- Institute for Bioengineering and Biosciences, Department of Bioengineering, Instituto Superior Técnico, Universidade de Lisboa, Lisbon, Portugal
Acetic acid is an important microbial growth inhibitor in the food industry; it is used as a preservative in foods and beverages and is produced during normal yeast metabolism in biotechnological processes. Acetic acid is also a major inhibitory compound present in lignocellulosic hydrolysates affecting the use of this promising carbon source for sustainable bioprocesses. Although the molecular mechanisms underlying Saccharomyces cerevisiae response and adaptation to acetic acid have been studied for years, only recently they have been examined in more detail in Zygosaccharomyces bailii. However, due to its remarkable tolerance to acetic acid and other weak acids this yeast species is a major threat in the spoilage of acidic foods and beverages and considered as an interesting alternative cell factory in Biotechnology. This review paper emphasizes genome-wide strategies that are providing global insights into the molecular targets, signaling pathways and mechanisms behind S. cerevisiae and Z. bailii tolerance to acetic acid, and extends this information to other weak acids whenever relevant. Such comprehensive perspective and the knowledge gathered in these two yeast species allowed the identification of candidate molecular targets, either for the design of effective strategies to overcome yeast spoilage in acidic foods and beverages, or for the rational genome engineering to construct more robust industrial strains. Examples of successful applications are provided.
Introduction
The yeast Saccharomyces cerevisiae plays an essential role in the production of foods (e.g., bread) and alcoholic beverages (e.g., wine and beer). However, this yeast species is also a food spoilage agent, being able to overcome several harsh conditions that are employed in the food industry to maintain the microbial stability of its products and avoid undesirable changes in their organoleptic properties (James and Stratford, 2003).
Yeasts belonging to the genus Zygosaccharomyces are associated with a detrimental role in food and beverage industries, being considered the most problematic food spoilage yeasts. In fact, they are able to adapt and proliferate in the presence of extremely high concentrations of weak acids (Zygosaccharomyces bailii and Zygosaccharomyces lentus), sugar and salt (Z. rouxii) compared to those tolerated by other spoilage yeasts (James and Stratford, 2003). Within the genus, Z. bailii stands out as the most problematic spoilage yeast, mainly in acidified food products, such as mayonnaise, salad dressings, fruit concentrates and various non-carbonated fruit drinks, also being frequently isolated in wine due to its tolerance to both organic acids at low pH and ethanol (Thomas and Davenport, 1985; James and Stratford, 2003). Z. bailii is also an emerging spoiler of new food types such as mustards and fruit-flavored carbonated soft drinks (Sá-Correia et al., 2014). The remarkable tolerance of Z. bailii to weak acid food preservatives allows growth to occur in food products with concentrations above those legally permitted (Sá-Correia et al., 2014). Depending on the food product, the limit concentrations approved for use of sorbic and benzoic acids as food additives mainly range from 0.5 to 2 g/L (European Commission, 2011). Concerning the use of acetic acid as a food additive, the concentration is quantum satis (European Commission, 2011) this meaning that acetic acid should be used in food products under conditions that do not result in consumer’s deception. In the case of Z. bailii, the average minimum inhibitory concentration (MIC) determined for several strains is approximately 8 and 10 g/L (pH 4.0) for sorbic and benzoic acids, respectively, and around 28 g/L (pH 4.0) for acetic acid, which are much higher than the values commonly determined for S. cerevisiae (Stratford et al., 2013b). Those different tolerance levels are highly relevant also because, despite their widespread use and classification as “generally recognized as safe” (GRAS), weak acids may cause intolerance (Joneja, 2003; Stratford, 2006; Theron and Lues, 2010).
Acetic acid is also an important inhibitory byproduct of alcoholic fermentation carried out by S. cerevisiae (Garay-Arroyo et al., 2004; Graves et al., 2006) and can achieve levels that, combined with high concentrations of ethanol and other toxic metabolites, may lead to fermentation arrest or reduced ethanol productivity (Rasmussen et al., 1995; Garay-Arroyo et al., 2004; Graves et al., 2006). Moreover, acetic acid is a highly important inhibitory compound in the context of lignocellulosic hydrolysates-based bioethanol production where its presence may seriously affect fermentation performance (Jönsson et al., 2013). Concentrations of acetic acid in lignocellulosic hydrolysates strongly depend on the feedstock and on the severity of the pretreatment (Jönsson et al., 2013). Levels of 3.4 g/L (pH 5.0) can, for instance, be achieved in wheat straw hydrolysates (Olofsson et al., 2010). Although these concentrations are below S. cerevisiae MIC for acetic acid (around 9 g/L at pH 4.0) (Stratford et al., 2013b), it is the combined effect of acetic acid and several other compounds produced during pretreatment of lignocellulosic hydrolysates that inhibits S. cerevisiae fermentation performance. It is therefore essential to understand the mechanisms underlying S. cerevisiae tolerance to acetic acid in order to develop robust industrial strains.
Considering the importance of acetic acid as a yeast growth inhibitor in modern Biotechnology and Food Industry, this review paper provides an updated critical review of scientific literature on the adaptive response and tolerance to this weak acid emphasizing the physiological toxicogenomics perspective. The understanding of yeast physiology exploring functional and comparative genomic strategies allows a holistic assessment of the complex adaptive responses to environmental stresses and the identification of tolerance or susceptibility determinants to these stresses at a genome-wide scale. Yeast physiological toxicogenomics is thus instrumental to guide synthetic pathway engineering and other approaches for cell robustness manipulation, either for the sustainable production of fuels and chemicals or for the control of spoiling yeasts.
Mechanisms Underlying the Adaptive Response and Tolerance to Acetic Acid in Yeasts
The Physiological Genomic Approaches
Upon exposure to inhibitory, but sublethal, concentrations of acetic acid, yeast cells may enter a more or less extended period of growth arrest, but after this adaptation period exponential growth is resumed with a lower maximum specific growth rate (Fernandes et al., 2005; Guerreiro et al., 2012). On the other hand, lethal concentrations of acetic acid may induce regulated cell death (RCD), either by apoptosis or necrosis, depending on the severity of acetic acid stress (Ludovico et al., 2001, 2003).
After two decades of post-genomic research in S. cerevisiae, a more comprehensive understanding of the molecular mechanisms underlying this species response and adaptation to sublethal or lethal concentrations of acetic acid was obtained through the integration of several functional genomic approaches (Figure 1). When compared to S. cerevisiae, the number of Omic-based approaches applied to Z. bailii is still limited (Figure 1). Among other reasons, the lack of a Z. bailii genome sequence with suitable annotation has limited those studies for years. However, since 2013 the annotated genome sequences of two Z. bailii strains (Galeote et al., 2013; Palma et al., 2017b) and two hybrid strains resulting from Z. bailii and an unidentified Zygosaccharomyces species were also disclosed (Mira et al., 2014; Ortiz-Merino et al., 2017). This genome data has largely accelerated the understanding of Z. bailii species as a biological system with interesting genetic and physiological traits. Moreover, it has provided genomic information essential to study the mechanisms underlying Z. bailii tolerance to acetic acid, yet to be explored.
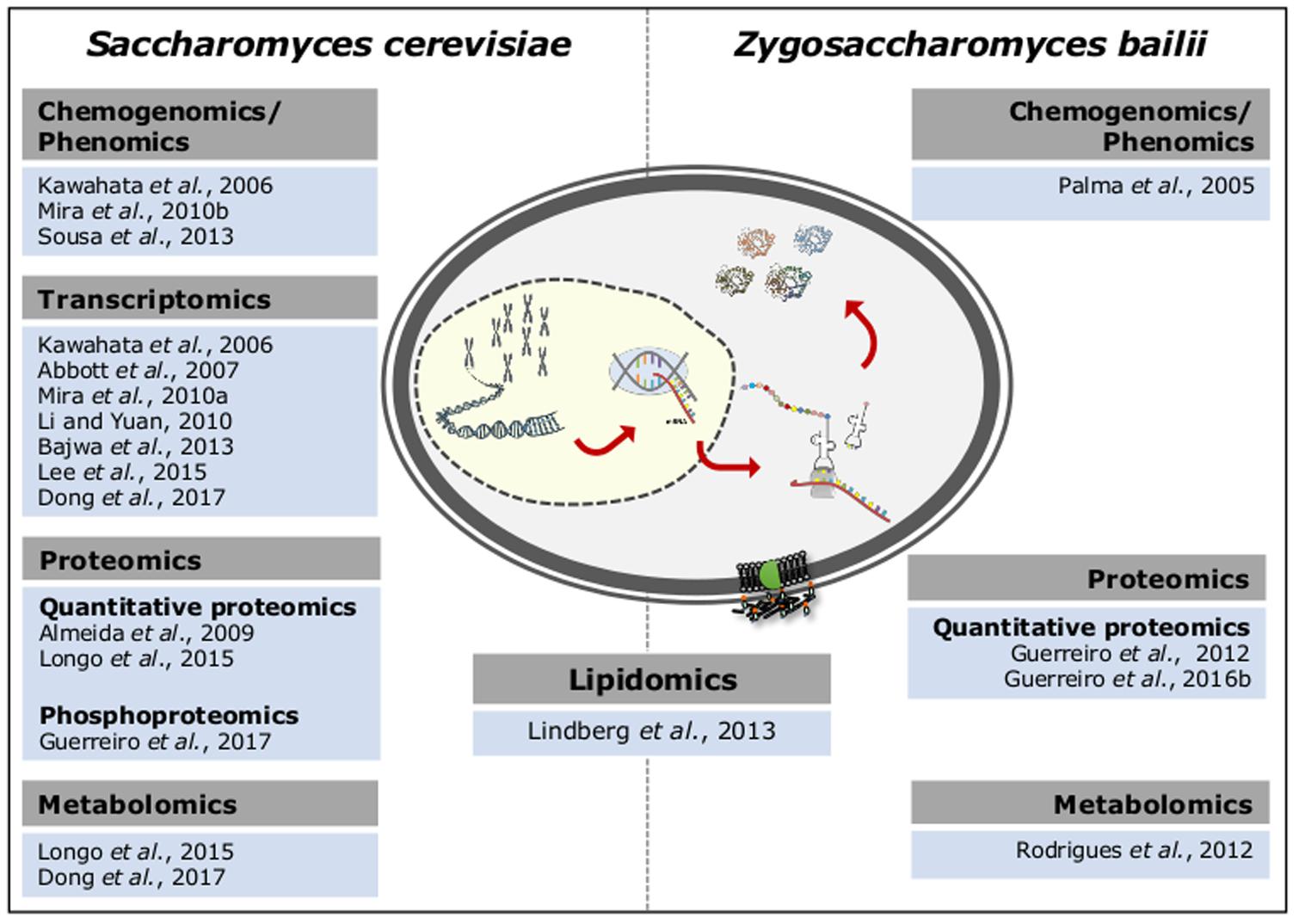
FIGURE 1. Functional genomics-based approaches explored to obtain mechanistic insights into the adaptive response and tolerance to sublethal and lethal concentrations of acetic acid in S. cerevisiae and Z. bailii.
In S. cerevisiae, two chemogenomics screenings of commercial haploid yeast mutant collections containing thousands of single deletion mutants in non-essential yeast genes were useful to identify candidate molecular determinants and mechanisms of tolerance to sublethal concentrations of acetic acid (Kawahata et al., 2006; Mira et al., 2010b). These screenings, that were performed under different experimental conditions, led to the identification of a number of genes required for maximum tolerance to acetic acid, but the two datasets only share a total of 150 genes (Mira et al., 2010c). These include, for example, genes involved in intracellular pH homeostasis, vacuolar transport, positive regulation of cellular processes, amino acid and carbohydrate metabolism (Kawahata et al., 2006; Mira et al., 2010b). Metabolic pathways that regulate RCD in S. cerevisiae response to lethal concentrations of acetic acid were also revealed based on a chemogenomics study, with carbohydrate metabolism emerging as an essential regulator of RCD (Sousa et al., 2013).
Given the unavailability of an equivalent single deletion mutant collection for Z. bailii, the identification of the determinants of tolerance to acetic acid was attempted using a genomic library of the Z. bailii-derived hybrid strain ISA1307 (Palma et al., 2015). This library was used to rescue the high acetic acid susceptibility phenotype of S. cerevisiae haa1 deletion mutant (Palma et al., 2015). Z. bailii genes putatively involved in cellular transport and transport routes, protein fate, protein synthesis, amino acid metabolism and transcription were proposed as strong candidate determinants of acetic acid tolerance in Z. bailii (Palma et al., 2015).
The transcriptional alterations occurring in S. cerevisiae cells challenged with acetic acid stress were examined in several studies performed under different experimental settings, such as different cell growth phase, acetic acid concentration and medium composition and pH (Kawahata et al., 2006; Abbott et al., 2007; Li and Yuan, 2010; Mira et al., 2010a; Bajwa et al., 2013; Lee et al., 2015; Dong et al., 2017). Transcriptional profiling of the early response of S. cerevisiae cells to acetic acid (Kawahata et al., 2006; Li and Yuan, 2010; Mira et al., 2010a) or after adaptation to this weak acid (Kawahata et al., 2006; Bajwa et al., 2013; Lee et al., 2015) were described. Response to acetic acid of steady-state anaerobic and glucose-limited chemostat S. cerevisiae cultures was also investigated (Abbott et al., 2007). Although the genes identified in different studies as up-regulated under acetic acid stress do not fully overlap, a few genes coding for plasma membrane proteins or proteins of a still unidentified function emerged in, at least, three of those works.
A quantitative proteomics analysis based on two-dimensional gel electrophoresis (2-DE) contributed to the identification of the alterations in the protein content of Z. bailii hybrid strain ISA1307 occurring in response to sudden exposure or during exponential growth in the presence of an inhibitory sublethal concentration of acetic acid (Guerreiro et al., 2012). The increased content of a particular set of proteins suggested that, in the presence of glucose, acetate is channeled into the tricarboxylic acid cycle, being co-consumed with glucose. These results were corroborated by a metabolomics study where the biochemical pathways associated with acetic acid utilization during co-metabolism with glucose were investigated in the same strain (Rodrigues et al., 2012). In Z. bailii, a quantitative proteomics study for the analysis of expression of mitochondrial proteins in cells exposed to lethal concentrations of acetic acid highlighted the importance of metabolic and energy processes, in particular of mitochondrial energetic metabolism, in acetic acid-induced RCD response (Guerreiro et al., 2016b). Cellular processes like oxidative stress response, protein translation, amino acid (in particular glutamate) and nucleotide metabolism, among others, were also found to be involved in this cellular response (Guerreiro et al., 2016b). In S. cerevisiae, quantitative proteomics was used to examine yeast response to a lethal concentration of acetic acid, revealing alterations in the levels of proteins implicated in the general amino-acid control system, further shown to be associated with a severe intracellular amino-acid starvation, as well as in the Target-of-rapamycin (TOR) pathway (Almeida et al., 2009). Moreover, quantitative proteomic and metabolomic analyses of S. cerevisiae parental and derived deletion mutant yca1 strains in acetic acid-induced RCD identified significant alterations in carbohydrate catabolism, lipid metabolism, proteolysis and stress-response, thus emphasizing the importance of Yca1 metacaspase in RCD caused by lethal concentrations of acetic acid (Longo et al., 2015).
The alterations occurring at the level of the membrane phosphoproteome during yeast early adaptive response to a sublethal concentration of acetic acid stress and the role played by the Hrk1 kinase in such response have recently been investigated (Guerreiro et al., 2017). Hrk1 is a protein kinase belonging to the “Npr1-family” of kinases dedicated to the regulation of plasma membrane transporters that was identified in previous Omics approaches as a determinant of acetic acid tolerance and involved in yeast response to acetic acid stress (Abbott et al., 2007; Mira et al., 2010a,b). The investigation of membrane phosphoproteome hinted toward the contribution of phosphorylation in the regulation of processes related with translation, protein folding and processing, transport, and cellular homeostasis in yeast response to acetic acid stress (Guerreiro et al., 2017).
The studies previously mentioned were exclusively dedicated to either S. cerevisiae or Z. bailii under acetic acid stress. In fact, high-throughput comparisons between S. cerevisiae and Z. bailii using similar experimental conditions are scarce in the context of acetic acid stress. One relevant exception is the lipidomic profiling of the major lipid species found in the plasma membrane of exponentially growing cells of both species under basal and acetic acid stress conditions (Lindberg et al., 2013). The correlation between the higher basal level of complex sphingolipids in Z. bailii when compared to S. cerevisiae, and consequent reduced plasma membrane permeability to acetic acid, was one of the most important findings in this study (Lindberg et al., 2013).
The exploitation of functional genomics approaches and tools, besides providing an integrative view on how yeast cells respond to a challenging environment, has strongly contributed to the understanding of the molecular players involved in such response. Nevertheless, the combined use of such global approaches with more focused molecular and cellular biology studies is essential to understand in depth the complexity of the mechanisms underlying the response and tolerance to a particular stress. In the following sections, the main molecular mechanisms underlying S. cerevisiae and Z. bailii response and tolerance to sublethal and lethal concentrations of acetic acid are reviewed based on genome-wide and high-throughput analyses complemented by more detailed molecular, biochemical and physiological studies.
Acetic Acid Uptake and Toxicity
Acetic acid cellular uptake is dependent both on the extracellular pH and on the specific growth conditions. During S. cerevisiae growth in glucose-repressible conditions and at a pH below acetic acid pKa (=4.76), acetic acid is mainly in its undissociated form, CH3COOH, which is able to passively diffuse across the cell membrane lipid bilayer (Casal et al., 1996). Additionally, the aquaglyceroporin Fps1 was proposed to facilitate the uptake of acetic acid into the yeast cell (Mollapour and Piper, 2007) (Figure 2). Once inside the cell, at the near-neutral cytosol, acetic acid dissociates leading to the release of protons (H+) and of the negatively charged acetate counterion (CH3COO-). During growth of derepressed S. cerevisiae cells or growth of cells at a pH higher than acetic acid pKa, the dissociated form of the acid prevails, and the acid anion is transported at least by the acetate carrier Ady2 (Casal et al., 1996; Paiva et al., 1999, 2004) (Figure 2). Once inside the cell, acetate is unable to move back across the plasma membrane by simple diffusion, and accumulates in the cell interior causing increased turgor pressure and severe oxidative stress (Piper et al., 2001). On the other hand, reduction of intracellular pH (pHi) caused by the release of protons upon acetic acid dissociation leads to the inhibition of metabolic activity, among other deleterious effects (Pampulha and Loureiro-Dias, 1989, 1990; Orij et al., 2012).
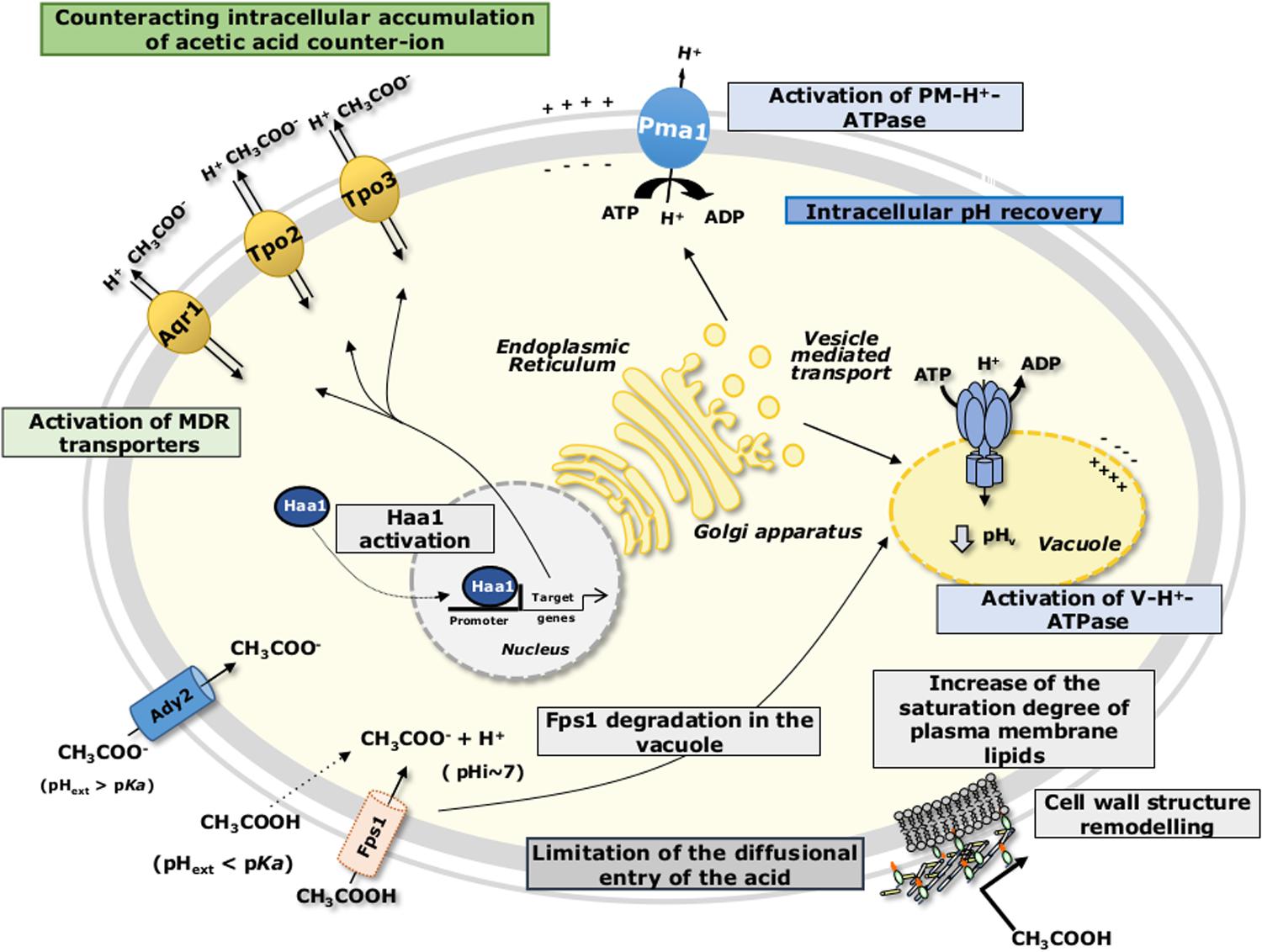
FIGURE 2. Schematic model for the adaptive response of S. cerevisiae to acetic acid-induced stress as detailed in the text. pHext, external pH; pHi, intracellular pH.
Differently from S. cerevisiae, in Z. bailii cells cultivated in the presence of both glucose and acetic acid, simple diffusion of the undissociated form of the acid seems to have a minor contribution to the overall uptake of acetic acid (Sousa et al., 1996). Under such experimental conditions or if glucose or fructose are the sole available carbon sources, a non-glucose repressible acetic acid carrier is present and controlled by the intracellular concentration of acetate (Sousa et al., 1996, 1998) (Figure 3). When Z. bailii cells are cultivated in a medium containing acetic acid as the sole carbon source, acetic acid is transported by a saturable transport system, which is also able to transport propionic and formic acids (Sousa et al., 1996) (Figure 3). However, these carriers are not yet identified.
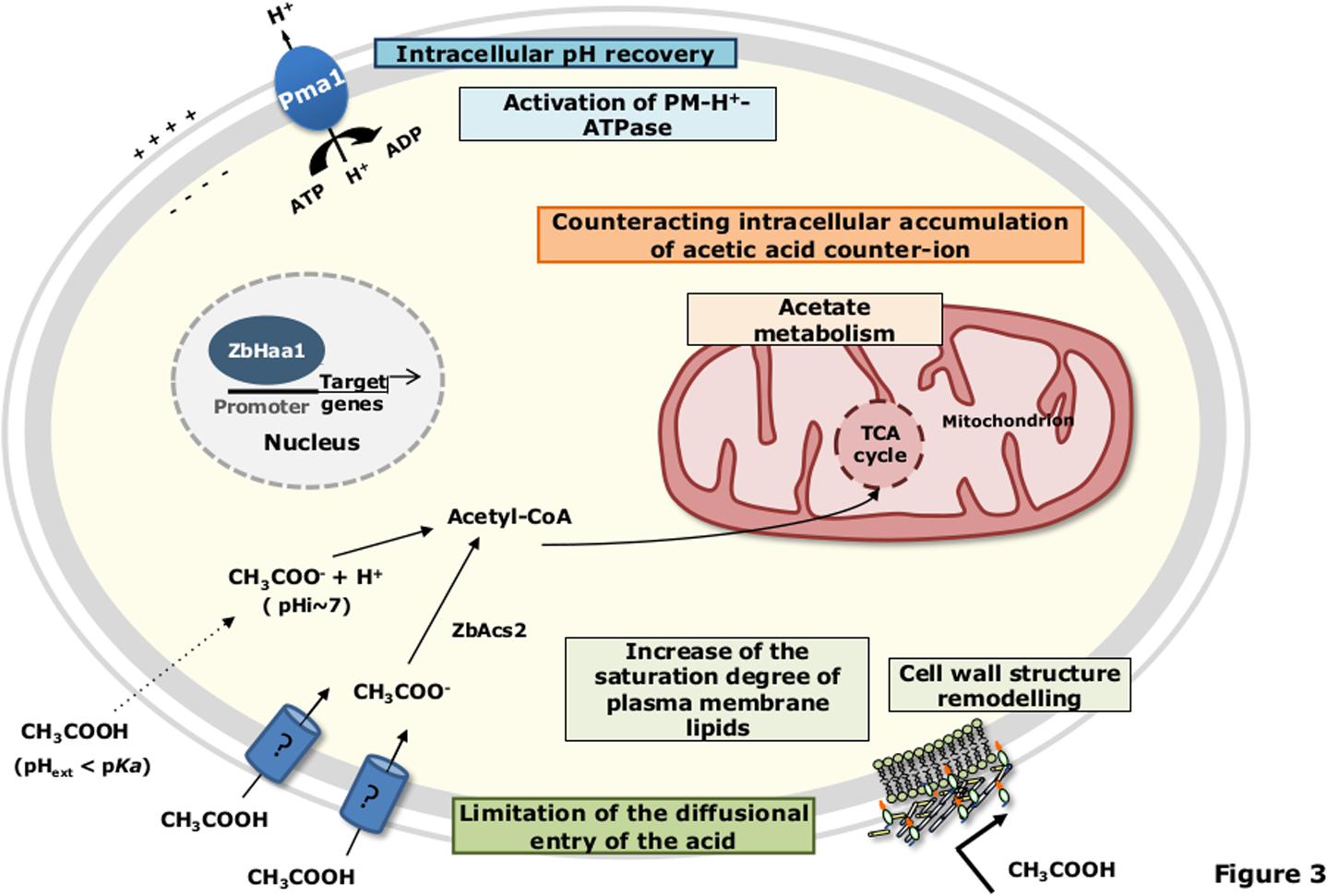
FIGURE 3. Schematic model for the adaptive response of Z. bailii to acetic acid-induced stress. Details are provided in the text. pHext, external pH; pHi, intracellular pH; TCA, tricarboxylic acid.
Intracellular Acidification and pH Recovery
Acidification of the cytosol has been considered one of the major causes of S. cerevisiae growth inhibition upon acetic acid stress (Ullah et al., 2012; Stratford et al., 2013a). However, growth inhibition by acetic acid is related not with the initial intracellular acidification levels, but rather with cells’ ability to recover a more physiological pHi (Ullah et al., 2012). In order to counteract the dissipation of the H+ gradient across plasma membrane, the yeast cell is strongly dependent on the activation of the plasma membrane H+-ATPase (mainly encoded by PMA1 gene), in coordination with the activation of vacuolar membrane H+-ATPase (Carmelo et al., 1997; Ullah et al., 2012; Stratford et al., 2013a) (Figure 2). The co-activation of these two proton pumps contributes to the active expulsion of protons accumulated in the cytosol upon acetic acid dissociation, both to the exterior of the cell and to the vacuole lumen (Carmelo et al., 1997; Stratford et al., 2013a) (Figure 2). The deletion of genes involved in pHi homeostasis, as for example VMA1, VMA2, VMA4-8, and VMA13 encoding vacuolar ATPase complex proteins confers susceptibility to S. cerevisiae cells exposed to sublethal concentrations of acetic acid (Kawahata et al., 2006; Mira et al., 2010b), strongly supporting the idea of the important role of vacuolar ATPase in adaptation to acetic acid. The energy-dependent recovery of pHi in acetic acid-stressed S. cerevisiae cells (Pampulha and Loureiro-Dias, 2000; Ullah et al., 2013), together with the inhibition of glycolytic activity induced by the release of protons and subsequent cytoplasmic acidification (Pampulha and Loureiro-Dias, 1990), leads to a reduction in cellular ATP levels. Cells exposed to different levels of acetic acid exhibit different responses in terms of intracellular ATP levels (Ullah et al., 2013). Under more severe acetic acid stress, causing complete growth inhibition, ATP levels were higher than those found in cells subjected to moderate stress, where growth was partially inhibited. This cell strategy of reducing ATP consumption upon severe stress was suggested to be advantageous to maintain energy reserves for later recovery of growth in more favorable conditions (Ullah et al., 2013).
Although the activation of plasma membrane or vacuolar membrane proton ATPases in Z. bailii cells exposed to acetic acid stress has never been studied, the activation of plasma membrane H+-ATPase activity occurs under benzoic acid stress, leading to the efflux of H+ and simultaneous influx and accumulation of K+ (Macpherson et al., 2005). These ionic movements were described as well for S. cerevisiae in short-term preservative stress (Macpherson et al., 2005).
Zygosaccharomyces bailii was suggested to better tolerate short-term decrease of pHi than S. cerevisiae (Arneborg et al., 2000), as well as significant pHi drops during exponential phase of growth that are restored afterward in stationary phase (Dang et al., 2012). Apparently, Z. bailii employs different strategies to cope with different levels of acetic acid stress (Dang et al., 2012). For more inhibitory acetic acid concentrations, pHi decreases and is maintained around the same value during exponential phase; recovery of pHi to more physiological levels is registered during the stationary phase of growth (Dang et al., 2012). However, when the growth medium is supplemented with a milder inhibitory concentration of acetic acid, there is an initial moderate drop in pHi that is maintained throughout growth, suggesting that cells adapt to the slightly lower pHi to the point where pHi recovery was not required (Dang et al., 2012).
In addition to the responses exhibited by the average yeast cell population, single cell-specific responses to acetic acid have also been investigated in both yeast species. Individual Z. bailii cells present in a population exposed to different weak acids exhibit variable tolerance to acetic, sorbic and benzoic acids (Steels et al., 2000; Stratford et al., 2013b). The most tolerant sub-population represents a small fraction of the bulk population and has a lower pHi, which leads to reduced intracellular dissociation of any weak acid and, consequently, reduced accumulation of the counterion in the cytoplasm, thus conferring tolerance to any weak acid, but not to other type of inhibitors (Stratford et al., 2013b). This cross-tolerance phenomenon indicates that tolerance is not dependent on the specific acid structure, but rather relies on a mechanism that decreases the uptake and/or accumulation of any weak acid.
Cell-to-cell heterogeneity was also implicated in S. cerevisiae tolerance to acetic acid since only the fraction of cells with low initial pHi values was able to recover pHi and resume growth in the presence of the acid (Fernández-Niño et al., 2015). However, the initial pHi is not the only factor influencing S. cerevisiae tolerance to acetic acid as shown when two strains with different tolerances to this weak acid were compared (Fernández-Niño et al., 2015).
Acetate Detoxification Mechanisms
Specific inducible transporters are presumably involved in the active expulsion of acetate from S. cerevisiae cell interior. Among them are the plasma membrane transporters of the Major Facilitator Superfamily (MFS), involved in Multidrug/Multixenobiotic Resistance (MDR/MXR) (dos Santos et al., 2014), Tpo2 and Tpo3 (Fernandes et al., 2005; Kawahata et al., 2006; Mira et al., 2010b), and Aqr1 (Tenreiro et al., 2002) (Figure 2). Remarkably, TPO2 was found to be activated in S. cerevisiae cells upon acetic acid stress in several genome-wide transcriptional profiling studies (Kawahata et al., 2006; Abbott et al., 2007; Mira et al., 2010a; Bajwa et al., 2013). In contrast to S. cerevisiae, no acetate export system has hitherto been suggested or described in Z. bailii. Moreover, there are significant differences between S. cerevisiae and Z. bailii concerning acetate catabolization. In S. cerevisiae the use of acetate as a carbon source is in general repressed by glucose, and cells cultivated in a medium containing both glucose and acetic acid exhibit diauxic growth with acetic acid only being consumed after glucose has been exhausted from the medium (Casal et al., 1996, 1998; Vilela-Moura et al., 2011). When acetic acid is the sole carbon source present in the medium, this weak acid is consumed through its conversion to acetyl coenzyme A (acetyl-CoA), catalyzed by two acetyl-CoA synthetases, Acs1 (peroxisomal) or Acs2 (cytosolic) (dos Santos et al., 2003). The acetyl-CoA produced from acetate then enters the mitochondria to be oxidized in the tricarboxylic acid (TCA) cycle or remains outside the mitochondria to be metabolized in the glyoxylate cycle, which replenishes the cell with succinate, a crucial metabolite to produce different biosynthetic precursors (dos Santos et al., 2003). Z. bailii has the ability to catabolize acetate even in the presence of glucose (Sousa et al., 1998; Guerreiro et al., 2012; Rodrigues et al., 2012). This mechanism involves the regulation of both acetic acid membrane transport and acetyl-CoA synthetase activity, allowing the maintenance of an intracellular concentration of the acetate below toxic levels (Rodrigues et al., 2004; Sousa et al., 1996). Other proteins related to carbohydrate metabolism (Mdh1, Aco1, Cit1, Idh2 and Lpd1) and energy generation (Atp1 and Atp2), as well as general and oxidative stress response (Sod2, Dak2, Omp2), are also involved in acetate catabolization in Z. bailii (Guerreiro et al., 2012). This strengthens the concept that glucose and acetic acid are co-consumed in Z. bailii, with acetate being channeled into the TCA. This behavior was hypothesized to contribute to the remarkable tolerance of Z. bailii to acetic acid, particularly in environments that are rich in both carbon sources, such as during vinification (Sousa et al., 1998) or fermentation of lignocellulosic hydrolysates (Jönsson et al., 2013).
Acetic Acid-Induced Alterations of the Cellular Envelope
It has been proposed that remodeling of cell wall and/or plasma membrane structure may occur and represent one of the most important adaptive mechanisms of tolerance to weak acids (Figures 2, 3) (Simões et al., 2006; Mollapour et al., 2009; Lindberg et al., 2013; Guerreiro et al., 2016a). Indeed, reducing cellular envelope permeability by altering cell wall and plasma membrane chemical structure and properties, thereby decreasing weak acid diffusion, is a much more energetically efficient method than relying on the active extrusion of protons and acid. Consistent with this concept, multiple genes involved in the synthesis of cell wall polysaccharides, cell wall structure assembly and remodeling, and in sphingolipid and sterol biosynthetic pathways were demonstrated to be determinants of tolerance, or to be transcriptionally responsive to acetic acid in S. cerevisiae, in several genome-wide studies (Kawahata et al., 2006; Abbott et al., 2007; Mira et al., 2010a,b; Lee et al., 2015; Longo et al., 2015).
Among the proteins found to mediate the alteration of cell wall structure in response to weak acids in S. cerevisiae, is Spi1, a glycosylphosphatidylinositol-anchored cell wall protein, which is particularly important for tolerance to lipophilic acids like benzoic or octanoic (Simões et al., 2006). Although the expression of SPI1 was considered not significant in the adaptation and tolerance to acetic acid (Simões et al., 2006), SPI1 transcription was activated in response to this acid in several transcriptional analyses (Kawahata et al., 2006; Abbott et al., 2007; Mira et al., 2010a), thereby suggesting Spi1 as an important player in response to acetic acid. Although the specific function of YGP1, encoding a cell wall-related secretory glycoprotein, is still unknown, this gene is also considered a key player in S. cerevisiae tolerance to acetic acid, since it is activated in cells exposed to acetic acid (Kawahata et al., 2006; Abbott et al., 2007; Mira et al., 2010a) and the mutant with this gene deleted is very susceptible to this acid (Mira et al., 2010b). Z. bailii YGP1 homologue was also found to be up-regulated in response to acetic acid (Palma et al., 2017a).
Major alterations occurring in the plasma membrane lipid composition are also involved in yeast tolerance to acetic acid. A lipidomic analysis revealed that, for both S. cerevisiae and Z. bailii, during aerobic growth in bioreactors, the supplementation of the growth medium with acetic acid induced significant changes in the cellular lipid content (Lindberg et al., 2013). In acetic acid-stressed Z. bailii cells, the total amount of glycerophospholipids (GPLs) was found to be slightly lower than in S. cerevisiae, while the degree of saturation of GPLs was increased under these same conditions (Lindberg et al., 2013). Increased levels of complex sphingolipids were detected for both species in the mid-exponential phase of acetic acid-adapted growth (Lindberg et al., 2013). Remarkably, the basal level of complex sphingolipids was significantly higher in Z. bailii than in S. cerevisiae leading the authors to suggest a link between high sphingolipid levels and the intrinsic tolerance of Z. bailii species to acetic acid (Lindberg et al., 2013). The correlation between the fraction of sphingolipids and membrane permeability to acetic acid was further investigated and confirmed based on in silico simulations of model membranes (Lindahl et al., 2016). Sphingolipids are essential structural components of cellular membranes, in particular the plasma membrane, playing important roles in signaling and intracellular trafficking, as well as in the regulation of diverse processes (Dickson, 2008). The importance of the regulation of sphingolipid biosynthetic pathway in S. cerevisiae response and tolerance to acetic acid was more recently examined during S. cerevisiae early adaptive response to acetic acid (Guerreiro et al., 2016a). It was demonstrated that Ypk1 phosphorylation and activation by the membrane-localized protein kinase complex TORC2 is stimulated in response to acetic acid stress, consequently activating lipid synthesis (Guerreiro et al., 2016a) (Figure 4). Several plasma membrane lipid and protein homeostasis processes are regulated by the protein kinase Ypk1 (reviewed in Roelants et al., 2017). For instance, TORC2/Ypk1 signaling was proposed to inactivate by phosphorylation the endoplasmic reticulum-associated Orm1/2 protein inhibitors of L-serine:palmitoyl-CoA acyltransferase enzyme complex Lcb1/2 that catalyzes the first step of sphingolipid biosynthesis in response to compromised sphingolipid synthesis, thus restoring sphingolipid biosynthesis (Roelants et al., 2011). In S. cerevisiae cells exposed to acetic acid Ypk1 phosphorylates Orm1 and two functionally redundant isoforms of the ceramide synthase complex, Lac1 and Lag1 (Guerreiro et al., 2016a) (Figure 4).
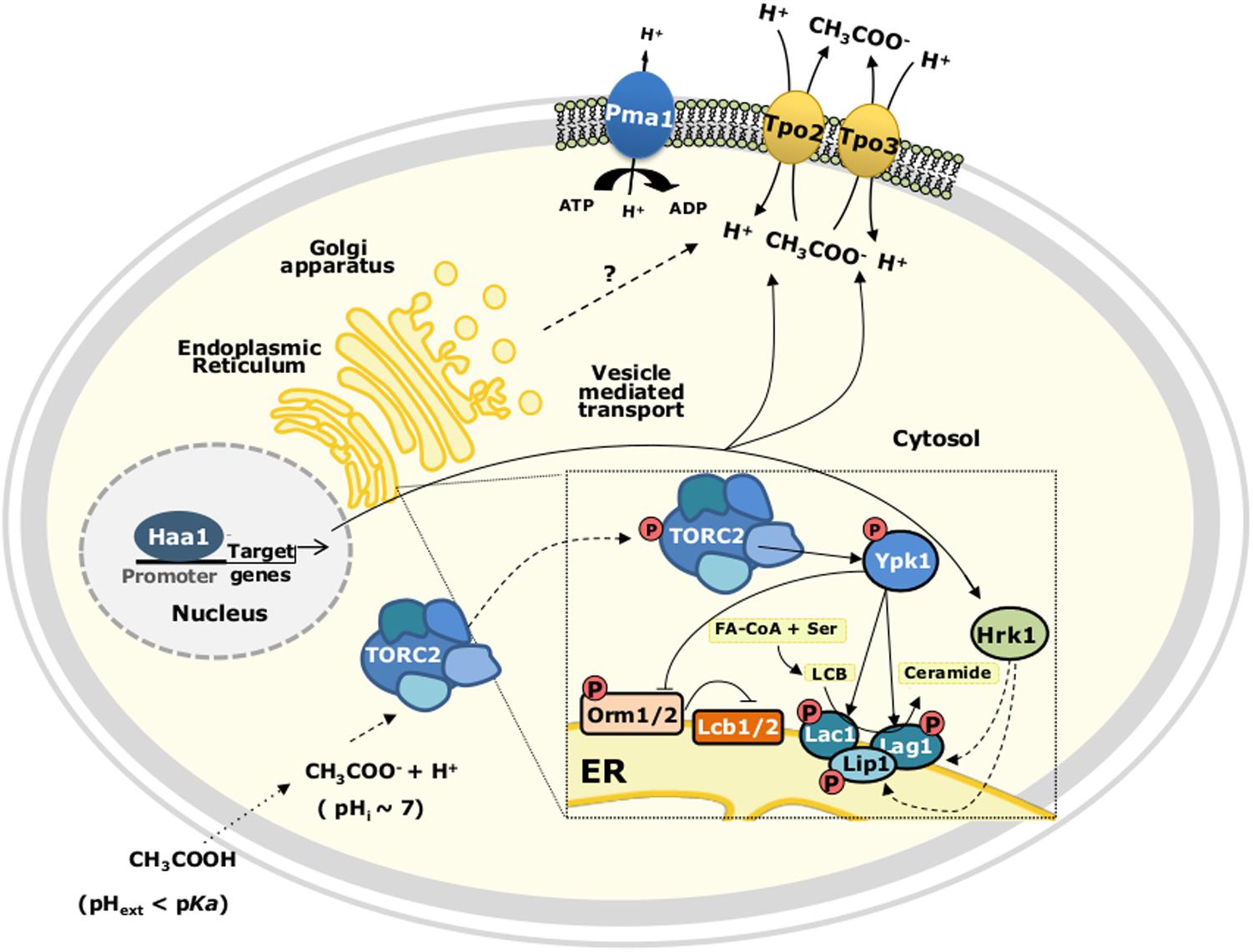
FIGURE 4. Proposed model for TORC2-Ypk1 dependent activation of the sphingolipid biosynthetic pathway in acetic acid-stressed S. cerevisiae cells. TORC2, Target of Rapamycin (TOR) Complex 2; FA-CoA, fatty acyl-CoA; LCB, long chain base.
In what concerns ergosterol content in acetic acid-challenged cells, the levels of this sterol decreased in S. cerevisiae, but not in the more tolerant species Z. bailii (Lindberg et al., 2013). Ergosterol is the major sterol present in the plasma membrane and has been shown to have vital functions in S. cerevisiae cells, affecting its membrane fluidity and permeability (Abe and Hiraki, 2009). In fact, the level of ergosterol present in the plasma membrane plays a crucial role in S. cerevisiae tolerance to several stresses (Swan and Watson, 1998; Higgins et al., 2003; Dupont et al., 2011; Henderson and Block, 2014), and multiple genes involved in sterols biosynthetic pathways were demonstrated to be determinants of tolerance or to be transcriptionally responsive to acetic acid (Mira et al., 2010a,b).
Transcriptional Regulatory Networks Controlling Adaptive Response and Tolerance to Acetic Acid
Genome-Wide Transcriptional Regulation Induced by Acetic Acid Stress in S. cerevisiae
Yeast response to stressful conditions relies on the activation of general or specific regulatory pathways that determine cell fate, either to adapt and survive in the hostile environment or to die. S. cerevisiae cells respond to several different external insults by altering the transcription level of a particular set of genes of the generally called Environmental Stress Response (ESR), mainly controlled by Msn2 and Msn4 transcriptional activators (Gasch et al., 2000). The activation of genes from the ESR program was also registered when S. cerevisiae cells are exposed to several stresses relevant in Food Industry and Industrial Biotechnology, in particular in the response to acetic acid (Kawahata et al., 2006; Abbott et al., 2007; Li and Yuan, 2010; Mira et al., 2010a) and/or other weak acids (Schüller et al., 2004; Abbott et al., 2007; Mira et al., 2009). In addition to the general stress response transcription factors Msn2/Msn4, other regulators are involved in the genome-wide transcriptional response of S. cerevisiae to weak acid-induced stress (reviewed in Mira et al., 2010c; Teixeira et al., 2011). Specifically, War1, responsible for the induction of PDR12 transcription and therefore being crucial for response and tolerance to propionic, benzoic and sorbic acids (Piper et al., 2001; Schüller et al., 2004), Rim101, required for maximal tolerance to weak acid-induced stress, including acetic acid (Mira et al., 2009), and Haa1, required for adaptation and tolerance to the more hydrophilic formic, acetic, lactic and propionic acids (Fernandes et al., 2005; Abbott et al., 2007; Henriques et al., 2017). In yeast cells exposed to stresses that lead to mitochondrial dysfunction, it is the mitochondrial retrograde (RTG) signaling pathway that establishes mitochondria-to-nucleus communication, regulating the necessary alteration of nuclear gene expression (Butow and Avadhani, 2004), which is mainly controlled by the transcriptional regulators Rtg1 and Rtg3 (Sekito et al., 2000). The integration of genome-wide data from transcriptomic profiling of S. cerevisiae response and tolerance to several weak acids has contributed to the understanding of cellular responses to weak acid-induced stress as a dynamic system, where each transcription factor-associated network can cross-talk with others (Mira et al., 2010c). Since Haa1 is considered the primary regulator of S. cerevisiae transcriptional response to acetic acid (Mira et al., 2010a), the next two sections are dedicated to this transcription factor and to its Z. bailii ortholog ZbHaa1.
The Haa1 Regulon as the Main Player in the Control of S. cerevisiae Response to Acetic Acid
Haa1 was first identified based on the homology and structural similarity with the DNA binding domain (DBD) of the copper-regulated transcription factor Cup2 (alias Ace1) (Hu et al., 1990; Keller et al., 2001). The paralog pair Haa1 and Cup2 DBDs comprise 123 and 124 amino acid residues, respectively, at the N-terminal and include a conserved zinc module and a set of four cysteine-cysteine clusters organized in a consensus sequence that forms the copper regulatory domain (CuRD). Such conservation at the level of the DBD led to the hypothesis that, like Cup2, Haa1 could play a role in copper homeostasis (Keller et al., 2001). However, metalloregulation and the involvement of Haa1 in S. cerevisiae tolerance to copper could not be associated to this transcription factor (Keller et al., 2001). Later, it was attributed for the first time a function to Haa1 as having an essential role in S. cerevisiae adaptation and tolerance to weak acids, especially to short-chain hydrophilic acids such as acetic acid (Fernandes et al., 2005). The alterations detected in yeast genomic expression during early response to acetic and lactic acids highlighted the involvement of Haa1 in the transcriptional reprogramming of S. cerevisiae cells during the adaptive response to these weak acids (Abbott et al., 2007; Mira et al., 2010a). Haa1 is required for the transcriptional activation of approximately 80% of the acetic acid-responsive genes, and thus proposed as being the main player in yeast genomic expression regulation under acetic acid stress (Mira et al., 2010a). Following Haa1-responsive element (HRE) identification (Mira et al., 2011), HRE was found to be present in the promoter region of about 55% of the genes whose expression is activated in response to acetic acid under the dependence of Haa1, suggesting that these genes are direct targets of this transcription factor (Mira et al., 2010a). The remaining Haa1-dependent acetic acid responsive genes are presumably indirectly regulated by Haa1 (Mira et al., 2010a, 2011), for example through the action of other genes encoding transcription factors directly regulated by Haa1 (Mira et al., 2010a, 2011). This is the case of Msn4, mediating the general stress response in yeast (Gasch et al., 2000), Fkh2, implicated in yeast response to oxidative stress (Postnikoff et al., 2012), and the transcriptional repressor Nrg1, also involved in yeast response to several stresses (Vyas et al., 2005; Mira et al., 2010a, 2011). These regulatory associations dependent on Haa1 upon weak acid-induced stress were highlighted recently in the upgrade of YEASTRACT database (Teixeira et al., 2017).
The biological activity of Haa1 was found to be regulated by its sub-cellular localization that, in turn, is regulated by Haa1 phosphorylation levels (Sugiyama et al., 2014). The rapid translocation of Haa1 from the cytosol to the nucleus, where it activates the transcription of its target-genes in response to lactic (Sugiyama et al., 2014) or acetic (Swinnen et al., 2017) acids, is concomitant with a decrease in Haa1 phosphorylation levels (Sugiyama et al., 2014). The casein kinase I isoform Hrr25 is an important negative regulator of Haa1, inhibiting this transcription factor’s activity by phosphorylation (Collins et al., 2017). It was also demonstrated that the exportin Msn5, which preferentially exports phosphorylated cargo proteins, interacts with Haa1 being essential to its exit from the nucleus where its function as transcription factor takes place (Sugiyama et al., 2014) (Figure 5).
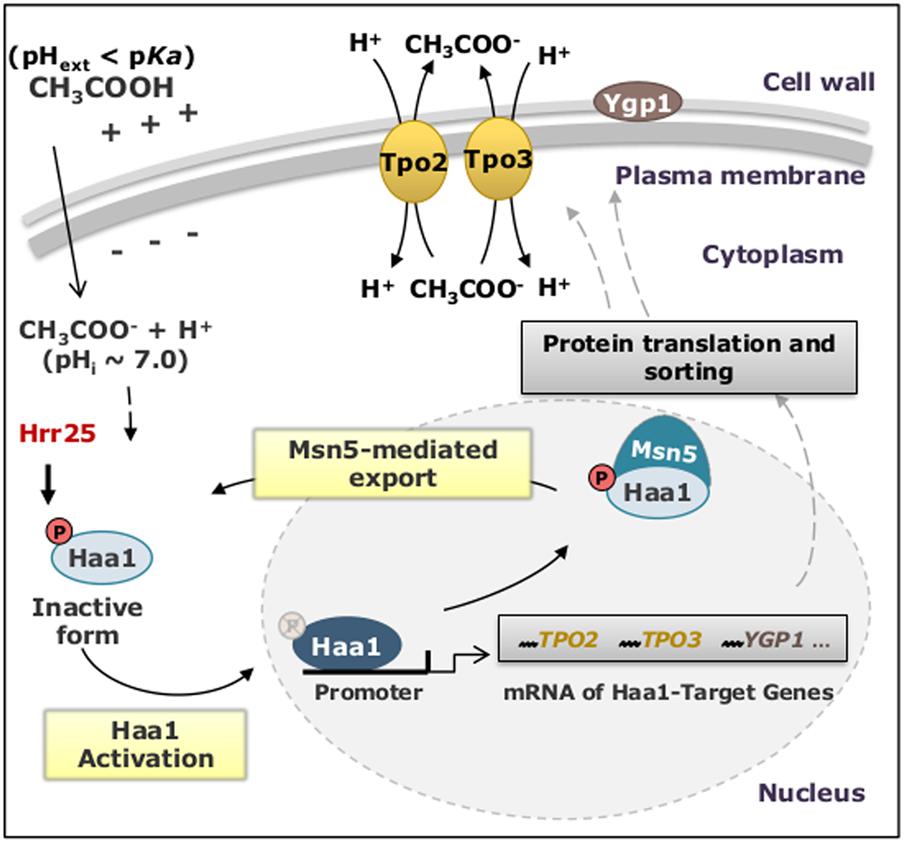
FIGURE 5. Schematic model of the mechanisms proposed to regulate Haa1 activity in S. cerevisiae, in response to lactic and acetic acid-induced stress. The description of the proposed events is detailed in the text. pHext, external pH; pHi, intracellular pH.
Among the genes of the Haa1-regulon whose expression was found to confer yeast protection against acetic acid are protein kinases, proteins involved in lipid metabolism (sphingolipids most notably ceramides, which are bioactive signaling molecules known to play a crucial role in lipid-based signaling in yeast response to stress), in nucleic acid processing, multidrug resistance transporters and proteins of unknown function. The expression of SAP30 (encoding a subunit of the Rpd3L histone deacetylase complex) and HRK1 provided the strongest protective effect toward acetic acid (Mira et al., 2010a). Even though the first biological function attributed to Hrk1 was the activation of the plasma membrane proton pump Pma1 in response to glucose metabolism (Goossens et al., 2000), its mild effect on Pma1 activity suggests that this kinase could play other roles in yeast. The fact that hrk1Δ cells showed a marked increase in the intracellular concentration of radiolabeled acetic acid and that Hrk1 belongs to a family of kinases dedicated to the regulation of plasma membrane transporters, led to hypothesize that Hrk1 could be involved in the regulation of one or more plasma membrane acetate exporters, such as the MDR/MXR transporters Tpo3, Tpo2 and Aqr1, all known determinants of tolerance to this weak acid (Mira et al., 2010a,b). To elucidate the biological role of Hrk1, the effect of Hrk1 expression in yeast membrane associated-phosphoproteome was examined in S. cerevisiae parental and hrk1Δ cells exposed, or not, to sublethal concentrations of acetic acid (Guerreiro et al., 2017). In this study, the MDR/MXR transporters Tpo3 and Tpo4 were found to exhibit altered phosphorylation in response to acetic acid stress under the dependence of Hrk1. This evidence led to hypothesize that the Hrk1-mediated phosphorylation of Tpo3 may contribute to regulate the activity of this drug pump (Guerreiro et al., 2017), thereby lowering the accumulation of acetic acid in the parental strain when compared to hrk1Δ or tpo3Δ cells, as previously described (Fernandes et al., 2005; Mira et al., 2010a). Other important membrane proteins in the context of response and tolerance to acetic acid-induced stress are present in the published dataset and certainly deserve further studies (Guerreiro et al., 2017).
The Z. bailii Haa1 (ZbHaa1) Is Required for Acetic Acid and Copper Stress Responses
The importance of ZbHaa1 in Z. bailii response and tolerance to acetic acid was demonstrated using the strain Z. bailii IST302 that proved to be susceptible to genetic engineering and has the genome fully sequenced (Palma et al., 2015, 2017a). ZbHaa1 was found to be a functional homolog of Haa1 by rescuing the acetic acid susceptibility phenotype of S. cerevisiae haa1Δ. Moreover, the disruption of ZbHAA1 and the expression of an extra ZbHAA1 copy in Z. bailii confirmed ZbHAA1 as a determinant of acetic acid tolerance in this yeast species. The expression of ZbHAA1 was found to be required for acetic acid stress-induced transcriptional activation of Z. bailii genes homologs to the demonstrated S. cerevisiae Haa1-target genes: HRK1, TPO3, MSN4, YGP1, YRO2 and HSP30 (Palma et al., 2017a). Remarkably, ZbHaa1 (the single ortholog of S. cerevisiae Haa1 and Cup2) was demonstrated to have a role in metalloregulation, being involved in copper tolerance and copper-induced transcriptional regulation, a role associated to S. cerevisiae Cup2, but not to Haa1 (Palma et al., 2017a). Phylogenetic and gene neighborhood analyses suggested the subfunctionalization of Z. bailii ancestral bifunctional protein Haa1/Cup2 after the whole-genome duplication event, originating S. cerevisiae Haa1 and Cup2 paralogs. As found for S. cerevisiae, ZbHaa1 is likely a candidate molecular target for the design of new strategies to overcome Z. bailii spoilage in foods and beverages.
Mechanisms Involved in Yeast Response to Lethal Concentrations of Acetic Acid
High concentrations of acetic acid are able to induce in Z. bailii either an apoptotic or a necrotic death process, depending on the acid concentration present in the medium, as observed for S. cerevisiae (Ludovico et al., 2001, 2003). However, since Z. bailii is highly resistant to acetic acid-induced cell death, this effect is observed at much higher concentrations of the acid for this yeast species (range of 320–800 mM, pH 3.0) than in S. cerevisiae (20–120 mM, pH 3.0) (Ludovico et al., 2001, 2002, 2003). The main known mechanisms involved in acetic acid-induced RCD were also much more extensively studied in S. cerevisiae than in Z. bailii.
In S. cerevisiae, acetic acid is known to induce a RCD process with an apoptotic phenotype that is dependent on mitochondria. Indeed, apart from its bioenergetic function, mitochondria have an essential role in the decision of cells’ life or death (reviewed in Ždralević et al., 2012) and many of the hallmarks involved in yeast mitochondria-dependent RCD process with an apoptotic phenotype have been characterized, including the translocation of pro-apoptotic factors (e.g., cytochrome c) from the mitochondria to the cytosol, phosphatidylserine externalization to the outer layer of the cytoplasmic membrane, production and consequent build-up of mitochondrial reactive oxygen species (ROS), DNA fragmentation and chromatin condensation (reviewed in Carmona-Gutierrez et al., 2010; Guaragnella et al., 2012).
Concerning tolerance to acetic acid-induced RCD, the activation of the RTG signaling pathway was proposed to be involved when S. cerevisiae cells were cultivated in a non-repressible carbon source such as raffinose (Guaragnella et al., 2013) or previously adapted to low pH environments (Giannattasio et al., 2005). In yeast, the mitochondrial RTG pathway acts in parallel with the TOR and the Ras-cAMP pathways in the regulation of acetic acid-induced RCD (Phillips et al., 2006; Almeida et al., 2009; Giannattasio et al., 2013).
In Z. bailii the morphological changes observed during RCD induced by high concentrations of acetic acid show extensive mitochondrial ultrastructural changes during the RCD process that were not seen for S. cerevisiae when equivalent deleterious concentrations were used (Ludovico et al., 2003). The acetic acid-induced RCD process with an apoptotic phenotype was also characterized in Z. bailii by the maintenance of plasma membrane integrity, DNA fragmentation, ROS production, and cytochrome c translocation from the mitochondria into the cytosol (Ludovico et al., 2003; Guerreiro et al., 2016b). The global mitochondrial proteomic response to acetic acid-induced RCD in Z. bailii hybrid strain ISA1307 was examined by 2-DE quantitative proteomics (Guerreiro et al., 2016b). The increase of different ROS (namely H2O2 and superoxide anion) observed in Z. bailii cells undergoing RCD induced by acetic acid, coupled with different changes in abundance of several antioxidant enzymes observed in that same cell population, suggest that dynamic modulation of ROS might be taking place in cells exposed to acetic acid concentrations that induce RCD (Guerreiro et al., 2016b). Nevertheless, the effectors that play a role in acetic acid-induced RCD remain poorly characterized.
Physiological Genomics-Guided Strategies to Improve Acetic Acid Tolerance
The topics covered in this review show that acetic acid tolerance phenotype is complex and multifactorial since it requires coordinated changes at several levels in the cell. For this reason, some of the most promising strategies being used to obtain more robust industrial strains involve the manipulation of genes that play a crucial role in the regulatory cascades controlling stress tolerance and the exploitation of genome engineering strategies, which allows the generation of diversity and subsequent selection of the strains that possess the trait of interest. The exploitation of this and other strategies are reviewed in the next sections.
Overexpression or Deletion of Single Genes
The genetic engineering of laboratory S. cerevisiae strains through the overexpression of single genes has yielded strains with increased acetic acid tolerance. Specifically, the overexpression of WHI2, encoding a protein required for full activation of the general stress response (Chen et al., 2016b), of the genes PEP3, encoding a vacuolar membrane protein involved in vesicular tethering/docking/fusion, STM1, encoding a protein required for optimal translation under nutrient stress, PEP5, encoding the E3 ubiquitin protein-ligase involved in the catabolism of histones (Ding et al., 2015a), or the gene ACS2, encoding acetyl-coA synthetase isoform (Ding et al., 2015b), decreased the duration of lag phase of S. cerevisiae cells cultured with acetic acid. Improvement of S. cerevisiae growth and alcoholic fermentation performance in the presence of acetic acid also resulted from the overexpression of SET5 and PPR1, coding for a methyltransferase for the methylation of histone H4 at Lys5, -8, and -12 and a transcription factor involved in the regulation of pyrimidine pathway, respectively (Zhang et al., 2015). The overexpression of ASC1 (G-protein beta subunit), GND1 (6-phosphogluconate dehydrogenase) (Lee et al., 2015), PMA1 (Lee et al., 2017) or COX20 (cytochrome oxidase chaperone) (Kumar et al., 2015) also increased S. cerevisiae tolerance to acetic acid.
Saccharomyces cerevisiae increased robustness to acetic acid has also been accomplished through expression of Z. bailii genes ZbMSN4, ZbTIF3 (Palma et al., 2015), encoding the homologs of S. cerevisiae general stress response transcription factor and translation initiation factor, respectively, or ZbHAA1 (Palma et al., 2017a). The overexpression of these genes in Z. bailii were also found to increase its tolerance to acetic acid (Palma et al., 2015).
An increase in S. cerevisiae tolerance to acetic acid was also demonstrated by individual deletion of several genes. Specifically, the deletion of HSP82 (protein chaperone), ATO2 (putative transmembrane protein involved in export of ammonia) and SSA3 (ATPase involved in protein folding and the response to stress) increased S. cerevisiae tolerance to acetic acid, presumably by contributing indirectly to enhanced proton export and diminished levels of H2O2 within the cell upon acetic acid stress (Lee et al., 2015). The deletion of RTT109 (histone acetyltransferase) also increased S. cerevisiae tolerance to acetic acid, which was suggested to be related to the activation of transcription of stress responsive genes and to increased resistance to oxidative stress (Cheng et al., 2016). In addition, the elimination of JJJ1 (co-chaperone) enhanced acetic acid tolerance, which was proposed to be related to increased levels of long-chain fatty acids and trehalose in the cell, together with an increase in catalase activity (Wu et al., 2016). Remarkably, a single mutation in cytochrome c (the substitution of the highly conserved residue tryptophan 65 by a serine) that impaired electron transfer to the functional cyt c oxidase, was found to lead to increased cellular viability upon acetic acid stress (Guaragnella et al., 2011).
Manipulation of Haa1-Regulon in S. cerevisiae
The manipulation of the Haa1-regulon, namely through HAA1 overexpression (Tanaka et al., 2012; Inaba et al., 2013; Sakihama et al., 2015; Chen et al., 2016a) or HAA1 mutation (Zahn and Jacobson, 2015; Swinnen et al., 2017) was shown to increase tolerance to acetic acid. In a first study, the HAA1 gene was placed under the control of the constitutive TDH3 promoter (HAA1-OP strain) (Tanaka et al., 2012). This promoter exchange led to increased HAA1 transcript levels, as well as of four of the Haa1-regulated genes (TPO2, TPO3, YRO2 and YGP1) even when cells were grown in the absence of acetic acid stress (Tanaka et al., 2012). The resulting strain, HAA1-OP, showed increased tolerance to acetic acid and decreased intracellular acetic acid accumulation when compared with the parental strain (Tanaka et al., 2012). A similar approach was used in an attempt to improve the industrial strain Ethanol-Red (ER). The resulting diploid ER HAA1-OP strain (with HAA1 transcription being under the control of the TDH3 promoter) showed increased HAA1 transcription levels in non-stress conditions, and was also less susceptible to acetic acid-induced stress than the corresponding parental strain (Inaba et al., 2013). HAA1 was also overexpressed from a multicopy plasmid in a successful attempt to increase ethanol productivity during xylose fermentation in the presence of acetic acid (Sakihama et al., 2015). Likewise, HAA1 overexpression improved specific sugar consumption and cell growth rate in the presence of acetic acid when compared with the non-transformed strain (Chen et al., 2016a). Based on error-prone PCR of the HAA1 coding sequence, a highly tolerant mutant allele holding two point mutations was identified as being able to improve the acetic acid tolerance of S. cerevisiae (Swinnen et al., 2017). Following dissection of the individual contribution of each mutation, it was found that the major improvement in acetic acid tolerance was caused by a single amino acid exchange at position 135 (serine to phenylalanine). Remarkably, the transcriptional levels of four Haa1-target genes, selected at random, significantly increased in acetic acid-challenged cells of the strain harboring the single Haa1 mutation when compared to cells of the parental strain (Swinnen et al., 2017). In a patented work, mutagenesis of the Haa1 at the level of its transactivation domain was also found to increase the activity to this transcription factor, with a consequent increase in the yield of ethanol produced during fermentation of lignocellulose hydrolysates containing acetic acid (Zahn and Jacobson, 2015). All these studies suggest that the genetic manipulation of the Haa1 pathway is suitable to obtain more robust S. cerevisiae strains in an industrial context.
S. cerevisiae Evolutionary Engineering and Genome Shuffling
The use of evolutionary engineering strategies, usually focusing on selection of a unique genetic trait responsible for that advantageous phenotype (Wright et al., 2011; Koppram et al., 2012), were also successfully employed for the improvement of S. cerevisiae tolerance to acetic acid. For example, a novel laboratory evolution strategy based on alternating cultivation cycles in the presence or absence of acetic acid was recently described as conferring a selective advantage to cells that are constitutively tolerant to acetic acid (González-Ramos et al., 2016). Mutations in four genes (ASG1, ADH3, SKS1 and GIS4) were identified in this study as being implicated in the constitutive acetic acid tolerance phenotype of the evolved strains (González-Ramos et al., 2016). More recently, an evolutionary engineering study involving the cross of a strain with high acetic acid tolerance with an industrial reference strain, followed by multiple rounds of inbreeding of the resulting haploid segregants and quantitative trait loci (QTL) mapping, envisaged the study of the polygenic nature of the high acetic acid tolerance phenotype (Meijnen et al., 2016). This study allowed the identification of a mutated HAA1 allele (serine to asparagine amino acid substitution at position 506) responsible for the superior character of the segregant strain under acetic acid stress (Meijnen et al., 2016). Among the novel genes identified in this study as contributing to high acetic acid tolerance is DOT5, encoding a nuclear thiol peroxidase and functioning as an alkyl-hydroperoxide reductase agent during post-diauxic growth. Remarkably, this gene also proved to play an important role in acetic acid tolerance in a study that also mapped the QTLs of segregants obtained from inbreeding of two industrial strains with distinct acetic acid tolerance phenotypes (Geng et al., 2016). Genome shuffling has also emerged as an alternative experiment strategy to improve tolerance to several stressors, including acetic acid (Zheng et al., 2011).
S. cerevisiae Transcriptome Remodeling
The remodeling of the yeast transcriptome through global transcription machinery engineering has also been applied successfully to obtain acetic acid-tolerant strains. This was achieved by re-programming the cell transcriptome through mutations in SPT15, encoding the TATA-binding protein, followed by screening of the mutants with improved tolerance to acetic acid (An et al., 2015). Another example involved the transformation of a yeast strain with an artificial zinc finger protein transcription factor library and subsequent selection of acetic acid-tolerant strains (Ma et al., 2015). Remodeling of transcription through introduction of point mutation in H3/H4 histones (Liu et al., 2014), as well as the generation of extensive alterations in mRNA metabolism through mutagenesis of the poly(A) binding protein encoding gene, PAB1 (Martani et al., 2015), have also led to the development of strains with improved robustness against acetic acid stress.
Supplementation of Growth Media with Cations
Saccharomyces cerevisiae tolerance to acetic acid can be alleviated by changing the composition of the growth media. The uptake of ions is essential in acetic acid tolerance, and several genes involved in ion homeostasis, for instance potassium transporters, were identified as determinants of tolerance to this weak acid (Kawahata et al., 2006; Mira et al., 2010b). This evidence led the authors to suggest and confirm that potassium supplementation of the growth medium may decrease acetic acid-induced growth inhibition (Mira et al., 2010b). Indeed, potassium is essential for many physiological functions, such as regulation of pHi, maintenance of plasma membrane potential, protein synthesis, and enzyme activation (Ariño et al., 2010), which are biological processes highlighted in this review as playing a role in response and tolerance to acetic acid. The increase of extracellular concentration of potassium was also found to be beneficial to increase the tolerance to higher alcohols and ethanol production in both commercial and laboratory strains (Lam et al., 2014). The supplementation of the growth medium with zinc sulfate also improved S. cerevisiae tolerance to acetic acid, with zinc presumably acting as an anti-oxidative agent (Wan et al., 2015). The addition of zinc and other metal ions (Mg2+ and Ca2+) was also associated to an increase of S. cerevisiae tolerance toward acetic acid (Ismail et al., 2014). The comparison of the transcriptional profiling of cultures supplemented or not with each of the three metal ions and acetic acid suggested that these ions are involved in the regulation of different genes, and that the up-regulation of cell wall and membrane genes is related with the increased tolerance to acetic acid upon metal ion supplementation (Ismail et al., 2014).
Concluding Remarks and Future Perspectives
The mechanisms underlying the adaptive response and tolerance to acetic acid in S. cerevisiae and Z. bailii have been enlightened over the past two decades based on functional and comparative genomics strategies. The knowledge gathered in the mechanisms of yeast response and tolerance to acetic acid have been mostly explored in the development of acetic acid tolerant industrial strains, rather than the in design of novel food preservation technologies. Future manipulation of S. cerevisiae tolerance to acetic acid will continue to rely on increasing industrial strains robustness, either through the genetic manipulation of genes that play a crucial role in the regulatory cascades that control stress tolerance, or through genome-scale engineering, thereby allowing the generation of strain diversity and subsequent selection of the strains that possess the trait of interest. Nevertheless, caution is needed during the application of the abovementioned strategies, considering that the impact of the modifications introduced in the engineered strains must be carefully evaluated regarding the potential changes they might cause in important industrial properties of that strain (Deparis et al., 2017). Moreover, many of the successful improvement studies described so far have used low acetic acid tolerant laboratory strains, but their usefulness when applied to highly acetic acid tolerant industrial strains remains to be proved.
Understanding the mechanisms of tolerance to acetic acid in S. cerevisiae and Z. bailii has undoubtedly brought to light the interspecies diversity and complexity of those mechanisms, which rely on several molecular and physiological responses orchestrated by the expression of multiple genes. Although the model yeast S. cerevisiae has been at the forefront of numerous molecular, physiological and genome-wide studies on acetic acid response and tolerance, the release of Z. bailii genome annotated sequences and the availability of new Z. bailii strains more prone to genetic and laboratory manipulations and of new molecular genetic tools, such as the genome editing tool CRISPR/Cas9, is changing this paradigm. Extensive transcriptomic profiling studies are expected to emerge to characterize the transcriptional regulatory networks underlying Z. bailii response and adaptation to acetic acid stress. Given the described relevance of ZbHaa1, the identification and manipulation of the ZbHaa1-signaling pathway in acetic acid challenged Z. bailii cells is anticipated as a promising strategy to identify novel molecular targets in this food spoilage yeast species that can also be regarded as potential cell factory for the overproduction of organic acids.
The integrative perspective of the cellular processes described herein has benefited from the exploitation of a systems microbiology approach. They are granting the rational design of strategies to improve alcoholic fermentation processes when yeasts are used as microbial factories.
Author Contributions
All authors listed have made a substantial, direct and intellectual contribution to the work, and approved it for publication.
Funding
The work performed in IS-C laboratory at iBB (Institute for Bioengineering and Biosciences) was supported by ‘Fundação para a Ciência e a Tecnologia’ (FCT) (current project contracts: PTDC/BBB-BEP/0385/2014, ERA-IB-2/0003/2015). Funding received by iBB from FCT (UID/BIO/04565/2013) and from Programa Operacional Regional de Lisboa 2020 (Project N. 007317) is also acknowledged.
Conflict of Interest Statement
The authors declare that the research was conducted in the absence of any commercial or financial relationships that could be construed as a potential conflict of interest.
Acknowledgments
IS-C acknowledges all those who have, over the years, contributed to the field of “Yeast adaptive response and tolerance to acetic acid” in her laboratory.
References
Abbott, D. A., Knijnenburg, T. A., de Poorter, L. M. I., Reinders, M. J. T., Pronk, J. T., and van Maris, A. J. A. (2007). Generic and specific transcriptional responses to different weak organic acids in anaerobic chemostat cultures of Saccharomyces cerevisiae. FEMS Yeast Res. 7, 819–833. doi: 10.1111/j.1567-1364.2007.00242.x
Abe, F., and Hiraki, T. (2009). Mechanistic role of ergosterol in membrane rigidity and cycloheximide resistance in Saccharomyces cerevisiae. Biochim. Biophys. Acta 1788, 743–752. doi: 10.1016/j.bbamem.2008.12.002
Almeida, B., Ohlmeier, S., Almeida, A. J., Madeo, F., Leão, C., Rodrigues, F., et al. (2009). Yeast protein expression profile during acetic acid-induced apoptosis indicates causal involvement of the TOR pathway. Proteomics 9, 720–732. doi: 10.1002/pmic.200700816
An, J., Kwon, H., Kim, E., Lee, Y. M., Ko, H. J., Park, H., et al. (2015). Tolerance to acetic acid is improved by mutations of the TATA-binding protein gene. Environ. Microbiol. 17, 656–669. doi: 10.1111/1462-2920.12489
Ariño, J., Ramos, J., and Sychrová, H. (2010). Alkali metal cation transport and homeostasis in yeasts. Microbiol. Mol. Biol. Rev. 74, 95–120. doi: 10.1128/MMBR.00042-09
Arneborg, N., Jespersen, L., and Jakobsen, M. (2000). Individual cells of Saccharomyces cerevisiae and Zygosaccharomyces bailii exhibit different short-term intracellular pH responses to acetic acid. Arch. Microbiol. 174, 125–128. doi: 10.1007/s002030000185
Bajwa, P. K., Ho, C.-Y., Chan, C.-K., Martin, V. J. J., Trevors, J. T., and Lee, H. (2013). Transcriptional profiling of Saccharomyces cerevisiae T2 cells upon exposure to hardwood spent sulphite liquor: comparison to acetic acid, furfural and hydroxymethylfurfural. Antonie Van Leeuwenhoek 103, 1281–1295. doi: 10.1007/s10482-013-9909-1
Butow, R. A., and Avadhani, N. G. (2004). Mitochondrial signaling: the retrograde response. Mol. Cell 14, 1–15. doi: 10.1016/S1097-2765(04)00179-0
Carmelo, V., Santos, H., and Sá-Correia, I. (1997). Effect of extracellular acidification on the activity of plasma membrane ATPase and on the cytosolic and vacuolar pH of Saccharomyces cerevisiae. Biochim. Biophys. Acta 1325, 63–70. doi: 10.1016/S0005-2736(96)00245-3
Carmona-Gutierrez, D., Eisenberg, T., Büttner, S., Meisinger, C., Kroemer, G., and Madeo, F. (2010). Apoptosis in yeast: triggers, pathways, subroutines. Cell Death Differ. 17, 763–773. doi: 10.1038/cdd.2009.219
Casal, M., Cardoso, H., and Leão, C. (1996). Mechanisms regulating the transport of acetic acid in Saccharomyces cerevisiae. Microbiology 6, 1385–1390. doi: 10.1099/13500872-142-6-1385
Casal, M., Cardoso, H., and Leão, C. (1998). Effects of ethanol and other alkanols on transport of acetic acid in Saccharomyces cerevisiae. Appl. Environ. Microbiol. 64, 665–668.
Chen, Y., Sheng, J., Jiang, T., Stevens, J., Feng, X., and Wei, N. (2016a). Transcriptional profiling reveals molecular basis and novel genetic targets for improved resistance to multiple fermentation inhibitors in Saccharomyces cerevisiae. Biotechnol. Biofuels 9:9. doi: 10.1186/s13068-015-0418-5
Chen, Y., Stabryla, L., and Wei, N. (2016b). Improved acetic acid resistance in Saccharomyces cerevisiae by overexpression of the WHI2 gene identified through inverse metabolic engineering. Appl. Environ. Microbiol. 82, 2156–2166. doi: 10.1128/AEM.03718-15
Cheng, C., Zhao, X., Zhang, M., and Bai, F. (2016). Absence of Rtt109p, a fungal-specific histone acetyltransferase, results in improved acetic acid tolerance of Saccharomyces cerevisiae. FEMS Yeast Res. 16:fow010. doi: 10.1093/femsyr/fow010
Collins, M. E., Black, J. J., and Liu, Z. (2017). Casein kinase I isoform Hrr25 is a negative regulator of Haa1 in the weak acid stress response pathway in Saccharomyces cerevisiae. Appl. Environ. Microbiol. 83: e00672–17. doi: 10.1128/AEM.00672-17
Dang, T. D. T., De Maeseneire, S. L., Zhang, B. Y., De Vos, W. H., Rajkovic, A., Vermeulen, A., et al. (2012). Monitoring the intracellular pH of Zygosaccharomyces bailii by green fluorescent protein. Int. J. Food Microbiol. 156, 290–295. doi: 10.1016/j.ijfoodmicro.2012.03.028
Deparis, Q., Claes, A., Foulquié-Moreno, M. R., and Thevelein, J. M. (2017). Engineering tolerance to industrially relevant stress factors in yeast cell factories. FEMS Yeast Res. 17:fox036. doi: 10.1093/femsyr/fox036
Dickson, R. C. (2008). Thematic review series: Sphingolipids. New insights into sphingolipid metabolism and function in budding yeast. J. Lipid Res. 49, 909–921. doi: 10.1194/jlr.R800003-JLR200
Ding, J., Holzwarth, G., Bradford, C. S., Cooley, B., Yoshinaga, A. S., Patton-Vogt, J., et al. (2015a). PEP3 overexpression shortens lag phase but does not alter growth rate in Saccharomyces cerevisiae exposed to acetic acid stress. Appl. Microbiol. Biotechnol. 99, 8667–8680. doi: 10.1007/s00253-015-6708-9
Ding, J., Holzwarth, G., Penner, M. H., Patton-Vogt, J., and Bakalinsky, A. T. (2015b). Overexpression of acetyl-CoA synthetase in Saccharomyces cerevisiae increases acetic acid tolerance. FEMS Microbiol. Lett. 362, 1–7. doi: 10.1093/femsle/fnu042
Dong, Y., Hu, J., Fan, L., and Chen, Q. (2017). RNA-Seq-based transcriptomic and metabolomic analysis reveal stress responses and programmed cell death induced by acetic acid in Saccharomyces cerevisiae. Sci. Rep. 7:42659. doi: 10.1038/srep42659
dos Santos, M. M., Gombert, A. K., Christensen, B., Olsson, L., and Nielsen, J. (2003). Identification of in vivo enzyme activities in the cometabolism of glucose and acetate by Saccharomyces cerevisiae by using 13C-labeled substrates. Eukaryot. Cell 2, 599–608. doi: 10.1128/EC.2.3.599-608.2003
dos Santos, S. C., Teixeira, M. C., Dias, P. J., and Sá-Correia, I. (2014). MFS transporters required for multidrug/multixenobiotic (MD/MX) resistance in the model yeast: understanding their physiological function through post-genomic approaches. Front. Physiol. 5:180. doi: 10.3389/fphys.2014.00180
Dupont, S., Beney, L., Ferreira, T., and Gervais, P. (2011). Nature of sterols affects plasma membrane behavior and yeast survival during dehydration. Biochim. Biophys. Acta 1808, 1520–1528. doi: 10.1016/j.bbamem.2010.11.012
European Commission (2011). COMMISSION REGULATION (EU) No 1129/2011, amending Annex II to Regulation (EC) No 1333/2008 of the European Parliament and of the Council by Establishing a Union List of Food Additives. Official Journal of the European Union. Brussels: European Commission.
Fernandes, A. R., Mira, N. P., Vargas, R. C., Canelhas, I., and Sá-Correia, I. (2005). Saccharomyces cerevisiae adaptation to weak acids involves the transcription factor Haa1p and Haa1p-regulated genes. Biochem. Biophys. Res. Commun. 337, 95–103. doi: 10.1016/j.bbrc.2005.09.010
Fernández-Niño, M., Marquina, M., Swinnen, S., Rodríguez-Porrata, B., Nevoigt, E., and Ariño, J. (2015). The Cytosolic pH of Individual Saccharomyces cerevisiae cells is a key factor in acetic acid tolerance. Appl. Environ. Microbiol. 81, 7813–7821. doi: 10.1128/AEM.02313-15
Galeote, V., Bigey, F., Devillers, H., Neuvéglise, C., and Dequin, S. (2013). Genome sequence of the food spoilage yeast Zygosaccharomyces bailii CLIB 213T. Genome Announc. 1, e00606–13. doi: 10.1128/genomeA.00606-13
Garay-Arroyo, A., Covarrubias, A. A., Clark, I., Nino, I., Gosset, G., and Martinez, A. (2004). Response to different environmental stress conditions of industrial and laboratory Saccharomyces cerevisiae strains. Appl. Microbiol. Biotechnol. 63, 734–741. doi: 10.1007/s00253-003-1414-4
Gasch, A. P., Spellman, P. T., Kao, C. M., Carmel-Harel, O., Eisen, M. B., Storz, G., et al. (2000). Genomic expression programs in the response of yeast cells to environmental changes. Mol. Biol. Cell 11, 4241–4257. doi: 10.1091/mbc.11.12.4241
Geng, P., Xiao, Y., Hu, Y., Sun, H., Xue, W., Zhang, L., et al. (2016). Genetic dissection of acetic acid tolerance in Saccharomyces cerevisiae. World J. Microbiol. Biotechnol. 32:145. doi: 10.1007/s11274-016-2101-9
Giannattasio, S., Guaragnella, N., Corte-Real, M., Passarella, S., and Marra, E. (2005). Acid stress adaptation protects Saccharomyces cerevisiae from acetic acid-induced programmed cell death. Gene 354, 93–98. doi: 10.1016/j.gene.2005.03.030
Giannattasio, S., Guaragnella, N., Ždralević, M., and Marra, E. (2013). Molecular mechanisms of Saccharomyces cerevisiae stress adaptation, and programmed cell death in response to acetic acid. Front. Microbiol. 4:33. doi: 10.3389/fmicb.2013.00033
González-Ramos, D., Gorter de Vries, A. R., Grijseels, S. S., van Berkum, M. C., Swinnen, S., van den Broek, M., et al. (2016). A new laboratory evolution approach to select for constitutive acetic acid tolerance in Saccharomyces cerevisiae and identification of causal mutations. Biotechnol. Biofuels 9:173. doi: 10.1186/s13068-016-0583-1
Goossens, A., de La Fuente, N., Forment, J., Serrano, R., and Portillo, F. (2000). Regulation of yeast H(+)-ATPase by protein kinases belonging to a family dedicated to activation of plasma membrane transporters. Mol. Cell. Biol. 20, 7654–7661. doi: 10.1128/MCB.20.20.7654-7661.2000
Graves, T., Narendranath, N. V., Dawson, K., and Power, R. (2006). Effect of pH and lactic or acetic acid on ethanol productivity by Saccharomyces cerevisiae in corn mash. J. Ind. Microbiol. Biotechnol. 33, 469–474. doi: 10.1007/s10295-006-0091-6
Guaragnella, N., Passarella, S., Marra, E., and Giannattasio, S. (2011). Cytochrome c Trp65Ser substitution results in inhibition of acetic acid-induced programmed cell death in Saccharomyces cerevisiae. Mitochondrion 11, 987–991. doi: 10.1016/j.mito.2011.08.007
Guaragnella, N., Zdralević, M., Antonacci, L., Passarella, S., Marra, E., and Giannattasio, S. (2012). The role of mitochondria in yeast programmed cell death. Front. Oncol. 2:70. doi: 10.3389/fonc.2012.00070
Guaragnella, N., Ždralević, M., Lattanzio, P., Marzulli, D., Pracheil, T., Liu, Z., et al. (2013). Yeast growth in raffinose results in resistance to acetic-acid induced programmed cell death mostly due to the activation of the mitochondrial retrograde pathway. Biochim. Biophys. Acta 1833, 2765–2774. doi: 10.1016/j.bbamcr.2013.07.017
Guerreiro, J. F., Mira, N. P., dos Santos, A. X., da, S., Riezman, H., and Sá-Correia, I. (2017). Membrane phosphoproteomics of yeast early response to acetic acid: role of Hrk1 kinase and lipid biosynthetic pathways, in particular sphingolipids. Front. Microbiol. 8:1302. doi: 10.3389/FMICB.2017.01302
Guerreiro, J. F., Mira, N. P., and Sá-Correia, I. (2012). Adaptive response to acetic acid in the highly resistant yeast species Zygosaccharomyces bailii revealed by quantitative proteomics. Proteomics 12, 2303–2318. doi: 10.1002/pmic.201100457
Guerreiro, J. F., Muir, A., Ramachandran, S., Thorner, J., and Sá-Correia, I. (2016a). Sphingolipid biosynthesis upregulation by TOR complex 2-Ypk1 signaling during yeast adaptive response to acetic acid stress. Biochem. J. 473, 4311–4325.
Guerreiro, J. F., Sampaio-Marques, B., Soares, R., Varela Coelho, A., Leão, C., Ludovico, P., et al. (2016b). Mitochondrial proteomics of the acetic acid -induced programmed cell death response in a highly tolerant Zygosaccharomyces bailii -derived hybrid strain. Microb. Cell 3, 65–78. doi: 10.15698/mic2016.02.477
Henderson, C. M., and Block, D. E. (2014). Examining the role of membrane lipid composition in determining the ethanol tolerance of Saccharomyces cerevisiae. Appl. Environ. Microbiol. 80, 2966–2972. doi: 10.1128/AEM.04151-13
Henriques, S. F., Mira, N. P., and Sá-Correia, I. (2017). Genome-wide search for candidate genes for yeast robustness improvement against formic acid reveals novel susceptibility (Trk1 and positive regulators) and resistance (Haa1-regulon) determinants. Biotechnol. Biofuels 10:96. doi: 10.1186/s13068-017-0781-5
Higgins, V. J., Beckhouse, A. G., Oliver, A. D., Rogers, P. J., and Dawes, I. W. (2003). Yeast genome-wide expression analysis identifies a strong ergosterol and oxidative stress response during the initial stages of an industrial lager fermentation. Appl. Environ. Microbiol. 69, 4777–4787. doi: 10.1128/AEM.69.8.4777-4787.2003
Hu, S., Fürst, P., and Hamer, D. (1990). The DNA and Cu binding functions of ACE1 are interdigitated within a single domain. New Biol. 2, 544–555.
Inaba, T., Watanabe, D., Yoshiyama, Y., Tanaka, K., Ogawa, J., Takagi, H., et al. (2013). An organic acid-tolerant HAA1-overexpression mutant of an industrial bioethanol strain of Saccharomyces cerevisiae and its application to the production of bioethanol from sugarcane molasses. AMB Express 3:74. doi: 10.1186/2191-0855-3-74
Ismail, K. S. K., Sakamoto, T., Hasunuma, T., Zhao, X.-Q., and Kondo, A. (2014). Zinc, magnesium, and calcium ion supplementation confers tolerance to acetic acid stress in industrial Saccharomyces cerevisiae utilizing xylose. Biotechnol. J. 9, 1519–1525. doi: 10.1002/biot.201300553
James, S. A., and Stratford, M. (2003). “Spoilage yeasts with emphasis on the genus Zygosaccharomyces,” in Yeasts in Food, eds T. Boekhout and V. Robert (Amsterdam: Elsevier), 171–196.
Joneja, J. V. (2003). “Benzoate intolerance,” in Dealing with Food Allergies, ed. J. V. Joneja (Boulder, CO: Bull Publishing Company), 277–287.
Jönsson, L. J., Alriksson, B., and Nilvebrant, N.-O. (2013). Bioconversion of lignocellulose: inhibitors and detoxification. Biotechnol. Biofuels 6:16. doi: 10.1186/1754-6834-6-16
Kawahata, M., Masaki, K., Fujii, T., and Iefuji, H. (2006). Yeast genes involved in response to lactic acid and acetic acid: acidic conditions caused by the organic acids in Saccharomyces cerevisiae cultures induce expression of intracellular metal metabolism genes regulated by Aft1p. FEMS Yeast Res. 6, 924–936. doi: 10.1111/j.1567-1364.2006.00089.x
Keller, G., Ray, E., Brown, P. O., and Winge, D. R. (2001). Haa1, a protein homologous to the copper-regulated transcription factor Ace1, is a novel transcriptional activator. J. Biol. Chem. 276, 38697–38702. doi: 10.1074/jbc.M107131200
Koppram, R., Albers, E., and Olsson, L. (2012). Evolutionary engineering strategies to enhance tolerance of xylose utilizing recombinant yeast to inhibitors derived from spruce biomass. Biotechnol. Biofuels 5:32. doi: 10.1186/1754-6834-5-32
Kumar, V., Hart, A. J., Keerthiraju, E. R., Waldron, P. R., Tucker, G. A., and Greetham, D. (2015). Expression of mitochondrial cytochrome C oxidase chaperone gene (COX20) improves tolerance to weak acid and oxidative stress during yeast fermentation. PLoS One 10:e0139129. doi: 10.1371/journal.pone.0139129
Lam, F. H., Ghaderi, A., Fink, G. R., and Stephanopoulos, G. (2014). Engineering alcohol tolerance in yeast. Science 346, 71–75. doi: 10.1126/science.1257859
Lee, Y., Nasution, O., Choi, E., Choi, I.-G., Kim, W., and Choi, W. (2015). Transcriptome analysis of acetic-acid-treated yeast cells identifies a large set of genes whose overexpression or deletion enhances acetic acid tolerance. Appl. Microbiol. Biotechnol. 99, 6391–6403. doi: 10.1007/s00253-015-6706-y
Lee, Y., Nasution, O., Lee, Y. M., Kim, E., Choi, W., and Kim, W. (2017). Overexpression of PMA1 enhances tolerance to various types of stress and constitutively activates the SAPK pathways in Saccharomyces cerevisiae. Appl. Microbiol. Biotechnol. 101, 229–239. doi: 10.1007/s00253-016-7898-5
Li, B.-Z., and Yuan, Y.-J. (2010). Transcriptome shifts in response to furfural and acetic acid in Saccharomyces cerevisiae. Appl. Microbiol. Biotechnol. 86, 1915–1924. doi: 10.1007/s00253-010-2518-2
Lindahl, L., Genheden, S., Eriksson, L. A., Olsson, L., and Bettiga, M. (2016). Sphingolipids contribute to acetic acid resistance in Zygosaccharomyces bailii. Biotechnol. Bioeng. 113, 744–753. doi: 10.1002/bit.25845
Lindberg, L., Santos, A. X., Riezman, H., Olsson, L., and Bettiga, M. (2013). Lipidomic profiling of Saccharomyces cerevisiae and Zygosaccharomyces bailii reveals critical changes in lipid composition in response to acetic acid stress. PLoS One 8:e73936. doi: 10.1371/journal.pone.0073936
Liu, X., Zhang, X., and Zhang, Z. (2014). Point mutation of H3/H4 histones affects acetic acid tolerance in Saccharomyces cerevisiae. J. Biotechnol. 187, 116–123. doi: 10.1016/j.jbiotec.2014.07.445
Longo, V., Ždralević, M., Guaragnella, N., Giannattasio, S., Zolla, L., and Timperio, A. M. (2015). Proteome and metabolome profiling of wild-type and YCA1-knock-out yeast cells during acetic acid-induced programmed cell death. J. Proteomics 128, 173–188. doi: 10.1016/j.jprot.2015.08.003
Ludovico, P., Rodrigues, F., Almeida, A., Silva, M. T., Barrientos, A., and Côrte-Real, M. (2002). Cytochrome c release and mitochondria involvement in programmed cell death induced by acetic acid in Saccharomyces cerevisiae. Mol. Biol. Cell 13, 2598–2606. doi: 10.1091/mbc.E01-12-0161
Ludovico, P., Sansonetty, F., Silva, M. T., and Côrte-Real, M. (2003). Acetic acid induces a programmed cell death process in the food spoilage yeast Zygosaccharomyces bailii. FEMS Yeast Res. 3, 91–96. doi: 10.1111/j.1567-1364.2003.tb00143.x
Ludovico, P., Sousa, M. J., Silva, M. T., Leão, C., and Côrte-Real, M. (2001). Saccharomyces cerevisiae commits to a programmed cell death process in response to acetic acid. Microbiology 147, 2409–2415. doi: 10.1099/00221287-147-9-2409
Ma, C., Wei, X., Sun, C., Zhang, F., Xu, J., Zhao, X., et al. (2015). Improvement of acetic acid tolerance of Saccharomyces cerevisiae using a zinc-finger-based artificial transcription factor and identification of novel genes involved in acetic acid tolerance. Appl. Microbiol. Biotechnol. 99, 2441–2449. doi: 10.1007/s00253-014-6343-x
Macpherson, N., Shabala, L., Rooney, H., Jarman, M. G., and Davies, J. M. (2005). Plasma membrane H+ and K+ transporters are involved in the weak-acid preservative response of disparate food spoilage yeasts. Microbiology 151, 1995–2003. doi: 10.1099/mic.0.27502-0
Martani, F., Marano, F., Bertacchi, S., Porro, D., and Branduardi, P. (2015). The Saccharomyces cerevisiae poly(A) binding protein Pab1 as a target for eliciting stress tolerant phenotypes. Sci. Rep. 5:18318. doi: 10.1038/srep18318
Meijnen, J.-P., Randazzo, P., Foulquié-Moreno, M. R., van den Brink, J., Vandecruys, P., Stojiljkovic, M., et al. (2016). Polygenic analysis and targeted improvement of the complex trait of high acetic acid tolerance in the yeast Saccharomyces cerevisiae. Biotechnol. Biofuels 9:5. doi: 10.1186/s13068-015-0421-x
Mira, N. P., Becker, J. D., and Sá-Correia, I. (2010a). Genomic expression program involving the Haa1p-regulon in Saccharomyces cerevisiae response to acetic acid. OMICS 14, 587–601. doi: 10.1089/omi.2010.0048
Mira, N. P., Palma, M., Guerreiro, J. F., and Sá-Correia, I. (2010b). Genome-wide identification of Saccharomyces cerevisiae genes required for tolerance to acetic acid. Microb. Cell Fact. 9:79. doi: 10.1186/1475-2859-9-79
Mira, N. P., Teixeira, M. C., and Sá-Correia, I. (2010c). Adaptive response and tolerance to weak acids in Saccharomyces cerevisiae: a genome-wide view. OMICS 14, 525–540. doi: 10.1089/omi.2010.0072
Mira, N. P., Henriques, S. F., Keller, G., Teixeira, M. C., Matos, R. G., Arraiano, C. M., et al. (2011). Identification of a DNA-binding site for the transcription factor Haa1, required for Saccharomyces cerevisiae response to acetic acid stress. Nucleic Acids Res. 39, 6896–6907. doi: 10.1093/nar/gkr228
Mira, N. P., Lourenço, A. B., Fernandes, A. R., Becker, J. D., and Sá-Correia, I. (2009). The RIM101 pathway has a role in Saccharomyces cerevisiae adaptive response and resistance to propionic acid and other weak acids. FEMS Yeast Res. 9, 202–216. doi: 10.1111/j.1567-1364.2008.00473.x
Mira, N. P., Munsterkotter, M., Dias-Valada, F., Santos, J., Palma, M., Roque, F. C., et al. (2014). The genome sequence of the highly acetic acid-tolerant Zygosaccharomyces bailii-derived interspecies hybrid strain ISA1307, isolated from a sparkling wine plant. DNA Res. 21, 299–313. doi: 10.1093/dnares/dst058
Mollapour, M., and Piper, P. W. (2007). Hog1 mitogen-activated protein kinase phosphorylation targets the yeast Fps1 aquaglyceroporin for endocytosis, thereby rendering cells resistant to acetic acid. Mol. Cell. Biol. 27, 6446–6456. doi: 10.1128/MCB.02205-06
Mollapour, M., Shepherd, A., and Piper, P. W. (2009). Presence of the Fps1p aquaglyceroporin channel is essential for Hog1p activation, but suppresses Slt2(Mpk1)p activation, with acetic acid stress of yeast. Microbiology 155, 3304–3311. doi: 10.1099/mic.0.030502-0
Olofsson, K., Palmqvist, B., and Lidén, G. (2010). Improving simultaneous saccharification and co-fermentation of pretreated wheat straw using both enzyme and substrate feeding. Biotechnol. Biofuels 3:17. doi: 10.1186/1754-6834-3-17
Orij, R., Urbanus, M. L., Vizeacoumar, F. J., Giaever, G., Boone, C., Nislow, C., et al. (2012). Genome-wide analysis of intracellular pH reveals quantitative control of cell division rate by pHc in Saccharomyces cerevisiae. Genome Biol. 13:R80. doi: 10.1186/gb-2012-13-9-r80
Ortiz-Merino, R. A., Kuanyshev, N., Braun-Galleani, S., Byrne, K. P., Porro, D., Branduardi, P., et al. (2017). Evolutionary restoration of fertility in an interspecies hybrid yeast, by whole-genome duplication after a failed mating-type switch. PLoS Biol. 15:e2002128. doi: 10.1371/journal.pbio.2002128
Paiva, S., Althoff, S., Casal, M., and Leão, C. (1999). Transport of acetate in mutants of Saccharomyces cerevisiae defective in monocarboxylate permeases. FEMS Microbiol. Lett. 170, 301–306. doi: 10.1111/j.1574-6968.1999.tb13387.x
Paiva, S., Devaux, F., Barbosa, S., Jacq, C., and Casal, M. (2004). Ady2p is essential for the acetate permease activity in the yeast Saccharomyces cerevisiae. Yeast 21, 201–210. doi: 10.1002/yea.1056
Palma, M., Dias, P. J., Roque, F. C., Luzia, L., Guerreiro, J. F., and Sá-Correia, I. (2017a). The Zygosaccharomyces bailii transcription factor Haa1 is required for acetic acid and copper stress responses suggesting subfunctionalization of the ancestral bifunctional protein Haa1/Cup2. BMC Genomics 18:75. doi: 10.1186/s12864-016-3443-2
Palma, M., Münsterkötter, M., Peça, J., Güldener, U., and Sá-Correia, I. (2017b). Genome sequence of the highly weak-acid-tolerant Zygosaccharomyces bailii IST302, amenable to genetic manipulations and physiological studies. FEMS Yeast Res. 17:fox025. doi: 10.1093/femsyr/fox025
Palma, M., Roque, F., de, C., Guerreiro, J. F., Mira, N. P., Queiroz, L., et al. (2015). Search for genes responsible for the remarkably high acetic acid tolerance of a Zygosaccharomyces bailii-derived interspecies hybrid strain. BMC Genomics 16:1070. doi: 10.1186/s12864-015-2278-6
Pampulha, M. E., and Loureiro-Dias, M. C. (1989). Combined effect of acetic acid, pH and ethanol on intracellular pH of fermenting yeast. Appl. Microbiol. Biotechnol. 31, 547–550. doi: 10.1007/BF00270792
Pampulha, M. E., and Loureiro-Dias, M. C. (1990). Activity of glycolytic enzymes of Saccharomyces cerevisiae in the presence of acetic acid. Appl. Microbiol. Biotechnol. 34, 375–380. doi: 10.1007/BF00170063
Pampulha, M. E., and Loureiro-Dias, M. C. (2000). Energetics of the effect of acetic acid on growth of Saccharomyces cerevisiae. FEMS Microbiol. Lett. 184, 69–72. doi: 10.1111/j.1574-6968.2000.tb08992.x
Phillips, A. J., Crowe, J. D., and Ramsdale, M. (2006). Ras pathway signaling accelerates programmed cell death in the pathogenic fungus Candida albicans. Proc. Natl. Acad. Sci. U.S.A. 103, 726–731. doi: 10.1073/pnas.0506405103
Piper, P., Calderon, C. O., Hatzixanthis, K., and Mollapour, M. (2001). Weak acid adaptation: the stress response that confers yeasts with resistance to organic acid food preservatives. Microbiology 147, 2635–2642. doi: 10.1099/00221287-147-10-2635
Postnikoff, S. D. L., Malo, M. E., Wong, B., and Harkness, T. A. A. (2012). The yeast forkhead transcription factors Fkh1 and Fkh2 regulate lifespan and stress response together with the anaphase-promoting complex. PLoS Genet. 8:e1002583. doi: 10.1371/journal.pgen.1002583
Rasmussen, J. E., Schultz, E., Snyder, R. E., Jones, R. S., and Smith, C. R. (1995). Acetic acid as a causative agent in producing stuck fermentations. Am. J. Enol. Vitic. 46, 278–280.
Rodrigues, F., Sousa, M. J., Ludovico, P., Santos, H., Côrte-Real, M., and Leão, C. (2012). The fate of acetic acid during glucose co-metabolism by the spoilage yeast Zygosaccharomyces bailii. PLoS One 7:e52402. doi: 10.1371/journal.pone.0052402
Rodrigues, F., Zeeman, A. M., Cardoso, H., Sousa, M. J., Steensma, H. Y., Corte-Real, M., et al. (2004). Isolation of an acetyl-CoA synthetase gene (ZbACS2) from Zygosaccharomyces bailii. Yeast 21, 325–331. doi: 10.1002/yea.1081
Roelants, F., Leskoske, K., Martinez Marshall, M. N., Locke, M., and Thorner, J. (2017). The TORC2-dependent signaling network in the yeast Saccharomyces cerevisiae. Biomolecules 7:66. doi: 10.3390/biom7030066
Roelants, F. M., Breslow, D. K., Muir, A., Weissman, J. S., and Thorner, J. (2011). Protein kinase Ypk1 phosphorylates regulatory proteins Orm1 and Orm2 to control sphingolipid homeostasis in Saccharomyces cerevisiae. Proc. Natl. Acad. Sci. U.S.A. 108, 19222–19227. doi: 10.1073/pnas.1116948108
Sá-Correia, I., Guerreiro, J. F., Loureiro-Dias, M. C., Leão, C., and Côrte-Real, M. (2014). “Zygosaccharomyces,” in Encyclopedia of Food Microbiology, eds C. A. Batt and M. L. Tortorello (Cambridge, MA: Elsevier Ltd.), 849–855. doi: 10.1016/B978-0-12-384730-0.00364-5
Sakihama, Y., Hasunuma, T., and Kondo, A. (2015). Improved ethanol production from xylose in the presence of acetic acid by the overexpression of the HAA1 gene in Saccharomyces cerevisiae. J. Biosci. Bioeng. 119, 297–302. doi: 10.1016/j.jbiosc.2014.09.004
Schüller, C., Mamnun, Y. M., Mollapour, M., Krapf, G., Schuster, M., Bauer, B. E., et al. (2004). Global phenotypic analysis and transcriptional profiling defines the weak acid stress response regulon in Saccharomyces cerevisiae. Mol. Biol. Cell 15, 706–720. doi: 10.1091/mbc.E03-05-0322
Sekito, T., Thornton, J., and Butow, R. A. (2000). Mitochondria-to-nuclear signaling is regulated by the subcellular localization of the transcription factors Rtg1p, and Rtg3p. Mol. Biol. Cell 11, 2103–2115. doi: 10.1091/mbc.11.6.2103
Simões, T., Mira, N. P., Fernandes, A. R., and Sá-Correia, I. (2006). The SPI1 gene, encoding a glycosylphosphatidylinositol-anchored cell wall protein, plays a prominent role in the development of yeast resistance to lipophilic weak-acid food preservatives. Appl. Environ. Microbiol. 72, 7168–7175. doi: 10.1128/AEM.01476-06
Sousa, M., Duarte, A., Fernandes, T. R., Chaves, S. R., Pacheco, A., Leão, C., et al. (2013). Genome-wide identification of genes involved in the positive and negative regulation of acetic acid-induced programmed cell death in Saccharomyces cerevisiae. BMC Genomics 14:838. doi: 10.1186/1471-2164-14-838
Sousa, M. J., Miranda, L., Corte-Real, M., and Leão, C. (1996). Transport of acetic acid in Zygosaccharomyces bailii: effects of ethanol and their implications on the resistance of the yeast to acidic environments. Appl. Environ. Microbiol. 62, 3152–3157.
Sousa, M. J., Rodrigues, F., Corte-Real, M., and Leão, C. (1998). Mechanisms underlying the transport and intracellular metabolism of acetic acid in the presence of glucose in the yeast Zygosaccharomyces bailii. Microbiology 144, 665–670. doi: 10.1099/00221287-144-3-665
Steels, H., James, S. A., Roberts, I. N., and Stratford, M. (2000). Sorbic acid resistance: the inoculum effect. Yeast 16, 1173–1183. doi: 10.1002/1097-0061(20000930)16:13<1173::AID-YEA617>3.0.CO;2-8
Stratford, M. (2006). “Food and beverage spoilage yeasts,” in Yeasts in Food and Beverages, eds A. Querol and G. Fleet (New York, NY: Springer-Verlag), 335–379. doi: 10.1007/978-3-540-28398-0_11
Stratford, M., Nebe-von-Caron, G., Steels, H., Novodvorska, M., Ueckert, J., and Archer, D. B. (2013a). Weak-acid preservatives: pH and proton movements in the yeast Saccharomyces cerevisiae. Int. J. Food Microbiol. 161, 164–171. doi: 10.1016/j.ijfoodmicro.2012.12.013
Stratford, M., Steels, H., Nebe-von-Caron, G., Novodvorska, M., Hayer, K., and Archer, D. B. (2013b). Extreme resistance to weak-acid preservatives in the spoilage yeast Zygosaccharomyces bailii. Int. J. Food Microbiol. 166, 126–134. doi: 10.1016/j.ijfoodmicro.2013.06.025
Sugiyama, M., Akase, S.-P., Nakanishi, R., Horie, H., Kaneko, Y., and Harashima, S. (2014). Nuclear localization of Haa1, which is linked to its phosphorylation status, mediates lactic acid tolerance in Saccharomyces cerevisiae. Appl. Environ. Microbiol. 80, 3488–3495. doi: 10.1128/AEM.04241-13
Swan, T. M., and Watson, K. (1998). Stress tolerance in a yeast sterol auxotroph: role of ergosterol, heat shock proteins and trehalose. FEMS Microbiol. Lett. 169, 191–197. doi: 10.1111/j.1574-6968.1998.tb13317.x
Swinnen, S., Henriques, S. F., Shrestha, R., Ho, P.-W., Sá-Correia, I., and Nevoigt, E. (2017). Improvement of yeast tolerance to acetic acid through Haa1 transcription factor engineering: towards the underlying mechanisms. Microb. Cell Fact. 16:7. doi: 10.1186/s12934-016-0621-5
Tanaka, K., Ishii, Y., Ogawa, J., and Shima, J. (2012). Enhancement of acetic acid tolerance in Saccharomyces cerevisiae by overexpression of the HAA1 gene, encoding a transcriptional activator. Appl. Environ. Microbiol. 78, 8161–8163. doi: 10.1128/AEM.02356-12
Teixeira, M. C., Mira, N. P., and Sá-Correia, I. (2011). A genome-wide perspective on the response and tolerance to food-relevant stresses in Saccharomyces cerevisiae. Curr. Opin. Biotechnol. 22, 150–156. doi: 10.1016/j.copbio.2010.10.011
Teixeira, M. C., Monteiro, P. T., Palma, M., Costa, C., Godinho, C. P., Pais, P., et al. (2017). YEASTRACT: an upgraded database for the analysis of transcription regulatory networks in Saccharomyces cerevisiae. Nucleic Acids Res. 46, D348–D353. doi: 10.1093/nar/gkx842
Tenreiro, S., Nunes, P. A., Viegas, C. A., Neves, M. S., Teixeira, M. C., Cabral, M. G., et al. (2002). AQR1 gene (ORF YNL065w) encodes a plasma membrane transporter of the major facilitator superfamily that confers resistance to short-chain monocarboxylic acids and quinidine in Saccharomyces cerevisiae. Biochem. Biophys. Res. Commun. 292, 741–748. doi: 10.1006/bbrc.2002.6703
Theron, M., and Lues, J. (eds) (2010). “Application of organic acids in food preservation,” in Organic Acids and Food Preservation, (Florida: CRC Press), 51–96.
Thomas, D. S., and Davenport, R. R. (1985). Zygosaccharomyces bailii — a profile of characteristics and spoilage activities. Food Microbiol. 2, 157–169. doi: 10.1016/S0740-0020(85)80008-3
Ullah, A., Chandrasekaran, G., Brul, S., and Smits, G. J. (2013). Yeast adaptation to weak acids prevents futile energy expenditure. Front. Microbiol. 4:142. doi: 10.3389/fmicb.2013.00142
Ullah, A., Orij, R., Brul, S., and Smits, G. J. (2012). Quantitative analysis of the modes of growth inhibition by weak organic acids in Saccharomyces cerevisiae. Appl. Environ. Microbiol. 78, 8377–8387. doi: 10.1128/AEM.02126-12
Vilela-Moura, A., Schuller, D., Mendes-Faia, A., Silva, R. D., Chaves, S. R., Sousa, M. J., et al. (2011). The impact of acetate metabolism on yeast fermentative performance and wine quality: reduction of volatile acidity of grape musts and wines. Appl. Microbiol. Biotechnol. 89, 271–280. doi: 10.1007/s00253-010-2898-3
Vyas, V. K., Berkey, C. D., Miyao, T., and Carlson, M. (2005). Repressors Nrg1 and Nrg2 regulate a set of stress-responsive genes in Saccharomyces cerevisiae. Eukaryot. Cell 4, 1882–1891. doi: 10.1128/EC.4.11.1882-1891.2005
Wan, C., Zhang, M., Fang, Q., Xiong, L., Zhao, X., Hasunuma, T., et al. (2015). The impact of zinc sulfate addition on the dynamic metabolic profiling of Saccharomyces cerevisiae subjected to long term acetic acid stress treatment and identification of key metabolites involved in the antioxidant effect of zinc. Metallomics 7, 322–332. doi: 10.1039/c4mt00275j
Wright, J., Bellissimi, E., de Hulster, E., Wagner, A., Pronk, J. T., and van Maris, A. J. A. (2011). Batch and continuous culture-based selection strategies for acetic acid tolerance in xylose-fermenting Saccharomyces cerevisiae. FEMS Yeast Res. 11, 299–306. doi: 10.1111/j.1567-1364.2011.00719.x
Wu, X., Zhang, L., Jin, X., Fang, Y., Zhang, K., Qi, L., et al. (2016). Deletion of JJJ1 improves acetic acid tolerance and bioethanol fermentation performance of Saccharomyces cerevisiae strains. Biotechnol. Lett. 38, 1097–1106. doi: 10.1007/s10529-016-2085-4
Zahn, K., and Jacobson, S. (2015). Acetate resistance in yeast based on introduction of a mutant HAA1 allele. U.S. Patent No. US9085781 B2.
Ždralević, M., Guaragnella, N., Antonacci, L., Marra, E., and Giannattasio, S. (2012). Yeast as a tool to study signaling pathways in mitochondrial stress response, and Cytoprotection. Sci. World J. 2012, 1–10. doi: 10.1100/2012/912147
Zhang, M.-M., Zhao, X.-Q., Cheng, C., and Bai, F.-W. (2015). Improved growth and ethanol fermentation of Saccharomyces cerevisiae in the presence of acetic acid by overexpression of SET5 and PPR1. Biotechnol. J. 10, 1903–1911. doi: 10.1002/biot.201500508
Keywords: Saccharomyces cerevisiae, Zygosaccharomyces bailii, weak acid food preservatives, acetic acid adaptive response, acetic acid tolerance, physiological genomics
Citation: Palma M, Guerreiro JF and Sá-Correia I (2018) Adaptive Response and Tolerance to Acetic Acid in Saccharomyces cerevisiae and Zygosaccharomyces bailii: A Physiological Genomics Perspective. Front. Microbiol. 9:274. doi: 10.3389/fmicb.2018.00274
Received: 13 December 2017; Accepted: 06 February 2018;
Published: 21 February 2018.
Edited by:
Jean Marie François, UMR5504 Laboratoire d’Ingénierie des Systèmes Biologiques et des Procédés (LISBP), FranceReviewed by:
Sergio Giannattasio, Istituto di Biomembrane, Bioenergetica e Biotecnologie Molecolari (IBIOM), ItalyGemma Beltran, Universitat Rovira i Virgili, Spain
Copyright © 2018 Palma, Guerreiro and Sá-Correia. This is an open-access article distributed under the terms of the Creative Commons Attribution License (CC BY). The use, distribution or reproduction in other forums is permitted, provided the original author(s) and the copyright owner are credited and that the original publication in this journal is cited, in accordance with accepted academic practice. No use, distribution or reproduction is permitted which does not comply with these terms.
*Correspondence: Isabel Sá-Correia, aXNhY29ycmVpYUB0ZWNuaWNvLnVsaXNib2EucHQ=