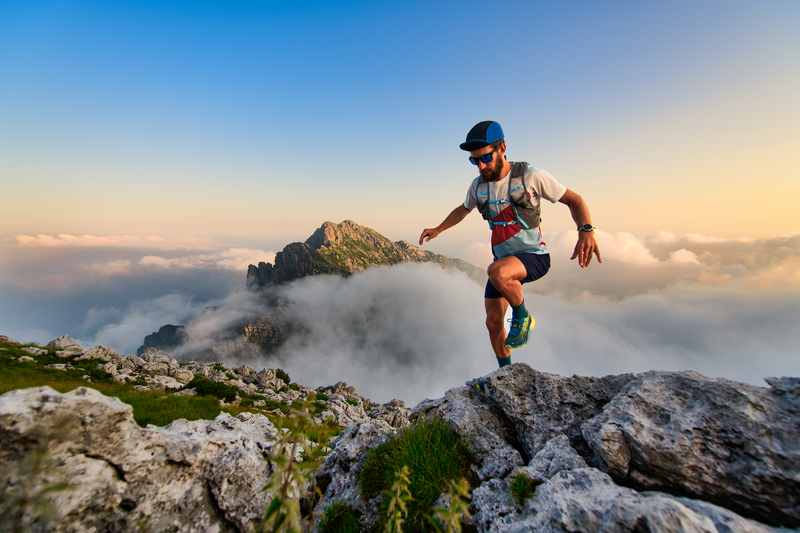
95% of researchers rate our articles as excellent or good
Learn more about the work of our research integrity team to safeguard the quality of each article we publish.
Find out more
ORIGINAL RESEARCH article
Front. Microbiol. , 16 February 2018
Sec. Microbiotechnology
Volume 9 - 2018 | https://doi.org/10.3389/fmicb.2018.00252
Argimycins P are a recently identified family of polyketide alkaloids encoded by the cryptic gene cluster arp of Streptomyces argillaceus. These compounds contain either a piperideine ring, or a piperidine ring which may be fused to a five membered ring, and a polyene side chain, which is bound in some cases to an N-acetylcysteine moiety. The arp cluster consists of 11 genes coding for structural proteins, two for regulatory proteins and one for a hypothetical protein. Herein, we have characterized the post-piperideine ring biosynthesis steps of argimycins P through the generation of mutants in arp genes, the identification and characterization of compounds accumulated by those mutants, and cross-feeding experiments between mutants. Based in these results, a biosynthesis pathway is proposed assigning roles to every arp gene product. The regulation of the arp cluster is also addressed by inactivating/overexpressing the positive SARP-like arpRI and the negative TetR-like arpRII transcriptional regulators and determining the effect on argimycins P production, and through gene expression analyses (reverse transcription PCR and quantitative real-time PCR) of arp genes in regulatory mutants in comparison to the wild type strain. These findings will contribute to deepen the knowledge on the biosynthesis of piperidine-containing polyketides and provide tools that can be used to generate new analogs by genetic engineering and/or biocatalysis.
Alkaloids are a group of natural products synthesized by plants, animals, and microorganisms that show a broad structural diversity and the presence of a basic nitrogen atom as a common feature (Cushnie et al., 2014; Sigrist et al., 2015). This family of compounds shows significant bioactivity, in some cases beneficial (antimalarial, antitumor, antiasthma, antihypertensive, anti-arrhythmic, analgesic), but in others harmful for humans and animals (Cushnie et al., 2014; Sigrist et al., 2015). In addition, they seem to play different roles in producer organisms. In microorganisms, alkaloids have been involved in growth and colony development, self-defense or as autoinducers (Heeb et al., 2011; Cushnie et al., 2014; Ye et al., 2017). Attending to the chemical structure there are two classes of alkaloids: heterocyclic or typical alkaloids and non-heterocyclic/atypical/protoalkaloids bearing nitrogen atoms in a side chain (Cushnie et al., 2014). Some of the most important alkaloids contain a piperidine ring, and in the so called true alkaloids these rings derive from amino acids. However, some piperidine-containing alkaloids or piperidine pseudoalkaloids from polyketide origin acquire their nitrogen atoms via transamination reactions (Sigrist et al., 2015).
Actinomycetes are a group of bacteria that have attracted the attention of academic and industrial researchers, because they produce a plethora of natural products displaying antibiotic, antitumor, immunosuppressant or anthelmintic activities (Bérdy, 2012; Newman and Cragg, 2016). Most of these compounds are peptides (ribosomal and non-ribosomal synthesized), polyketides or terpenes. Particularly, several piperidine-containing alkaloids of polyketide origin have been isolated from actinomycetes, such as coelimycin P (Gómez-Escribano et al., 2012), cyclizidine (Freer et al., 1982), iromycins (Surup et al., 2007), latumcidin (also known as abikoviromycin; Seto et al., 1973), piericidins (Zhou and Fenical, 2016), strepchazolins (Yang et al., 2017), streptazolins (Mayer and Thiericke, 1993; Puder et al., 2001), streptazones A–D (Puder et al., 2000), streptazone E (Liu et al., 2013), or streptopyridines (Groenhagen et al., 2014). These compounds show a range of activities such as antimicrobial, antiviral, cytotoxic, decreasing blood pressure and cholesterol biosynthesis, or analgesic (Umezawa et al., 1951; Terashima et al., 1970; Grabley et al., 1991; Puder et al., 2001). However, information about their biosynthesis pathways is quite scarce. Thus, only the biosynthesis gene clusters for coelimycin P, cyclizidine and streptazone E have been identified so far (Gómez-Escribano et al., 2012; Huang et al., 2015; Ohno et al., 2015). Biosynthesis of these compounds involves a Type I or modular polyketide synthase (PKS). These are macroenzyme complexes typically organized into modules, which are responsible for a single elongation cycle and contains a β-ketoacyl synthase (KS), an acyltransferase (AT) and an acyl carrier protein (ACP) domain. In addition, these modules can contain optional domains such as ketoreductase (KR), dehydratase (DH) or enoyl reductase (ER) that modify the β-keto group after an elongation event (Weissman, 2015).
Argimycins P (Figure 1A) comprise a group of polyketide alkaloid compounds produced by Streptomyces argillaceus ATCC 12956 that show either a piperideine ring, or a piperidine ring fused or not with a five-membered ring, and a polyene side chain. In addition, some argimycins P contain an N-acetylcysteine moiety. Very recently, we have reported the isolation and chemical characterization of these compounds that seem to play some role in both growth and colony development in the producer strain (Ye et al., 2017). In addition, we also reported the identification of the argimycins P biosynthesis gene cluster arp and showed that biosynthesis of nigrifactin, a putative intermediate containing a piperideine ring, requires the ArpP PKS and the ArpN aminotransferase (Ye et al., 2017). Herein we describe new insights into the argimycin P biosynthesis pathway through the inactivation of arp genes putatively involved in post-piperideine ring formation biosynthesis steps, the identification and characterization of the compounds produced by each mutant, and cross-feeding experiments between mutants. In addition, we also address the regulation of argimycins P biosynthesis by studying the expression pattern of the arp genes in mutants in specific regulatory genes in comparison with the wild type strain.
FIGURE 1. Chemical structures of argimycins P previously identified in Streptomyces argillaceus wild type strain (A) and new ones identified in the wild type strain and/or in arp mutants (this work) (B). Relative configuration is shown for argimycins PI, PII, PIV, PV, PVI, PX, PXI, and PXII.
Streptomyces argillaceus ATCC 12956 was used as source of DNA to generate mutants and for argimycins P production experiments. The argimycin P-non producers S. argillaceus MARPPIII (PKS-minus mutant), S. argillaceus MARPN (aminotransferase-minus mutant), S. argillaceus DARPO-HII (mutant with a deletion from arpO to arpHII), S. argillaceus MARPRI (positive regulatory arpRI-minus mutant) and S. argillaceus MARPRII (negative regulatory arpRII-minus mutant) (Table 1) (Ye et al., 2017) were used in co-synthesis and/or bioconversion experiments, and to quantify argimycins P production. Escherichia coli DH10B (Invitrogen) and E. coli ET12567/pUB307 (Kieser et al., 2000) were used as cloning hosts for plasmid propagation and for conjugation experiments, respectively. MA and SM10 media (Fernández et al., 1998; Ye et al., 2017) were used for sporulation and argimycins P production by S. argillaceus, respectively. When required, antibiotics were added to media at the following final concentrations: ampicillin (100 μg/mL), kanamycin (50 μg/mL), nalidixic acid (25 μg/mL), apramycin (25 μg/mL), and thiostrepton (50 μg/mL). Plasmids pCRBlunt (Invitrogen) and pUO9090 (M. C. Martín, unpublished results) were used for subcloning. Plasmid pHZ1358 (Sun et al., 2002) was used for generating mutants by gene replacement. pIAGO (Aguirrezabalaga et al., 2000), pSETE (I. García, personal communication), pSETec and pSETeTc (Cano-Prieto et al., 2015) were used for complementing mutants and overexpressing arp genes. DNA manipulations, intergeneric conjugations and transformations were carried out according to standard procedures for Streptomyces (Kieser et al., 2000) and for E. coli (Sambrook and Russell, 2001). PCR amplifications were done using Herculase (Stratagene) and 5% dimethyl sulfoxide (DMSO). Purified amplicons were sequenced and compared to others in databases. Sequence analyses were carried out using BLAST (Altschul et al., 1997).
Mutants in specific arp genes were generated by replacing most of the corresponding genes by an apramycin resistance cassette that was inserted in the same direction of transcription (Table 1). Accordingly, several plasmids were constructed in pHZ1358 by PCR amplifying DNA regions flanked the target gene using oligonucleotides described in Supplementary Table S1, and by inserting an apramycin resistance cassette between those fragments (see Supplementary Material). Amplified DNA fragments were checked by DNA sequencing. The resultant constructs were introduced in S. argillaceus and apramycin-resistant, thiostrepton-sensitive transconjugants were selected to identify mutants in which the wild type copy of the target gene was replaced by the mutated one. These mutants were confirmed by PCR amplification of the mutated region using oligonucleotides from Supplementary Table S1, followed by sequencing of the amplified DNA fragments.
Several plasmids were constructed to complement different S. argillaceus arp mutants (Table 1). To this aim, single arp genes were amplified using the corresponding oligonucleotides from Supplementary Table S2, and subcloned under the control of the erythromycin resistance promoter. arpHI, arpHII, arpO, and arpK were amplified as SpeI-XbaI DNA fragments and subcloned into the XbaI site of pIAGO; arpDHII was also amplified as a SpeI-XbaI DNA fragment but subcloned into the XbaI site of pSETeTc; arpDHI was amplified as a NheI-XbaI DNA fragment and subcloned into the XbaI site of pIAGO.
Plasmids pSETEcRI and pSETERII were constructed to independently overexpress regulatory genes arpRI and arpRII, respectively, in the wild type strain (Table 1). To construct pSETEcRI, arpRI was amplified using oligonucleotides SARP_ATG_Abisbis/378SARP_B (Supplementary Table S2), subcloned into pCR-Blunt, and the fragment released as an EcoRI fragment (using these sites from the vector) to be subcloned into the same site of pSETec, downstream of the erythromycin resistance promoter. To construct pSETERII, arpRII was amplified as a BamHI DNA fragment using oligonucleotides SA1701orf4A/SA1701orf4B (Supplementary Table S2) and subcloned into the BamHI site of pSETE, downstream of the erythromycin resistance promoter.
Argimycins P were extracted with n-butanol and analyzed by reversed-phase chromatography as previously reported (Ye et al., 2017). Chromatograms were recorded at 400, 272, and 230 nm. For purification purposes, S. argillaceus mutant strains were grown by a two-step culture method as previously described (Fernández et al., 1998), using forty 250-mL Erlenmeyer flasks in the production step. Purification of new argimycins P was carried out as previously described (Ye et al., 2017), using isocratic chromatography conditions optimized for each compound. Structures of new compounds were characterized by LC/MS (liquid chromatography mass spectrometry) and Nuclear Magnetic Resonance (NMR) analyses. A combination of 1D (1H and 13C), and 2D (1H-1H COSY, TOCSY, HSQC and HMBC) analyses were carried for structural elucidation by NMR.
For co-synthesis analyses, 100 μl of seed cultures from each strain were used to inoculate 3 ml of SM10 medium contained into a well of a 24-square deep-well plate. After 24 h of growth, co-synthesis cultures were prepared by combining cultures from two individual cultures (1.5 ml from each) into a well. Samples were harvested after 0, 24, and 48 h of incubation.
For bioconversion experiments with nigrifactin, cultures of the different mutant strains in SM10 medium were fed with nigrifactin (0.1 mM final concentration). Samples were harvested after 24 h of incubation.
Total RNA was obtained from S. argillaceus wild type and S. argillaceus mutants MARPRI and MARPRII as previously described (Flórez et al., 2015). This RNA was used as template for gene expression analysis by reverse transcriptase-PCR (RT-PCR) and real time PCR (qPCR). Qualitative gene expression was studied using the SuperScript®IIITM One-Step RT-PCR system with Platinum® Taq DNA polymerase (Invitrogen). cDNA synthesis was performed using 50 ng or 100 ng of total RNA as template at 50°C for 30 min, followed by heating at 94°C for 2 min. Sample mixtures included 32.2 U of RNA-guard RNase inhibitor (Amersham Biosciences). Amplification conditions were as follows: 33 cycles of 98°C for 15 s; 62°C for 45 s; 72°C for 1 min; and a final extension step at 72°C for 10 min. Oligonucleotides (Supplementary Table S3) were designed within every arp gene to produce cDNAs of approximately 350–550 bp. Primers for arpRI and arpRII were designed to amplify an internal DNA region located upstream of the inserted apramycin resistance cassette. In the case of arpRII the cDNA product was of approximately 150 bp. The identity of these fragments was verified by direct sequencing. Negative controls for each pair of primers were carried out with Platinum Taq DNA polymerase (Invitrogen) in the absence of reverse transcriptase. hrdB gene expression levels were used to normalize RNA concentration of the tested strains. The RT-PCR products were resolved by 1.4% agarose gel electrophoresis stained with ethidium bromide and visualized using a Gel Doc analyzer (Bio-Rad).
qPCR was used to quantify gene expression of selected genes in mutants MARPRI and MARPRII, in relation to the wild type strain. A total amount of 0.6 μg of RNA was used to synthesize cDNA using the AffinityScript QPCR cDNA synthesis kit (Bio-Rad). qPCRs were carried out on an Stratagene Mx3000P (Agilent Technologies), with a Brilliant II SYBR® green QPCR master mix (Agilent Technologies). Triplicate PCR reactions were carried out for each sample analyzed. hrdB was used as housekeeping gene in each sample in order to standardize the results by eliminating variation in RNA and cDNA quantity and quality. Absence of chromosomal DNA contamination was checked by qPCR. Primers (Supplementary Table S3) were designed using the algorithms provided by Primer Express software 2.0 (Applied Biosystems), and their efficiency was calculated based on the slope of a standard curve. To determine amplification specificity an additional dissociation curve analysis was performed after the last cycle, showing in all cases one single peak. PCR results were given as the increase in the fluorescence signal of the reporter dye detected and visualized by the MxPro Software provided with the version 4.1 (Agilent Technologies). Changes in gene expression are represented with respect to the control sample (wild-type strain).
The arp gene cluster contains 14 genes: 11 for structural proteins (arpDHI, arpDHII, arpHI, arpHII, arpK, arpN, arpO, arpPI, arpPII, arpPIII, and arpT), two coding for regulatory proteins (arpRI and arpRII), and one for a hypothetical protein (arpX). We have previously shown that formation of the piperideine ring of argimycins P is achieved by expressing the arpPI, arpPII, and arpPIII genes that encode a hexamodular PKS and the arpN aminotransferase gene (Ye et al., 2017). In order to obtain further insights into argimycin P biosynthesis and to assign functions to other arp coding genes, a set of gene-knockout experiments were carried out, followed by metabolic profile analyses. Mutants were obtained by replacing the wild type copy of the gene in the S. argillaceus chromosome by an in vitro mutated one. Several constructs in the unstable plasmid pHZ1358 were generated, in which most of the target arp gene was replaced by an apramycin resistance cassette inserted in the direction of transcription of the targeted gene. The resultant constructs were introduced into S. argillaceus, and apramycin-resistant thiostrepton-sensitive transconjugants were selected. One mutant for each inactivated gene was selected for further characterization. The occurrence of a double recombination event in each mutant strain was genetically verified by PCR analyses using proper oligoprimers (Supplementary Table S1). In order to determine that no other gene except the target gene was affected, in trans complementation of each mutant was carried out using plasmids expressing the corresponding gene and by confirming the recovery of argimycins P production (Table 1 and Supplementary Figure S1). The mutants were further characterized for production of argimycins P (or analogs) by Ultra Performance Liquid Chromatography (UPLC) and HPLC-MS analyses, and the new compounds accumulated by the mutants purified and characterized.
The arp gene cluster contains two contiguous genes transcribed in the same direction (arpDHI and arpDHII) that code for dehydrogenases: ArpDHI is similar to acyl-CoA dehydrogenases, and it has been hypothesized to be involved in the hydroxylation of the piperidine ring and/or in the reduction of the C7–C8 double bond of the polyene chain during argimycins P biosynthesis (Ye et al., 2017). ArpDHII is similar to 6-phosphogluconate dehydrogenases, and it has been proposed to be an imine reductase catalyzing the reduction of the imine group (Ye et al., 2017). Using pHZMutorf6 and pHZMutorf7long, the wild type copy of arpDHI and arpDHII were replaced by an apramycin resistance cassette, generating mutants MARPDHI and MARPDHII, respectively (Table 1 and Supplementary Figures S2, S3). Analysis of cultures of mutant MARPDHI (Figure 2) revealed production of compounds containing a single piperideine or a piperidine ring (nigrifactin and argimycin PIX; Figure 1A) and the disappearance of argimycins P compounds containing the five-membered ring (Figure 1A). Further analysis of this mutant showed that it also produced argimycins PVII and PVIII (peak 3∗ in Figure 2; see below). These results indicated that ArpDHI is involved in an early biosynthesis step of argimycins P prior to the five-membered ring formation. Since argimycin PVIII and PIX are produced by mutant MARPDHI, this ruled out ArpDHI from the reduction of the C7–C8 double bond of the polyene side chain and in the reduction of the imine.
FIGURE 2. UPLC chromatograms of butanol extracts of S. argillaceus wild type (WT) and of arp mutant strains. Chromatograms are shown at 400 nm (A), 272 nm (B), and 230 nm (C). Peaks corresponding to the different argimycins P are indicated as follows: argimycins PI and PII (I and II); nigrifactin (N); argimycin PIV (IV); argimycin PV (V); argimycin PVI (VI); argimycin PIX (IX). Peaks 1–4 contain new argimycins P identified in this work: argimycin PX (1); argimycin PXI (2); argimycin PVII and argimycin PVIII (3∗); argimycin PVII, argimycin PVIII and argimycin PXII (3); argimycin PXIV (4).
Cultures of MARPDHII contained traces of unidentified argimycin P-like compounds and a small amount of nigrifactin (Figure 2B). This result reinforced the hypothesis of ArpDHII acting in an early biosynthesis step as an imine reductase, most likely before ArpDHI. Based on this result, bioconversions experiments were designed to confirm this activity. Accordingly, nigrifactin which contains an imine group was fed to cultures of the argimycin P non-producer S. argillaceus MARPPIII (PKS-minus mutant) to determine if nigrifactin could be converted to other argimycins P. After 24 h of incubation, a new peak was detected (peak VII in Figure 3) that showed the same retention time, mass and absorption spectrum as argimycin PVII (Figure 1B; see below). This bioconversion indicated that the imine group in nigrifactin is reduced to the amino group in argimycin PVII. Since none of the argimycins P bearing the five-membered ring was detected, this result also suggested that neither nigrifactin nor argimycin PVII were biosynthetic intermediates for the formation of the five-membered ring compounds. To determine if the imine reductase coding gene was an arp gene, nigrifactin was fed to S. argillaceus DARPO-HII (a mutant with a deletion from arpO to arpHII; Table 1). Argimycin PVII could not be detected (Figure 3), indicating that one of the arp deleted genes in this mutant was responsible for reduction of the imine. Finally, to identify this arp gene, nigrifactin was fed to S. argillaceus DARPO-HII expressing arpDHII (S. argillaceus DARPO-HII-pSETETorf7; Table 1). In this case, argimycin PVII was detected (Figure 3). These results confirmed the function of ArpDHII as the imine reductase involved in argimycins P biosynthesis.
FIGURE 3. UPLC chromatograms of butanol extracts of bioconversion products of nigrifactin (N) using different S. argillaceus mutant strains. Chromatograms are shown at 272 nm. Peak VII corresponds to argimycin PVII.
arpHI and arpHII are two small genes convergently transcribed that code for proteins belonging to the NTF2_like superfamily, which have been proposed to be cyclases (Ye et al., 2017). Using pHZMutorf9a and pHZMutorf9b, each gene was individually mutated by replacing the corresponding wild type copies by apramycin resistance cassettes, generating mutants MARPHI and MARPHII, respectively (Table 1 and Supplementary Figures S4, S5). The metabolite profiles from both mutants were similar (Figure 2): they only produced nigrifactin and argimycin PIX. In addition, two new peaks, absent in the wild type strain, were detected at 272 nm (peak 1; Figure 2B) and 230 nm (peaks 1 and 2; Figure 2C), with retention times of 3.04 and 3.13 min, respectively. Peak 2 contained a compound displaying a maximum at ca. 228 nm in the UV (DAD) spectrum that was identical to the previously characterized argimycin PIX (Ye et al., 2017). After purification of this compound its molecular formula was established as C12H19NO based on the pseudomolecular ion peak [M+H]+ observed at 194.1541 (calcd. for C12H20NO+ = 194.1539). NMR analysis revealed the presence of an epoxide, which accounted for the extra oxygen and degree of unsaturation (Supplementary Figures S6, S7, S10, and Supplementary Table S4). This new compound was named argimycin PXI (Figure 1B). On the other hand, peak 1 contained two compounds with different masses (m/z values of 192 [M+H]+ and 194 [M+H]+). Importantly, levels of the lower-mass compound greatly decreased when cultures were incubated in the dark, which enabled the purification of the higher-mass compound by cultivating MARPHII in darkness. NMR analysis confirmed it as having a structure equal to argimycin PXI but with Z stereochemistry in the middle double bond of the side chain (Supplementary Figures S8–S10 and Table S5). This new compound was named argimycin PX (Figure 1B). In addition, cultures of MARPHI and MARPHII revealed another peak eluting around 3.5 min (peak 3; Figures 2B,C). Analysis of peak 3 by HPLC-MS showed three compounds with different masses (178 [M+H]+, 180 [M+H]+ and 230 [M+H]+). The lowest-mass compound (178 [M+H]+) differed only in two units from nigrifactin (176 [M+H]+), and it was hypothesized that this compound (named argimycin PVII) could be an analog of nigrifactin bearing an amino rather than an imine group. To prove this, nigrifactin was subjected to chemical reduction with sodium borohydride (NaBH4). Thus, after 1 h of reaction nigrifactin was completely consumed and a new compound appeared with the same retention time and mass (178 [M+H]+) as argimycin PVII (Figure 4). NMR analyses (Supplementary Figures S11, S12 and Supplementary Table S6) confirmed the structure of argimycin PVII (Figure 1B). This compound was previously reported by Ohno et al. (2015). To enable isolation of the other two compounds from peak 3, MARPHII was cultivated on darkness since the production of argimycin PVII greatly decreased under these conditions. Accordingly, a sample enriched in the two other compounds was purified. The compound with the highest mass, the most abundant, showed a molecular formula of C12H20ClNO based on the pseudomolecular ion peak [M+H]+ observed at 230.1307 (calcd. for C12H21ClNO+ = 230.1306). NMR elucidation revealed a chlorohydrin structurally related to the epoxide found in argimycin PXI (Supplementary Figures S10, S13, S14 and Supplementary Table S8). This new compound was named argimycin PXII (Figure 1B). Regarding the minor compound, the molecular formula was established as C12H21N based on the pseudomolecular ion peak [M+H]+ observed at 180.1748 (calcd. for C12H22N+ = 180.1747) that was identical to argimycin PIX (Ye et al., 2017), and NMR analyses (Supplementary Figures S13, S14 and Supplementary Table S7) confirmed that this compound, which was named argimycin PVIII (Figure 1B), is a geometric isomer of argimycin PIX. On the other hand, careful analysis of cultures from the wild type strain and from some other arp mutants showed that argimycin PVII and PVIII but not argimycin PXII, were identified in the metabolite profiles of these strains (peak 3∗, Figures 2B,C). All these results confirmed that MARPHI and MARPHII are blocked before the cyclization event that leads to the formation of the five-membered ring.
FIGURE 4. Chemical reduction of nigrifactin with sodium borohydride (NaBrH4). UPLC chromatogram and UV/vis spectrum of nigrifactin before (A) and after (B) the chemical reduction. N, nigrifactin; VII, argimycin PVII. Chromatograms are shown at 272 nm.
arpO is divergently transcribed from arpDHI and arpDHII. It shows high similarity to several putative oxidases including amino oxidases, and contains domains present in dehydrogenases proteins. Using pHZMutorf5 most of the wild type copy of arpO was replaced by an apramycin resistance cassette, generating mutant MARPO (Table 1 and Supplementary Figure S15). Analysis of the metabolite profile produced by MARPO revealed that it produced all argimycins P except argimycins PI and PII (Figure 2). These compounds differ from other argimycins P with two rings by containing a pyridine instead of a piperidine ring and by the presence of an N-acetylcysteine residue attached to the polyene chain (Figure 1A). Moreover, two new peaks were detected in cultures of MARPO at 276 nm (Figure 2B), which eluted at 2.75 and 3.15 min, respectively. The early peak contained a single compound that was too unstable to be purified. The late peak (peak 4 in Figure 2B) was purified and showed a maximum at 288 nm in the UV/vis spectrum. The HRMS information rendered a molecular formula of C12H17N based on the observed ion [M+H]+ at 176.1433 (calcd. for C12H18N+ = 176.1434). Such formula was identical to that of argimycin PVI. Elucidation of the structure was assisted by comparing its NMR data (Supplementary Figures S16–S18, and Supplementary Table S9) to those from argimycins PIV, PV and PVI (Ye et al., 2017). The proposed structure for this new compound, named argimycin PXIV (Figure 1B), was similar to that of argimycin PVI (Figure 1A) but differing in the position of the double bond, which was between C4–C4a in the former and between C6–C7 in the latter. These results suggest that ArpO could be a dehydrogenase involved in the oxidation of the piperidine ring leading to the pyridine ring.
ArpK is highly similar to flavin reductases and its coding gene is located upstream of arpHI. A mutant by gene replacement was generated in this gene (MARPK) using pHZMutorf9 (Table 1 and Supplementary Figure S19). This mutant showed a similar metabolite profile as the wild type strain, but argimycins PI/PII and argimycin PIV were not detected (Figures 2A,B). This result supports the function of ArpK as regenerating flavin nucleotides that could be used by ArpO.
Downstream of arpRII is located arpX that codes for a protein of unknown function. A mutant by gene replacement was generated using pHZMutorf14 (Table 1 and Supplementary Figure S20). Analysis of the metabolite profile of the resultant mutant MARPX showed that it produced diminished amounts of all argimycins P (Figure 2).
In order to elucidate the order of the biosynthesis steps in argimycin P pathway, co-synthesis experiments were carried out between every mutant in arp structural gene and MARPPIII (PKS-minus mutant). No argimycin P containing two rings were detected when MARPN, MARPDHI, or MARPDHII were co-cultivated with MARPPIII (data not shown). These results indicated that either compounds accumulated by these mutants were not diffusible or unable to enter in the cell, or they were not real biosynthesis intermediates. However, when MARPHI or MARPHII were co-cultivated with MARPPIII, formation of argimycin PV and argimycin PVI was detected, while none of these compounds were produced by any of these mutants when grown individually (Figure 5A). This indicated that these mutants accumulated biosynthesis intermediates that were modified by MARPPIII leading to the formation of five-membered ring compounds. However, co-cultivation between MARPHI and MARPHII did not affect argimycin P production profiles (data not shown). These results confirm that ArpN, ArpDHI, and ArpDHII were involved in earlier biosynthesis steps, before formation of the five-membered ring, and strongly support that ArpHI and ArpHII would cooperate in the cyclization reaction leading to the formation of the five-membered ring. On the other hand, by co-cultivating MARPO with MARPPIII production of argimycin PI/PII was recovered, indicating that MARPO accumulated biosynthesis intermediates that can be modified by an arpO expressing strain resulting in the formation of these two argimycins P (Figure 5B).
FIGURE 5. UPLC chromatograms of butanol extracts of co-synthesis products between S. argillaceus MARPPIII and different arp mutants. Chromatograms are shown at 272 nm (A) and 400 nm (B).
Based on all these results, we propose a model for the biosynthesis of argimycins P that can be divided into several stages (Figure 6). In the initial steps the piperideine ring would be synthesized. The ArpPI, ArpPII, and ArpPIII PKS would render the thioester hexaketide 1 according to the specificities of the PKS catalytic domains (Supplementary Figure S21). This polyketide, similar to cyclizidine (Peng et al., 2016) and coelimycin P (Awodi et al., 2017) biosynthesis pathways, would be reductively released by the thioester reductase domain present in the PKS subunit ArpPIII to render the putative aldehyde 2. Then, transamination by aminotransferase ArpN of the aldehyde group of the released polyketide chain would occur rendering the hypothetical product 3, followed by its non-enzymatic cyclization to generate the putative biosynthesis intermediate 4 with a piperideine ring. Spontaneous dehydration and reduction of 4 would occur to provide nigrifactin.
FIGURE 6. Proposed biosynthesis pathway for argimycins P. R1 = OH and R2 = OH, argimycin PIV; R1 = H and R2 = OH, argimycin PV; R1 = H and R2 = H, argimycin PVI.
The next step would be the formation of the piperidine ring. This is supported by the fact that all mutants in arp genes except MARPDHII accumulate compounds with a piperidine ring, which suggests the reduction of the imine group should be an early biosynthesis step. Most probably, this reaction would occur following the transamination reaction as it has been recently reported in the cyclizidine biosynthesis pathway (Peng et al., 2016). ArpDHII is proposed as a candidate to carry out this imine reduction step. Unlike the proposed streptazone E biosynthesis pathway (Ohno et al., 2015), neither nigrifactin nor argimycin PVII, PVIII, and PIX seem to be intermediates in the biosynthesis of argimycins P containing two rings, since recovery of its production was not achieved either in co-synthesis experiments between mutants (e.g., MARPDHI or MARPDHII vs. MARPPIII), or in bioconversion experiments with nigrifactin by mutant MARPPIII. Therefore, formation of nigrifactin and its reduction to argimycin PVII would rather form part of a biosynthesis side pathway leading to argimycin PVIII and PIX, while a different intermediate should be used in the main pathway leading to the formation of two rings-argimycins P. That intermediate could be the putative biosynthesis intermediate 4, that after reduction by ArpDHII would generate the putative intermediate 5. This compound has not been identified so far in cultures of S. argillaceus wild type or any arp mutant, however, structurally related compounds have been identified in other Streptomyces strains that also produce piperidine polyketide compounds (Puder et al., 2000; Groenhagen et al., 2014).
Five-membered ring cyclization would be preceded by formation of biosynthesis intermediates containing epoxy groups. This type of compounds (argimycin PX and PXI) is only produced by mutants MARPHI and MARPHII. Since these two mutants co-synthesize with mutant MARPPIII, it is deduced that compounds accumulated by these mutants are intermediates for the biosynthesis of argimycins P containing two rings. It is proposed that ArpDHI would be involved in the formation of these epoxide containing compounds by oxidizing a putative intermediate with a double bond generated by spontaneous dehydration of compound 5. The next stage would be the five-membered ring formation. According to our results and similarly to the streptazone E biosynthesis pathway (Ohno et al., 2015), this cyclization event could be coupled to ring-opening of the epoxide, and would be carried out in a cooperative manner by ArpHI and ArpHII.
The final biosynthesis step would be formation of argimycins PI/PII that contain a pyridine ring and an N-acetylcysteine moiety. It is proposed a role of ArpO (and ArpK) in formation of these compounds by oxidizing a biosynthesis intermediate accumulated by MARPO, including oxidation of the amino group to render the imine. This type of oxidation has already been reported in the biosynthesis of the alkaloid polyketide abikoviromycin by Tsuruoka et al. (1973), which purified an oxidoreductase from its producer that carries out the oxidation of the amino group into the imine group of this compound. Further attachment of N-acetylcysteine to the oxidized product synthesized by ArpO would generate argimycins PI/PII. Most probably, this would be a non-enzymatic event as it has been reported for the biosynthesis of other N-acetylcysteine-containing compounds (Suzuki et al., 2006; Gómez-Escribano et al., 2012; Maier et al., 2014).
The arp gene cluster contains two cluster situated regulators (CSR; Huang et al., 2005): arpRI codes for a SARP-like activator and its inactivation completely blocks argimycins P production; arpRII codes for a TetR-like repressor and its inactivation increases argimycins P production (Ye et al., 2017). To confirm their regulatory role, both genes were independently overexpressed into S. argillaceus and production of nigrifactin, argimycin PI/II, PV, and PVI was quantified in the resultant recombinant strains and in regulatory mutant strains MARPRI and MARPRII as well (Supplementary Figure S22). S. argillaceus containing the empty vector and S. argillaceus wild type strains were used as controls. Production of argimycins P was completely blocked in MARPRI, while it increased in MARPRII between 56 and 259%, depending of the argimycin P analyzed. On the other hand, overexpression of arpRI (pSETEcRI) led to an increase (between 23 and 94%), and that of arpRII (pSETERII) to a decrease (between 48 and 87%) in argimycins P production, depending of the argimycin P quantified. These results confirmed the role of ArpRI and ArpRII as activator and repressor in argimycin P biosynthesis, respectively. In order to delve deeper into their role in transcriptional regulation of arp cluster, expression analysis of arp genes in these mutants was evaluated by RT-PCR. Total RNA was isolated from cultures of S. argillaceus wild type strains and mutants MARPRI and MARPRII, after 24 h of growth. As shown in Figure 7A, all genes were expressed in the wild type strain including both regulatory genes. On the contrary, in MARPRI no transcripts were detected for arpO, arpDHI, arpN, arpPI, arpPIII, and arpT. Noticeable, arpDHII, arpK, arpHI, arpHII, arpPII, and arpX were still transcribed but at lower extent. This was confirmed by qRT-PCR analyses that showed the expression levels of these genes were lower in MARPRI in relation to the wild type strain (Figure 7B). This could be explained by the existence of alternative promoters ArpRI-independent upstream of those genes. These results were consistent with the idea that ArpRI was an essential regulator of argimycins P biosynthesis. In MARPRII mutant, all arp genes were transcribed apparently at higher levels than in the wild type strain. One exception was arpRII, since it was not transcribed (Figure 7A). To better understand the relationship between both regulatory genes, qRT-PCR analyses of them were carried out in each regulatory mutant. As observed in Figure 7C, expression of arpRI and arpRII was slightly lower in MARPRI, and expression of arpRI was higher and that of arpRII was much lower in MARPRII, in comparison to the wild type strain. Based on these results, we propose a model for the regulation of argimycins P biosynthesis (Figure 7D), in which ArpRII positively controls expression of its coding gene and negatively controls expression of arpRI whose gene product in turn positively controls expression of all arp genes.
FIGURE 7. Gene expression analysis of the arp gene cluster. (A) Transcription analysis by RT-PCR of arp genes in the wild type (WT) and in regulatory mutants S. argillaceus MARPRI and MARPRII. The band intensity represents the level of transcription of each gene in the different strains. Expression of the hrdB gene was used to standardize the concentration of RNA. qRT-PCR quantification of the expression levels of (B) selected arp genes in MARPRI strain, and (C) of regulatory genes arpRI and arpRII in regulatory mutants MARPRI and MARPRII. Graphs show the relative expression of these genes in relation to the WT strain (WT), in (B) MARPRI, and (C) in MARPRI and MARPRII mutants. (D) Proposed model for transcriptional regulation of the arp gene cluster. Regulatory genes are represented in black. Black arrows indicate deduced transcriptional units ArpRI-dependent. Gray arrows indicate deduced transcriptional units ArpRI-independent. Sharp-ended and round-ended arrows indicate activation and repression, respectively.
We have characterized the post-piperideine ring biosynthesis steps of argimycins P, a group of piperidine-containing polyketides, significantly broadening the knowledge about the biosynthesis steps of this type of compounds and of its regulation. Moreover, we have pointed out important differences between the biosynthesis pathway of argimycins P and that of the structurally related streptazone E. Thus, the arp cluster shows high synteny to other clusters in Streptomyces, such as the streptazone E gene cluster (stz) from Streptomyces sp. MSC090213JE08 (Ohno et al., 2015) (Supplementary Figure S23). Interestingly, Streptomyces sp. MSC090213JE08 does not produce the two ring-containing argimycins P but Streptazone E and in addition, S. argillaceus produce a number of argimycins P (PVII to PXII) bearing a single piperidine ring, which have not been identified in cultures of Streptomyces sp. MSC090213JE08. These facts suggest differences between both pathways at different levels. Thus, the hexaketides synthesized by the Arp and the Stz PKS should be different, since module 5 from ArpPIII contains an inactive dehydratase domain (Supplementary Figure S21), while the corresponding module from StzB contains an active one. Another difference could be at the regulatory level. Transcriptional analyses of the arp cluster have shown that some genes could be expressed from two different promoters, an ArpRI-dependent and an ArpRI-independent, which could account for higher expression of those genes and consequently of the corresponding gene products. The imine reductase arpDHII is one such gene and this could partially explain why most one ring-containing argimycins P so far identified in S. argillaceus contain a piperidine ring, while in the case of Streptomyces sp. MSC090213JE08 these compounds contain a piperideine ring. In addition, differences could exist at the enzymatic level. Thus, ArpO shows high similarity (80% identical aminoacids) to the FAD-dependent monooxygenase StzK identified in the streptazone E gene cluster (Ohno et al., 2015). However, StzK has been involved in the formation of the epoxide ring which is later opened in the cyclization step to form the five-membered ring, while ArpO would act after the five-membered ring was formed, oxidizing the amino group. All these differences indicate that similar gene clusters can direct the biosynthesis of structurally similar but different compounds.
On the other hand, we have identified five new compounds (argimycins PVIII, PX, PXI, PXII, and PXIV) that can be expanded to produce other analogs, and generated several mutant strains that can be used as hosts to express genes from other biosynthesis gene clusters (e.g., from other polyketide alkaloid gene clusters) and vice versa, the characterized arp coding genes can be expressed in other producers to generate new potentially bioactive compounds (Méndez and Salas, 2003; Olano et al., 2014). Moreover, arp coding enzymes such as the ArpDHII imine reductase could contribute to extend the biocatalysis toolbox with new enzymes with potential application to make pharmaceuticals and agrochemicals (Schrittwieser et al., 2015; Sigrist et al., 2015).
CM and JS conceived and designed the project. SY conducted the experiments. AB and SY carried out compound purifications. CM wrote the manuscript. JS, CO, JG-S, and FM contributed to preparing the final version of the paper. All authors read and approved the final manuscript.
This work was supported by grants to CM from the Spanish Ministry of Economy and Competitiveness, MINECO (Grants BIO2011-25398, BIO2014-56752-R, and PIM2010EEI-00752), and by the grant “Apoyo a Grupos de Excelencia”, Principado de Asturias-FEDER (FC-15-GRUPIN14-014). EntreChem, S.L. acknowledges funding from the ERA-IB program and MINECO (PIM2010EEI-00752). SY was recipient of predoctoral fellowships from MINECO. We thank Fundación Bancaria Cajastur for financial support to CO.
The authors declare that part of this work was included in a Spanish patent.
We thank Dr. Fernando Reyes from Fundación MEDINA for technical support in structural elucidation compounds.
The Supplementary Material for this article can be found online at: https://www.frontiersin.org/articles/10.3389/fmicb.2018.00252/full#supplementary-material
Aguirrezabalaga, I., Olano, C., Allende, N., Rodríguez, L., Braña, A. F., Méndez, C., et al. (2000). Identification and expression of genes involved in biosynthesis of L-oleandrose and its intermediate L-olivose in the oleandomycin producer Streptomyces antibioticus. Antimicrob. Agents Chemother. 44, 1266–1275. doi: 10.1128/AAC.44.5.1266-1275.2000
Altschul, S. F., Madden, T. L., Schäffer, A. A., Zhang, J., Zhang, Z., Miller, W., et al. (1997). Gapped BLAST and PSI-BLAST: a new generation of protein database search programs. Nucleic Acids Res. 25, 3389–3402. doi: 10.1093/nar/25.17.3389
Awodi, U. R., Ronan, J. L., Masschelein, J., de los Santos, E. L. C., and Challis, G. L. (2017). Thioester reduction and aldehyde transamination are universal steps in actinobacterial polyketide alkaloid biosynthesis. Chem. Sci. 8, 411–415. doi: 10.1039/c6sc02803a
Bérdy, J. (2012). Thoughts and facts about antibiotics: where we are now and where we are heading. J. Antibiot. 65, 385–395. doi: 10.1038/ja.2012.27
Cano-Prieto, C., García-Salcedo, R., Sánchez-Hidalgo, M., Braña, A. F., Fiedler, H.-P., Méndez, C., et al. (2015). Genome mining of Streptomyces sp. Tü 6176: characterization of the nataxazole biosynthesis pathway. ChemBioChem 16, 1461–1473. doi: 10.1002/cbic.201500153
Cushnie, T. P. T., Cushnie, B., and Lamb, A. J. (2014). Alkaloids: an overview of their antibacterial, antibiotic-enhancing and antivirulence activities. Int. J. Antimicrob. Agents 44, 377–386. doi: 10.1016/j.ijantimicag.2014.06.001
Fernández, E., Weibbach, U., Sánchez Reillo, C., Braña, A. F., Méndez, C., Rohr, J., et al. (1998). Identification of two genes from Streptomyces argillaceus encoding glycosyltransferases involved in transfer of a disaccharide during biosynthesis of the antitumor drug mithramycin. J. Bacteriol. 180, 4929–4937.
Flórez, A. B., Álvarez, S., Zabala, D., Braña, A. F., Salas, J. A., and Méndez, C. (2015). Transcriptional regulation of mithramycin biosynthesis in Streptomyces argillaceus: dual role as activator and repressor of the PadR-like regulator MtrY. Microbiology 161, 272–284. doi: 10.1099/mic.0.080895-0
Freer, A. A., Gardner, D., Greatbanks, D., Poyser, J. P., and Sim, G. A. (1982). Structure of cyclizidine (antibiotic M146791): X-ray crystal structure of an indolizidinediol metabolite bearing a unique cyclopropyl side-chain. J. Chem. Soc. Chem. Commun. 1160–1162. doi: 10.1039/C39820001160
Gómez-Escribano, J. P., Song, L., Fox, D. J., Yeo, V., Bibb, M. J., and Challis, G. L. (2012). Structure and biosynthesis of the unusual polyketide alkaloid coelimycin P1, a metabolic product of the cpk gene cluster of Streptomyces coelicolor M145. Chem. Sci. 3, 2716–2720. doi: 10.1039/C2SC20410J
Grabley, S., Hammann, P., Kluge, H., Wink, J., Kricke, P., and Zeeck, A. (1991). Secondary metabolites by chemical screening. 4. Detection, isolation and biological activities of chiral synthons from Streptomyces. J. Antibiot. 44, 797–800. doi: 10.7164/antibiotics.44.797
Groenhagen, U., Maczka, M., Dickschat, J. S., and Schulz, S. (2014). Streptopyridines, volatile pyridine alkaloids produced by Streptomyces sp. FORM5. Beilstein J. Org. Chem. 10, 1421–1432. doi: 10.3762/bjoc.10.146
Heeb, S., Fletcher, M. P., Chhabra, S. R., Diggle, S. P., Williams, P., and Cámara, M. (2011). Quinolones: from antibiotics to autoinducers. FEMS Microbiol. Rev. 35, 247–274. doi: 10.1111/j.1574-6976.2010.00247.x
Huang, J., Shi, J., Molle, V., Sohlberg, B., Weaver, D., Bibb, M. J., et al. (2005). Cross-regulation among disparate antibiotic biosynthetic pathways of Streptomyces coelicolor. Mol. Microbiol. 58, 1276–1287. doi: 10.1111/j.1365-2958.2005.04879.x
Huang, W., Kim, S. J., Liu, J., and Zhang, W. (2015). Identification of the polyketide biosynthetic machinery for the indolizidine alkaloid cyclizidine. Org. Lett. 17, 5344–5347. doi: 10.1021/acs.orglett.5b02707
Kieser, T., Bibb, M. J., Buttner, M. J., Chater, K. F., and Hopwood, D. A. (2000). Practical Streptomyces Genetics. Norwich: The John Innes Foundation.
Liu, Q. F., Wang, J. D., Wang, X. J., Liu, C. X., Zhang, J., Pang, Y. W., et al. (2013). Two new piperidine alkaloids from Streptomyces sp. NEAU-Z4. J. Asian Nat. Prod. Res. 15, 221–224. doi: 10.1080/10286020.2012.761209
Maier, S., Pflüger, T., Loesgen, S., Asmus, K., Brötz, E., Paululat, T., et al. (2014). Insights into the bioactivity of mensacarcin and epoxide formation by MsnO8. ChemBioChem 15, 749–756. doi: 10.1002/cbic.201300704
Mayer, M., and Thiericke, R. (1993). Biosynthesis of streptazolin. J. Org. Chem. 58, 3486–3489. doi: 10.1021/jo00065a006
Méndez, C., and Salas, J. A. (2003). On the generation of novel anticancer drugs by recombinant DNA technology: the use of combinatorial biosynthesis to produce novel drugs. Comb. Chem. High Throughput Screen. 6, 513–526. doi: 10.2174/138620703106298699
Newman, D. J., and Cragg, G. M. (2016). Natural products as sources of new drugs from 1981 to 2014. J. Nat. Prod. 79, 629–661. doi: 10.1021/acs.jnatprod.5b01055
Ohno, S., Katsuyama, Y., Tajima, Y., Izumikawa, M., Takagi, M., Fujie, M., et al. (2015). Identification and characterization of the streptazone E biosynthetic gene cluster in Streptomyces sp. MSC090213JE08. ChemBioChem 16, 2385–2391. doi: 10.1002/cbic.201500317
Olano, C., Méndez, C., and Salas, J. A. (2014). “Strategies for the design and discovery of novel antibiotics using genetic engineering and genome mining,” in Antimicrobial Compounds: Current Strategies and New Alternatives, eds T. G. Villa and P. Veiga-Crespo (Berlin: Springer), 1–25.
Peng, H., Wei, E., Wang, J., Zhang, Y., Cheng, L., Ma, H., et al. (2016). Deciphering piperidine formation in polyketide-derived indolizidines reveals a thioester reduction, transamination, and unusual imine reduction process. ACS Chem. Biol. 11, 3278–3283. doi: 10.1021/acschembio.6b00875
Puder, C., Krastel, P., and Zeeck, A. (2000). Streptazones A, B1, B2, C, and D: new piperidine alkaloids from streptomycetes. J. Nat. Prod. 63, 1258–1260. doi: 10.1021/np0001373
Puder, C., Loya, S., Hizi, A., and Zeeck, A. (2001). New co-metabolites of the streptazolin pathway. J. Nat. Prod. 64, 42–45. doi: 10.1021/np000377i
Sambrook, J., and Russell, D. W. (2001). Molecular Cloning: A Laboratory Manual, 3rd Edn. Cold Spring Harbor, NY: Cold Spring Harbor Laboratory.
Schrittwieser, J. H., Velikogne, S., and Kroutil, W. (2015). Biocatalytic imine reduction and reductive amination of ketones. Adv. Synth. Catal. 357, 1655–1685. doi: 10.1002/adsc.201500420
Seto, H., Sato, T., and Yonehara, H. (1973). Application of carbon-13 in biosynthetic studies; FT-13C nuclear magnetic resonance spectra of dihydrolatumcidin. J. Antibiot. 26, 609–614. doi: 10.7164/antibiotics.26.609
Sigrist, R., da Costa, B. Z., Marsaioli, A. J., and de Oliveira, L. G. (2015). Nature-inspired enzymatic cascades to build valuable compounds. Biotechnol. Adv. 33, 394–411. doi: 10.1016/j.biotechadv.2015.03.010
Sun, Y., Zhou, X., Liu, J., Bao, K., Zhang, G., Tu, G., et al. (2002). Streptomyces nanchangensis, a producer of the insecticidal polyether antibiotic nanchangmycin and the antiparasitic macrolide meilingmycin, contains multiple polyketide gene clusters. Microbiology 148, 361–371. doi: 10.1099/00221287-148-2-361
Surup, F., Wagner, O., von Frieling, J., Schleicher, M., Oess, S., Müller, P., et al. (2007). The iromycins, a new family of pyridine metabolites from Streptomyces sp. I. Structure, NOS inhibitory activity, and biosynthesis. J. Org. Chem. 72, 5085–5090. doi: 10.1021/jo0703303
Suzuki, H., Furusho, Y., Higashi, T., Ohnishi, Y., and Horinouchi, S. (2006). A novel o-aminophenol oxidase responsible for formation of the phenoxazinone chromophore of grixazone. J. Biol. Chem. 281, 824–833. doi: 10.1074/jbc.M505806200
Terashima, T., Kuroda, Y., and Kaneko, Y. (1970). Studies on alkaloids of Streptomyces. Part II. Pharmacological activity and isolation of nigrifactin and taxonomical studies of Streptomyces strain No. FFD-101. Agr. Biol. Chem. 34, 753–759. doi: 10.1271/bbb1961.34.753
Tsuruoka, T., Shomura, T., Ogawa, Y., Ezaki, N., Watanabe, H., Amano, S., et al. (1973). Some properties of SF-973 B substance, the enzyme catalyzing the conversion of dihydroabikoviromycin into abikoviromycin. J. Antibiot. 26, 168–174. doi: 10.7164/antibiotics.26.168
Umezawa, H., Tazaki, T., and Fukuyama, S. (1951). An antiviral substance, abikoviromycin, produced by Streptomyces species. Jpn. J. Med. 4, 331–346. doi: 10.7883/yoken1948.4.331
Weissman, K. J. (2015). Uncovering the structures of modular polyketide synthases. Nat. Prod. Rep. 32, 436–453. doi: 10.1039/c4np00098f
Yang, C.-L., Wang, Y.-S., Liu, C.-L., Zeng, Y.-J., Cheng, P., Jiao, R.-H., et al. (2017). Strepchazolins A and B: two new alkaloids from a marine Streptomyces chartreusis NA02069. Mar. Drugs 15:244. doi: 10.3390/md15080244
Ye, S., Molloy, B., Braña, A. F., Zabala, D., Olano, C., Cortés, J., et al. (2017). Identification by genome mining of a type I polyketide gene cluster from Streptomyces argillaceus involved in the biosynthesis of pyridine and piperidine alkaloids argimycins P. Front. Microbiol. 8:194. doi: 10.3389/fmicb.2017.00194
Keywords: type I polyketide synthase, pyridine, piperidine, piperideine, imine reductase, specialized metabolites, regulation, alkaloid
Citation: Ye S, Braña AF, González-Sabín J, Morís F, Olano C, Salas JA and Méndez C (2018) New Insights into the Biosynthesis Pathway of Polyketide Alkaloid Argimycins P in Streptomyces argillaceus. Front. Microbiol. 9:252. doi: 10.3389/fmicb.2018.00252
Received: 08 November 2017; Accepted: 31 January 2018;
Published: 16 February 2018.
Edited by:
Qiang Wang, Institute of Hydrobiology (CAS), ChinaReviewed by:
Alejandro A. Murillo, EMBL Heidelberg, GermanyCopyright © 2018 Ye, Braña, González-Sabín, Morís, Olano, Salas and Méndez. This is an open-access article distributed under the terms of the Creative Commons Attribution License (CC BY). The use, distribution or reproduction in other forums is permitted, provided the original author(s) and the copyright owner are credited and that the original publication in this journal is cited, in accordance with accepted academic practice. No use, distribution or reproduction is permitted which does not comply with these terms.
*Correspondence: Carmen Méndez, Y21lbmRlemZAdW5pb3ZpLmVz
Disclaimer: All claims expressed in this article are solely those of the authors and do not necessarily represent those of their affiliated organizations, or those of the publisher, the editors and the reviewers. Any product that may be evaluated in this article or claim that may be made by its manufacturer is not guaranteed or endorsed by the publisher.
Research integrity at Frontiers
Learn more about the work of our research integrity team to safeguard the quality of each article we publish.