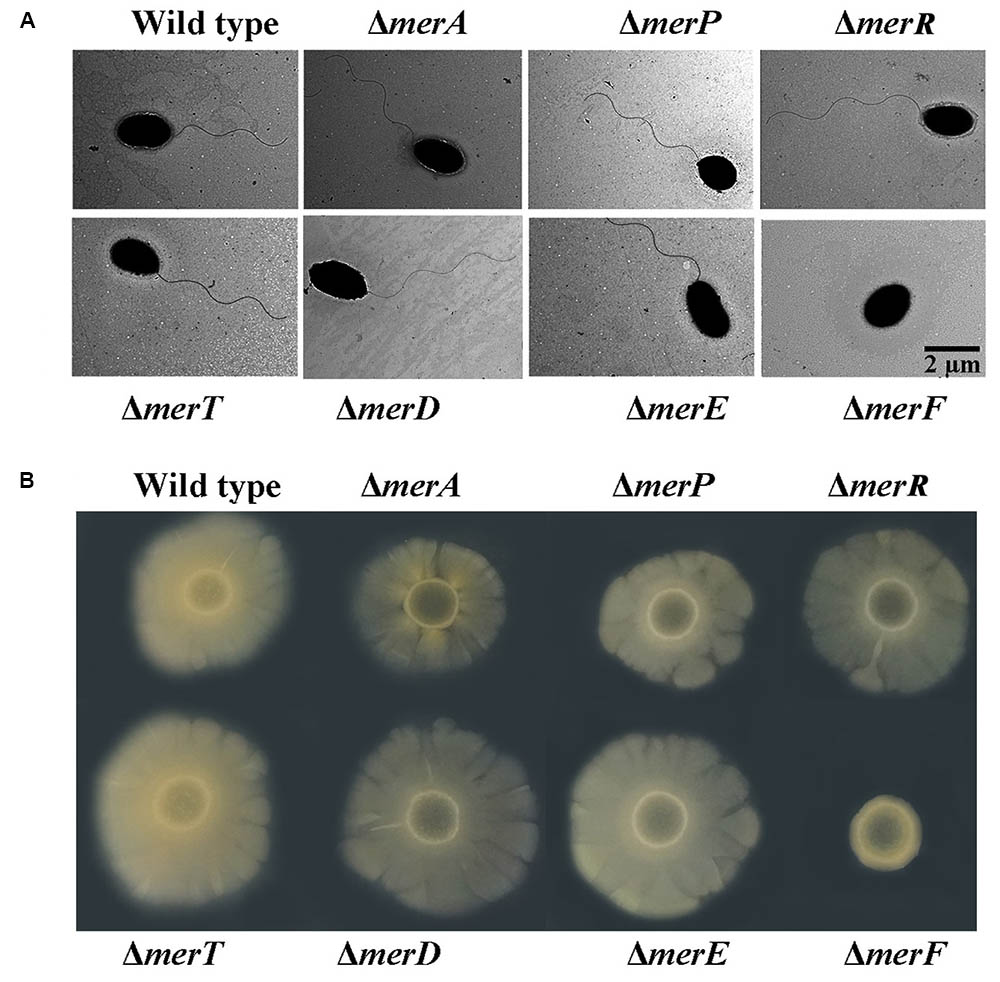
FIGURE 5. Morphology and motility assays in wild type and different deletion mutants of genes within the mer gene cluster. (A) Flagellar formation in P. stutzeri 273 wild type and mutants (ΔmerA, ΔmerP, ΔmerR, ΔmerT, ΔmerD, ΔmerE, and ΔmerF) by TEM. (B) Motility assays of P. stutzeri 273 wild type and mutants (ΔmerA, ΔmerP, ΔmerR, ΔmerT, ΔmerD, ΔmerE, and ΔmerF) on agar plate.
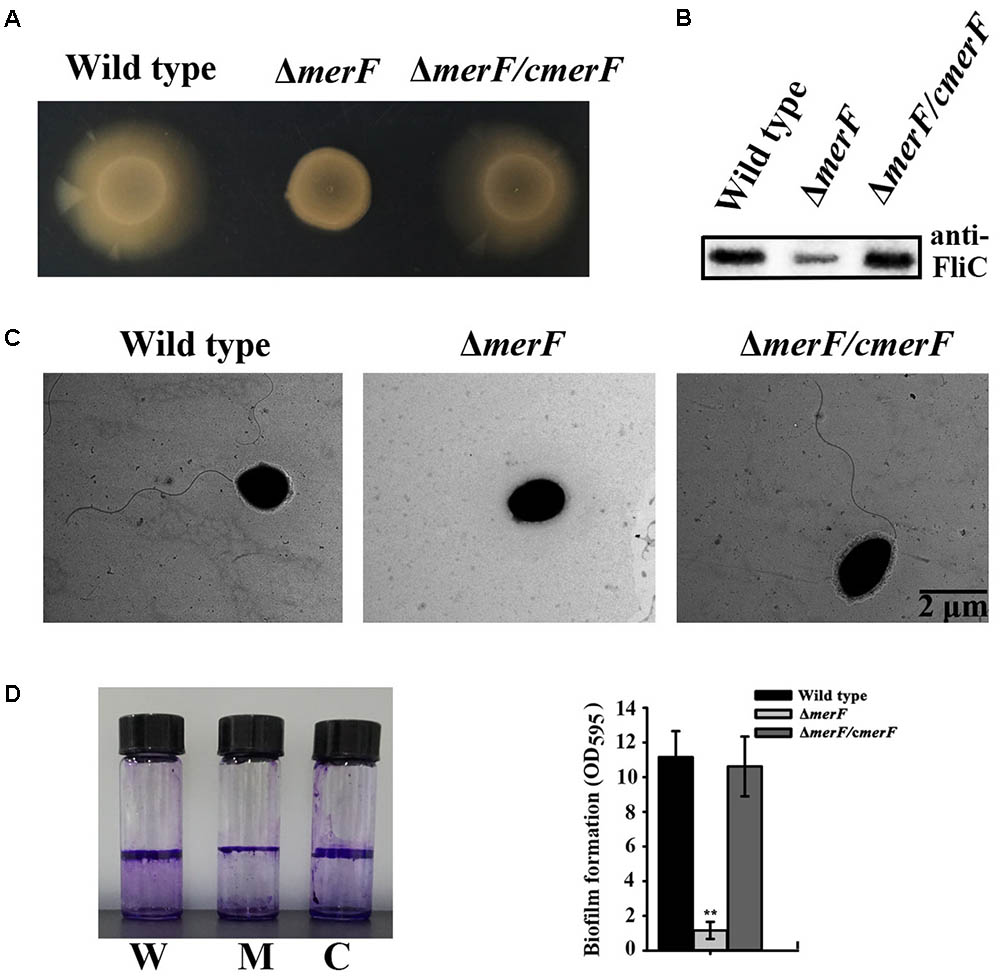
FIGURE 6. MerF determines flagellar formation and motility of P. stutzeri 273. (A) Motility assays of P. stutzeri 273 wild type, merF deletion mutant ΔmerF and its complementation strain ΔmerF/cmerF on agar plate. (B) Western blot analysis of FliC protein expression in P. stutzeri 273 wild type, merF deletion mutant ΔmerF and its complementation strain ΔmerF/cmerF. (C) Flagella formation in P. stutzeri 273 wild type, merF deletion mutant ΔmerF and its complementation strain ΔmerF/cmerF. (D) Comparison of biofilm formation in P. stutzeri 273 wild type (labeled with W), merF deletion mutant ΔmerF (labeled with M) and its complementation strain ΔmerF/cmerF (labeled with C) by crystal violet staining (left panel). Quantification shown in right panel.
Considering the important role of MerF for flagella development, motility and biofilm formation, we searched for homologs in the NCBI database. Interestingly, MerF homologs of P. stutzeri 273 exist in many prevalent pathogens, including some bacterial strains belong to P. aeruginosa, Vibrio cholera, Enterobacter cloacae, Vibrio shilonii, and Enterobacter hormaechei (Figure 7A). Moreover, MerF homologs in these other bacterial strains not only show high identity but also share sveral conserved domains with MerF in P. stutzeri 273 (Figure 7B).
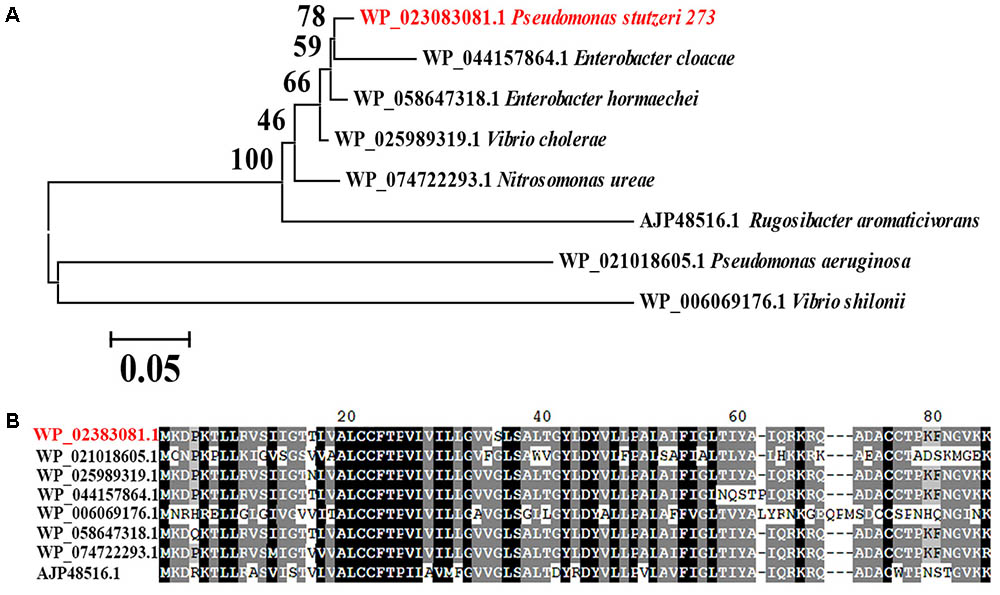
FIGURE 7. Phylogenetic and conservation analyses of MerF. (A) The consensus phylogenetic tree of MerF in P. stutzeri 273 with other related MerF obtained from GenBank (accession numbers are indicated before the species name) constructed by the neighbor-joining method. Numbers above the branches are bootstrap values based on 1000 replicates. (B) Amino acid sequence alignment of MerF of P. stutzeri 273 (WP_023083081.1) with other MerF existing in different pathogenic bacterial strains (WP_021018605.1, Pseudomonas aeruginosa; WP_025989319.1, Vibrio cholera; WP_044157864.1, Enterobacter cloacae; WP_006069176.1, Vibrio shilonii; WP_058647318.1, Enterobacter hormaechei; WP_074722293.1, Nitrosomonas ureae; AJP48516.1, Rugosibacter aromaticivorans).
Discussion
The extremely broad phenotypic and genotypic diversity of P. stutzeri has proven an ideal model system for biochemical characterization of bioremediation and yielded significant advances in this area (Chen et al., 2011). P. stutzeri 273 is a marine bacterium isolated from sediments of the East China Sea, an environment existing different heavy metals (Yuan et al., 2004). Bacteria possess an exceptional ability to adapt to their environment, and their genetic and physiological flexibility enables them to develop a variety of survival mechanisms (Mathema et al., 2011). Several gene loci involved in resistance to different heavy metals exist in the genome of P. stutzeri 273, such as mercury, cadmium and copper (Wu et al., 2017). Specifically, P. stutzeri 273 possesses strong mercury resistance and removal capabilities and there is a mer gene cluster located in the chromosome (Figure 1). P. stutzeri 273 also produces exopolysaccharide EPS273, which effectively inhibited both biofilm formation of P. aeruginosa PAO1 and biofouling in the marine environment (Wu et al., 2016). Together with our present discoveries, we deduce that P. stutzeri 273 has evolved different strategies to respond to the harsh conditions and compete with other microbes for survival. Overall, we propose P. stutzeri 273 will be an ideal candidate to study the adaptation and evolution of marine bacteria to the harsh environment.
In most mercury resistance bacteria, MerR tightly controls the expression of the whole mer gene cluster; however, the exact function of the other potential regulatory protein MerD remains unresolved. MerD may function as either an activator (Nucifora et al., 1989) or a repressor of the mer operon (Mukhopadhyay et al., 1991). In P. stutzeri 273, MerR and MerD were demonstrated to be a repressor and an activator in the absence of Hg2+ (Figure 2C, left panel), respectively. MerR functions as an activator only in the presence of low concentration of Hg2+ (such as 20 μM) (Figure 2C, middle panel), however, MerD acts as an activator in both low and high concentrations of Hg2+ (Figure 2C, middle and right panels). In the presence of mercury stress, MerR and MerD might cooperate to regulate the expression of the mer gene cluster as described previously (Champier et al., 2004). We are still not clear the exact action mechanisms of MerR and MerD, which need further elucidation in the future.
Bacteria maintain an uneasy relationship with metal ions and alter their physiological behavior to minimize or nullify the toxicity of metals when residing in such regions or encountering such conditions (Singh et al., 2014). Bacteria can sense metal stress through a receptor and signal(s) using two-component regulatory systems and transmitting information to flagellar motors, which move them in the required direction by the chemotaxis system (Bren and Eisenbach, 2000). Here, we consistently demonstrated using transcriptional profiles that most genes involved in bacterial flagellar assembly, chemotaxis and corresponding two-component system of P. stutzeri 273 were dramatically down-regulated when challenged with 50 μM Hg2+ (Figures 3B–D). Correspondingly, Hg2+ significantly altered bacterial physiology development including flagella formation, motility, chemotaxis, and biofilm formation (Figure 4).
The question remains why bacteria undergo such physiological responses under mercuric stress. Many metals have no known beneficial function and can be quite toxic, even at low levels. Given our experimental conditions in the presence of Hg2+, P. stutzeri 273 could only remove mercury near their living microenvironment. However, some amounts of Hg2+ still remained in the media, which the bacterial chemotaxis system could sense. Therefore, the optimal bacterial response will decrease motility to avoid more mercuric stress exposure. So, bacteria down-regulate flagella development to reduce motility under these harsh conditions. This is consistent with our finding that flagellum biosynthesis is inhibited by stressful environmental conditions including metal stress due to flagellum biosynthesis which requires significant cellular resources (Soutourina et al., 2002). The results in this study extend our knowledge of the regulatory mechanisms of the bacterial flagellar system and the complex mechanisms governing bacterial motility in response to heavy metal challenges.
While we understand the transformation of toxic Hg2+ to non-toxic Hg0 mediated by mer genes from a genetic perspective, the roles of mer genes playing in bacterial physiological development to cope with the mercuric stress remain elusive. Surprisingly, merF deletion from the chromosome of P. stutzeri 273 led to defective flagella formation (Figure 5A) and decreased swarming motility (Figure 5B) and biofilm formation (Figure 6D), while merF complement could restore these disabled functions (Figures 6A–D). MerF was reported to have 81 residues, two transmembrane helices and 20% sequence identity with two-thirds of the N-terminal of MerT (116 residues and three transmembrane helices) (Wilson et al., 2000). Previous studies propose that MerF is a mercuric ion transport protein possessing similar functions as MerT and MerC (Wilson et al., 2000). Thus, our findings discovered the connection between the mer related gene and bacterial flagella formation. MerF exists in many strains of pathogens, including P. aeruginosa, Vibrio cholera, and Enterobacter cloacae. This finding strongly suggests that MerF homologs in these pathogens may also regulate flagella and biofilm formation. Due to the importance of flagella formation, motility and biofilm formation in pathogenic infection (Chaban et al., 2015; Al-Wrafy et al., 2017), our study provides novel candidates to target some pathogen containing merF. Based on the novel MerF function we identified, we hypothesize that mer genes have gradually increased their gene complements and functional diversity. We further propose that mer evolution has progressed by sequential recruitment of novel functions over evolutionary time (Barkay et al., 2003).
Taken together, we propose a model for the mercury-adapted lifestyle of the marine bacterium P. stutzeri 273 which adopts both passive and active strategies to respond to mercury stress (Figure 8). We also propose that the mer gene cluster in P. stutzeri 273 is representative of that found in the genomes of bacteria living in marine environment contaminated by mercury, and this bacterium might be a good candidate to understand bacterial adaptation mechanisms to mercury stress and mer genes evolution in marine microbes.
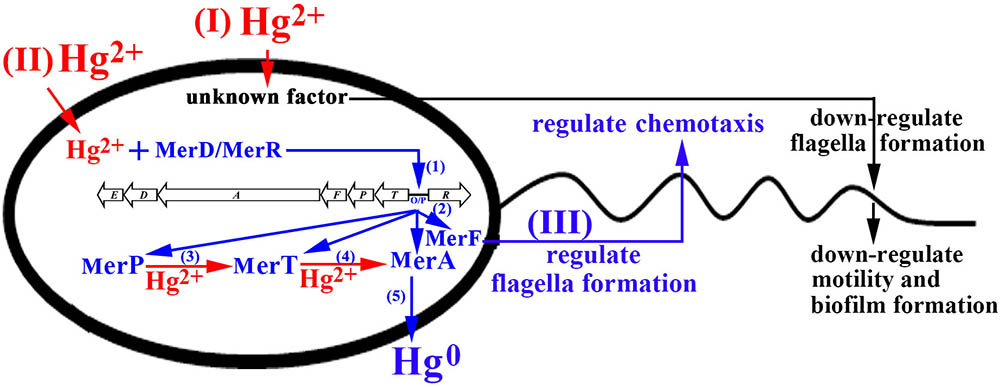
FIGURE 8. Proposed model of genetic and physiological adaptations of marine bacterium P. stutzeri 273 to mercury stress. With the functional mer gene cluster in P. stutzeri 273, while sensing toxic levels of Hg2+, an unknown factor begins to down-regulate flagella/biofilm formation to decrease bacterial motility for passive adaptation to mercury stress (I). (II) Meanwhile, Hg2+ enters into the cell and interacts with MerD or MerR to bind mer operator/promoter (O/P) region for activating the expression of the mer gene cluster (step 1); With the activation of the mer gene cluster, MerP/MerT/MerA/MerF are produced in cells (step 2); the Hg2+ existing in the cells binds with MerP and then transfers to MerT (step 3). MerT subsequently transfers Hg2+ to MerA and converts toxic Hg2+ to non-toxic Hg0 (step 4). (III) MerF regulates flagella formation and chemotaxis to promote bacterial motility for positive adaptation to mercury stress.
Author Contributions
RZ and CS conceived and designed the experiments. RZ performed the experiments. RZ, SW, NM, and CS analyzed the data. RZ, SW, and CS wrote the paper.
Funding
This work was supported by Taishan Young Scholar Foundation of Shandong Province (No. tsqn20161051), AoShan Talents Program supported by Qingdao National Laboratory for Marine Science and Technology (No. 2015ASTP), Natural Science Outstanding Youth Fund of Shandong Province (JQ201607), and “100-Talent Project” of the Chinese Academy of Sciences for CS. The plasmid pEX18Gm was kindly provided by Luyan Ma of the Institute of Microbiology, Chinese Academy of Sciences.
Conflict of Interest Statement
The authors declare that the research was conducted in the absence of any commercial or financial relationships that could be construed as a potential conflict of interest.
Acknowledgments
We thank Dr. Brandi Mattson from the professional editing group “Life Science Editors” for the helpful comments on the manuscript.
Supplementary Material
The Supplementary Material for this article can be found online at: https://www.frontiersin.org/articles/10.3389/fmicb.2018.00682/full#supplementary-material
References
Al-Wrafy, F., Brzozowska, E., Gorska, S., and Gamian, A. (2017). Pathogenic factors of Pseudomonas aeruginosa - the role of biofilm in pathogenicity and as a target for phage therapy. Postepy. Hig. Med. Dosw. 71, 78–91. doi: 10.5604/01.3001.0010.3792
Ammendola, S., D’Amico, Y., Chirullo, B., Drumo, R., Civardelli, D., Pasquali, P., et al. (2016). Zinc is required to ensure the expression of flagella and the ability to form biofilms in Salmonella enterica sv Typhimurium. Metallomics 8, 1131–1140. doi: 10.1039/c6mt00108d
Barkay, T., Miller, S. M., and Summers, A. O. (2003). Bacterial mercury resistance from atoms to ecosystems. FEMS Microbiol. Rev. 27, 355–384. doi: 10.1016/S0168-6445(03)00046-9
Boyd, E. S., and Barkay, T. (2012). The mercury resistance operon: from an origin in a geothermal environment to an efficient detoxification machine. Front. Microbiol. 3:349. doi: 10.3389/Fmicb.2012.00349
Bren, A., and Eisenbach, M. (2000). How signals are heard during bacterial chemotaxis: protein-protein interactions in sensory signal propagation. J. Bacteriol. 182, 6865–6873. doi: 10.1128/Jb.182.24.6865-6873.2000
Bundeleva, I. A., Shirokova, L. S., Pokrovsky, O. S., Bénézeth, P., Ménez, B., Gérard, E., et al. (2014). Experimental modeling of calcium carbonate precipitation by cyanobacterium Gloeocapsa sp. Chem. Geol. 374, 44–60. doi: 10.1016/j.chemgeo.2014.03.007
Chaban, B., Hughes, H. V., and Beeby, M. (2015). The flagellum in bacterial pathogens: for motility and a whole lot more. Semin. Cell Dev. Biol. 46, 91–103. doi: 10.1016/j.semcdb.2015.10.032
Champier, L., Duarte, V., Michaud-Soret, I., and Coves, J. (2004). Characterization of the MerD protein from Ralstonia metallidurans CH34: a possible role in bacterial mercury resistance by switching off the induction of the mer operon. Mol. Microbiol. 52, 1475–1485. doi: 10.1111/j.1365-2958.2004.04071.x
Chen, M., Yan, Y. L., Zhang, W., Lu, W., Wang, J., Ping, S. Z., et al. (2011). Complete genome sequence of the type strain Pseudomonas stutzeri CGMCC 1.1803. J. Bacteriol. 193, 6095–6095. doi: 10.1128/Jb.06061-11
Dash, H. R., and Das, S. (2012). Bioremediation of mercury and the importance of bacterial mer genes. Int. Biodeter. Biodegr. 75, 207–213. doi: 10.1016/j.ibiod.2012.07.023
Dietrich, G. J., Dietrich, M., Kowalski, R. K., Dobosz, S., Karol, H., Demianowicz, W., et al. (2010). Exposure of rainbow trout milt to mercury and cadmium alters sperm motility parameters and reproductive success. Aquat. Toxicol. 97, 277–284. doi: 10.1016/j.aquatox.2009.12.010
Dressaire, C., Moreira, R. N., Barahona, S., de Matos, A. P. A., and Arraiano, C. M. (2015). BolA is a transcriptional switch that turns off motility and turns on biofilm development. MBio 6:e02352-14. doi: 10.1128/mBio.02352-14
Gadd, G. M. (2010). Metals, minerals and microbes: geomicrobiology and bioremediation. Microbiology 156, 609–643. doi: 10.1099/mic.0.037143-0
Han, Z., Yan, H., Zhao, H., Zhou, S., Han, M., Meng, X., et al. (2014). Bio-precipitation of calcite with preferential orientation induced by Synechocystis sp. PCC6803. Geomicrobiol. J. 31, 884–899. doi: 10.1080/01490451.2014.907379
Hong, L., Sharp, M. A., Poblete, S., Bieh, R., Zamponi, M., Szekely, N., et al. (2014). Structure and dynamics of a compact state of a multidomain protein, the mercuric ion reductase. Biophys. J. 107, 393–400. doi: 10.1016/j.bpj.2014.06.013
Kearns, D. B. (2010). A field guide to bacterial swarming motility. Nat. Rev. Microbiol. 8, 634–644. doi: 10.1038/nrmicro2405
Kime, D. E. (1995). The effects of pollution on reproduction in fish. Rev. Fish Biol. Fish. 5, 52–95. doi: 10.1007/Bf01103366
Larkin, M. A., Blackshields, G., Brown, N. P., Chenna, R., McGettigan, P. A., McWilliam, H., et al. (2007). Clustal W and clustal X version 2.0. Bioinformatics 23, 2947–2948. doi: 10.1093/bioinformatics/btm404
Lee, K.-J., Kim, J.-A., Hwang, W., Park, S.-J., and Lee, K.-H. (2013). Role of capsular polysaccharide (CPS) in biofilm formation and regulation of CPS production by quorum-sensing in Vibrio vulnificus. Mol. Microbiol. 90, 841–857. doi: 10.1111/mmi.12401
Liu, P. L., Chen, X., Huang, Q. Y., and Chen, W. L. (2015). The role of CzcRS two-component systems in the heavy metal resistance of Pseudomonas putida X4. Int. J. Mol. Sci. 16, 17005–17017. doi: 10.3390/ijms160817005
Mathema, V. B., Thakuri, B. C., and Sillanpaa, M. (2011). Bacterial mer operon-mediated detoxification of mercurial compounds: a short review. Arch. Microbiol. 193, 837–844. doi: 10.1007/s00203-011-0751-4
Morby, A. P., Hobman, J. L., and Brown, N. L. (1995). The role of cysteine residues in the transport of mercuric ions by the Tn501 mert and merp mercury-resistance proteins. Mol. Microbiol. 17, 25–35. doi: 10.1111/j.1365-2958.1995.mmi_17010025.x
Mukhopadhyay, D., Yu, H. R., Nucifora, G., and Misra, T. K. (1991). Purification and functional-characterization of MerD - a coregulator of the mercury resistance operon in Gram-negative bacteria. J. Biol. Chem. 266, 18538–18542.
Nucifora, G., Silver, S., and Misra, T. K. (1989). Down regulation of the mercury resistance operon by the most promoter-distal gene merD. Mol. Gen. Genet. 220, 69–72. doi: 10.1007/BF00260858
Petrus, A. K., Rutner, C., Liu, S., Wang, Y., Wiatrowski, H. A., and Kostka, J. E. (2015). Mercury reduction and methyl mercury degradation by the soil bacterium Xanthobacter autotrophicus Py2. Appl. Environ. Microbiol. 81, 7833–7838. doi: 10.1128/aem.01982-15
Radin, J. N., Gaddy, J. A., Gonzalez-Rivera, C., Loh, J. T., Algood, H. M., and Cover, T. L. (2013). Flagellar localization of a Helicobacter pylori autotransporter protein. MBio 4:e00613–12. doi: 10.1128/mBio.00613-12
Schue, M., Glendinning, K. J., Hobman, J. L., and Brown, N. L. (2008). Evidence for direct interactions between the mercuric ion transporter (MerT) and mercuric reductase (MerA) from the Tn501mer operon. Biometals 21, 107–116. doi: 10.1007/s10534-007-9097-4
Singh, A. K., Dhanjal, S., and Cameotra, S. S. (2014). Surfactin restores and enhances swarming motility under heavy metal stress. Colloid Surf. B Biointerfaces 116, 26–31. doi: 10.1016/j.colsurfb.2013.12.035
Sone, Y., Nakamura, R., Pan-Hou, H., Itoh, T., and Kiyono, M. (2013). Role of MerC, MerE, MerF, MerT, and/or MerP in resistance to mercurials and the transport of mercurials in Escherichia coli. Biol. Pharm. Bull. 36, 1835–1841. doi: 10.1248/bpb.b13-00554
Sone, Y., Uraguchi, S., Takanezawa, Y., Nakamura, R., Pan-Hou, H., and Kiyono, M. (2017). Cysteine and histidine residues are involved in Escherichia coli Tn21 MerE methylmercury transport. FEBS Open Bio. 7, 1994–1999. doi: 10.1002/2211-5463.12341
Soutourina, O. A., Krin, E., Laurent-Winter, C., Hommais, F., Danchin, A., and Bertin, P. N. (2002). Regulation of bacterial motility in response to low pH in Escherichia coli: the role of H-NS protein. Microbiology 148, 1543–1551. doi: 10.1099/00221287-148-5-1543
Steele, R. A., and Opella, S. J. (1997). Structures of the reduced and mercury-bound forms of MerP, the periplasmic protein from the bacterial mercury detoxification system. Biochemistry 36, 6885–6895. doi: 10.1021/bi9631632
Stelmack, P. L., Gray, M. R., and Pickard, M. A. (1999). Bacterial adhesion to soil contaminants in the presence of surfactants. Appl. Environ. Microbiol. 65, 163–168.
Tamura, K., Stecher, G., Peterson, D., Filipski, A., and Kumar, S. (2013). MEGA6: molecular evolutionary genetics analysis version 6.0. Mol. Biol. Evol. 30, 2725–2729. doi: 10.1093/molbev/mst197
Vetriani, C., Chew, Y. S., Miller, S. M., Yagi, J., Coombs, J., Lutz, R. A., et al. (2005). Mercury adaptation among bacteria from a deep-sea hydrothermal vent. Appl. Environ. Microbiol. 71, 220–226. doi: 10.1128/AEM.71.1.220-226.2005
Wahba, H. M., Stevenson, M. J., Mansour, A., Sygusch, J., Wilcox, D. E., and Omichinski, J. G. (2017). Structural and biochemical characterization of organotin and organolead compounds binding to the organomercurial lyase MerB provide new insights into its mechanism of carbon-metal bond cleavage. J. Am. Chem. Soc. 139, 910–921. doi: 10.1021/jacs.6b11327
Wang, M. H., Lee, J. S., and Li, Y. (2017). Global proteome profiling of a marine copepod and the mitigating effect of ocean acidification on mercury toxicity after multigenerational exposure. Environ. Sci. Technol. 51, 5820–5831. doi: 10.1021/acs.est.7b01832
Weller-Stuart, T., Toth, I., De Maayer, P., and Coutinho, T. (2017). Swimming and twitching motility are essential for attachment and virulence of Pantoea ananatis in onion seedlings. Mol. Plant Pathol. 18, 734–745. doi: 10.1111/mpp.12432
Wilson, J. R., Leang, C., Morby, A. P., Hobman, J. L., and Brown, N. L. (2000). MerF is a mercury transport protein: different structures but a common mechanism for mercuric ion transporters? FEBS Lett. 472, 78–82. doi: 10.1016/S0014-5793(00)01430-7
Wu, S., Zheng, R., Sha, Z., and Sun, C. (2017). Genome sequence of Pseudomonas stutzeri 273 and identification of the exopolysaccharide EPS273 biosynthesis locus. Mar. Drugs 15:218. doi: 10.3390/md15070218
Wu, S. M., Liu, G., Jin, W. H., Xiu, P. Y., and Sun, C. M. (2016). Antibiofilm and anti-infection of a marine bacterial exopolysaccharide against Pseudomonas aeruginosa. Front. Microbiol. 7:102. doi: 10.3389/fmicb.2016.00102
Yuan, C. G., Shi, J. B., He, B., Liu, J. F., Liang, L. N., and Jiang, G. B. (2004). Speciation of heavy metals in marine sediments from the East China Sea by ICP-MS with sequential extraction. Environ. Int. 30, 769–783. doi: 10.1016/j.envint.2004.01.001
Keywords: marine, Pseudomonas stutzeri, mercury, stress, motility, flagella
Citation: Zheng R, Wu S, Ma N and Sun C (2018) Genetic and Physiological Adaptations of Marine Bacterium Pseudomonas stutzeri 273 to Mercury Stress. Front. Microbiol. 9:682. doi: 10.3389/fmicb.2018.00682
Received: 01 February 2018; Accepted: 22 March 2018;
Published: 05 April 2018.
Edited by:
Conor P. O’Byrne, National University of Ireland Galway, IrelandReviewed by:
Romé Voulhoux, UMR7255 Laboratoire d’Ingénierie des Systèmes Macromoléculaires (LISM), FranceCalvin A. Henard, National Renewable Energy Laboratory (DOE), United States
Copyright © 2018 Zheng, Wu, Ma and Sun. This is an open-access article distributed under the terms of the Creative Commons Attribution License (CC BY). The use, distribution or reproduction in other forums is permitted, provided the original author(s) and the copyright owner are credited and that the original publication in this journal is cited, in accordance with accepted academic practice. No use, distribution or reproduction is permitted which does not comply with these terms.
*Correspondence: Chaomin Sun, sunchaomin@qdio.ac.cn