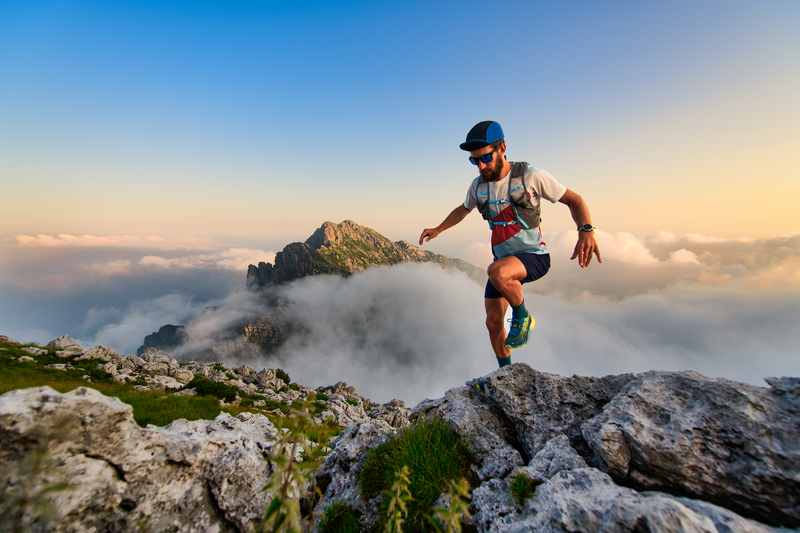
95% of researchers rate our articles as excellent or good
Learn more about the work of our research integrity team to safeguard the quality of each article we publish.
Find out more
ORIGINAL RESEARCH article
Front. Microbiol. , 26 January 2018
Sec. Evolutionary and Genomic Microbiology
Volume 9 - 2018 | https://doi.org/10.3389/fmicb.2018.00066
This article is part of the Research Topic Horizontal Gene Transfer and Genetic Diversity in Bacteria View all 20 articles
Members of the genus Acinetobacter possess distinct plasmid types which provide effective platforms for the acquisition, evolution, and dissemination of antimicrobial resistance structures. Many plasmid-borne resistance structures are bordered by short DNA sequences providing potential recognition sites for the host XerC and XerD site-specific tyrosine recombinases (XerC/D-like sites). However, whether these sites are active in recombination and how they assist the mobilization of associated resistance structures is still poorly understood. Here we characterized the plasmids carried by Acinetobacter baumannii Ab242, a multidrug-resistant clinical strain belonging to the ST104 (Oxford scheme) which produces an OXA-58 carbapenem-hydrolyzing class-D β-lactamase (CHDL). Plasmid sequencing and characterization of replication, stability, and adaptive modules revealed the presence in Ab242 of three novel plasmids lacking self-transferability functions which were designated pAb242_9, pAb242_12, and pAb242_25, respectively. Among them, only pAb242_25 was found to carry an adaptive module encompassing an ISAba825-blaOXA-58 arrangement accompanied by a TnaphA6 transposon, the whole structure conferring simultaneous resistance to carbapenems and aminoglycosides. Ab242 plasmids harbor several XerC/D-like sites, with most sites found in pAb242_25 located in the vicinity or within the adaptive module described above. Electrotransformation of susceptible A. nosocomialis cells with Ab242 plasmids followed by imipenem selection indicated that the transforming plasmid form was a co-integrate resulting from the fusion of pAb242_25 and pAb242_12. Further characterization by cloning and sequencing studies indicated that a XerC/D site in pAb242_25 and another in pAb242_12 provided the active sister pair for the inter-molecular site-specific recombination reaction mediating the fusion of these two plasmids. Moreover, the resulting co-integrate was found also to undergo intra-molecular resolution at the new pair of XerC/D sites generated during fusion thus regenerating the original pAb242_25 and pAb242_12 plasmids. These observations provide the first evidence indicating that XerC/D-like sites in A. baumannii plasmids can provide active pairs for site-specific recombination mediating inter-molecular fusions and intra-molecular resolutions. The overall results shed light on the evolutionary dynamics of A. baumannii plasmids and the underlying mechanisms of dissemination of genetic structures responsible for carbapenem and other antibiotics resistance among the Acinetobacter clinical population.
Acinetobacter baumannii represents nowadays a significant cause of healthcare-associated infections generally affecting immunocompromised and severely-injured patients with the global spread of a number of epidemic clonal complexes (CC) displaying multidrug-resistance (MDR) phenotypes (Peleg et al., 2008; Roca et al., 2012; Antunes et al., 2014; Wong et al., 2017). MDR strains contain an arsenal of antimicrobial resistance determinants some located in chromosomal resistance islands and others in plasmids, and have shown an outstanding ability to rapidly acquire additional resistance when confronted to new antimicrobials (Peleg et al., 2008; Bertini et al., 2010; Roca et al., 2012; Ramírez et al., 2013; Antunes et al., 2014; Touchon et al., 2014; Nigro et al., 2015; Blackwell and Hall, 2017; Wong et al., 2017). It is in this context that the increasing resistance among MDR A. baumannii strains to last-resort therapeutic options such as the carbapenem β-lactams represents a most worrisome problem (Peleg et al., 2008; Roca et al., 2012; Antunes et al., 2014; Wong et al., 2017).
Carbapenem resistance in A. baumannii clinical strains results from the interplay of different factors that include as the main cause the acquisition of β-lactamases endowed with carbapenemase activity (Poirel and Nordmann, 2006; Peleg et al., 2008; Poirel et al., 2010; Mussi et al., 2011; Ravasi et al., 2011; Roca et al., 2012; Antunes et al., 2014; Moran-Barrio et al., 2017; Wong et al., 2017). The most frequent acquired carbapenemases in A. baumannii are the carbapenem-hydrolyzing class-D β-lactamases (CHDL) OXA-23, OXA-40/24, and OXA-58 and associated variants, whose respective blaOXA genes are embedded in distinct plasmid-borne genetic structures which are thought to play pivotal roles in their mobilization and dissemination (Poirel and Nordmann, 2006; Zarrilli et al., 2008; D'Andrea et al., 2009; Merino et al., 2010; Poirel et al., 2010; Ravasi et al., 2011; Towner et al., 2011; Grosso et al., 2012; Roca et al., 2012; Evans and Amyes, 2014; Fu et al., 2014; Nigro et al., 2015; Da Silva and Domingues, 2016). A detailed characterization of the plasmids carried by carbapenem-resistant A. baumannii strains may help our understanding of the mechanisms of dissemination of these resistance structures, and contribute to the adoption of measures that limit the spread of antimicrobial resistance at both local and global scales.
Several authors have noted that many plasmid-borne blaOXA-containing structures are bordered by short sequences displaying homology to the 28-nucleotide dif motif located at the bacterial chromosome replication terminus and recognized by the XerC/D site-specific recombinases, leading to proposals that their mobilization could be mediated by site-specific recombination (Poirel and Nordmann, 2006; Zarrilli et al., 2008; D'Andrea et al., 2009; Merino et al., 2010; Poirel et al., 2010; Towner et al., 2011; Grosso et al., 2012; Evans and Amyes, 2014; Fu et al., 2014; Da Silva and Domingues, 2016; Blackwell and Hall, 2017). The highly conserved Xer site-specific recombination system normally acts to resolve bacterial chromosome dimers that form by homologous recombination during DNA replication, therefore allowing normal chromosome segregation to daughter cells during cell division (Cornet et al., 1994; Carnoy and Roten, 2009; Tran et al., 2012; Colloms, 2013; Midonet and Barre, 2014; Castillo et al., 2017). This resolution is mediated by the XerC and the XerD tyrosine recombinases which act coordinately to catalyze the addition of a cross-over between a directly-oriented pair of dif sites. Many plasmids contain sites recognized by the XerC/D recombinases (XerC/D-like sites) and exploit the host Xer system to resolve their own multimeric states, thus avoiding segregational instability or “dimer catastrophe” (Cornet et al., 1994; Carnoy and Roten, 2009; Garcillán-Barcia et al., 2011; Tran et al., 2012; Colloms, 2013; Midonet and Barre, 2014; Castillo et al., 2017). In addition, a number of mobile elements collectively designated as integrating mobile element exploiting Xer (IMEX) use this system to integrate (and eventually excise) their genomes into (from) the chromosomes of their respective hosts (Midonet and Barre, 2014; Castillo et al., 2017). Studies with model systems have indicated that, depending on their particular sequences and the immediate genetic context in which they are embedded, a pair of XerC/D-like sites may behave differently in recombination (Cornet et al., 1994; Colloms, 2013). Thus, a given pair may mediate only intra-molecular resolution, allow both intra-molecular resolution and inter-molecular fusion, or be inactive (Cornet et al., 1994). In this context whether the XerC/D-like sites linked to blaOXA-containing structures carried by A. baumannii plasmids are active, how do they act in recombination, and how this behavior may influence the mobilization and eventual dissemination of these resistance structures is still poorly understood.
We previously reported the presence of a plasmid-borne blaOXA-58 gene overexpressed as the result of the upstream insertion of an ISAba825 element among a group of clonally- and epidemiologically-related MDR A. baumannii clinical strains isolated in public hospitals of Rosario, Argentina (Ravasi et al., 2011; Piazza et al., 2013). In this work we characterized in detail the plasmids present in one of these strains, designated Ab242, to obtain clues into their dynamics and roles in the dissemination of carbapenem resistance determinants among the Acinetobacter clinical population.
Part of these results was presented recently in the 11th International Symposium on the Biology of Acinetobacter, Seville, Spain (Cameranesi et al., 2017a).
Acinetobacter baumannii Ab242 is a MDR clinical strain displaying carbapenem resistance (Table S1) isolated in 1997 from a clonal group disseminating in a public healthcare institution of Rosario, Argentina (Limansky et al., 2004; Mussi et al., 2011). MLST analysis assigned this strain to ST104 (CC104) in the Oxford scheme (cpn60-29, gdhB-12, gltA-12, gpi-57, gyrB-17, recA-1, rpoD-39) and to ST15 in the Pasteur scheme (cpn60-6, fusA-6, gltA-8, pyrG-2, recA-3, rplB-5, rpoB-4) (https://pubmlst.org/bigsdb?db=pubmlst_abaumannii_oxford_seqdef).
The A. nosocomialis M2 strain (Carruthers et al., 2013) was used in this study as a recipient for transformation assays with plasmids extracted from Ab242 (see below).
The above Acinetobacter strains were routinely grown in Lysogeny Broth (LB) liquid medium at 37°C under aerobic conditions with vigorous shaking for plasmid extraction procedures, or in LB agar medium with the indicated antibiotics for transformation studies and the selection of individual colonies. Appropriate procedures for working with a level 2 pathogen were followed throughout this work (Biosafety in Microbiological and Biomedical Laboratories, 5th edition, U.S. Department of Health and Human Services, Centers for Disease Control and Prevention, National Institutes for Health).
The MICs for different antimicrobials of A. baumannii Ab242, A. nosocomialis M2, and A. nosocomialis M2 transformed with Ab242 plasmids (Table S1) were evaluated using the VITEK-2 antimicrobial susceptibility testing system (bioMérieux). The MICs for kanamycin were determined by the macrodilution method using Mueller-Hinton (MH) broth in accordance to CLSI recommended procedures (Clinical and Laboratory Standards Institute, 2016).
The plasmids from Ab242 or from transformed A. nosocomialis cells were extracted using the Wizard® DNA purification kit (Promega, Madison, WI, USA) or the E.Z.N.A® Plasmid DNA Mini Kit I (OMEGA bio-tek, Norcross, GA, USA). The plasmids were analyzed by 0.7% agarose gel electrophoresis and ethidium bromide staining following conventional procedures (Sambrook et al., 1989).
Ab242 plasmids were transformed into competent cells of A. nosocomialis M2 by electroporation using a Bio-Rad GenePulser II set at 2.5 kV, 25 mF, and 200 V. The transformed cell mixture was plated on LB agar supplemented with 2 μg/ml IPM incubated overnight at 37°C and resistant colonies were analyzed for the presence of plasmids bearing the ISAba825-blaOXA-58 arrangement by PCR using primers PISAba825-F and OXA-58R (Table S2).
Plasmids extracted from the transformed A. nosocomialis cells were first characterized by restriction mapping with EcoRI and BamHI. The EcoRI-derived fragments were further cloned into E. coli cloning vectors for sequencing purposes. Briefly, these fragments were ligated into the equivalent sites of the pSU18 plasmid bearing a chloramphenicol (Cm)-resistance cassette (Bartolome et al., 1991), transformed into E. coli DH5α competent cells, and plated on LB agar medium containing 20 μg/ml Cm, 1 mM IPTG, and 0.5 mM X-gal to identify E. coli colonies bearing insert-containing plasmids (Bartolome et al., 1991). These plasmids were further isolated using the Wizard DNA purification kit (Promega, Madison, WI), restriction mapped with EcoRI, and selected inserts were further subjected to DNA sequencing (see below).
S1 nuclease treatment of Ab242 plasmids was conducted essentially as described (Barton et al., 1995) followed by agarose gel electrophoresis analysis of the digested material (Figure 1B). Southern blot analysis was performed on these gels following described protocols (Sambrook et al., 1989). Briefly, the gels were incubated in 0.25 M HCl for 10 min, then in 0.5 M NaOH, 1.5 M NaCl for 20 min, and finally equilibrated in 1 M Tris-HCl (pH 7.0), 1.5 M NaCl, and pressure blotted for 16 h into a nitrocellulose membrane (Amersham Biosciences Hybond™ -ECL, GE Healthcare UK Limited, UK). The membranes were hybridized with a biotinilated probe specific for the blaOXA-58 gene made of a 689-bp PCR-generated fragment using biotin-labeled primers (OXA-58-Fw-5′Biot and OXA-58-Rv-5′Biot, see Table S2; Sambrook et al., 1989). After incubation with a streptavidine-alkaline phosphatase conjugate (Sigma-Aldrich®, Saint Louis, MI, USA), specific bands were detected by chromogenic blot development with nitro-blue tetrazolium and 5-bromo-4-chloro-3′-indolyphosphate.
Figure 1. Agarose gel electrophoresis, S1 nuclease, and Southern blot analyses of plasmids isolated from A. baumannii Ab242 and A. nosocomialis M2 transformed with Ab242 plasmids. (A) Gel electrophoresis (0.7% agarose)/ethidium bromide staining of total plasmids extracted from Ab242 cells (lane 1) and A. nosocomialis M2 cells transformed with Ab242 plasmids (lane 2). (B) Ab242 total plasmids were treated with S1 nuclease (lane 1) and plasmids extracted from A. nosocomialis M2 cells transformants were treated with BamHI (lane 2), both analyzed by agarose gel electrophoresis/ethidium bromide staining. The gels from lane 1 were capillary transferred to nitrocellulose membranes and subjected to a Southern blot analysis using a 689-bp biotin-labeled blaOXA-58 probe (lane 3). The three closed arrowheads at the left margin indicate, from top to bottom, discrete DNA bands obtained after S1 nuclease digestion of sizes of around 25, 12, and 9 kbp, respectively. The open arrowhead at the top points to faint band of a larger size. The final positions of the size markers (38.4 kbp SacI-digested pLD209 plasmid, Marchiaro et al., 2014; plus lambda DNA digested with EcoRI and HindIII) are indicated at the left margin.
DNA sequencing of Ab242 plasmids was done at the Instituto de Agrobiotecnología Rosario (INDEAR, Rosario, Argentina) using a 454 pyrosequencing platform (Roche Diagnostics). The obtained reads were assembled in silico and the resulting sequences were refined by visual inspection. Three plasmids were inferred from this analysis and designated pAb242_25, pAb242_12, and pAb242_9, where the latter numbers indicate the approximate corresponding lengths in kbp. The circular structures of these plasmids were confirmed by PCR using specifically designed primer pairs followed by sequencing of the obtained amplicons, as well as by primer walking (see Table S2 and Figure S1 for details). DNA sequencing was done at the Sequencing Facility of Maine University. The nucleotide sequences of the plasmids described in this study were deposited in the GenBank nucleotide sequence database under accession numbers KY984047 (pAb242_25), KY984046 (pAb242_12), and KY984045 (pAb242_9).
The Rapid Annotation using Subsystem Technology standard operating procedures (RAST, http://rast.nmpdr.org/seedviewer.cgi) (Aziz et al., 2008) and the National Center for Biotechnology Information database (NCBI, U.S. National Library of Medicine, Bethesda MD, USA) were used to annotate the open reading frames (ORFs).
Search for antimicrobial resistance determinants was done using ResFinder 2.1 (https://cge.cbs.dtu.dk/services/ResFinder/; Zankari et al., 2012). The detection of IS was done with IS Finder (Siguier et al., 2006) (https://www-is.biotoul.fr/) and ISsaga (Varani et al., 2011). Comparative analysis of the carbapenem and aminoglycoside adaptive module located in pAb242_25 with similar structures reported in other Acinetobacter plasmids was conducted using Mauve (Darling et al., 2010).
A consensus 28-mer XerC/D recognition sequence (5′-atTTcgtaTAAggtgtaTTATGttAAat-3′) was generated from the comparisons of 17 reported Acinetobacter XerC/D-like sites, which included the single dif site present in the Acinetobacter baylyi chromosome (Carnoy and Roten, 2009) and 16 sites described in different Acinetobacter plasmids (Table S5). In the above consensus sequence the uppercase letters denote a completely conserved nucleotide at a given position in the 17 sequences analyzed, otherwise lowercase letters were used. The “Consensus finder” online tool (http://www.insilicase.com/Web/ConsensusSite.aspx) was used employing the above motif and allowing for up to two mismatches at conserved positions for the detection of XerC/D-like sites in Ab242 plasmids.
The A. baumannii Ab242 clinical strain used in this study was assigned to the ST104 of the CC104 (Oxford scheme), one of the CCs responsible for the dissemination of blaOXA-58 genes in South America (Clímaco et al., 2013; Ramírez et al., 2013). Antimicrobial susceptibility testing indicated a MDR profile for Ab242, with clinical resistance to β-lactams including carbapenems (IPM, meropenem), extended spectrum cephalosporins (cefotaxime, ceftazidime, cefepime), ampicillin/sulbactam and piperacillin/tazobactam; as well as to aminoglycosides (amikacin, gentamicin); to quinolones (ciprofloxacin); and to folate pathway inhibitors (trimethoprim/sulphamethoxazole) (Table S1).
Plasmid extraction followed by agarose gel electrophoresis analysis indicated the presence of plasmids in Ab242 (Figure 1A). S1 nuclease analysis revealed the linearized plasmid forms showing at least 3 well-defined species whose sizes ranged from around 9 kbp to slightly more than 23 kbp, from which the latter appeared as the predominant plasmid form (Figure 1B). Pyrosequencing analysis followed by in silico assembly and gap closure (Figure S1A, Table S2, see Materials and Methods for details) indicated three plasmids in this strain of approximate sizes of 9, 12, and 25 kbp hereafter designated pAb242_9, pAb242_12, and pAb242_25, respectively (Figure 2A). Database sequence comparisons predicted the presence of 15 complete ORFs in pAb242_9 from which 9 encode proteins with attributed functions (black arrows in Figure 2A); 13 complete ORFs in pAb242_12 from which 6 encode proteins with attributed functions; and 21 complete ORFs in pAb242_25 from which 16 encode proteins with attributed functions (see Table S3 for details). From them only pAb242_25 carried antimicrobial resistance determinants, including a blaOXA-58 gene preceded by an ISAba825 insertion promoting overexpression of the CHDL gene (Ravasi et al., 2011) accompanied by a composite transposon (TnaphA6) bearing an aphA6 aminoglycoside resistance gene (Nigro et al., 2011). Transformation analyses of susceptible Acinetobacter strains indicated that the above genes conform an antimicrobial resistance module effectively conferring both carbapenem- and aminoglycoside-resistance to an Acinetobacter host (Table S1, see also below). Of note also, pAb242_25 was the only plasmid identified in Ab242 carrying different IS including IS26, ISAba825, ISAba125, and ISAba3 (Iida et al., 1984; Mussi et al., 2005; Poirel and Nordmann, 2006; Ravasi et al., 2011; Figure 2A, Tables S3, S4).
Figure 2. Organization and gene content of A. baumannii Ab242 plasmids. (A) From left to right: inferred schemes of Ab242 plasmids pAb242_9 (KY984045), pAb242_12 (KY984046), and pAb242_25 (KY984047). The ORFs and their corresponding orientations are denoted by arrows. Closed arrows correspond to genes with described functions, whereas open arrows relate to unknown functions. Disrupted and incomplete genes are indicated with a “Δ” symbol preceding the gene denomination. The dark circles denote the predicted oriV regions, whose starting bases were arbitrarily set to 1 in the clockwise scale in each case (innermost circles) that also indicates the corresponding lengths in kbp. The different XerC/D-like binding sites identified in this work are highlighthed as ovals (not drawn to scale), with the XerC and XerD recognition regions depicted as dark gray and light gray semi-ovals, respectively, separated by the corresponding cr. Note that not all sites located in a given plasmid show the same polarity. The sequences of the different XerC/D sites are shown in Table 2. For more details see also Table S3. (B) Expanded view of the antimicrobial resistance structure bearing blaOXA-58 and aphA6 antimicrobial resistance genes and its close bordering regions. The orientation of each gene is indicated, with the two resistance genes denoted in black. Genes interrupted by IS are indicated in gray boxes with a “Δ” symbol, with the double internal arrowheads indicating the original direction of transcription. Thus, ΔISAba3 located immediately upstream of blaOXA-58 denotes the remnants of an original ISAba3 copy disrupted by an ISAba825 insertion. In turn, the two ΔlysE fragments bordering the TnaphA6 composite transposon denote the 5′ and 3′ remnants, respectively, of the original lysE gene. DR1 and DR2 denote the direct duplications generated by the indicated mobile elements at the corresponding target insertion sites (see Table S4 for details).
The replication modules of Acinetobacter plasmids differ from those of other bacterial groups, leading to a classification scheme based on the comparison of replicase (Rep) sequences that originally distinguished 19 different homology groups (GR) among them (Bertini et al., 2010; Towner et al., 2011). Our searching predicted a total of four rep genes among Ab242 plasmids, all encoding replicases of the Rep-3 superfamily (Table 1; Bertini et al., 2010; Towner et al., 2011). A more detailed characterization of the encoded proteins against representative replicases of each of the presently defined A. baumannii plasmids GR groups confidentially assigned the replicase encoded in pAb242_9 to GR4, as judged by the 95% sequence identity with the RepAci4 enzyme from A. baumannii p844 (Table 1, Table S3). On the contrary, the affiliation of the three other Ab242 plasmids replicases to any of the 19 presently-defined GR was more ambiguous. For instance, in the bi-replicon plasmid pAb242_25 (Figure 2, Table S3) one of the rep genes (designated repAci23, Table 1) encodes a protein with 100% amino acid identity with the Rep protein encoded in plasmid p11921 (Table S3), which was marginally affiliated to GR8 in the current classification scheme (Bertini et al., 2010). Also, the closest assigned homolog to the second replicase encoded in pAb242_25 (designated repAci22, Table 1) was the RepAciX protein (GR10) from pACICU1, but with only 53% identity at the protein sequence level, thus uncovering a novel class of replicase in pAb242_25. Similarly, the closest assigned homolog of the replicase encoded in pAb242_12 (designated repAci21, Table 1) was the GR12 enzyme from pABIR, but again with only 41% protein identity. These comparisons suggested the presence of three novel replicases in Ab242 plasmids. In this context, we have recently re-evaluated the Rep-based strategy of A. baumannii plasmids classification using 122 replicases sequences deposited in public databases. This analysis confirmed that repAci21, repAci22, and repAci23 represent examples of three novel GR groups (Cameranesi et al., 2017b).
Concerning stability function genes, different toxin-antitoxin (TA) systems (Bertini et al., 2010; Fondi et al., 2010; Garcillán-Barcia et al., 2011; Towner et al., 2011) were detected in Ab242 plasmids (Figure 2A, Table 1 and Table S3). Each of the Ab242 plasmids carries at least one TA system which include RelBE-type and VapBC-type systems (Table 1). Homologous systems were separately detected in other Acinetobacter plasmids including pAB2, pM131-5, and pACICU1 (Jurėnaitė et al., 2013), which otherwise show little sequence homology to Ab242 plasmids (Figure S2). This strongly suggests that these TA systems are mobilized as modules between the Acinetobacter population. It is worth noting that the relE toxin gene of the TA module located in pAb242_25 contains a mutation introducing a premature termination codon splitting this gene in two regions (Table 1, Table S3). This premature interruption was not observed in the relE gene of the homologous and active TA system present in pACICU1 (Jurėnaitė et al., 2013), casting doubts on the functionality of the RelE toxin in pAb242_25.
Regarding transferability genes no complete conjugative systems were detected among Ab242 plasmids, in line with previous observations indicating that most A. baumannii plasmids generally lack transfer functions (Bertini et al., 2010; Fondi et al., 2010; Garcillán-Barcia et al., 2011; Towner et al., 2011). Still, some mob homologs were detected in pAb242_9 and pAb242_25 such as mob_9 and the cluster mob_25-trbL, respectively (Table 1, Table S3).
Concerning antimicrobial resistance genes, as noted above only pAb242_25 carries an adaptive module of around 7.8 kbp containing both blaOXA-58 and aphA6 genes in which different IS play roles in the overexpression (ISAba825, Ravasi et al., 2011) or in the mobilization (ISAba3, Poirel and Nordmann, 2006; ISAba125, Lambert et al., 1990; Nigro et al., 2011) of these resistance genes (Figure 2B, Table 1, Tables S3, S4). The blaOXA-58 gene is immediately flanked by the remnants of a composite transposon originally formed by two oppositely-oriented ISAba3 copies (Figure 2B) as in other Acinetobacter plasmids carrying related structures (Poirel and Nordmann, 2006; Zarrilli et al., 2008; D'Andrea et al., 2009; Evans and Amyes, 2014). The insertion of ISAba825 at the ISAba3 copy located immediately upstream of the blaOXA-58 gene (Figure 2B) has been found to generate an alternative strong promoter driving overexpression of the CHDL gene (Ravasi et al., 2011). Similarly to related structures (Poirel and Nordmann, 2006; Zarrilli et al., 2008; D'Andrea et al., 2009; Ravasi et al., 2011; Evans and Amyes, 2014; Fu et al., 2014), an araC1 gene was located downstream of the intact ISAba3 copy of the composite transposon, followed in turn by a lysE gene disrupted in this case by a TnaphA6 formed by two ISAba125 elements bracketing an aphA6 gene (Nigro et al., 2011). TnaphA6 and ISAba825 likely represent recent acquisitions by this adaptive module as judged by the noticeable retention of the short direct duplications generated by the insertions of these mobile elements at the corresponding target sites (Figure 2B and Table S4).
Short DNA stretches displaying homology to the recognition motif of XerC and XerD site-specific recombinases (designated Re27, XerC/D-like, or pdif sites by different authors) have been previously noticed at the borders of blaOXA-58-containing resistance structures carried by Acinetobacter plasmids (Poirel and Nordmann, 2006; Zarrilli et al., 2008; D'Andrea et al., 2009; Merino et al., 2010; Towner et al., 2011; Grosso et al., 2012; Evans and Amyes, 2014; Fu et al., 2014; Da Silva and Domingues, 2016; Blackwell and Hall, 2017). We obtained a 28-mer consensus motif from the comparisons of XerC/D-like recognition sites described in Acinetobacter plasmids (Table S5), and used it as a query to detect similar sequences among Ab242 plasmids (see Materials and Methods for details). Our searching identified 17 XerC/D-like sites among them: 5 in pAb242_9; 4 in pAb242_12; and 8 in pAb242_25 (Table 2). As seen in Table 2, the XerC/D-like sites identified in Ab242 plasmids were not identical between them, with the XerD recognition region more conserved than the corresponding XerC region and the central region (cr) being the most variable, similarly to the situation of chromosomal dif sites (Carnoy and Roten, 2009). Also reflecting the case of chromosomal dif sites, the XerC/D sites identified in Ab242 plasmids possess the highly conserved positions 8 to 11 (XerC recognition region) and 18 to 21 (XerD recognition region) bordering the cr (Table 2) which are critical for the binding of the corresponding recombinases (Carnoy and Roten, 2009).
Of note, Ab242 plasmids are endowed with 3.7 XerC/D-like sites per 10 kbp of length in average, a number far exceeding the single site found on whole bacterial chromosomes, on most other plasmids using the Xer system for multimer resolution, or on IMEX elements (Cornet et al., 1994; Carnoy and Roten, 2009; Tran et al., 2012; Colloms, 2013; Midonet and Barre, 2014; Castillo et al., 2017). Moreover, since the different XerC/D sites are neither palindromic nor identical (e.g., see Table 2), they show defined polarities when located in the same plasmid molecule with some displaying the same orientation (directly-oriented sites) and others opposite orientations (inversely-oriented sites) (Figure 2A). Since the outcome of intra-molecular recombinational exchanges occurring on a circular molecule differs depending on the relative orientations of the recombining sister sites (Colloms, 2013), both the content and varied orientations of XerC/D-like sites in each of the Ab242 plasmids (Figure 2A) suggest function(s) other than a sole participation in the resolution of the corresponding multimeric forms as is other cases.
In the above context we noted that 7 out of the 8 XerC/D-like sites identified in pAb242_25 are located in the close vicinity of the adaptive module carried by this plasmid, while the remaining site (#5) is embedded between the intact ISAba3 copy located downstream of blaOXA-58, and the araC1 gene (Figure 2B). Three XerC/D-like sites designated from #6 to #8 (Table 2) were located upstream of the blaOXA-58 gene (left side of the adaptive module in Figure 2B), with site #6 positioned closer to the disrupted ISAba3 copy. Site #7 is separated from #6 by a metAP gene and an orf9, and displays an inverse-orientation as compared to #6. In turn, site #8 is separated from #7 by a cinH gene, and is directly-oriented as compared to #7 (Figure 2B). On the opposite extreme of the adaptive module four XerC/D-like sites were located and designated from #4 to #1, with site #4 situated immediately downstream of the 3′end of the disrupted lysE gene and directly-oriented as compared to site #6 (Figure 2B). Notably, sites #4, #3, and #2 are positioned in alternate inverse orientations between them, while #1 shows the same orientation as site #2.
We analyzed next whether the adaptive module present in pAb242_25 could induce simultaneous resistance to carbapenems and aminoglycosides when introduced into a susceptible Acinetobacter host. For this purpose total plasmids were extracted from Ab242 and electrotransformed into susceptible A. nosocomialis M2 cells (see Materials and Methods for details). The transformed cells were plated on LB agar supplemented with 2 μg/ml IPM to select for carbapenem resistance, and after incubation different colonies were screened for the presence of the ISAba825-blaOXA-58 arrangement by PCR. The antimicrobial susceptibility profile of a representative A. nosocomialis transformant clone testing positive for this arrangement is shown in Table S1. As seen in this Table, the corresponding cells had acquired resistance not only to carbapenems such as IPM and meropenem but also to several aminoglycosides including amikacin and kanamycin, thus indicating the presence of the adaptive module containing the ISAba825-blaOXA-58 arrangement and the TnaphA6 transposon.
A more detailed characterization of the plasmids extracted from the transformed A. nosocomialis cells was then conducted. The plasmid pattern obtained after agarose gel electrophoresis analysis showed an expected lower number of bands as compared to that generated by Ab242 cells (Figure 1A). However, a BamHI restriction enzyme analysis (lane 2 in Figure 1B) revealed that the (expected) large fragment of approximately 25 kbp corresponding to the BamHI-linearized form of pAb242_25 was accompanied by an extra band of around 12 kbp (for the location of BamHI sites in pAb242_25 and pAb242_12 see Figure 3A). As seen in Figure 3A the extra 12 kbp band corresponds to the BamHI-linearized form of pAb242_12, suggesting that the plasmid originally transforming and establishing in A. nosocomialis was a co-integrate between pAb242_25 and pAb242_12 which had then undergone resolution in this new host (see scheme in Figure 3A).
Figure 3. Site-specific recombination between XerC/D sites mediates fusion between pAb242_25 and pAb242_12. (A, Upper panel) Scheme depicting the formation of the plasmid co-integrate pAb242_37 by inter-molecular site-specific recombination at the sister pair XerC_7/XerD_7 (C7/D7) and XerC_9/XerD_9 (C9/D9) located in pAb242_25 and pAb242_12, respectively. The resolution of pAb242_37 by intra-molecular site-specific recombination at the new pair of XerC/D sites generated during the fusion reaction (C9/D7 and C7/D9, respectively) is also shown. The blaOXA-58- and aphA6-containing adaptive module in pAb242_25 and pAb242_37 is highlighted in dark gray. The positions of EcoRI (E) and BamHI (B) restriction sites in these plasmids are also indicated. The different XerC/D-like recognition sites (see Table 2 for sequence details) are highlighted as ovals (not drawn to scale) as detailed in the legend to Figure 2. The pair of sites identified as recombinationally active in this work have been arbitrarily enlarged. Open and closed inner circles within XerC_7/XerD_7 and XerC_9/XerD_9, respectively, are shown to facilitate the visualization of the fusions generating XerC_9/XerD_7 (C9/D7) and XerC_7/XerD_9 (C7/D9) in pAb242_37. The cloned EcoRI fragments used to identify these fusions (see text for details) are also indicated above the pAb242_37 scheme. (B, Lower panel) Enlarged vision including the nucleotide sequences of the XerC/D sites involved in the fusion/resolution reactions showing that the polarity of the sites was maintained after site-specific recombination. Relevant nucleotides in each site are highlighted in bold. Slash (/) symbols at the borders indicate that further sequence data existed beyond this position. The scheme is not drawn to scale.
At least two different mechanisms, one involving transposition of a mobile element (Iida et al., 1984; Mahillon and Chandler, 1998) and the other a fusion reaction mediated by site-specific recombination at XerC/D sites (Cornet et al., 1994; Tran et al., 2012; Colloms, 2013; Midonet and Barre, 2014; Castillo et al., 2017) could account for the formation of co-integrates between two circular DNA molecules lacking shared long regions of sequence identity. If co-integrate formation between pAb242_25 and pAb242_12 resulted from recombinational exchange between a sister pair of XerC/D sites, the polarity of the recombining sites would be maintained so that, after cleavage by the recombinases, the left-hand half of each site would be rejoined to the right-hand half of the other on the fused product (Carnoy and Roten, 2009; Tran et al., 2012; Colloms, 2013; Midonet and Barre, 2014; Castillo et al., 2017). In turn, if co-integrate formation resulted from the transposition of a mobile element originally present in one of the plasmids, the responsible element would be retained as a scar in the fusion site linking both plasmids (Iida et al., 1984; Mahillon and Chandler, 1998). Thus, the characterization of the fusion region between the two plasmids can allow differentiating between the two potential mechanisms of co-integrate formation.
We thus attempted the identification of enzyme restriction fragments containing fusions between pAb242_25 and pAb242_12 in the plasmid mixture obtained from the A. nosocomialis transformant described above. The plasmid mixture was digested with EcoRI and the derived fragments were cloned into the equivalent sites of the E. coli vector plasmid pSU18 and analyzed (see Materials and Methods for details). From more than 100 inserts analyzed we identified two EcoRI fragments of around 8 kbp and 11 kbp, respectively, containing the products corresponding to a fusion between sites XerC_9/XerD_9 of pAb242_12 and XerC_7/XerD_7 of pAb242_25, i.e., XerC_9/XerD_7 and XerC_7/XerD_9 (Figure 3 and Table 2 for sequence details). The identification of this particular fusion confirmed the formation of a co-integrate between pAb242_12 and pAb242_25 (i.e., pAb242_37) from an inter-molecular site-specific recombinational exchange mediated by the indicated pair of XerC/D sites.
The presence of pAb242_37 in the plasmid mixture recovered from the A. nosocomialis transformant cells was subsequently confirmed using a combination of PCR and primer walking strategies specifically designed to identify XerC_9/XerD_7 and XerC_7/XerD_9 fusions (Figure S1B). By using similar procedures (Figure S1A) we identified also the presence of plasmids bearing the original XerC_7/D_7 and XerC_9/XerD_9 sites in this mixture, in agreement to our results above indicating that pAb242_37 had mostly undergone resolution in the A. nosocomialis transformant cells (Figure 3A). Of note, the two pairs of sister sites identified above share a common cr sequence, GGTGTA, but show one-nucleotide differences at both the XerC (position 4) and XerD (position 26) recognition motifs (Table 2). Still, these variant positions are relatively separated from the conserved palindromic sequence encompassing positions 8 to 11 and 18 to 21 bracketing cr and presumed critical for the binding of the recombinases for subsequent cleavage (Carnoy and Roten, 2009).
Similar PCR and primer walking strategies described above (Figure S1B) were also used to verify the presence of the pAb242_37 co-integrate in plasmid extracts obtained from Ab242 cells. An evaluation of the relative abundance of this co-integrate as compared to pAb242_25 was then attempted on S1 endonuclease digests by means of a Southern blot analysis using blaOXA-58 as a probe (Figure 1B). As seen in this Figure, the analysis indicated the presence of two forms carrying blaOXA-58, a main band of approximately 25 kbp corresponding to the linearized form of pAb242_25 and a less prominent band of a larger size most likely representing the pAb242_37 co-integrate.
The overall results shown above support the existence on Ab242 cells of a co-integrate resulting from the fusion of pAb242_25 and pAb242_12 mediated by an inter-molecular site-specific recombination involving sites XerC/D_7 in pAb242_25 and XerC/D_9 in pAb242_12. Moreover, they also indicated that the two XerC/D sites product of this recombinational exchange provided another active sister pair, thus allowing co-integrate resolution (see scheme in Figure 3). The low recovery of restriction fragments containing fused products between pAb242_25 and pAb242_12 in the plasmid mixture derived from transformed A. nosocomialis cells, added to the higher abundance of pAb242_25 as compared to the larger co-integrate form in Ab242 as judged by Southern blot analysis (see above) suggest that the recombination reaction is mainly biased toward resolution in the cell (Figure 3).
A BlastN search against the nucleotide GenBank database using each of the three Ab242 plasmid sequences as individual queries indicated no significantly extended homology to deposited plasmid sequences. However, short segments of Ab242 plasmids displaying nucleotide identity values ranging from 84 to 99% with particular regions of other Acinetobacter plasmids were detected (Figure S2, Table S6). Notable, many of these homologous regions were found bracketed by XerC/D-like recognition sites (Figure S2).
Figure 4 shows a comparative analysis of the structures and genetic contexts of blaOXA-58-containing adaptive modules between pAb242_25 and other plasmids of Acinetobacter species of the A. calcoaceticus/A. baumannii complex (see Table S7 for details). These comparisons revealed a common skeleton structure embedded in different genetic contexts in all cases, and which is represented by an ISAba3 composite transposon carrying the blaOXA-58 gene accompanied by araC1 and lysE genes (Poirel and Nordmann, 2006; Evans and Amyes, 2014; Fu et al., 2014; Da Silva and Domingues, 2016). This co-occurrence of blaOXA-58 with araC1 has been noted previously, and suggested to play roles in the regulation of the CHDL gene (Fondi et al., 2010). The basic structure described above is reflected in pAG304 from A. pittii (Figure 4d) and also in some plasmids from A. nosocomialis (Fu et al., 2014). The various IS inserted in the ISAba3 copy located upstream of the blaOXA-58 gene in the modules driving overexpression of the CHDL gene (Table S7) represent most probably recent and independent acquisitions in different hosts selected by carbapenem therapy. Of note, only two of these blaOXA-58-containing adaptive modules, those carried by pBJAB0715 (Zhu et al., 2013) or pWH8144 (Fu et al., 2014; Figure 4b) and by pAb242_25 (Figure 4a; this work), also carried TnaphA6 elements and simultaneously conferred resistance to carbapenems and aminoglycosides to susceptible Acinetobacter hosts. However, the different insertion sites and orientations of TnaphA6 within lysE between them indicate independent acquisitions of this mobile element by the corresponding structures. The evidence above, added to the presence of an additional ISAba20 disrupting araC1 in pBJAB0715 or pWH8144 (absent in pAb242_25), provide additional support of separate histories of IS and transposon acquisitions by each of these modules after their departure from a common ancestral module.
Figure 4. Comparison between blaOXA-58-containing genetic structures carried by Acinetobacter plasmids. Nine blaOXA-58 containing-adaptive modules carried by Acinetobacter plasmids (indicated at the right) and the corresponding genetic contexts in which they are inserted (a–i) are depicted, with that found in pAb242_25 (this work) shown at the top. The gray-shaded background interconnecting the different structures highlights the homologous regions (nucleotide sequences ≥ 95% identity). Inverted regions between a given pair of structures are depicted with gray cross sectors. The different CDS and corresponding orientations are denoted, with truncated or interrupted genes indicated by gray boxes with white arrowheads inside indicating their original orientations. The blaOXA-58 CHDL and aphA6 aminoglycosides resistance genes are labeled in black. The different IS interrupting the ISAba3 element located upstream of blaOXA-58 are indicated by the designations adopted in the corresponding references. In (a,b), the regions encompassing the composite transposon TnaphA6, their different directions and insertion sites within the lysE gene, and the remnants of this gene (ΔlysE) in each case are indicated. The inferred XerC/D-like sites are shown as in Figure 2. In (b–i), the sites noted previously by other authors (see references below) are indicated by asterisks below the structures. In the case of pMAD (c) the two original XerC/D sites located at the borders of the module are indicated by their original designations Re27-1 and Re27-2, respectively. The slash (/) symbols at the borders of a given structure indicate that further sequence data was provided in databases beyond these positions; otherwise the structure was interrupted at the point where no further sequence information was available. Further details on the XerC/D sites, plasmids, and the Acinetobacter species from which they were isolated or characterized are provided in Tables S3, S7 and in references (Poirel and Nordmann, 2006; Zarrilli et al., 2008; D'Andrea et al., 2009; Merino et al., 2010; Grosso et al., 2012; Fu et al., 2014; Blackwell and Hall, 2017).
Concerning the XerC/D-like sites associated to these structures, it is noteworthy the existence of three XerC/D-like sites located in alternate inverse orientations in association to the basic module located in pAG304, with two directly-oriented sites bracketing this module and the third located within it (Figure 4d). Two of these sites, the internal one and the site located immediately downstream of lysE gene (i. e., the equivalents to #5 and #4, respectively, in Figure 4a) are absolutely conserved both in location and orientation among all of these structures (Figure 4). In turn, the XerC/D site located at the left boundary of the module (i.e., the equivalent to #6 in in Figure 4a) is less conserved but still present in many of these structures. The conspicuous absence of the latter site in some structures (e.g., Figures 4b,h,i,) probably resulted from disruptive IS insertions at this region. Of note, the content of XerC/D-like sites outside the limits posed by the genetic structure common to all plasmids also varies substantially between them, with the adaptive module located in pAb242_25 (Figure 4a) representing the most promiscuous case both in number and orientations of XerC/D-like sites.
In this work we characterized in detail the plasmids present in A. baumannii Ab242, a MDR clinical strain of the CC104 isolated in Argentina, to obtain clues into different aspects related to plasmid diversity, evolutionary dynamics, and underlying mechanisms of dissemination among the Acinetobacter population of genetic structures conferring blaOXA-58-mediated carbapenem resistance. Sequence analysis indicated the existence of three novel plasmids in Ab242 carrying distinct replication and stability modules and lacking self-transferability functions (Figure 2, Table 1). Only one of them, a bi-replicon plasmid of around 25 kbp in size designated pAb242_25, was found to harbor an adaptive module carrying a blaOXA-58 gene with an upstream ISAba825 insertion which we previously reported to promote the over-expression of the CHDL gene (Ravasi et al., 2011) accompanied by a TnaphA6 transposon inserted in a lysE gene located downstream of the CHDL gene (Figure 2). Transformation analysis with plasmids isolated from Ab242 indicated that the adaptive module present in pAb242_25 can simultaneously provide resistance to carbapenems and aminoglycosides to an Acinetobacter host (Table S1).
Amikacin was amply used in the past to treat infections due to susceptible A. baumannii strains, and it was replaced by carbapenem therapy around 20 years ago for strains which have acquired MDR (Limansky et al., 2004; Peleg et al., 2008; Nigro et al., 2011). The aphA6 gene conferring resistance to amikacin and other aminoglycosides was first described among clinical Acinetobacter spp. isolates recovered after 1984 (Lambert et al., 1990). Evidence for transposability was also obtained (Lambert et al., 1990), and it was confirmed later that this gene was in fact part of a composite transposon designated TnaphA6 (Nigro et al., 2011). It is not totally unexpected then the finding of A. baumannii plasmids carrying TnaphA6 and blaOXA gene(s) with the ability to confer simultaneous resistance to amikacin and carbapenems, as is the case described here for Ab242 isolated in 1997 (Limansky et al., 2004; Mussi et al., 2011). Acinetobacter plasmids with these characteristics can be broadly divided in two groups. On the one hand, self-transferable plasmids such as pD46-3 and pABUH1 with very similar backbones associated to GR6 replicases, and which have acquired separately TnaphA6 and blaOXA-23-containing complex transposons such as Tn2006 or Tn2008 (Nigro et al., 2015). On the other hand, plasmids lacking self-transferability such as pAb242_25 (this work), pBJAB07104 (Zhu et al., 2013) or pWH8144 (Fu et al., 2014), which show dissimilar backbones associated to different GR replicases and share blaOXA-58-containing adaptive modules exhibiting TnaphA6 and distinct IS driving blaOXA-58 overexpression collected on different occasions (Figure 4). The diverse genetic contexts in which these adaptive modules are embedded in the different plasmids, and the lack of self-transferability of the plasmids that carry them pose questions on how these resistance structures were mobilized to these locations and on their dissemination mechanisms among the Acinetobacter population (Bertini et al., 2010; Towner et al., 2011; Evans and Amyes, 2014; Da Silva and Domingues, 2016). The absence of mobilization and transfer functions, however, seems not to pose particular barriers to the horizontal gene transfer (HGT) of Acinetobacter plasmids (Bertini et al., 2010; Fondi et al., 2010; Towner et al., 2011), suggesting that transformation, transduction, or even non-canonical HGT, represent effective mechanisms for dissemination (Fondi et al., 2010; Da Silva and Domingues, 2016). Less is known on how the above blaOXA-58-containing modules could be mobilized to other genome locations, and in this context the observations by several authors that these resistance structures are bordered by XerC/D-like sites has led to proposals that this process could be mediated by site-specific recombination (Poirel and Nordmann, 2006; Zarrilli et al., 2008; D'Andrea et al., 2009; Merino et al., 2010; Poirel et al., 2010; Towner et al., 2011; Grosso et al., 2012; Evans and Amyes, 2014; Fu et al., 2014; Da Silva and Domingues, 2016; Blackwell and Hall, 2017). However, whether the XerC/D-like sites located in A. baumannii plasmids could be proficient for site-specific recombination, and how they could mediate the mobilization of the associated resistance structures remained obscure.
We showed in this work that at least some XerC/D-like sites present in Ab242 plasmids can constitute active pairs proficient for site-specific recombination, thus providing clues on how the associated blaOXA-58-containing adaptive modules could be mobilized. We inferred the presence of several XerC/D-like sites not only in pAb242_25 carrying the blaOXA-58- and TnaphA6-adaptive module described above, but also in the other Ab242 plasmids lacking antimicrobial resistance genes (Figure 2). We also demonstrated that two of these XerC/D-like sites, #7 located in the proximity of the adaptive module in pAb242_25 and #9 in pAb242_12, conform a proficient pair for site-specific recombination mediating the fusion of these two plasmids (Figure 3). Moreover, the two XerC/D sites generated by this fusion also represented a recombinationally proficient sister pair, mediating in this case the intra-molecular resolution of the co-integrate with the regeneration of the original plasmids.
The finding that Acinetobacter plasmids contain XerC/D-like sites capable of conforming recombinationally active pairs mediating both fusions and resolutions has a significant impact on the dynamics of these mobile elements and the possibilities of dissemination of resistance structures they carry, and even on the integration of resistance determinants into chromosomal dif sites. In principle, it certainly opens the possibility of co-integrate formation between temporarily-coexisting plasmids in which one of the constituents is endowed with self-transferability capabilities, thus allowing the dissemination of “cargo” plasmids to other cells by conduction (Garcillán-Barcia et al., 2011). The rapid resolution of the co-integrates once in the host cell (Figure 3) certainly adds support to this possibility. Also, the generation of co-integrates between different plasmids increases the possibilities of further intra-molecular rearrangements such as resolutions, deletions, and/or inversions (Colloms, 2013) depending on the locations and orientations of the new available pairs of XerC/D recombining sites. The reported influences of sequences of the sites and their immediate DNA contexts on the directionality of the recombining reaction or even on its feasibility (Cornet et al., 1994) certainly adds further levels of complexity to the process.
Acinetobacter baumannii strains carrying more than one replicon have been previously noted (Bertini et al., 2010; Towner et al., 2011) suggesting that replicon fusion may be relatively frequent in this bacterial species and even provide some selective advantages for plasmid dissemination. Among them, we already noted above that co-integrate formation may allow conduction of plasmids lacking mobility or transferability functions. Replicon fusion may also expand the host range of the co-integrate by providing establishment and/or stability functions that facilitate a successful establishment into a new host (Sýkora, 1992; Garcillán-Barcia et al., 2011), a situation independent of the mechanism (conjugation, transformation, transduction, non-canonical HGT) employed for co-integrate dissemination. In this context, we observed above that the co-integrate between pAb242_25 and pAb242_12, but not pAb242_25 alone, was the form that could successfully establish into A. nosocomialis when this organism was used as a host for transformation. Last, but not less important, similarly to the case of plasmid multimers the formation of co-integrates may favor the rapid accumulation of plasmid variants carrying adaptive mutations particularly under conditions of selective stress (Mazin et al., 1996).
A close look at the dissimilar genetic contexts in which blaOXA-58-containing adaptive modules are embedded in different Acinetobacter plasmids (Figure 4) provides evidence that some of these regions may have derived from XerC/D-mediated recombinational rearrangements. For instance, the gene cluster orf2_sulP preceded by a XerC/D-like site and located at the left side of the blaOXA-58-containing module in pTVICU14 (Figure 4f) is very similar in sequence and arrangement to that found in pAb242_12 (Figure S2, this work). This suggests that in pTVICU14 this particular region may have originated from the fusion of two plasmids mediated by a XerC/D sister pair similarly to the case described here. Also, the opposite orientation of the homologous DNA fragments located immediately downstream of lysE and bearing orf6_orf4 genes between pTVICU14 (Figure 4f) and pAG13TU119 (Figure 4g) provides evidence for an intra-molecular inversion event mediated by the pair of oppositely-oriented XerC/D-like sites bracketing this region.
It should be kept on mind that, although the possibilities of plasmid shuffling mediated by site specific recombination at XerC/D sites may be large (and even immensurable as the number of potential sites is increased), the whole process is filtered by selection and only a few plasmid structures will eventually take over a large proportion of the population (Garcillán-Barcia et al., 2011). This selection process may range from point mutations that regulate (or impede) the recombinational activity of a given XerC/D site to complete deletions of the site. Again, a detailed comparative look at the genetic context of the blaOXA-58-containing adaptive modules of Figure 4 provides evidences of this process, as judged both by the differences in XerC/D-like sites content between the different plasmids and the notorious losses of some conserved sites in a number of them.
Further work is in progress to characterize in detail the different XerC/D-like sites located in A. baumannii plasmids and to understand their roles in the evolution and dissemination of antimicrobial resistance platforms among the Acinetobacter clinical population.
AV, MC, AL, and JM-B conceived and designed the work. MC performed the experimental work. MC and GR conducted the bioinformatic analysis. AV, MC, AL, JM-B, and GR analyzed the data. AV wrote the manuscript. All authors read and approved the final manuscript.
This work was supported by grants from Agencia Nacional de Promoción Científica y Tecnológica (ANPCyT PICT-2011-1020) and CONICET (PIP 1055) to AV; ANPCyT PICT-2012-0680 to AL; and Ministerio de Ciencia, Tecnología e Innovación Productiva, Provincia de Santa Fe, Argentina, to AV and AL MC is Fellow of CONICET, JM-B, GR, and AV are Career researchers of CONICET, and AL is a Researcher of the UNR.
The authors declare that the research was conducted in the absence of any commercial or financial relationships that could be construed as a potential conflict of interest.
We are indebted to Dr. M. D. Carruthers (Center for Microbial Pathogenesis, the Research Institute at Nationwide Children's Hospital and Department of Pediatrics, The Ohio State University College of Medicine, Columbus, Ohio, USA.) for providing the Acinetobacter nosocomialis M2 strain used in transformation assays described in this work.
The Supplementary Material for this article can be found online at: https://www.frontiersin.org/articles/10.3389/fmicb.2018.00066/full#supplementary-material
Antunes, C. S., Visca, P., and Towner, K. J. (2014). Acinetobacter baumannii: evolution of a global pathogen. Pathog. Dis. 71, 292–301. doi: 10.1111/2049-632X.12125
Aziz, R. K., Bartels, D., Best, A. A., De Jongh, M., Disz, T., Edwards, R. A., et al. (2008). The RAST Server: rapid annotations using subsystems technology. BMC Genomics 9:75. doi: 10.1186/1471-2164-9-75
Bartolome, B., Jubete, Y., Martinez, E., and de la Cruz, F. (1991). Construction and properties of a family of pACYC184-derived cloning vectors compatible with pBR322 and its derivatives. Gene 102, 75–78. doi: 10.1016/0378-1119(91)90541-I
Barton, B. M., Harding, G. P., and Zuccarelli, A. J. (1995). A general method for detecting and sizing large plasmids. Anal. Biochem. 226, 235–240. doi: 10.1006/abio.1995.1220
Bertini, A., Poirel, L., Mugnier, P. D., Villa, L., Nordmann, P., and Carattoli, A. (2010). Characterization and PCR-based replicon typing of resistance plasmids in Acinetobacter baumannii. Antimicrob. Agents Chemother. 54, 4168–4177. doi: 10.1128/AAC.00542-10
Blackwell, G. A., and Hall, R. M. (2017). The tet39 determinant and the msrE-mphE genes in Acinetobacter plasmids are each part of discrete modules flanked by inversely oriented pdif (XerC-XerD) sites. Antimicrob. Agents Chemother. 61, e00780-17. doi: 10.1128/AAC.00780-17
Cameranesi, M. M., Moran-Barrio, J., Repizo, G. D., Limansky, A. S., and Viale, A. M. (2017a). “XerC/D site-specific recombination mediates plasmid plasticity and dissemination of blaOXA-58 containing structures in Acinetobacter baumannii strains isolated in Argentina,” in 11th International Symposium on the Biology of Acinetobacter, Abstract P3-25. (Seville), 98.
Cameranesi, M. M., Limansky, A. S., Morán-Barrio, J., Repizo, G. D., and Viale, A. M. (2017b). Three novel Acinetobacter baumannii plasmid replicase-homology groups inferred from the analysis of a multidrug-resistant clinical strain isolated in Argentina. J. Infect. Dis. Epidemiol. 3:46. doi: 10.23937/2474-3658/1510046
Carnoy, C., and Roten, C. A. (2009). The dif/Xer recombination systems in proteobacteria. PLoS ONE 4:e6531. doi: 10.1371/journal.pone.0006531
Carruthers, M. D., Harding, C. M., Baker, B. D., Bonomo, R. A., Hujer, K. M., Rather, P. N., et al. (2013). Draft Genome Sequence of the Clinical Isolate Acinetobacter nosocomialis Strain M2. Genome Announc. 1, e00906–e00913. doi: 10.1128/genomeA.00906-13
Castillo, F., Benmohamed, A., and Szatmari, G. (2017). Xer site specific recombination: double and single recombinase systems. Front. Microbiol. 8:453. doi: 10.3389/fmicb.2017.00453
Clímaco, E. C., de Oliveira, M. L., Pitondo-Silva, A., Oliveira, M. G., Medeiros, M., Lincopan, N., et al. (2013). Clonal complexes 104, 109 and 113 playing a major role in the dissemination of OXA-carbapenemase-producing Acinetobacter baumannii in Southeast Brazil. Infect. Genet. Evol. 19, 127–133. doi: 10.1016/j.meegid.2013.06.024
Colloms, S. D. (2013). The topology of plasmid-monomerizing Xer site-specific recombination. Biochem. Soc. Trans. 41, 589–594. doi: 10.1042/BST20120340
Cornet, F., Mortier, I., Patte, J., and Louarn, J. M. (1994). Plasmid pSC101 harbors a recombination site, psi, which is able to resolve plasmid multimers and to substitute for the analogous chromosomal Escherichia coli site dif. J. Bacteriol. 176, 3188–3195. doi: 10.1128/jb.176.11.3188-3195.1994
Clinical and Laboratory Standards Institute. (2016). Performance Standards for Antimicrobial Susceptibility Testing, Document M100S, 26th Edn. (Wayne, PA).
D'Andrea, M. M., Giani, T., D'Arezzo, S., Capone, A., Petrosillo, N., Visca, P., et al. (2009). Characterization of pABVA01, a plasmid encoding the OXA-24 carbapenemase from Italian isolates of Acinetobacter baumannii. Antimicrob. Agents Chemother. 53, 3528–3533. doi: 10.1128/AAC.00178-09
Darling, A. E., Mau, B., and Perna, N. T. (2010). Progressive mauve: multiple genome alignment with gene gain, loss and rearrangement. PLoS ONE 5:e11147. doi: 10.1371/journal.pone.0011147
Da Silva, G., and Domingues, S. (2016). Insights on the horizontal gene transfer of carbapenemase determinants in the opportunistic pathogen Acinetobacter baumannii. Microorganisms 4:E29. doi: 10.3390/microorganisms4030029
Evans, B. A., and Amyes, S. G. B. (2014). OXA β-lactamases. Clin. Microbiol. Rev. 27, 241–263. doi: 10.1128/CMR.00117-13
Fondi, M., Bacci, G., Brilli, M., Papaleo, M. C., Mengoni, A., Vaneechoutte, M., et al. (2010). Exploring the evolutionary dynamics of plasmids: the Acinetobacter pan-plasmidome. BMC Evol. Biol. 10:59. doi: 10.1186/1471-2148-10-59
Fu, Y., Jiang, J., Zhou, H., Jiang, Y., Fu, Y., Yu, Y., et al. (2014). Characterization of a novel plasmid type and various genetic contexts of bla OXA-58 in Acinetobacter spp. from multiple cities in China. PLoS ONE 9:e84680. doi: 10.1371/journal.pone.0084680
Garcillán-Barcia, M. P., Alvarado, A., and De la Cruz, F. (2011). Identification of bacterial plasmids based on mobility and plasmid population biology. FEMS Microbiol. Rev. 35, 936–956. doi: 10.1111/j.1574-6976.2011.00291.x
Grosso, F., Quinteira, S., Poirel, L., Novais, Â., and Peixe, L. (2012). Role of common blaOXA-24/OXA-40-carrying platforms and plasmids in the spread of OXA-24/OXA-40 among Acinetobacter species clinical isolates. Antimicrob. Agents Chemother. 56, 3969–3972. doi: 10.1128/AAC.06255-11
Iida, S., Mollet, B., Meyer, J., and Arber, W. (1984). Functional characterization of the prokaryotic mobile genetic element IS26. Mol. Gen. Genet. 198, 84–89 doi: 10.1007/BF00328705
Jurėnaitė, M., Markuckas, A., and Sužiedeliene, E. (2013). Identification and characterization of type II toxin-antitoxin systems in the opportunistic pathogen Acinetobacter baumannii. J. Bacteriol. 195, 3165–3172. doi: 10.1128/JB.00237-13
Lambert, T., Gerbaud, G., Bouvet, P., Vieu, J. F., and Courvalin, P. (1990). Dissemination of amikacin resistance gene aphA6 in Acinetobacter spp. Antimicrob. Agents Chemother. 34, 1244–1248.
Limansky, A. S., Zamboni, M. I., Guardati, M. C., Rossignol, G., Campos, E., and Viale, A. M. (2004). Evaluation of phenotypic and genotypic markers for clinical strains of Acinetobacter baumannii. Medicina (B. Aires). 64, 306–312.
Marchiaro, P. M., Brambilla, L., Morán-Barrio, J., Revale, S., Pasteran, F., Vila, A. J., et al. (2014). The Complete nucleotide sequence of the carbapenem resistance-conferring conjugative plasmid pld209 from a Pseudomonas putida clinical strain reveals a chimeric design formed by modules derived from both environmental and clinical bacteria. Antimicrob. Agents Chemother. 58, 1816–1821. doi: 10.1128/AAC.02494-13
Mazin, A. V., Timchenko, T. V., Saparbaev, M. K., and Mazina, O. M. (1996). Dimerization of plasmid DNA accelerates selection for antibiotic resistance. Mol. Microbiol. 20, 101–108. doi: 10.1111/j.1365-2958.1996.tb02492.x
Merino, M., Acosta, J., Poza, M., Sanz, F., Beceiro, A., Chaves, F., et al. (2010). OXA-24 carbapenemase gene flanked by XerC/XerD-like recombination sites in different plasmids from different Acinetobacter species isolated during a nosocomial outbreak. Antimicrob. Agents Chemother. 54, 2724–2727. doi: 10.1128/AAC.01674-09
Midonet, C., and Barre, F.-X. (2014). Xer Site-Specific Recombination: Promoting Vertical and Horizontal Transmission of Genetic Information. Microbiol. Spectr. 2. doi: 10.1128/microbiolspec.MDNA3-0056-2014
Moran-Barrio, J., Cameranesi, M. M., Relling, V., Limansky, A. S., Brambilla, L., and Viale, A. M. (2017). The Acinetobacter outer membrane contains multiple specific channels for carbapenem β-lactams as revealed by kinetic characterization analyses of imipenem permeation into Acinetobacter baylyi cells. Antimicrob. Agents Chemother. 61, e01737-16. doi: 10.1128/AAC.01737-16
Mussi, M. A., Limansky, A. S., Relling, V., Ravasi, P., Arakaki, A., Actis, L. A., et al. (2011). Horizontal gene transfer and assortative recombination within the Acinetobacter baumannii clinical population provide genetic diversity at the single carO gene, encoding a major outer membrane protein channel. J. Bacteriol. 193, 4736–4748. doi: 10.1128/JB.01533-10
Mussi, M. A., Limansky, A. S., and Viale, A. M. (2005). Acquisition of resistance to carbapenems in multidrug-resistant clinical strains of Acinetobacter baumannii: natural insertional inactivation of a gene encoding a member of a novel family of beta-barrel outer membrane proteins. Antimicrob. Agents Chemother. 49, 1432–1440. doi: 10.1128/AAC.49.4.1432-1440.2005
Nigro, S. J., Holt, K. E., Pickard, D., and Hall, R. M. (2015). Carbapenem and amikacin resistance on a large conjugative Acinetobacter baumannii plasmid. J. Antimicrob. Chemother. 70, 1259–1261. doi: 10.1093/jac/dku486
Nigro, S. J., Post, V., and Hall, R. M. (2011). Aminoglycoside resistance in multiply antibiotic-resistant Acinetobacter baumannii belonging to global clone 2 from Australian hospitals. J. Antimicrob. Chemother. 66, 1504–1509. doi: 10.1093/jac/dkr163
Peleg, A. Y., Seifert, H., and Paterson, D. L. (2008). Acinetobacter baumannii: emergence of a successful pathogen. Clin. Microbiol. Rev. 21, 538–582. doi: 10.1128/CMR.00058-07
Piazza, A., Limansky, A. S., Mussi, M. A., Morán-Barrio, J., Brambilla, L., and Viale, A. M. (2013). “Interplay of horizontal gene transfer, ISAba825-induced blaOXA-58 gene overexpression, and carO allele exchange in the evolution of carbapenem resistance among epidemiologically related Acinetobacter baumannii strains in a public hospital of Rosario, Argentina,” in 9th International Symposium on the Biology of Acinetobacter Abstract: P3–P31. (Cologne), 74.
Poirel, L., Naas, T., and Nordmann, P. (2010). Diversity, epidemiology, and genetics of class D beta-lactamases. Antimicrob. Agents Chemother. 54, 24–38. doi: 10.1128/AAC.01512-08
Poirel, L., and Nordmann, P. (2006). Genetic structures at the origin of acquisition and expression of the carbapenem-hydrolyzing oxacillinase gene blaOXA-58 in Acinetobacter baumannii. Antimicrob. Agents Chemother. 50, 1442–1448. doi: 10.1128/AAC.50.4.1442-1448.2006
Ramírez, M. S., Vilacoba, E., Stietz, M. S., Merkier, A. K., Jeric, P., Limansky, A. S., et al. (2013). Spreading of AbaR-type genomic islands in multidrug resistance Acinetobacter baumannii strains belonging to different clonal complexes. Curr. Microbiol. 67, 9–14. doi: 10.1007/s00284-013-0326-5
Ravasi, P., Limansky, A. S., Rodriguez, R. E., Viale, A. M., and Mussi, M. A. (2011). ISAba825, a functional insertion sequence modulating genomic plasticity and blaOXA-58 expression in Acinetobacter baumannii. Antimicrob. Agents Chemother. 55, 917–920. doi: 10.1128/AAC.00491-10
Roca, I., Espinal, P., Vila-Fanés, X., and Vila, J. (2012). The Acinetobacter baumannii oxymoron: commensal hospital dweller turned pan-drug-resistant menace. Front. Microbiol. 3:148. doi: 10.3389/fmicb.2012.00148
Sambrook, J. E., Fritsch, F., and Maniatis, T. (1989). Molecular Cloning. A Laboratory Manual, 2nd Edn., New York, NY: Cold Spring Harbor.
Siguier, P., Perochon, J., Lestrade, L., Mahillon, J., and Chandler, M. (2006). ISfinder: the reference centre for bacterial insertion sequences. Nucleic Acids Res. 34, D32–D36. doi: 10.1093/nar/gkj014
Sýkora, P. (1992). Macroevolution of plasmids: a model for plasmid speciation. J. Theor. Biol. 159, 53–65. doi: 10.1016/S0022-5193(05)80767-2
Touchon, M., Cury, J., Yoon, E. J., Krizova, L., Cerqueira, G. C., Murphy, C., et al. (2014). The genomic diversification of the whole Acinetobacter genus: origins, mechanisms, and consequences. Genome Biol. Evol. 6, 2866–2882. doi: 10.1093/gbe/evu225
Towner, K. J., Evans, B., Villa, L., Levi, K., Hamouda, A., Amyes, S. G. B., et al. (2011). Distribution of intrinsic plasmid replicase genes and their association with carbapenem-hydrolyzing class D β-lactamase genes in European clinical isolates of Acinetobacter baumannii. Antimicrob. Agents Chemother. 55, 2154–2159. doi: 10.1128/AAC.01661-10
Tran, T., Andres, P., Petroni, A., Soler-Bistu,é, A., Albornoz, E., Zorreguieta, A., et al. (2012). Small plasmids harboring qnrB19: a model for plasmid evolution mediated by site-specific recombination at oriT and Xer sites. Antimicrob. Agents Chemother. 56, 1821–1827. doi: 10.1128/AAC.06036-11
Varani, A. M., Siguier, P., Gourbeyre, E., Charneau, V., and Chandler, M. (2011). ISsaga is an ensemble of web-based methods for high throughput identification and semi-automatic annotation of insertion sequences in prokaryotic genomes. Genome Biol. 12:R30 doi: 10.1186/gb-2011-12-3-r30
Wong, D., Nielsen, T. B., Bonomo, R. A., Pantapalangkoor, P., Luna, B., and Spellberg, B. (2017). Clinical and Pathophysiological Overview of Acinetobacter Infections: a Century of Challenges. Clin. Microbiol. Rev. 30, 409–447. doi: 10.1128/CMR.00058-16
Zankari, E., Hasman, H., Cosentino, S., Vestergaard, M., Rasmussen, S., Lund, O., et al. (2012). Identification of acquired antimicrobial resistance genes. J. Antimicrob. Chemother. 67, 2640–2644. doi: 10.1093/jac/dks261
Zarrilli, R., Vitale, D., Di Popolo, A., Bagattini, M., Daoud, Z., Khan, A. U., et al. (2008). A plasmid-borne blaOXA-58 gene confers imipenem resistance to Acinetobacter baumannii isolates from a Lebanese hospital. Antimicrob. Agents Chemother. 52, 4115–4120. doi: 10.1128/AAC.00366-08
Keywords: Acinetobacter baumannii, blaOXA-58, carbapenem resistance, XerC/D site-specific recombination, antimicrobial resistance plasmids
Citation: Cameranesi MM, Morán-Barrio J, Limansky AS, Repizo GD and Viale AM (2018) Site-Specific Recombination at XerC/D Sites Mediates the Formation and Resolution of Plasmid Co-integrates Carrying a blaOXA-58- and TnaphA6-Resistance Module in Acinetobacter baumannii. Front. Microbiol. 9:66. doi: 10.3389/fmicb.2018.00066
Received: 24 November 2017; Accepted: 11 January 2018;
Published: 26 January 2018.
Edited by:
Peng Luo, Key Laboratory of Marginal Sea Geology, South China Sea Institute of Oceanology (CAS), ChinaReviewed by:
Filipa Grosso, Faculdade de Farmácia, Universidade do Porto, PortugalCopyright © 2018 Cameranesi, Morán-Barrio, Limansky, Repizo and Viale. This is an open-access article distributed under the terms of the Creative Commons Attribution License (CC BY). The use, distribution or reproduction in other forums is permitted, provided the original author(s) and the copyright owner are credited and that the original publication in this journal is cited, in accordance with accepted academic practice. No use, distribution or reproduction is permitted which does not comply with these terms.
*Correspondence: Alejandro M. Viale, dmlhbGVAaWJyLWNvbmljZXQuZ292LmFy
Disclaimer: All claims expressed in this article are solely those of the authors and do not necessarily represent those of their affiliated organizations, or those of the publisher, the editors and the reviewers. Any product that may be evaluated in this article or claim that may be made by its manufacturer is not guaranteed or endorsed by the publisher.
Research integrity at Frontiers
Learn more about the work of our research integrity team to safeguard the quality of each article we publish.