- 1Commensal and Probiotics-Host Interactions Laboratory, Micalis Institute, Institut National de la Recherche Agronomique, AgroParisTech, Jouy-en-Josas, France
- 2UMR7245, Muséum National d'Histoire Naturelle, Centre National de la Recherche Scientifique, Sorbonne-Universités, Paris, France
- 3JRU BIPAR, ANSES, Ecole Nationale Vétérinaire d'Alfort, INRA, Université Paris-Est, Animal Health Laboratory, Maisons-Alfort, France
- 4Department of Biological Sciences, University of Calgary, Calgary, AB, Canada
- 5JRU BIPAR, Ecole Nationale Vétérinaire d'Alfort, ANSES, INRA, Université Paris-Est, Maisons-Alfort, France
Giardia duodenalis (syn. G. lamblia, G. intestinalis) is the protozoan parasite responsible for giardiasis, the most common and widely spread intestinal parasitic disease worldwide, affecting both humans and animals. After cysts ingestion (through either contaminated food or water), Giardia excysts in the upper intestinal tract to release replicating trophozoites that are responsible for the production of symptoms. In the gut, Giardia cohabits with the host's microbiota, and several studies have revealed the importance of this gut ecosystem and/or some probiotic bacteria in providing protection against G. duodenalis infection through mechanisms that remain incompletely understood. Recent findings suggest that Bile-Salt-Hydrolase (BSH)-like activities from the probiotic strain of Lactobacillus johnsonii La1 may contribute to the anti-giardial activity displayed by this strain. Here, we cloned and expressed each of the three bsh genes present in the L. johnsonii La1 genome to study their enzymatic and biological properties. While BSH47 and BSH56 were expressed as recombinant active enzymes, no significant enzymatic activity was detected with BSH12. In vitro assays allowed determining the substrate specificities of both BSH47 and BSH56, which were different. Modeling of these BSHs indicated a strong conservation of their 3-D structures despite low conservation of their primary structures. Both recombinant enzymes were able to mediate anti-giardial biological activity against Giardia trophozoites in vitro. Moreover, BSH47 exerted significant anti-giardial effects when tested in a murine model of giardiasis. These results shed new light on the mechanism, whereby active BSH derived from the probiotic strain Lactobacillus johnsonii La1 may yield anti-giardial effects in vitro and in vivo. These findings pave the way toward novel approaches for the treatment of this widely spread but neglected infectious disease, both in human and in veterinary medicine.
Introduction
Giardia duodenalis (syn. Giardia lamblia and Giardia intestinalis) is a flagellated protozoan parasite responsible for giardiasis, an intestinal zoonotic disease infection that may cause acute or chronic diarrhea, weight loss, malabsorption, abdominal pain, and nausea (Ankarklev et al., 2010; Cotton et al., 2011). It is one of the most common intestinal parasites and one of the most frequent causes of diarrhea, with over 280 million human symptomatic cases worldwide (Lane and Lloyd, 2002; Platts-Mills et al., 2015). Infections occur mainly by the ingestion of cysts present in contaminated food and water. After ingestion, infectious cysts differentiate into trophozoite stages, which in turn colonize the upper small intestine. Included in the “Neglected Disease Initiative” of the World Health Organization (WHO) in 2004, giardiasis has a significant public health impact in both developed and developing countries (Savioli et al., 2006; Platts-Mills et al., 2015). Metronidazole is the most frequently used drug for treating G. duodenalis infections, whereas albendazole, tinidazole, and nitazoxanide may also be used with efficacy (Gardner and Hill, 2001; Petri, 2005). Although these drugs have different modes of action, there is an increasing incidence of parasite resistance, and treatment failure is relatively common (Ansell et al., 2015). Moreover, these standard treatments are commonly associated with undesirable side effects in both medical and veterinary usages (Barr et al., 1994; Gardner and Hill, 2001). A successful vaccine has proven elusive, and Giardia is able to escape host immunity by switching its variant-specific surface proteins (Singer et al., 2001). Together, these observations underscore the need for new therapeutic alternatives for the treatment of giardiasis.
In the last decade, some probiotics (i.e., live microorganisms which, when administered in adequate amounts, confer a health benefit on their hosts, WHO 2001), in particular several species belonging to the genus Lactobacillus, have shown anti-giardial efficacy in various murine models (see Travers et al., 2011 for review). The mechanisms remain incompletely understood but may involve host immunomodulation and/or extracellular compounds released by the bacteria (Perez et al., 2001; Humen et al., 2005; Shukla et al., 2008; Shukla and Sidhu, 2011; Goyal et al., 2013). In this context, we have recently shown that unconjugated bile salts, generated by secreted or released enzymes by the probiotic strain of Lactobacillus johnsonii La1 (also known as L. johnsonii NCC533), may contribute to the inhibition of Giardia trophozoite growth in vitro (Perez et al., 2001; Travers et al., 2016). BSH (also called cholylglycine hydrolase, EC 3.5.1.2) are enzymes that hydrolyze the amide bond of conjugated bile salts, liberating the amino acid moiety from the steroid core and generating deconjugated bile salts (i.e., cholic acid, deoxycholic acid and chenodeoxycholic acid) (Begley et al., 2006). Conjugated-bile salts are synthesized in the liver where conjugation to either glycine or taurine occurs, and these conjugated-bile salts play an important role in the solubility and absorption of lipids and cholesterol in the intestinal tract (Eyssen, 1973; Kim et al., 2005; Begley et al., 2006). Moreover, glyco- and tauro-conjugated bile salts exert detergent and antimicrobial properties (Ruiz et al., 2013). BSH activities lead to bile salt detoxification and confer a competitive advantage to the microbial communities that express them, such as lactobacilli in the upper part of the small intestine (Ridlon et al., 2006; Ruiz et al., 2013).
In this study, we cloned and expressed each one of the three-bsh genes (i.e., bsh12, bsh47, and bsh56) from L. johnsonii La1 (Pridmore et al., 2004) in Escherichia coli in order to evaluate their substrate specificities and to assess their anti-Giardia activities, both in vitro and in vivo. A comparative structural analysis of the three BSHs was also performed using in silico approaches to explore whether structural differences could explain possible differences in substrate specificities. The three recombinant BSHs, rBSH12, rBSH47, and rBSH56, were tested against two different strains of the human assemblage A of G. duodenalis (WB6 and NF) in vitro. Then, rBSH47 was selected to be tested in vivo on OF1 suckling mice infected with the G. duodenalis strain WB6.
Materials and Methods
In Silico Analysis of BSHs
Bile salt hydrolases amino acid sequences from different bacterial species were retrieved from databases using BLASTP program from the National Center for Biotechnology Information (NCBI, http://www.ncbi.nlm.nih.gov/) and analyzed in silico. Multiple sequence alignments of BSH amino acid sequences were performed using CLUSTALO 1.2.1 (http://www.ebi.ac.uk/Tools/msa/clustalo/) to identify the conserved motifs between the different enzymes. Phylogenetic relationships and phylogenic clustering of BSHs from different species were established by neighbor-joining methods using MEGA5 software (http://www.megasoftware.net/; Tamura et al., 2011). Three-dimensional modeling of L. johnsonii La1-BSHs was performed using I-TASSER from University of Michigan (http://zhanglab.ccmb.med.umich.edu/I-TASSER/; Roy et al., 2010). According to C-score results, the BSH from Bifidobacterium longum (Kumar et al., 2006) was chosen as template for modeling BSH56, whereas the BSH from Clostridium perfringens was used as template for modeling both BSH12 and BSH47 (Rossocha et al., 2005). Models for structure predictions were selected according to the highest values of their C-score (measured for evaluating global and local similarity between query and template protein). Protein structure analysis was performed using Pymol (PyMOL Molecular Graphics System, Version 1.8 Schrödinger, LLC).
Bacteria and Growth Conditions
Lactobacillus johnsonii La1 strain (Pridmore et al., 2004) was cultured in Man Rogosa Sharpe broth (MRS, Difco) and grown at 37°C in an anaerobic jar using BBL GasPak Anaerobic System (BD), incubated overnight (ON). E. coli TOP10 chemically competent cells (Invitrogen) were used for the subcloning of PCR fragments. E. coli CYS21 and SE1 chemically competent strains (DelphiGenetic, Belgium) were used, respectively, for the cloning and expression of BSHs. E. coli strains were grown in Luria-Bertani (LB) medium at 37°C ON with vigorous shaking at 180 rpm. All bacterial strains were stored at −80°C with 15% (v/v) glycerol for cryoprotection.
Cloning of bsh Genes from L. johnsonii La1
Genomic DNA of L. johnsonii La1 was extracted from 2 mL of an ON culture using Wizard Genomic DNA Purification Kit Protocol (Promega) and used as template to amplify the 3 bsh genes: bsh12 (Gene ID: 2743525), bsh47 (Gene ID: 2743183), and bsh56 (Gene ID: 2743142) (Pridmore et al., 2004). The coding sequences of bsh12, bsh47, and bsh56 genes (excluding the putative signal sequences) were amplified by PCR (Phusion Taq, Thermo Fisher Scientific) using primers described in Table 1. These primers were designed to incorporate two restriction sites: NheI (forward primer) and XhoI (reverse primer). The amplified PCR fragments were purified using SV Gel and PCR Clean-Up System (Wizard) and were subcloned into the vector pCR®2.1-TOPO® (Invitrogen). The resulting constructions (pLB487, pLB488, and pLB489) were validated by sequencing (MWG-Genomic Company, Germany) before recovering the bsh genes with NheI and XhoI restriction enzymes and cloning them into pStaby 1.2 vector (DelphiGenetics) previously digested with the same enzymes. The pStaby 1.2 plasmid was used for intermediate cloning to introduce a C-terminal six-Histidine tag (His-tag), allowing subsequent purification of rBSHs using affinity chromatography. The resulting plasmids were transferred into E. coli CYS21 strains and transformants were grown at 37°C ON in 10 mL of LB containing ampicillin (Amp, 100 μg/ml) with shaking at 180 rpm. Plasmid DNA were extracted from positive clones, sequenced to confirm identity, and subsequently transformed into E. coli SE1 expression strain. Bacterial strains, plasmids, and primer sequences used in this study are described in Table 1. Immunoblotting experiments were performed on E. coli SE1 (pLB490), E. coli SE1 (pLB491), and E. coli SE1 (pLB492) strains lysates (see below) using mouse monoclonal 6x-His Epitope Tag Antibody (Thermo Fisher Scientific) to detect recombinant BSHs.
Expression and Purification of Recombinant BSH12, BSH47, and BSH56 in E. coli
E. coli SE1 strains harboring pLB490 (bsh12), pLB491 (bsh47), and pLB492 (bsh56) were grown at 37°C ON in 10 mL of LB supplemented with ampicillin (100 μg/mL) with vigorous shaking at 180 rpm and subsequently grown in 1.5 L of LB/ampicillin (100 μg/mL) at 37°C. When an optical density (OD600nm) = 0.6–0.8 was reached, gene expression was induced by the addition of 1 mM of Isopropyl β-D-1-Thiogalactopyranoside (IPTG), and cultures were incubated at 21°C ON with shaking at 180 rpm. Bacteria were harvested by centrifugation and cell pellets were washed with PBS and resuspended in 15 ml of Tris-KCl buffer (Tris 50 mM, KCl 100 mM, MgCl2 10 mM, pH 7.5) supplemented with Triton-X-100 (Sigma-Aldrich) to a final concentration of 1% and protease inhibitors 1X (Roche). Cells were subsequently sonicated for 4 min with alternated pulses on ice (on: 5 s, off: 30 s). The lysed cells were then placed in ultracentrifuge tubes and spun at 220,000 × g at 4°C for 45 min to separate soluble supernatants from pellets.
The soluble fractions containing the recombinant BSH (rBSH) were then collected and rBSHs were purified using affinity chromatography. Briefly, columns kept in nickel-nitrilotriacetic acid (Ni-NTA; Qiagen) agarose were first washed with milliQ water and equilibrated with 50 mM Tris-KCl buffer pH 7.5 according to the supplier's protocol. Soluble lysates were passed through Ni-NTA columns and washed with 50 mM Tris-KCl buffer pH 7.5 to remove unbound proteins. Finally, rBSHs were eluted by increasing imidazole concentrations (25, 75, and 500 mM) as recommended by the supplier. The eluted proteins were desalted using Sephadex G-25 columns (Amersham Biosciences). All fractions were analyzed on Sodium Dodecyl Sulfate-PolyAcrylamide Gel Electrophoresis (SDS-PAGE) and stained with Coomassie Brilliant Blue.
Bile Salt Hydrolase Activity Assays
The substrate specificity of each rBSHs was assessed on plates using an agar test. E. coli strains harboring pLB490, pLB491, and pLB492 were cultured in LB broth in presence of ampicillin (100 μg/ml). Overnight cultures were then spotted on LB agar plates supplemented with either 0.5% taurodeoxycholic acid (TDCA, Sigma-Aldrich) or 0.5% glycodeoxycholic acid (GDCA, Merck Millipore) and incubated at 37°C for 48 h.
The BSH hydrolyzing activities were also monitored using purified recombinant enzymes in presence of conjugated bile salts, in solution, by measuring the liberation of amino acids (glycine or taurine) as previously described (Grill et al., 2000). A volume of 100 μl of rBSH (20 μg) was mixed with 100 μl of 2.4 g/L of each conjugated bile salts (GDCA, TDCA, glycocholic acid, or taurocholic acid) and incubated for 30 min at 37°C. BSH from C. perfringens (Sigma-Aldrich, reference C4018) was used as a positive control. A solution without bile salts was used as a negative control. The hydrolysis of bile salts was stopped by adding 200 μl of 15% trichloroacetic acid (TCA) (v/v%) and the mixture was spun at 10,000 g for 15 min to remove precipitated proteins. The supernatant (80 μl) was subsequently collected and added to 680 μl of 0.3 M borate buffer, 1% SDS (pH 9.5), and 80 μl of 0.3% picrylsulfonic acid solution (Sigma-Aldrich). Mixtures were incubated for 30 min in the dark at room temperature and 800 μL of 0.6 mM HCl was added to stop the colorimetric reaction. The amount of glycine or taurine released was measured at 416 nm using a spectrophotometer and standard curves were established with free glycine and taurine.
Giardia duodenalis Cultures
Two different isolates of assemblage A were used in this study: G. duodenalis strains WB clone 6 (WB6, ATCC50803), isolated from a patient with chronic giardiasis, and G. duodenalis NF (kindly provided by Dr. André Buret, University of Calgary), obtained from an outbreak of human giardiasis. Trophozoites were cultured in axenic conditions grown in Keiser's modified TYI-S-33 medium (KM) adjusted at pH 6.0 and supplemented with heat-inactivated fetal calf serum (10%) (FCS, reference A15-101, PAA laboratories, GE Healthcare) as recently described (Travers et al., 2016). In vitro experiments were performed with or without bovine bile (Difco, DB Diagnostic System, reference 212820) supplementation (0.6 g/L).
Anti-giardial Activity Assays
Increasing concentrations of rBSHs were co-incubated with fresh cultures of G. duodenalis WB6 trophozoites (2 × 105 parasites/ml) in KM medium supplemented with 10% FCS in a final volume of 480 μl, at 37°C in anaerobic conditions for 22 h. Experiments were performed with or without bovine bile (0.6 g/L) supplementation. BSH from C. perfringens (1U, Sigma-Aldrich, reference C4018) was used as a positive control. Trophozoites were detached from tubes by chilling on ice for 10 min and the parasite load was measured by using hemocytometer (flagella mobility was used as viability criteria). The inhibition levels were determined in comparison with values of non-treated trophozoite cultures (percentage of growth). Three biological replicates were performed, each in duplicates. The half maximal inhibitory concentrations (IC50) were calculated using Prism 5 software (GraphPad).
G. duodenalis Viability Assays on Cell Cultures
Caco-2 epithelial cells (human colonic adenocarcinoma, ATTC HTB-37) were grown in Dulbecco's Modified Eagle's Medium (DMEM) containing 200 mM L-glutamine, 100 U/mL penicillin, 100 U/mL streptomycin, and 10% fetal bovine serum (FBS) (Gibco, reference 12484-028) at 37°C and 5% CO2. Caco-2 cells were cultured (passage 28–32) at 80% confluence with trypsin-EDTA and seeded at 105 cells/mL onto 12-wells plates (Caco-2 growth medium). Cells were cultured until the monolayer was confluent (3–4 days) with medium changes every 48 h. 3 days prior to co-incubation, cultures of G. duodenalis NF strain trophozoites were axenically cultured in KM medium supplemented with 10% heat-inactivated FBS at 37°C to confluence. Parasites were ice-chilled for 15 min, harvested by centrifugation for 10 min at 1,300 × g (4°C), and resuspended in Caco-2 growth medium supplemented with bovine bile (0.6 g/L). For co-culture experiments, trophozoites were seeded at a multiplicity of infection (MOI) of 10:1. Recombinant BSHs were then added to co-cultures at different concentrations and the plates were incubated at 37°C and 5% CO2. After 20 h of incubation, trophozoites were collected after chilling of plates on ice and the parasite load was determined using hemocytometer (flagella mobility was used as viability criteria).
Scanning Electron Microscopy
For scanning electron microscopy (SEM), fresh cultures of G. duodenalis trophozoites WB6 strain were treated with either rBSH47 (0.5 μg/ml), rBSH56 (0.08 μg/ml), or DCA (0.1 g/L and 0.2 g/L) in KM supplemented with 10% heat-inactivated FCS (10%), with or without bovine bile (0.6 g/L) supplementation. Cultures of treated and untreated Giardia trophozoites were subsequently seeded in 12 wells plates on poly-lysine glass coverslips placed at the bottom, and parasites were let to settle on the glass coverslips. After 16 h incubation, the supernatants were removed gently and cells were fixed on the glass coverslips with cacodylate 0.1 M and glutaraldehyde 2.5% (pH 7.2) overnight at 4°C. After two washing steps with 0.1 M cacodylate (pH 7.2), cells were dehydrated in a graded ethanol series (50, 70, 90, and 100%) and critical point-dried in liquid CO2 (Emitech K850, Quorum Technologies). Coverslips were then mounted onto holders and coated with 20 nm of gold (JEOL Fine Coater JFC-1200). The samples were then examined with a Hitachi Scanning Electron SU3500 Premium.
Experimental Infection Model
OF1 mice were obtained from Charles River (Saint-Germain-Nuelles, France). Mice were housed in pathogen-free conditions and all experiments were performed under a laminar flow hood. Neonatal (suckling) mice were challenged with 105 G. duodenalis WB6 trophozoites at day 10 by intragastric gavage (100 μl). Recombinant BSH47 was diluted in DMEM with NaHCO3 16.4% (vehicle) and daily administered by intragastric gavage to neonatal mice from days 10 to 15. Control animals received vehicle instead of rBSH47. Animals were sacrificed by cervical dislocation at day 16 (peak of infection, as determined in parallel assays) and assayed for the presence of G. duodenalis trophozoites in the small intestine. Small intestines were resuspended in 5 ml of cold PBS, incubated on ice for 10 min, and mixed thoroughly. The parasite load was estimated using hemocytometer chambers. Mice with no detectable trophozoites (threshold: <103 parasites/5 ml intestine suspension) were considered as parasite-free. All protocols were carried out in accordance with the institutional ethical guidelines of the ethics committee ANSES's Animal Health Laboratory at Maisons-Alfort on the campus of the French National Veterinary School of Alfort (ENVA), which approved this study.
Statistical Analysis
Data analysis was performed with Prism 5 software (GraphPad). One-way ANOVA, Mann-Whitney, and t-test were used to evaluate difference between means. Results were presented as means ± standard error of the mean (SEM). Statistical significance was calculated at a P value of 0.05 and 95% confidence interval.
Results
In Silico Analysis of L. johnsonii La1-BSHs Protein Sequences
The amino acid sequences of L. johnsonii-BSH12, BSH47, and BSH56 enzymes were blasted against reported sequences from several Gram-positive bacteria using Blastp. For the three L. johnsonii La1 BSH, results indicated high identity levels with BSH of different Lactobacillus species ranging from 54 to 100% but lower levels of identity (less than 54%) with BSH from Bifidobacterium and Clostridium species. In particular, the L. johnsonii-BSH12 shared 54, 57, 60, 79–84, and 60–100% identities with BSHs from C. perfringens, L. acidophilus, L. reuteri, L. gasseri, and L. johnsonii, respectively. L. johnsonii-BSH47 shared 54–55, 56–58, 60–66, 57, 70, and 97–100% identities with BSHs from L. crispatus, L. reuteri, L. gasseri, L. acidophilus, L. amylovorus, and L. johnsonii, respectively. Finally, L. johnsonii-BSH56 showed 94 and 99% identity with BSHs from L. gasseri and both L. acidophilus and L. johnsonii, respectively.
The 3D structures of CBAH-1 from C. perfringens (Rossocha et al., 2005) and BlBSH from B. longum (Kumar et al., 2006) have been determined (PDB: 2BJF and PDB: 2RF8, respectively), revealing the presence of key residues in the enzymatic active site (Cys-2, Arg-18, Asp-21, Asn-82, Asn-172, and Arg-225; numbering referring to CBAH-1). In addition, experimental studies validated the importance of Arg-18 in the catalytic site (Fang et al., 2009; Lin, 2014; Lin et al., 2014). Therefore, multiple amino acid sequence alignments of BSH12, BSH47, and BSH56 with CBAH-1 and BlBSH were performed using ClustalO program (GONNET PAM 250 matrix), thereby indicating that these key residues were indeed highly conserved in all three L. johnsonii La1-BSHs (Figure 1). Moreover, motifs surrounding these key amino acid positions were also found to be well conserved such as 16FGRNXD, 72NEXGLXXAGLNF, 170VXXLTNXPXF, and 213GXGXGXXGXPGD, a point that has been also reported in other studies (Elkins et al., 2001; Kim and Lee, 2008). However, residues, which are predicted to be involved in the substrate-binding site based on the 3D structure of C. perfringens, did not appear to be conserved in either L. johnsonii BSH enzyme (Ridlon et al., 2006).
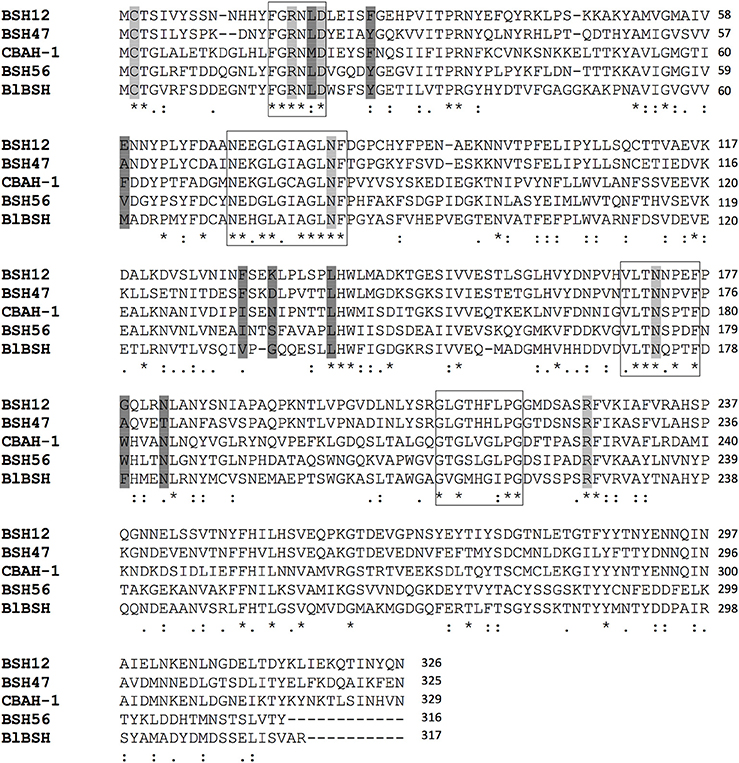
Figure 1. L. johnsonii La1 BSH possess conserved key amino acids in their predicted active sites. Multiple sequence alignments of BSH were performed with the ClustalO program (http://www.ebi.ac.uk/Tools/msa/clustalo/) using GONNET 250 matrix. An “*” (asterisk) indicates positions of fully conserved residues in all five sequences; a “:” (colon) indicates conservation of amino acid with strongly similar chemical properties (Gonnet PAM 250 matrix score > 0.5); a “.” indicates conservation of amino acid with similar chemical properties (Gonnet PAM 250 matrix score ≤ 0.5). Residues highlighted in light gray correspond to the predicted key active site amino acids, based on the 3D structures of BSHs from both C. perfringens (CBAH-1, PDB: 2BJF) (Rossocha et al., 2005) and B. longum (BlBSH, PDB: 2RF8) (Kumar et al., 2006). Residues highlighted in dark gray indicate amino acids putatively involved in substrate binding based on CBAH-1 3D structure (Rossocha et al., 2005; Ridlon et al., 2006). Boxes indicate conserved signatures, i.e., 16FGRNXD, 72NEXGLXXAGLNF, 170VXXLTNXPXF, and 213GXGXGXXGXPGD (CBAH-1 numbering).
Predicted tridimensional structures of L. johnsonii BSH12, BSH47, and BSH56 were modeled with I-TASSER software, using existing 3D structures (Figure S1, BSH47 and BSH56 and Figure S2, BSH12). Clostridium perfringens CBAH-1 was used as a template for BSH47 modeling (RMSD: 0.64; TM-score: 0.991; Identity, 35.4%) and BSH12 (RMSD: 1.5; TM-score: 0.967; Identity: 37.3%). Bifidobacterium longum BlBSH was used as a template for BSH56 modeling (RMSD: 1.16; TM-score: 0.966; Identity: 43.8%). A superimposition of BSH47 and BSH56 models revealed very similar structures. In particular, the distinctive αββα-folding pattern is conserved in both proteins (Patel et al., 2010; Lin et al., 2014). In addition, the residues involved in the catalytic site are superimposed which confirms a conservation of 3D structure despite a high variability of amino acid sequence among BSHs. Similar results were obtained with BSH12 (Figure S2).
Heterologous Expression and Purification of BSHs in E. coli
To study the biochemical and enzymatic characteristics of L. johnsonii La1-BSHs, bsh genes (i.e., bsh12, bsh47, and bsh56) were cloned in E. coli CYS21 strain and expressed in E. coli SE1 strain. Western blot analysis from E. coli SE1 cells expressing His-tagged BSHs showed an efficient production of BSH12, BSH47, and BSH56, respectively (Figure 2A). High yields of heterologous proteins were produced from 1.5 L of recombinant E. coli cultures upon 1 mM IPTG induction. No cytotoxic effects were observed during bacterial growth. C-terminal His-tagged rBSHs were subsequently purified using Ni-NTA agarose affinity chromatography and desalted. The purity of BSHs was assessed by Coomassie-blue staining of SDS-PAGE (Figure 2B). The molecular weights observed on SDS-PAGE corresponded with those expected (based on theoretical predictions) for rBSH 47 (37.1 kDa) and rBSH56 (35.8 kDa). However, the molecular weight for rBSH12 appeared slightly higher than theoretically expected (37.4 kDa). Nanodrop quantifications of desalted proteins showed that 35 mg of rBSH47 and 28 mg of rBSH56 were successfully purified from 1.5 L of culture. However, only 3 mg of rBSH12 could be recovered.
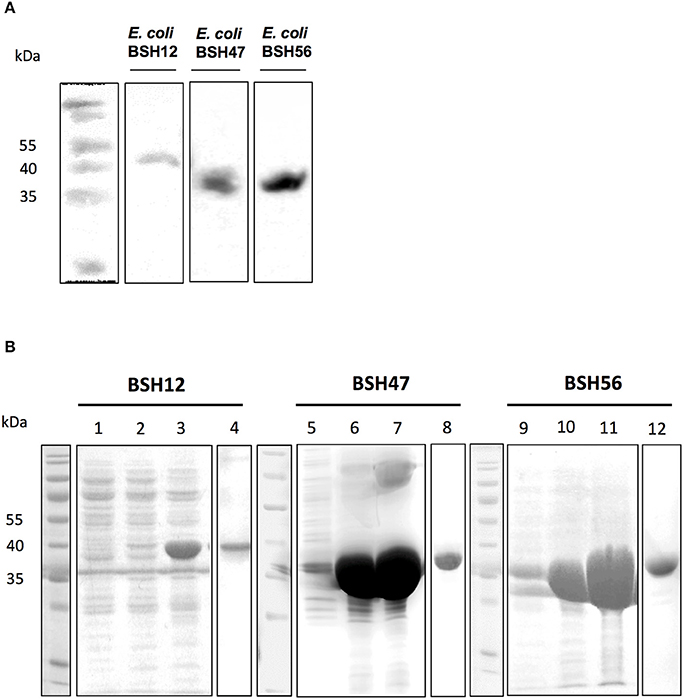
Figure 2. Expression and purification of L. johnsonii La1 BSHs. (A) Detection of heterologous production of BSH in E. coli by Western Blotting using anti-His tag antibody. Production of BSH was done under the control of pStaby system in E. coli (induced with 1 mM IPTG). Cytoplasmic fractions were extracted from E. coli SE1 harboring pLB490 plasmid (E. coli BSH12), pLB491 plasmid (E. coli BSH47) or pLB492 plasmid (E. coli BSH56). (B) SDS–PAGE analysis of purified BSHs. Purification steps of rBSH12, rBSH47, and rBSH56 with Ni-NTA affinity chromatography. Lanes 1–3 correspond to eluted fractions of rBSH12 with imidazole gradient (25, 75, and 500 mM). Lane 4 is rBSH12 after desalting. Lanes 5–7 correspond to eluted fractions of rBSH47 (imidazole gradient: 25, 75, and 500 mM); lane 8 is rBSH47 after desalting step. Lanes 9–11 correspond to eluted fractions of rBSH56 (imidazole gradient: 25, 75, and 500 mM); lane 12 is rBSH56 after desalting step.
L. johnsonii La1 BSH Activities and Substrate Specificities
The substrate specificities of the L. johnsonii BSHs were assayed by two approaches. Enzymatic activities were monitored in solution, using recombinant enzymes, by measuring amino acids released from the hydrolysis of conjugated bile salts as described in materials and methods. These enzymatic activity assays revealed a slight activity toward glycocholic acid, but a much higher level of activity toward taurocholic acid, for both rBSH47 and rBSH56 (Table 2). No significant enzymatic activity was detected with the purified rBSH12 with any substrate (Table 2). In parallel, the substrate specificities of the three L. johnsonii BSHs (produced in E. coli) were determined using LB agar supplemented with either taurodeoxycholic (0.3%) or glycodeoxycholic (0.3%) acids. A white and iridescent precipitate around colonies is indicative of BSH hydrolytic activity. E. coli SE1 strain expressing rBSH47 efficiently hydrolyzed tauro-conjugated bile salts, whereas no BSH activity was detected for glyco-conjugated bile salts (Figure 3A). E. coli SE1 strain expressing rBSH56 efficiently hydrolyzed both tauro- and glycol-conjugated bile salts (data not shown). A slight deconjugation was observed with E. coli strain producing rBSH12 against glyco-conjugated bile salts. However, BSH12 was not further tested in this study.
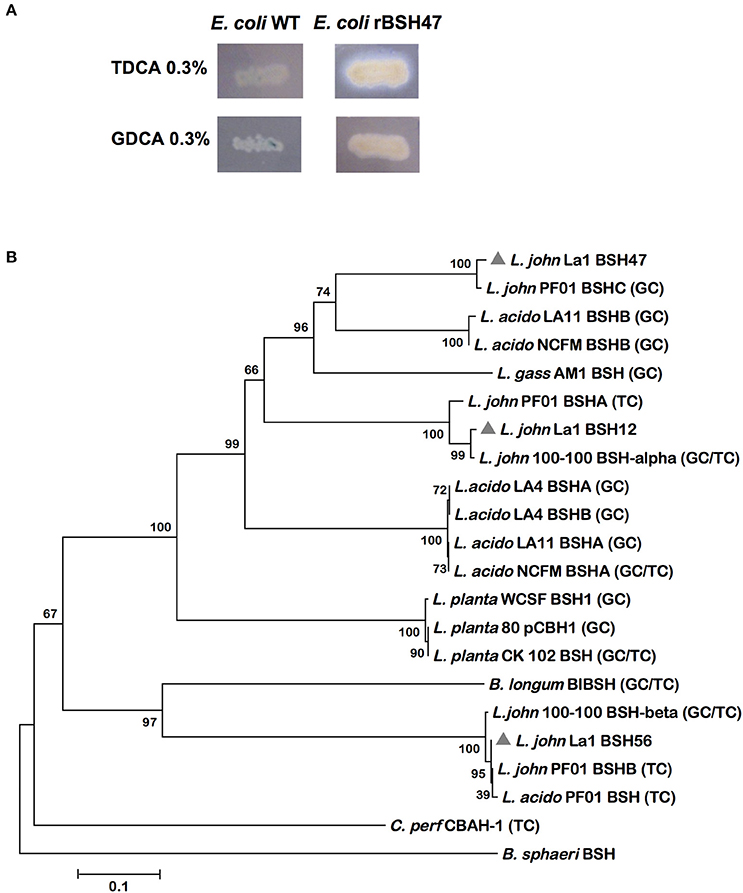
Figure 3. Substrate specificities of L. johnsonii La1 BSHs. (A) Hydrolase activity of rBSH47 produced in E. coli tested on LB plates. E. coli SE1 wild type or E. coli SE1 expressing rBSH47 were plated in the presence of taurodeoxycholic acid 0.3% (TDCA) or glycodeoxycholic acid 0.3% (GDCA) and incubated for 48–72 h. Activity is detected when a whitish halo forms around colonies. Data with E. coli SE1 expressing rBSH12 and rBSH56, respectively, are not shown. (B) Phylogenetic relationship among selected BSH sequences and substrate prediction. Programme MEGA5 was used for the phylogenetic tree and for the 500-replication bootstrap analysis. The following predicted amino acid sequences were obtained from UniProtKB/Swiss-Prot databases: BSH12, L. johnsonii La1 (Q74IV4_LACJO); BSH47, L. johnsonii La1 (Q74JG0_LACJO); BSH12, L. johnsonii La1 (Q74LX7_LACJO); CBAH-1, C. perfringens strain 13 (P54965.3); BSHA, L. acidophilus LA4 (ACL98173.1); BSHB, L. acidophilus LA4 (ACL98173.1); BSHA, L. acidophilus LA11 (ACL98175.1); BSHB, L. acidophilus LA11 (ACL98176.1); BSHA, L. acidophilus NCFM (YP_193782.1); BSHB, L. acidophilus NCFM (AAV42923.1); BSH, L. gasseri AM1 (ACL98172.1); BSH-α, L. johnsonii 100-100 (AAG22541.1); BSH-β, L. johnsonii 100-100 (AAC34381.1); BSHA, L. johnsonii pf01 (EGP12224.1); BSHB, L. johnsonii pf01 (EGP13287.1); BSHC, L. johnsonii pf01 (EGP12391.1); pCBH1, L. plantarum 80 (AAB24746.1); BSH1, L. plantarum WCSF (CCC80500.1); BlBSH, B. longum subsp. longum (2HF0); BSH, L. acidophilus PF01 (ABQ01980.1); BSH, L. plantarum CK 102 (Ha et al., 2006); Penicillin V Acylase (PVA), Bacillus sphaericus (3PVA). Substrate specificity, when known (see text for referenced literature) is indicated for each BSH enzyme (in brackets): (TC), specificity for tauro-conjugated bile salts; (GC), specificity for glyco-conjugated bile salts; (TC/GC), specificity for both tauro and glyco-conjugated bile salts.
Phylogenetic relationship among selected BSH sequences and related substrate predictions were represented on a neighbor-joining tree, constructed using amino acid sequences of BSHs whose substrate specificities have been previously characterized, with 500 bootstrap replications using MEGA5 software (http://www.megasoftware.net/). Such a phylogenetic analysis showed that L. johnsonii La1-BSH12 is more closely related to L. johnsonii 100-100-BSH-α (Figure 3B), an enzyme which is able to hydrolyze both tauro- and glyco-conjugated bile salts. The L. johnsonii La1-BSH56 is phylogenetically related to a compact cluster including L. johnsonii PF01-BSHB (LjBSHB), L. acidophilus PF01-BSH (LaBSH), and L. johnsonii 100-100-BSH-β (LjBSH-β). Both BSH56 and LjBSH-β display broad substrate specificity, with a slight preference for tauro-conjugated over glyco-conjugated bile salts, whereas LaBSH and LjBSHB exclusively hydrolyze tauro-conjugated bile salts (Chae et al., 2013). Finally, L. johnsonii La1-BSH47 was more closely related to L. johnsonii PF01-BSHC (LjBSHC) hydrolyzing only glyco-conjugated bile acids, whereas BSH47 displays a preference for tauro-conjugated substrates. These observations suggest that substrate specificities are not systematically conserved among lactobacilli BSHs, despite a good conservation of their 3D-structures and of key amino acids in their active sites, which makes substrate specificity prediction based on phylogenetic analysis not straightforward for the moment. Besides, enzymatically active BSHs from L. johnsonii La1 that could be measured here showed a higher activity for tauro-conjugated than glyco-conjugated substrates, whereas a clear preference has been observed for glyco-conjugated bile salts among other lactobacilli-BSHs characterized so far (Tanaka et al., 1999).
Enzymatic Activities of L. johnsonii La1 BSH47 and BSH56 Display Anti-giardial Effects
To evaluate the anti-giardial potential of purified L. johnsonii La1-BSHs, G. duodenalis WB6 trophozoites were incubated for 22 h in the presence of increasing concentrations of rBSH47 and rBSH56 from 2 × 10−5 to 17.4 μg/ml (rBSH12 was not tested for anti-giardial activity due to weak BSH-activity). Positive controls with C. perfringens BSH (Sigma-Aldrich) and negative experimental controls were set up in each independent inhibition assay. Treatments of Giardia trophozoites with BSHs showed a dose-dependent inhibition of the parasite growth in presence of bile, when compared to the controls without bile (Figures 4A,B). The IC50 of rBSH56 (IC50BSH56 = 0.018 ± 0.002 μg/ml) was slightly lower than that of rBSH47 (IC50BSH47 = 0.030 ± 0.003 μg/ml). Concentrations higher than 1 μg/ml of either rBSH47 or rBSH56, respectively, were sufficient to kill 100% of trophozoites in 22 h. Co-cultures of trophozoites and rBSHs in a growth medium without bile supplementation did not exhibit any toxic effects, further supporting the fact that the anti-giardial effect is mediated by BSH activity and requires the presence of an appropriate substrate (bile).
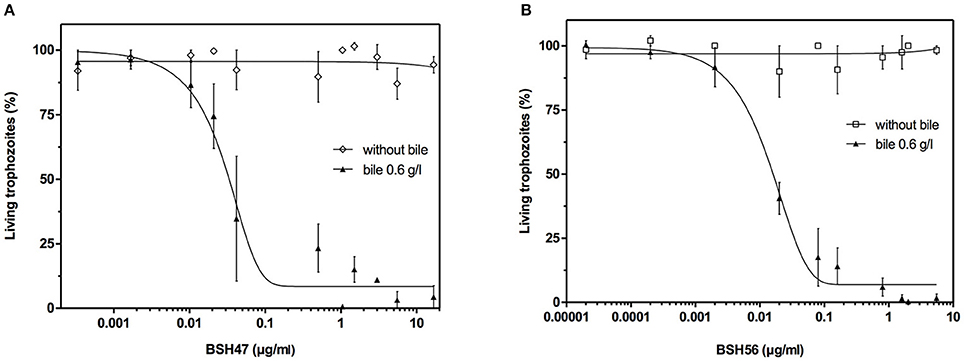
Figure 4. Anti-giardial activity of L. johnsonii La1 BSHs in vitro. Inhibition of G. duodenalis WB6 strain growth by enzymatically active rBSH47 (A) and rBSH56 (B) from L. johnsonii La1 with and without bovine bile supplementation (0.6 g/L) after 22 h of incubation. Increasing doses of BSH were tested ranging from 2 × 10−5 to 17.4 μg/ml. Values are in percentage ± SEM (standard error of the mean). Growth inhibition curves of G. duodenalis were calculated using Prism 5 software (GraphPad). IC50BSH56 = 0.018 ± 0.002 μg/ml; IC50BSH47 = 0.030 ± 0.003 μg/ml.
To better characterize the damages induced by BSHs activity, the morphology of G. duodenalis trophozoites WB6 was analyzed by SEM after 16 h of treatment with either rBSH56 (0.08 μg/ml), rBSH47 (0.5 μg/ml), or deoxycholic acid (DCA, 0.1 and 0.2 g/L), which is a major product of bile hydrolysis. SEM analysis of non-treated trophozoites showed the characteristic giardial tear-drop shape with no apparent sign of morphological alteration (Figures 5a,b). Trophozoites treated with either DCA, or rBSH56 or rBSH47 in presence of bile revealed significant structural damage when compared to controls (Figures 5c–h). BSH-treated parasites displayed several alterations such as protrusions and perforations at the surface of their plasma membrane (Figures 5d,f–h). In DCA-treated trophozoites, membrane and median body were dramatically disrupted (Figures 5g,h). In contrast, with both treatments, the ventral disk microtubule array was still observable.
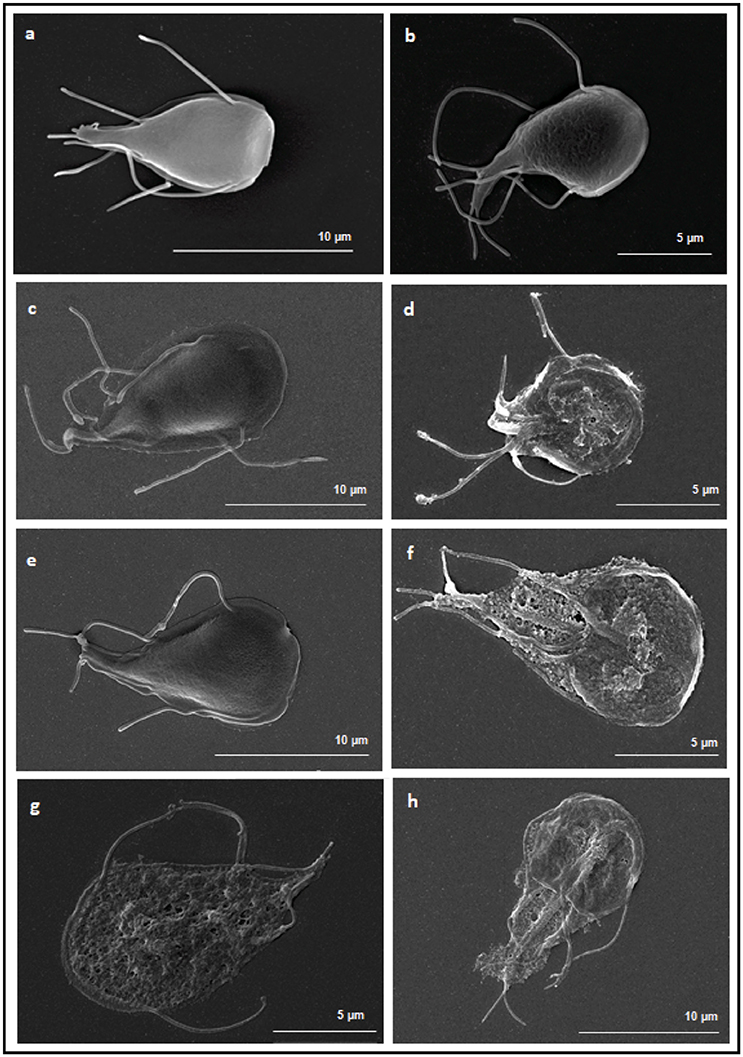
Figure 5. Morphological alterations following in vitro treatments of G. duodenalis by BSH or deoxycholic acid (DCA). Scanning electron microscopy of G. duodenalis trophozoites WB6 strain treated with either BSH47 (0.5 μg/ml), BSH56 (0.08 μg/ml) or DCA (0.1 and 0.2 g/L). (a) KM control (KM+ 10% FCS) and (b) KM control with bile (bovine bile 0.6 g/l) show the characteristic pear-shaped of trophozoites. (c) G. duodenalis treated with rBSH47and (d) G. duodenalis treated with rBSH47 with bile reveal altered morphology and plasma membrane disruption in presence of bile. (e) G. duodenalis treated with rBSH56 and (f) G. duodenalis treated with rBSH56 with bile showed similar cell lysis. (g,h) DCA-treated (0.1 and 0.2 g/l, respectively) Giardia present similar alterations and a disruption of plasma membrane exposing cell interior. Scale bar = 5 μm (b,d,f,g) or 10 μm (a,c,e,h).
Assessment of in Vivo Anti-giardial Activities of L. johnsonii La1 BSH47
Numerous studies have reported that bile acids conjugated to taurine are predominant in mice (Claus et al., 2011). Recombinant BSH47 efficiently hydrolyzed tauro-conjugated bile acids, and its efficacy against Giardia has been demonstrated in vitro. Since this enzyme was available in larger quantities compared to rBSH56 and rBSH12, rBSH47 was selected to evaluate the potential of rBSH to treat giardiasis in a murine model (Figure 6A). OF1 suckling mice were divided into four groups (n = 7–12). Mice were challenged with G. duodenalis WB6 trophozoites (1 × 105) at day 10 by intragastric gavage. Increasing doses of rBSH47 corresponding to 0.5, 5, and 50 μg (50 μl, diluted in NaHCO3 16.4%) were thawed and daily administered by intragastric gavage to neonatal mice from day 10 to 15. The control group received vehicle (PBS + NaHCO3 16.4%). Animals were sacrificed at day 16, corresponding to the peak of trophozoite colonization, and small intestinal contents were sampled and analyzed. Six days after inoculation, trophozoites were able to efficiently colonize and persist in the small intestine with a parasite load 20-fold higher than the inoculum (Figure 6B). In groups treated with rBSH47, the parasite burden decreased in a dose-dependent manner (Figure 6B). Interestingly, the highest dose of rBSH47 (50 μg daily for 5 days) induced a significant reduction of 68.8% of G. duodenalis trophozoites compared to the control group.
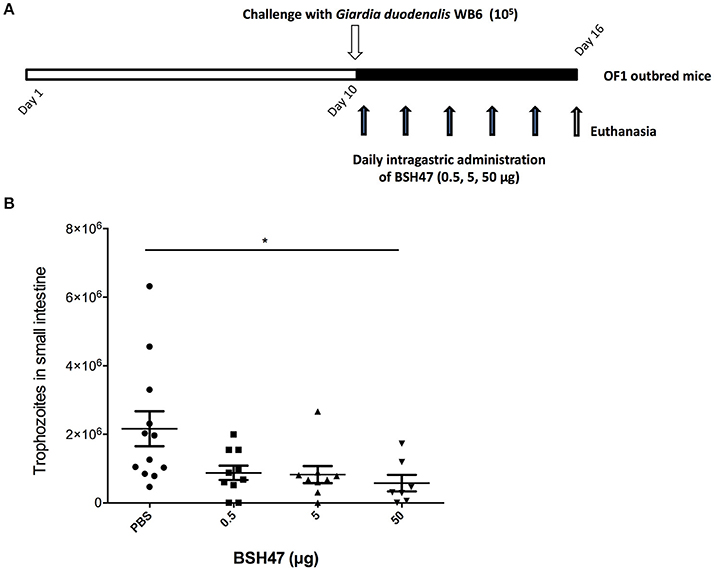
Figure 6. Anti-giardial activity of rBSH47 in vivo. (A) Experimental design of G. duodenalis infection in OF1 suckling mice model. Increasing doses of rBSH47 were daily administered by intragastric gavage (0.5, 5, 50 μg) to OF1 suckling mice from day 10 to 15. Control animals received PBS+ NaHCO3 16.4% (vehicle). Mice were challenged with G. duodenalis WB6 trophozoites (105) at day 10 by intragastric gavage. Animals were euthanized by cervical dislocation at day 16. (B) G. duodenalis trophozoites enumeration in small intestine after rBSH47-treatement. Group I (control): mice received vehicle (n = 12); Group II: mice received 0.5 μg of BSH47 daily for 5 days (n = 10); Group III: mice received 5 μg of BSH47 daily for 5 days (n = 9); mice received 50 μg of BSH47 daily for 5 days (n = 7). Small intestines were resuspended in PBS and trophozoites were counted using a hemocytometer. Values are mean ± SEM; p < 0.0001.
Discussion
L. johnsonii La1 is a probiotic strain with pathogen inhibition and host immunomodulation properties (Vidal et al., 2002; Cruchet et al., 2003; Pridmore et al., 2008). The activity of BSH and lactobacilli's bile resistance have been widely accepted as key factors for gut persistence and colonization by these bacteria (Tannock et al., 1994; Tanaka et al., 2000; Begley et al., 2006). Three bsh and two bile acid transporters genes were identified in the genome of L. johnsonii La1 (Pridmore et al., 2004). In this study, we cloned, purified, and characterized these 3 BSH enzymes in order to assess their antiprotozoal effect on Giardia. Nucleotide homology comparisons previously highlighted the similarities between L. johnsonii La1 bsh12 and bsh56 with cbsHα and cbsHβ from L. johnsonii 100-100, respectively (Elkins and Savage, 1998; Elkins et al., 2001; Pridmore et al., 2004). A neighbor-joining tree of various BSHs protein sequences from several lactic acid bacteria confirmed that L. johnsonii La1-BSH enzymes are phylogenetically related to BSHs from other species. In addition, they share a high degree of similarity to various sub-groups of BSHs; for instance, BSH12 shares 99% identity with L. johnsonii 100-100 cbsHα and L. johnsonii PF01 BSHA at amino acid level, and BSH56 shares 99% identity with L. johnsonii 100-100 cbsHβ and 98% with L. johnsonii PF01 BSHB at amino acid level. L. johnsonii La1 and L. johnsonii PF01 both possess a third BSH gene, bsh47 and bshC, respectively, which is absent from L. johnsonii 100-100. Besides, the close relationship among L. johnsonii La1 BSH47, L. johnsonii PF01 BSHC, and L. acidophilus NCFM BSHB, at amino acid level, suggests that these enzymes likely share a common ancestor. These observations contribute to the idea that BSH might have been acquired through horizontal gene transfer from microorganisms sharing the same intestinal environment (Corzo and Gilliland, 1999; Franz et al., 2001; McAuliffe et al., 2005; Begley et al., 2006), although this latter hypothesis remains to be tested.
Multiple amino acid sequence alignment of L. johnsonii La1 BSHs indicated a high variability among characterized BSHs; however, well-conserved motifs were observed around residues involved in the active site (Cys-2, Arg-18, Asp-21, Tyr-82, Asn-172, and Arg-225). These observations are in agreement with previous studies (Rossocha et al., 2005; Fang et al., 2009; Lin et al., 2014) and highlight that the biological functions of BSHs are strictly conserved despite sequence/phenotypic variabilities. BSHs (EC 3.5.1.24) belong to N-terminal nucleophilic (Ntn) hydrolases, a superfamily of enzymes containing N-terminal cysteine residue involved in the catalytic site (Suresh et al., 1999; Kim et al., 2004). Penicillin V acylases (EC 3.5.1.11), which are closely related to BSH, also belong to Ntn hydrolases and share similar structures. In silico modeling, BSH47, BSH56, and BSH12 showed that the typical αββα tertiary structure arrangement, which is characteristic of Ntn superfamily, is conserved (Oinonen and Rouvinen, 2000; Patel et al., 2010; Lin et al., 2014). Taken together, these structural observations indicate that the folding of BSHs remained stable during evolution despite low sequence identity, which suggest a high evolutionary pressure to maintain their functionality (Chothia and Lesk, 1986; Sander and Schneider, 1991; Krieger et al., 2003).
Experimental determination of BSH activities showed that at least 2 of the 3 BSHs from L. johnsonii La1 have broad substrate specificities. The enzyme BSH56 exhibited high hydrolysis activities toward tauro- and glyco-conjugated bile salts, with a preference toward tauro-conjugated substrates. Interestingly, BSH56 belongs to a phylogenetic cluster of BSH enzymes exhibiting activity exclusively directed toward tauro-conjugated bile acids. Similarly, BSH47 was more efficient at hydrolyzing tauro-conjugated bile salts and exhibited a very low relative activity toward glyco-conjugated bile salts. Surprisingly, the amino acid sequence of BSH47 is more closely related to that of L. johnsonii BSHC that has been reported to hydrolyze glyco-conjugated bile salts (Chae et al., 2013). Our results, therefore, contrast with previous studies reporting that most BSH enzymes isolated from lactobacilli are very efficient at hydrolyzing glyco-conjugated bile salts (Coleman and Hudson, 1995; Liong and Shah, 2005). In addition, these observations point out the limits of substrate predictions based on phylogenetic relationships considering whole enzyme sequences. The mechanisms responsible for substrate specificity of BSH enzymes are still unclear. Putative amino acids have been associated with substrate binding pockets in C. perfringens (Rossocha et al., 2005; Ridlon et al., 2006), but these residues are different in all 3 BSHs from L. johnsonii. Several studies emphasized that BSH preferably recognize conjugated substrates at amino acid moieties (Coleman and Hudson, 1995; Tanaka et al., 2000; Kim et al., 2004; Rossocha et al., 2005), whereas others still suggest that steroid moieties are recognized in priority (Moser and Savage, 2001; Begley et al., 2006; Patel et al., 2010) which could explain broad substrate specificities. Experimentally solving the 3D structures of rBSH47 and rBSH56 harboring appropriate substrates would certainly provide invaluable information regarding this important question.
The third BSH isolated from L. johnsonii La1, BSH12, was successfully expressed in E. coli and clearly observed in SDS-PAGE and by Western blotting, but no specific activity could be detected toward either substrate in liquid tests. However, a slight positive signal was observed with E. coli strain-expressing rBHS12 on agar plates supplemented with glyco-specific bile salts, suggesting a weak glyco-specific activity. Similar difficulties to express recombinant BSHs have been observed with BSHs from L. plantarum JPP2 (Ren et al., 2011) and BSH2 from L. plantarum WCFS1 (Lambert et al., 2008). Proteolytic degradation and misfolding of the recombinant protein produced in E. coli may have affected the enzyme's function (Baneyx and Mujacic, 2004). The functionality of BSH12 is, therefore, still under investigation.
As mentioned previously, the putative natural role of BSHs is to decrease the toxicity of conjugated bile salts for bacterial cells (De Smet et al., 1995). In this work, we assessed the anti-parasitic activity of recombinant BSHs and we demonstrated that rBSH47 and rBSH56 were highly active against viable trophozoites of G. duodenalis strains WB6 and NF. The minimum concentration found to kill 100% of G. duodenalis WB6 trophozoites in vitro was 1 μg/mL for both rBSH47 and rBSH56. When tested on human enterocyte Caco-2 cells, both rBSH47 and rBSH56 inhibited the growth of G. duodenalis NF in the presence of bile (0.6 g/L) in a dose-dependent fashion and prevented the attachment of the parasites to the cell monolayers (Figure S3). The rBSH56 was more effective than rBSH47 in killing both G. duodenalis WB6 and NF strains, although it induced more cell damages to Caco-2 cells at high concentrations (Figure S3). Recombinant BSH47 was, therefore, chosen to assess the in vivo effectiveness of BSH to treat Giardia infection in a suckling murine model. Moreover, given that bile acids are predominantly conjugated to taurine in mice (Claus et al., 2011), the ability of BSH47 to preferentially hydrolyze tauro-conjugated bile salts seemed more appropriate. We observed that rBSH47 inhibited Giardia growth in a dose-dependent manner in vivo, in keeping with the data obtained in vitro. At the highest dose (50 μg/mice/day) administered daily for 5 days, the parasite (trophozoite) burden was significantly reduced by 68.8% in the small intestine of the mice. Despite this important decrease of the parasite load, none of the treated mice were free of parasites at 16 days post-inoculation. The anti-giardial effects mediated by rBSH47 in vivo were, therefore, modest compared to their efficacy in the in vitro assays. This can be explained by a partial degradation of BSHs by acidic proteases and peptidases in the stomach. The activity of BSHs correlates with the amount of conjugated bile salts released in the duodenum, which is highly variable in neonates (Heubi et al., 2007). The production of deconjugated bile salts at inhibiting levels is, therefore, dependent to the bioavailability of BSH in the small intestine.
It has been reported that bile salts, and more specifically conjugated bile salts, have growth promoting effects on G. duodenalis in vivo (Halliday et al., 1988). Indeed, bile uptake contributes to cholesterol and exogenous phospholipids needs, which are essentials for parasite growth (Yichoy et al., 2011). So far, to our knowledge, there is no evidence showing that Giardia is able to deconjugate bile salts. Conjugated bile salts are, thus, directly consumed by Giardia without being detoxified (Farthing et al., 1985). In contrast, bacterial deconjugation aims at reducing the detergent properties of conjugated bile salts, which are more toxic for bacterial cells than secondary bile salts, i.e., cholic acid (CA), deoxycholic acid (DCA), and chenodeoxycholic acid (CDCA) (De Boever and Verstraete, 1999). It is likely that detoxification of bile salts by the duodenal microbiota has a collateral effect on parasite survival. Earlier work carried out in our lab showed that DCA and CDCA exerted cytotoxic effects on G. duodenalis trophozoites in vitro at non-micellar concentrations (Travers et al., 2016). Therefore, we investigated the morphological perturbations induced by DCA and BSHs on trophozoite cultures and we noticed both induced degenerations and perforations of the parasite plasma membrane. It has been established that DCA perturbs eukaryotic membranes structure by altering the membrane lipid microdomains (Jean-Louis et al., 2006). Furthermore, secondary bile salts induced a redistribution of cholesterol and decrease membrane fluidity. Hence, we hypothesized that secondary bile salt, and more specifically DCA, would kill Giardia trophozoites by damaging the cell structure. In the upper parts of the small intestine, where bile salt deconjugation occurs at high rate, the Gram-positive bacteria might be protected against secondary deconjugated bile salts by cell wall peptidoglycan.
A major side effect of BSH-based treatment would be a shift of bile salt balance in the gut. It has been reported in previous studies that an enhancement of BSH activity might impact host physiology by disturbing fat digestion and lipid metabolism (Begley et al., 2006; Lin et al., 2014). Moreover, secondary bile acids resulting from the deconjugation of bile salts have also been linked to DNA damage in bacterial and host cells, colon cancer, and inflammation (Cheah and Bernstein, 1990; Moser and Savage, 2001; Bernstein et al., 2005). On the other hand, BSH activity is a natural process that plays a central role in the reduction of cholesterol (Jones et al., 2013).
The aim of this study was to evaluate the anti-giardial potential of BSHs against G. duodenalis. We expressed for the first time the BSHs isolated from the probiotic strain L. johnsonii La1 and showed that rBSH47 and rBSH56 exhibited high specific activity and broad substrate specificities. Antiprotozoal assays demonstrated that BSHs were highly effective against G. duodenalis in vitro and in vivo and represent a promising therapeutic strategy based on their natural catalytic activity. Future studies will determine whether such treatment approaches should be of short duration in order to avoid putative side effects related to the enhancement of bile salt deconjugation. Besides, this anti-giardial effect can be extended to any BSH activity as long as it efficiently converts conjugated bile salts into their deconjugated counterparts. However, further works are still needed to investigate the positive impact of such treatment on the pathophysiology of giardiasis, including protective effects on epithelial permeability, mucosal injury, and malfunction. Moreover, newer galenic formulations are needed in order to provide a better persistence of rBSHs in vivo, which can improve health outcomes in routine clinical and veterinary usages.
Author Contributions
IF, PG, BP, TA, and LB-H conceived and designed the study. TA, IF, and LB-H produced and isolated the recombinant BSH, performed the biochemical characterizations with SC and the in silico analyses. TA, SC, AB, and IF performed the Giardia assays in vitro and SEM. TA, MT, and BP performed the Giardia assays in the suckling mice model. IV, PL, and PG discussed the experiments and results. TA, IF, and LB-H wrote the manuscript with contributions from all authors.
Funding
This work was partially funded by Region Ile de France-DIM (Maladies Infectieuses Parasitaires et Nosocomiales Emergentes, programm n° 120092).
Conflict of Interest Statement
The authors declare that the research was conducted in the absence of any commercial or financial relationships that could be construed as a potential conflict of interest.
Acknowledgments
We deeply thank Geraldine Toutirais (PTME, Plateau Technique de Microscopie Électronique et de Microanalyses du Museum National d'Histoire Naturelle, Paris) for assistance with SEM imaging. We thank Jasmine Kirati (UMR BIPAR, Anses, ENVA, INRA) for help with in vivo assays and Olivier Berteau (Micalis Institute, INRA) for assistance in protein purifications.
Supplementary Material
The Supplementary Material for this article can be found online at: https://www.frontiersin.org/articles/10.3389/fmicb.2017.02707/full#supplementary-material
Figure S1. Protein structure prediction of L. johnsonii La1 BSHs. Protein structures were modeled for L. johnsonii BSH56 (a) and BSH47 (b) using I-TASSER software (http://zhanglab.ccmb.med.umich.edu/I-TASSER/). CBAH-1 (PDB: 2BJF) from C. perfringens (Rossocha et al., 2005) and BlBSH from B. longum (Kumar et al., 2006) (PDB: 2HF0) were used as templates for modeling BSH47 and BSH56, respectively. Best values of the C-score were chosen for model structure prediction. Visualization of predicted structures for BSH47 (red, a) and BSH56 (blue, b) and superimposition (c,d) were performed using Pymol software (https://www.pymol.org/).
Figure S2. Protein structure prediction of L. johnsonii La1 BSH12. Protein structure was modeled for L. johnsonii BSH12 using I-TASSER software (http://zhanglab.ccmb.med.umich.edu/I-TASSER/). Bile salt hydrolase (PDB: 2RF8) from C. perfringens was used as a template for modeling. Best value of the C-score was chosen for model structure prediction. Visualization of predicted structure was performed using Pymol software (https://www.pymol.org/).
Figure S3. Anti-giardial effect of BSH in co-culture with Caco2 cells. To further investigate the effect of L. johnsonii La1-BSHs on the adherence of Giardia enterocytes, differentiated Caco-2 cells were co-incubated with fresh trophozoites cultures of G. duodenalis NF strain and treated with either rBSH47 or rBSH56, over a range of concentrations from 0.005 to 1 μg/ml. G. duodenalis NF strain trophozoites were inoculated to Caco2 cells at a multiplicity of infection (MOI) of 10:1 in the presence of increasing doses of rBSH47, rBSH56 or DMEM. Anti-giardial activity assays were performed with bovine bile (0.6 g/l) added to the medium. The results are expressed as relative percentage of growth compared to untreated trophozoites as negative control. Data are presented as mean ± SEM and correspond to triplicates. As expected from previous experiments done with the G. duodenalis WB6 strain, both BSHs triggered a growth inhibition of G. duodenalis NF trophozoites, in a dose-dependent fashion, after 20 h of exposure (this figure). In contrast, high concentrations of rBSH in assays induced cell damages on Caco-2 monolayer (data not shown). This phenomenon might be due to a putative cytotoxicity of deconjugated bile salt. Interestingly, neither rBSH47 nor rBSH56 displayed cytotoxic effects when tested in absence of bile (data not shown).
References
Ankarklev, J., Jerlstrom-Hultqvist, J., Ringqvist, E., Troell, K., and Svard, S. G. (2010). Behind the smile: cell biology and disease mechanisms of Giardia species. Nat. Rev. Microbiol. 8, 413–422. doi: 10.1038/nrmicro2317
Ansell, B. R., McConville, M. J., Ma'ayeh, S. Y., Dagley, M. J., Gasser, R. B., Svard, S. G., et al. (2015). Drug resistance in Giardia duodenalis. Biotechnol. Adv. 33, 888–901. doi: 10.1016/j.biotechadv.2015.04.009
Baneyx, F., and Mujacic, M. (2004). Recombinant protein folding and misfolding in Escherichia coli. Nat. Biotechnol. 22, 1399–1408. doi: 10.1038/nbt1029
Barr, S. C., Bowman, D. D., and Heller, R. L. (1994). Efficacy of fenbendazole against giardiasis in dogs. Am. J. Vet. Res. 55, 988–990.
Begley, M., Hill, C., and Gahan, C. G. (2006). Bile salt hydrolase activity in probiotics. Appl. Environ. Microbiol. 72, 1729–1738. doi: 10.1128/AEM.72.3.1729-1738.2006
Bernstein, H., Bernstein, C., Payne, C. M., Dvorakova, K., and Garewal, H. (2005). Bile acids as carcinogens in human gastrointestinal cancers. Mutat. Res. 589, 47–65. doi: 10.1016/j.mrrev.2004.08.001
Chae, J. P., Valeriano, V. D., Kim, G. B., and Kang, D. K. (2013). Molecular cloning, characterization and comparison of bile salt hydrolases from Lactobacillus johnsonii PF01. J. Appl. Microbiol. 114, 121–133. doi: 10.1111/jam.12027
Cheah, P. Y., and Bernstein, H. (1990). Modification of DNA by bile acids: a possible factor in the etiology of colon cancer. Cancer Lett. 49, 207–210. doi: 10.1016/0304-3835(90)90160-Y
Chothia, C., and Lesk, A. M. (1986). The relation between the divergence of sequence and structure in proteins. EMBO J. 5, 823–826.
Claus, S. P., Ellero, S. L., Berger, B., Krause, L., Bruttin, A., Molina, J., et al. (2011). Colonization-induced host-gut microbial metabolic interaction. MBio 2, e00271–00210. doi: 10.1128/mBio.00271-10
Coleman, J. P., and Hudson, L. L. (1995). Cloning and characterization of a conjugated bile acid hydrolase gene from Clostridium perfringens. Appl. Environ. Microbiol. 61, 2514–2520.
Corzo, G., and Gilliland, S. E. (1999). Bile salt hydrolase activity of three strains of Lactobacillus acidophilus. J. Dairy Sci. 82, 472–480. doi: 10.3168/jds.S0022-0302(99)75256-2
Cotton, J. A., Beatty, J. K., and Buret, A. G. (2011). Host parasite interactions and pathophysiology in giardia infections. Int. J. Parasitol. 41, 925–933. doi: 10.1016/j.ijpara.2011.05.002
Cruchet, S., Obregon, M. C., Salazar, G., Diaz, E., and Gotteland, M. (2003). Effect of the ingestion of a dietary product containing Lactobacillus johnsonii La1 on Helicobacter pylori colonization in children. Nutrition 19, 716–721. doi: 10.1016/S0899-9007(03)00109-6
De Boever, P., and Verstraete, W. (1999). Bile salt deconjugation by Lactobacillus plantarum 80 and its implication for bacterial toxicity. J. Appl. Microbiol. 87, 345–352. doi: 10.1046/j.1365-2672.1999.00019.x
De Smet, I., Van Hoorde, L., Vande Woestyne, M., Christiaens, H., and Verstraete, W. (1995). Significance of bile salt hydrolytic activities of lactobacilli. J. Appl. Bacteriol. 79, 292–301. doi: 10.1111/j.1365-2672.1995.tb03140.x
Elkins, C. A., and Savage, D. C. (1998). Identification of genes encoding conjugated bile salt hydrolase and transport in Lactobacillus johnsonii 100-100. J. Bacteriol. 180, 4344–4349.
Elkins, C. A., Moser, S. A., and Savage, D. C. (2001). Genes encoding bile salt hydrolases and conjugated bile salt transporters in Lactobacillus johnsonii 100-100 and other Lactobacillus species. Microbiology 147, 3403–3412. doi: 10.1099/00221287-147-12-3403
Eyssen, H. (1973). Role of the gut microflora in metabolism of lipids and sterols. Proc. Nutr. Soc. 32, 59–63. doi: 10.1079/PNS19730016
Fang, F., Li, Y., Bumann, M., Raftis, E. J., Casey, P. G., Cooney, J. C., et al. (2009). Allelic variation of bile salt hydrolase genes in Lactobacillus salivarius does not determine bile resistance levels. J. Bacteriol. 191, 5743–5757. doi: 10.1128/JB.00506-09
Farthing, M. J., Keusch, G. T., and Carey, M. C. (1985). Effects of bile and bile salts on growth and membrane lipid uptake by Giardia lamblia. Possible implications for pathogenesis of intestinal disease. J. Clin. Invest. 76, 1727–1732. doi: 10.1172/JCI112162
Franz, C. M., Specht, I., Haberer, P., and Holzapfel, W. H. (2001). Bile salt hydrolase activity of Enterococci isolated from food: screening and quantitative determination. J. Food Prot. 64, 725–729. doi: 10.4315/0362-028X-64.5.725
Gardner, T. B., and Hill, D. R. (2001). Treatment of giardiasis. Clin. Microbiol. Rev. 14, 114–128. doi: 10.1128/CMR.14.1.114-128.2001
Goyal, N., Rishi, P., and Shukla, G. (2013). Lactobacillus rhamnosus GG antagonizes Giardia intestinalis induced oxidative stress and intestinal disaccharidases: an experimental study. World J. Microbiol. Biotechnol. 29, 1049–1057. doi: 10.1007/s11274-013-1268-6
Grill, J. P., Cayuela, C., Antoine, J. M., and Schneider, F. (2000). Isolation and characterization of a Lactobacillus amylovorus mutant depleted in conjugated bile salt hydrolase activity: relation between activity and bile salt resistance. J. Appl. Microbiol. 89, 553–563. doi: 10.1046/j.1365-2672.2000.01147.x
Ha, C.-G., Cho, J.-K., Chai, Y.-G., Ha, Y.-A., and Shin, S.-H. (2006). Purification and characterization of bile salt hydrolase from Lactobacillus plantarum CK 102. J. Microbiol. Biotechnol. 16, 1047–1052.
Halliday, C. E., Clark, C., and Farthing, M. J. (1988). Giardia-bile salt interactions in vitro and in vivo. Trans. R. Soc. Trop. Med. Hyg. 82, 428–432. doi: 10.1016/0035-9203(88)90153-8
Heubi, J. E., Setchell, K. D., and Bove, K. E. (2007). Inborn errors of bile acid metabolism. Semin. Liver Dis. 27, 282–294. doi: 10.1055/s-2007-985073
Humen, M. A., De Antoni, G. L., Benyacoub, J., Costas, M. E., Cardozo, M. I., Kozubsky, L., et al. (2005). Lactobacillus johnsonii La1 antagonizes giardia intestinalis in vivo. Infect. Immun. 73, 1265–1269. doi: 10.1128/IAI.73.2.1265-1269.2005
Jean-Louis, S., Akare, S., Ali, M. A., Mash, E. A. Jr., and Meuillet, E. (2006). Deoxycholic acid induces intracellular signaling through membrane perturbations. J. Biol. Chem. 281, 14948–14960. doi: 10.1074/jbc.M506710200
Jones, M. L., Tomaro-Duchesneau, C., Martoni, C. J., and Prakash, S. (2013). Cholesterol lowering with bile salt hydrolase-active probiotic bacteria, mechanism of action, clinical evidence, and future direction for heart health applications. Expert Opin. Biol. Ther. 13, 631–642. doi: 10.1517/14712598.2013.758706
Kim, G. B., and Lee, B. H. (2008). Genetic analysis of a bile salt hydrolase in Bifidobacterium animalis subsp. lactis KL612. J. Appl. Microbiol. 105, 778–790. doi: 10.1111/j.1365-2672.2008.03825.x
Kim, G. B., Brochet, M., and Lee, B. H. (2005). Cloning and characterization of a bile salt hydrolase (bsh) from Bifidobacterium adolescentis. Biotechnol. Lett. 27, 817–822. doi: 10.1007/s10529-005-6717-3
Kim, G. B., Yi, S. H., and Lee, B. H. (2004). Purification and characterization of three different types of bile salt hydrolases from Bifidobacterium strains. J. Dairy Sci. 87, 258-266. doi: 10.3168/jds.S0022-0302(04)73164-1
Krieger, F., Fierz, B., Bieri, O., Drewello, M., and Kiefhaber, T. (2003). Dynamics of unfolded polypeptide chains as model for the earliest steps in protein folding. J. Mol. Biol. 332, 265–274. doi: 10.1016/S0022-2836(03)00892-1
Kumar, R. S., Brannigan, J. A., Prabhune, A. A., Pundle, A. V., Dodson, G. G., Dodson, E. J., et al. (2006). Structural and functional analysis of a conjugated bile salt hydrolase from Bifidobacterium longum reveals an evolutionary relationship with penicillin V acylase. J. Biol. Chem. 281, 32516–32525. doi: 10.1074/jbc.M604172200
Lambert, J. M., Bongers, R. S., De Vos, W. M., and Kleerebezem, M. (2008). Functional analysis of four bile salt hydrolase and penicillin acylase family members in Lactobacillus plantarum WCFS1. Appl. Environ. Microbiol. 74, 4719–4726. doi: 10.1128/AEM.00137-08
Lane, S., and Lloyd, D. (2002). Current trends in research into the waterborne parasite giardia. Crit. Rev. Microbiol. 28, 123–147. doi: 10.1080/1040-840291046713
Lin, J. (2014). Antibiotic growth promoters enhance animal production by targeting intestinal bile salt hydrolase and its producers. Front. Microbiol. 5:33. doi: 10.3389/fmicb.2014.00033
Lin, J., Negga, R., Zeng, X., and Smith, K. (2014). Effect of bile salt hydrolase inhibitors on a bile salt hydrolase from Lactobacillus acidophilus. Pathogens 3, 947–956. doi: 10.3390/pathogens3040947
Liong, M. T., and Shah, N. P. (2005). Acid and bile tolerance and cholesterol removal ability of lactobacilli strains. J. Dairy Sci. 88, 55–66. doi: 10.3168/jds.S0022-0302(05)72662-X
McAuliffe, O., Cano, R. J., and Klaenhammer, T. R. (2005). Genetic analysis of two bile salt hydrolase activities in Lactobacillus acidophilus NCFM. Appl. Environ. Microbiol. 71, 4925–4929. doi: 10.1128/AEM.71.8.4925-4929.2005
Moser, S. A., and Savage, D. C. (2001). Bile salt hydrolase activity and resistance to toxicity of conjugated bile salts are unrelated properties in lactobacilli. Appl. Environ. Microbiol. 67, 3476–3480. doi: 10.1128/AEM.67.8.3476-3480.2001
Oinonen, C., and Rouvinen, J. (2000). Structural comparison of Ntn-hydrolases. Protein Sci. 9, 2329–2337. doi: 10.1110/ps.9.12.2329
Patel, A. K., Singhania, R. R., Pandey, A., and Chincholkar, S. B. (2010). Probiotic bile salt hydrolase: current developments and perspectives. Appl. Biochem. Biotechnol. 162, 166–180. doi: 10.1007/s12010-009-8738-1
Perez, P. F., Minnaard, J., Rouvet, M., Knabenhans, C., Brassart, D., De Antoni, G. L., et al. (2001). Inhibition of giardia intestinalis by extracellular factors from lactobacilli: an in vitro study. Appl. Environ. Microbiol. 67, 5037–5042. doi: 10.1128/AEM.67.11.5037-5042.2001
Petri, W. A. (2005). Treatment of giardiasis. Curr. Treat. Options Gastroenterol. 8, 13–17. doi: 10.1007/s11938-005-0047-3
Platts-Mills, J. A., Babji, S., Bodhidatta, L., Gratz, J., Haque, R., Havt, A., et al. (2015). Pathogen-specific burdens of community diarrhoea in developing countries: a multisite birth cohort study (MAL-ED). Lancet Glob. Health 3, e564–e575. doi: 10.1016/S2214-109X(15)00151-5
Pridmore, R. D., Berger, B., Desiere, F., Vilanova, D., Barretto, C., Pittet, A. C., et al. (2004). The genome sequence of the probiotic intestinal bacterium Lactobacillus johnsonii NCC 533. Proc. Natl. Acad. Sci. U.S.A. 101, 2512–2517. doi: 10.1073/pnas.0307327101
Pridmore, R. D., Pittet, A. C., Praplan, F., and Cavadini, C. (2008). Hydrogen peroxide production by Lactobacillus johnsonii NCC 533 and its role in anti-Salmonella activity. FEMS Microbiol. Lett. 283, 210–215. doi: 10.1111/j.1574-6968.2008.01176.x
Ren, J., Sun, K., Wu, Z., Yao, J., and Guo, B. (2011). All 4 bile salt hydrolase proteins are responsible for the hydrolysis activity in Lactobacillus plantarum ST-III. J. Food Sci. 76, M622–M628. doi: 10.1111/j.1750-3841.2011.02431.x
Ridlon, J. M., Kang, D. J., and Hylemon, P. B. (2006). Bile salt biotransformations by human intestinal bacteria. J. Lipid Res. 47, 241–259. doi: 10.1194/jlr.R500013-JLR200
Rossocha, M., Schultz-Heienbrok, R., Von Moeller, H., Coleman, J. P., and Saenger, W. (2005). Conjugated bile acid hydrolase is a tetrameric N-terminal thiol hydrolase with specific recognition of its cholyl but not of its tauryl product. Biochemistry 44, 5739–5748. doi: 10.1021/bi0473206
Roy, A., Kucukural, A., and Zhang, Y. (2010). I-TASSER: a unified platform for automated protein structure and function prediction. Nat. Protoc. 5, 725–738. doi: 10.1038/nprot.2010.5
Ruiz, L., Margolles, A., and Sanchez, B. (2013). Bile resistance mechanisms in Lactobacillus and Bifidobacterium. Front. Microbiol. 4:396. doi: 10.3389/fmicb.2013.00396
Sander, C., and Schneider, R. (1991). Database of homology-derived protein structures and the structural meaning of sequence alignment. Proteins 9, 56-68. doi: 10.1002/prot.340090107
Savioli, L., Smith, H., and Thompson, A. (2006). Giardia and Cryptosporidium join the ‘neglected diseases initiative’. Trends Parasitol. 22, 203–208. doi: 10.1016/j.pt.2006.02.015
Shukla, G., and Sidhu, R. K. (2011). Lactobacillus casei as a probiotic in malnourished Giardia lamblia-infected mice: a biochemical and histopathological study. Can. J. Microbiol. 57, 127–135. doi: 10.1139/W10-110
Shukla, G., Devi, P., and Sehgal, R. (2008). Effect of Lactobacillus casei as a probiotic on modulation of giardiasis. Dig. Dis. Sci. 53, 2671–2679. doi: 10.1007/s10620-007-0197-3
Singer, S. M., Elmendorf, H. G., Conrad, J. T., and Nash, T. E. (2001). Biological selection of variant-specific surface proteins in Giardia lamblia. J. Infect. Dis. 183, 119–124. doi: 10.1086/317659
Suresh, C. G., Pundle, A. V., Sivaraman, H., Rao, K. N., Brannigan, J. A., McVey, C. E., et al. (1999). Penicillin V acylase crystal structure reveals new Ntn-hydrolase family members. Nat. Struct. Biol. 6, 414–416. doi: 10.1038/8213
Tamura, K., Peterson, D., Peterson, N., Stecher, G., Nei, M., and Kumar, S. (2011). MEGA5: molecular evolutionary genetics analysis using maximum likelihood, evolutionary distance, and maximum parsimony methods. Mol. Biol. Evol. 28, 2731–2739. doi: 10.1093/molbev/msr121
Tanaka, H., Doesburg, K., Iwasaki, T., and Mierau, I. (1999). Screening of lactic acid bacteria for bile salt hydrolase activity. J. Dairy Sci. 82, 2530–2535. doi: 10.3168/jds.S0022-0302(99)75506-2
Tanaka, H., Hashiba, H., Kok, J., and Mierau, I. (2000). Bile salt hydrolase of Bifidobacterium longum-biochemical and genetic characterization. Appl. Environ. Microbiol. 66, 2502–2512. doi: 10.1128/AEM.66.6.2502-2512.2000
Tannock, G. W., Luchansky, J. B., Miller, L., Connell, H., Thode-Andersen, S., Mercer, A. A., et al. (1994). Molecular characterization of a plasmid-borne (pGT633) erythromycin resistance determinant (ermGT) from Lactobacillus reuteri 100-63. Plasmid 31, 60–71. doi: 10.1006/plas.1994.1007
Travers, M. A., Florent, I., Kohl, L., and Grellier, P. (2011). Probiotics for the control of parasites: an overview. J. Parasitol. Res. 2011:610769. doi: 10.1155/2011/610769
Travers, M. A., Sow, C., Zirah, S., Deregnaucourt, C., Chaouch, S., Queiroz, R. M., et al. (2016). Deconjugated bile salts produced by extracellular bile-salt hydrolase-like activities from the probiotic lactobacillus johnsonii La1 inhibit Giardia duodenalis in vitro growth. Front. Microbiol. 7:1453. doi: 10.3389/fmicb.2016.01453
Vidal, K., Donnet-Hughes, A., and Granato, D. (2002). Lipoteichoic acids from Lactobacillus johnsonii strain La1 and Lactobacillus acidophilus strain La10 antagonize the responsiveness of human intestinal epithelial HT29 cells to lipopolysaccharide and gram-negative bacteria. Infect. Immun. 70, 2057–2064. doi: 10.1128/IAI.70.4.2057-2064.2002
Keywords: Giardia duodenalis, lactobacilli, Lactobacillus johnsonii, bile salt hydrolases, BSH, conjugated bile salts, anti-giardial activity
Citation: Allain T, Chaouch S, Thomas M, Vallée I, Buret AG, Langella P, Grellier P, Polack B, Bermúdez-Humarán LG and Florent I (2018) Bile-Salt-Hydrolases from the Probiotic Strain Lactobacillus johnsonii La1 Mediate Anti-giardial Activity in Vitro and in Vivo. Front. Microbiol. 8:2707. doi: 10.3389/fmicb.2017.02707
Received: 27 September 2017; Accepted: 29 December 2017;
Published: 31 January 2018.
Edited by:
Andrea Gomez-Zavaglia, Centro de Investigación y Desarrollo en Criotecnología de Alimentos (CIDCA), ArgentinaReviewed by:
Graciela L. Lorca, University of Florida, United StatesGiuseppe Spano, University of Foggia, Italy
Copyright © 2018 Allain, Chaouch, Thomas, Vallée, Buret, Langella, Grellier, Polack, Bermúdez-Humarán and Florent. This is an open-access article distributed under the terms of the Creative Commons Attribution License (CC BY). The use, distribution or reproduction in other forums is permitted, provided the original author(s) and the copyright owner are credited and that the original publication in this journal is cited, in accordance with accepted academic practice. No use, distribution or reproduction is permitted which does not comply with these terms.
*Correspondence: Luis G. Bermúdez-Humarán, bHVpcy5iZXJtdWRlekBpbnJhLmZy
Isabelle Florent, aXNhYmVsbGUuZmxvcmVudEBtbmhuLmZy
†These authors have contributed equally to this work.