- 1Oxford University Clinical Research Unit, Ho Chi Minh City, Vietnam
- 2Centre for Tropical Medicine and Global Health, Nuffield Department of Medicine, University of Oxford, Oxford, United Kingdom
- 3Sir William Dunn School of Pathology, University of Oxford, Oxford, United Kingdom
- 4Hospital for Tropical Diseases, Ho Chi Minh City, Vietnam
Introduction: Mycobacteria have several unique cellular characteristics, such as multiple cell envelope layers, elongation at cell poles, asymmetric cell division, and accumulation of intracytoplasmic lipid inclusions, which contributes to their survival under stress conditions. However, the understanding of these characteristics in clinical Mycobacterium tuberculosis (M. tuberculosis) isolates and under host stress is limited. We previously reported the influence of host stress on the cell length distribution in a large set of clinical M. tuberculosis isolates (n = 158). Here, we investigate the influence of host stress on the cellular ultrastructure of few clinical M. tuberculosis isolates (n = 8) from that study. The purpose of this study is to further understand the influence of host stress on the cellular adaptations of clinical M. tuberculosis isolates.
Methods: We selected few M. tuberculosis isolates (n = 8) for analyzing the cellular ultrastructure ex vivo in sputum and under in vitro stress conditions by transmission electron microscopy. The cellular adaptations of M. tuberculosis in sputum were correlated with the ultrastructure of antibiotic sensitive and resistant isolates in liquid culture, under oxidative stress, iron deficiency, and exposure to isoniazid.
Results: In sputum, M. tuberculosis accumulated intracytoplasmic lipid inclusions. In liquid culture, clinical M. tuberculosis revealed isolate to isolate variation in the extent of intracytoplasmic lipid inclusions, which were absent in the laboratory strain H37Rv. Oxidative stress, iron deficiency, and exposure to isoniazid increased the accumulation of lipid inclusions and decreased the thickness of the cell envelope electron transparent layer in M. tuberculosis cells. Furthermore, intracytoplasmic compartments were observed in iron deficient cells.
Conclusion: Our ultrastructural analysis has revealed significant influence of host stress on the cellular adaptations in clinical M. tuberculosis isolates. These adaptations may contribute to the survival of M. tuberculosis under host and antibiotic stress conditions. Variation in the cellular adaptations among clinical M. tuberculosis isolates may correlate with their ability to persist in tuberculosis patients during antibiotic treatment. These observations indicate the need for further analyzing these cellular adaptations in a large set of clinical M. tuberculosis isolates. This will help to determine the significance of these cellular adaptations in the tuberculosis treatment.
Introduction
Mycobacterium tuberculosis (M. tuberculosis), causes tuberculosis (TB) and is a major public health problem (World Health Organization [WHO], 2015). The ability of M. tuberculosis cells to survive under host and antibiotic stress partly explains why M. tuberculosis is a successful human pathogen. Hence, cellular adaptations conferring stress tolerance in M. tuberculosis and in related species are an active area of research (Kieser and Rubin, 2014).
Investigations into cell biology of mycobacteria have revealed several unique characteristics in growth and division, which contributes to their survival under stress conditions (Thanky et al., 2007; Hett and Rubin, 2008; Kieser and Rubin, 2014). One such cellular structure is the complex cell envelope of mycobacteria (Brennan and Nikaido, 1995). Electron microscopy has revealed the ultrastructure of cell envelope layers in mycobacteria (Takade et al., 1983; Hoffmann et al., 2008; Zuber et al., 2008; Vijay et al., 2012). The cell envelope is essential for M. tuberculosis survival as it acts as a permeability barrier for the entry of antibiotics and also modulates host immune response (Jarlier and Nikaido, 1994; Briken et al., 2004; Torrelles and Schlesinger, 2010). Therefore, it is also an important drug and vaccine target (Chatterjee, 1997; Abrahams and Besra, 2016; Tima et al., 2017). The composition of cell envelope layers has been determined using cell envelope mutants (Etienne et al., 2002, 2005) and antibiotic treatments which inhibit the envelope synthesis in mycobacteria (Mdluli et al., 1998). These studies have advanced our understanding of the cell envelope role as a permeability barrier and in inhibiting phagocytosis of mycobacteria by macrophages (Mdluli et al., 1998; Etienne et al., 2002, 2005).
Another feature revealed by electron microscopy was the accumulation of intracytoplasmic lipid inclusions in mycobacteria under different host infection model systems (Peyron et al., 2008; Caire-Brandli et al., 2014; Barisch and Soldati, 2017a). In an in vitro human granuloma model of infection, M. tuberculosis cells accumulated lipid inclusions during infection of lipid loaded macrophages called foam cells (Peyron et al., 2008). Similarly, M. avium accumulated host-derived lipids as inclusions in foam cells and exhibited a thin cell envelope (Caire-Brandli et al., 2014). Recently, M. marinum was also found to have lipid inclusions derived from host lipids during the infection of Dictyostelium (Barisch and Soldati, 2017a). These studies have identified triacylglycerols as the major lipid in mycobacterial lipid inclusions derived from host cells (Peyron et al., 2008; Daniel et al., 2011; Caire-Brandli et al., 2014; Barisch and Soldati, 2017a). M. tuberculosis and M. smegmatis can also accumulate lipid inclusions containing triacylglycerols under in vitro stress conditions independent of host cells (Garton et al., 2002; Anuchin et al., 2009; Deb et al., 2009). Several studies have shown that M. tuberculosis uses diverse host carbon sources such as cholesterol, pyruvate, and glucose (Pandey and Sassetti, 2008; Marrero et al., 2013; Baker et al., 2014). Utilization of such diverse carbon sources by M. tuberculosis contributes to its pathogenesis and persistence in the host (Pandey and Sassetti, 2008; Marrero et al., 2013; Baker et al., 2014).
Importantly, the accumulation of lipid inclusions in M. tuberculosis was associated with persistence, antibiotic tolerance, cavitation, and poor treatment outcome (Deb et al., 2009; Russell et al., 2009; Daniel et al., 2011; Hammond et al., 2015; Kayigire et al., 2015; Sloan et al., 2015). It is possible that this is due to growth arrest of M. tuberculosis and loss of antimicrobial functions by foamy macrophages leading to persistent infection (Peyron et al., 2008; Daniel et al., 2011; Caire-Brandli et al., 2014). This phenomenon may lead to clinical complications, such as relapse of infection and the emergence of antibiotic-resistant M. tuberculosis (Cohen et al., 2013; Sebastian et al., 2017). Thus, intracytoplasmic lipid inclusions and the cell envelope are important for the survival of M. tuberculosis. The understanding of these cellular characteristics and their adaptations to stress in clinical M. tuberculosis isolates is limited. This understanding is vital for the development of novel therapeutic targets. In our previous study, we have observed that host stresses influenced cell length distribution in a large set (n = 158) of clinical M. tuberculosis isolates (Vijay et al., 2017). In this study we investigated the accumulation of lipid inclusions and cell envelope ultrastructure of M. tuberculosis in sputum by transmission electron microscopy (TEM). The ultrastructure of M. tuberculosis in sputum was compared with the ultrastructure of clinical M. tuberculosis isolates and H37Rv in liquid culture, and under conditions of oxidative stress, iron deficiency, and exposure to the antibiotic isoniazid.
Materials and Methods
Bacterial Isolates
Six M. tuberculosis clinical isolates were selected from a collection of M. tuberculosis clinical isolates from pre-treated patients with pulmonary tuberculosis (n = 158) in Vietnam, along with the laboratory strain H37Rv. We selected three sensitive and three antibiotic-resistant isolates as determined by drug susceptibility test for the electron microscopy analysis. Table 1 presents drug sensitivity data.
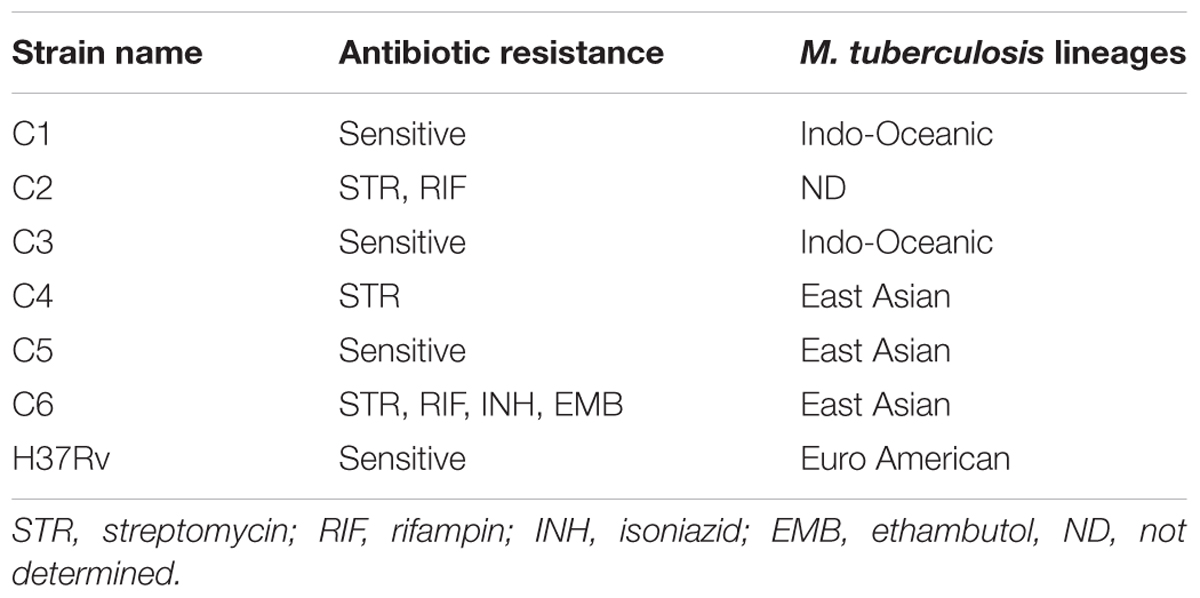
TABLE 1. Mycobacterium tuberculosis clinical strains selected for the study based on antibiotic sensitive and resistant phenotypes.
Ethics Approval Statement
Between January 2015 and October 2016, patients were recruited from two district TB control units in Ho Chi Minh City (HCMC), Vietnam. The clinical M. tuberculosis isolates were collected from patients before treatment. The patients were ≥18 years of age, had clinical symptoms of active pulmonary TB, which was confirmed by chest X-ray and positive sputum culture, and none of the patients were HIV positive. Written informed consent was obtained from each patient in accordance with the declaration of Helsinki. Protocols were approved by the human subjects review committees, at the Hospital for Tropical Diseases HCMC, Vietnam (124/BVBNƉ.HƉƉƉ) and the Oxford Tropical Research Ethics Committee, United Kingdom (OxTREC Reference: 16-14).
Bacterial Culture
Mycobacterium tuberculosis isolates were cultured from sputum samples in bio safety level-3 laboratory and were stored as glycerol stocks in 7H9 media. These M. tuberculosis isolates were used for the experiments with a limited number of sub-culturing (approximately two to three passages) to avoid phenotypic/genotypic changes in clinical M. tuberculosis isolates. For mid-log culture, 50 ml culture tubes with 10 ml of 7H9T medium [7H9 broth supplemented with 10% oleic acid/albumin/dextrose/catalase (OADC) enrichment, and 0.05% Tween 80, BD DifcoTM] were inoculated with the clinical isolates and laboratory strain H37Rv, incubated at 37°C without shaking. The samples were processed for TEM at O.D600 of 0.3–0.6.
Drug Susceptibility Test
Drug susceptibility was performed using BACTECTM MGITTM 960 SIRE Kit (BD), according to manufacturer guidelines. Drug susceptibility was tested for streptomycin (1.0 μg/ml), isoniazid (0.1 μg/ml), rifampicin (1.0 μg/ml), and ethambutol (5.0 μg/ml).
M. tuberculosis Lineage Identification
The lineages of the selected clinical M. tuberculosis isolates were determined in the previous study (Vijay et al., 2017).
Oxidative Stress, Iron Deficiency, and Isoniazid Treatment
For TEM analysis of M. tuberculosis cells under different stress conditions, M. tuberculosis culture in 7H9T medium at O.D600 0.3–0.5 was treated with H2O2 (Merk) at different concentrations, ranging from 21 to 210 mM for 48 h at 37°C and selected 21 mM H2O2-treated samples for electron microscopy (Voskuil et al., 2011). For iron deficiency, M. tuberculosis isolates were cultured in the presence of deferoxamine mesylate salt (DFO) (Sigma–Aldrich) at final concentrations of 100, 250, and 500 μM in 7H9T medium until the O.D600 reached 0.3–0.5, with the 100 and 500 μM DFO-treated samples processed for electron microscopy (Pal et al., 2015). For isoniazid treatment, M. tuberculosis isolates were grown in the presence of isoniazid (Sigma–Aldrich) in 7H9T medium at a concentration of 0.015 μg/ml until the O.D600 reached 0.3–0.5. All treated and untreated control isolates, along with about 500 μl of sputum with high density of acid fast bacilli (3+) as observed by microscopy from two pulmonary tuberculosis patients, were then processed for TEM.
Transmission Electron Microscopy
Mycobacterium tuberculosis cells were fixed as described previously (Vijay et al., 2012). M. tuberculosis cells were harvested by centrifugation and fixed in 1% (vol/vol) osmium tetroxide (Sigma–Aldrich) and 0.15 M sodium cacodylate buffer (pH 7.2) (Sigma–Aldrich) for 1 h at room temperature. After this samples were washed once with the same buffer, and post fixed for 2 h at room temperature in 0.15 M cacodylate buffer (pH 7.2) containing 2% (wt/vol) tannic acid and 2% (vol/vol) glutaraldehyde (both from Sigma–Aldrich). Samples were then washed once with 0.15 M cacodylate buffer and then refixed in 1% (vol/vol) osmium tetroxide overnight at 4°C and stored at 4°C for 2–4 weeks before further processing. Next the samples were washed with water and cells were re-suspended in 4% low melting point agarose, spun down, and stored at 4°C for few minutes. These samples were cut into small fragments of less than 1 mm3 and stained with 0.5% uranyl acetate overnight and washed with water. Subsequent steps were performed using a Leica EM TP automated processing unit (Leica Microsystems). Samples were dehydrated in a graded series of ice cold ethanol (Merck) and then infiltrated with epoxy resin (Taab Low Viscosity Resin, Taab Laboratories) as follows: 25% resin in ethanol for 2 h, 50% resin for 3 h, 75% resin for 2 h, then 100% resin over 48 h with several changes of resin. Samples were polymerized in beem capsules at 60°C for 48 h. Ultrathin sections (90 nm) were obtained using a Leica UC7 Ultramicrotome and a Diatome Diamond Knife (Leica microsystems and Diatome). Sections were transferred to formvar coated 100 mesh Cu grids and post-stained with Reynolds’ lead citrate (Reynolds, 1963). Sections were imaged on an FEI Tecnai 12 Transmission Electron Microscope operated at 120 kV using a Gatan OneView digital camera. In each condition approximately 100 M. tuberculosis cells per sample were observed, except sample S2 (n = 10 cells). Cell envelope layer measurements were carried out using ImageJ (Schneider et al., 2012).
Results
M. tuberculosis in Sputum Displayed Triple Layered Cell Envelope and Accumulation of Intracytoplasmic Lipid Inclusions
Initially, we investigated M. tuberculosis cell envelope ultrastructure and lipid inclusions in pulmonary tuberculosis patient’s sputum samples. The ultrastructure of these cells displayed a triple layered cell envelope which could be clearly distinguished as consisting of an electron dense outer layer (OL), electron transparent layer (ETL), and peptidoglycan layer (PGL) (Figure 1). M. tuberculosis cells in sputum were identified by the characteristic triple layered cell envelope of mycobacteria and distinguished from other bacteria present in the sputum (Figures 1A–C). M. tuberculosis cells revealed the accumulation of intracytoplasmic lipid inclusions in sputum sample S1 (Figure 1A and Table 2). The ETL of the cell envelope had an average thickness of 10.7 nm (±9 nm) in one of the patient sputum sample (S1) and 40 nm (±38 nm) in M. tuberculosis cells from another patient sputum sample (S2, Figure 1B). This revealed that M. tuberculosis cells in human hosts accumulate lipid inclusions and that envelope ultrastructure varies between hosts.
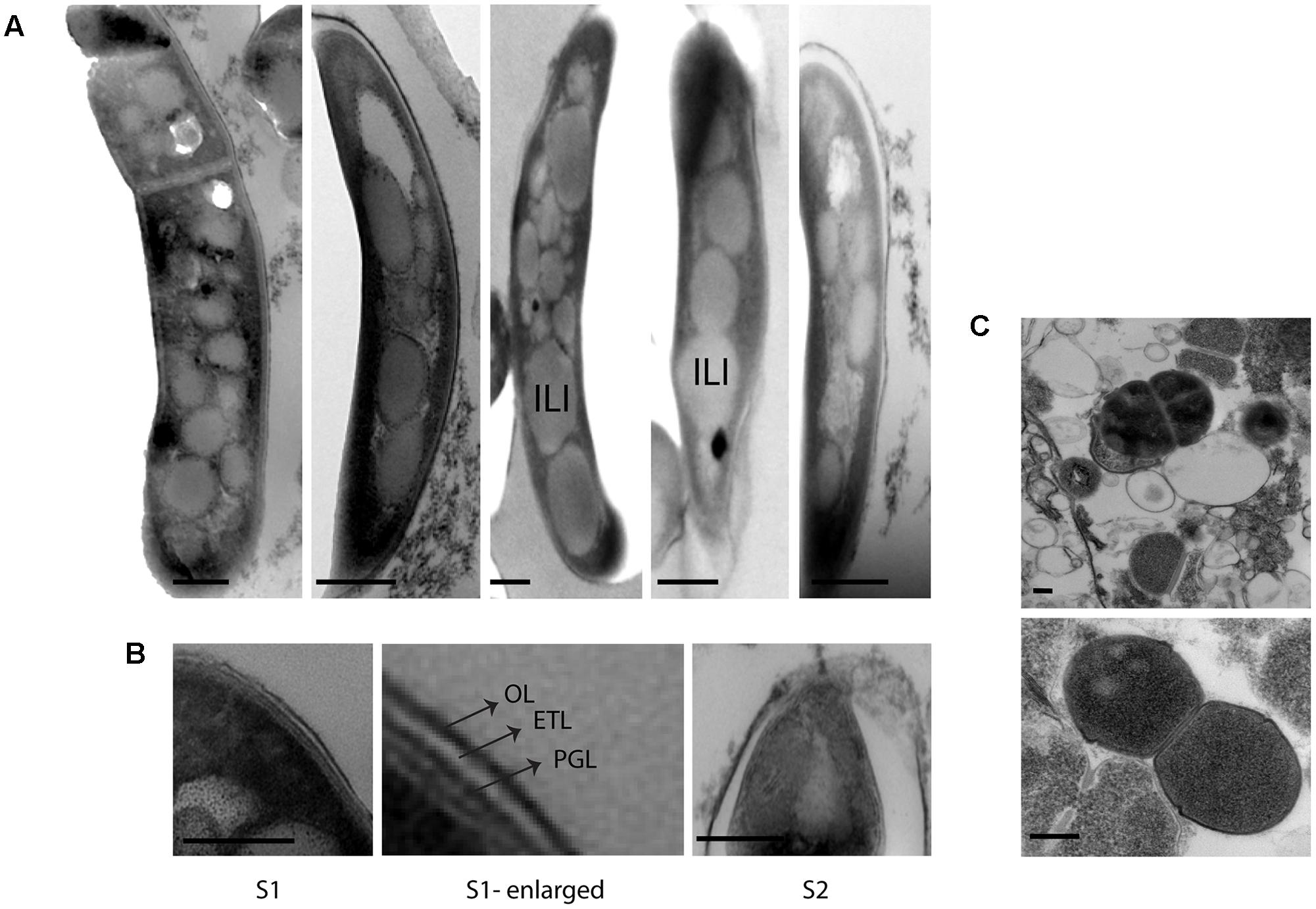
FIGURE 1. Cellular ultrastructure of M. tuberculosis cells from patient sputum samples. (A) TEM images of M. tuberculosis cells with intracytoplasmic lipid inclusions in sputum sample S1. (B) TEM images of M. tuberculosis cells with detailed ultrastructure of cell envelope layers OL, ETL, and PGL from two different sputum samples S1 and S2. (C) TEM images of other bacterial cells in sputum sample S1. OL, outer layer; ETL, electron transparent layer; PGL, peptidoglycan layer; ILI, intracytoplasmic lipid inclusions; TEM, transmission electron microscopy. Scale bar = 200 nm.
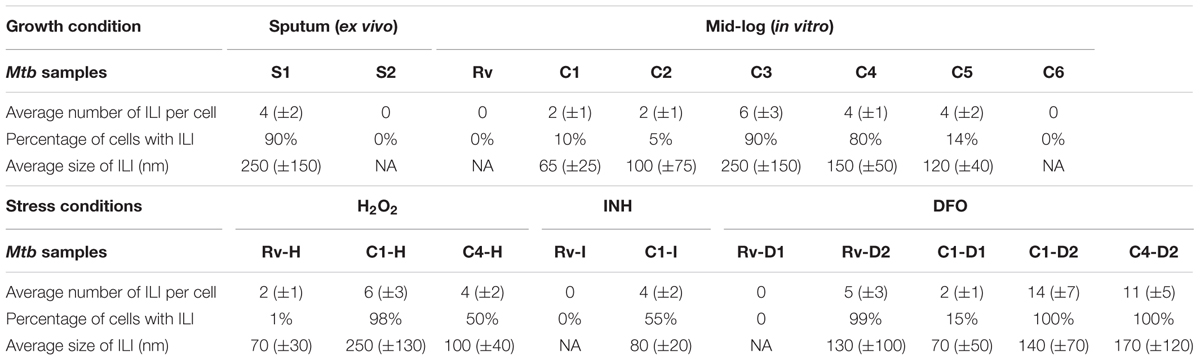
TABLE 2. Quantification of intracytoplasmic lipid inclusions (ILI) in M. tuberculosis isolates from the study (n ∼ 100 cells in each isolate/condition, except S2, n = 10 cells).
Strain-to-Strain Variation in Accumulation of Intracytoplasmic Lipid Inclusions among Clinical M. tuberculosis Isolates in Mid-Log Culture Condition
We analyzed the cellular ultrastructure of six clinical M. tuberculosis isolates (C1–C6) along with H37Rv under mid-log culture condition (Figure 2A). Major cellular ultrastructural features of M. tuberculosis isolates include the triple layered cell envelope, nucleoid, and cytoplasm. These features were similar in both sensitive (C1, C3, and C5) and resistant (C2, C4, and C6) M. tuberculosis isolates (Figures 2A,B). We also observed mild (Figure 2A and Table 2, C1, C2, C4, and C5) to extensive (Figure 2A and Table 2, C3) accumulation of cytoplasmic lipid inclusions in clinical M. tuberculosis isolates, but not in H37Rv and C6 (Figure 2A and Table 2). All M. tuberculosis isolates in mid-log condition had an ETL of average thickness 31.7 nm (±13.1 nm) (Supplementary Figure S1A). We also observed high variation in ETL thickness in the same cell and between different M. tuberculosis cells (Supplementary Figure S1A). Based on these ex vivo and in vitro ultrastructure of clinical M. tuberculosis isolates we further analyzed the cellular adaptations under different stress conditions.
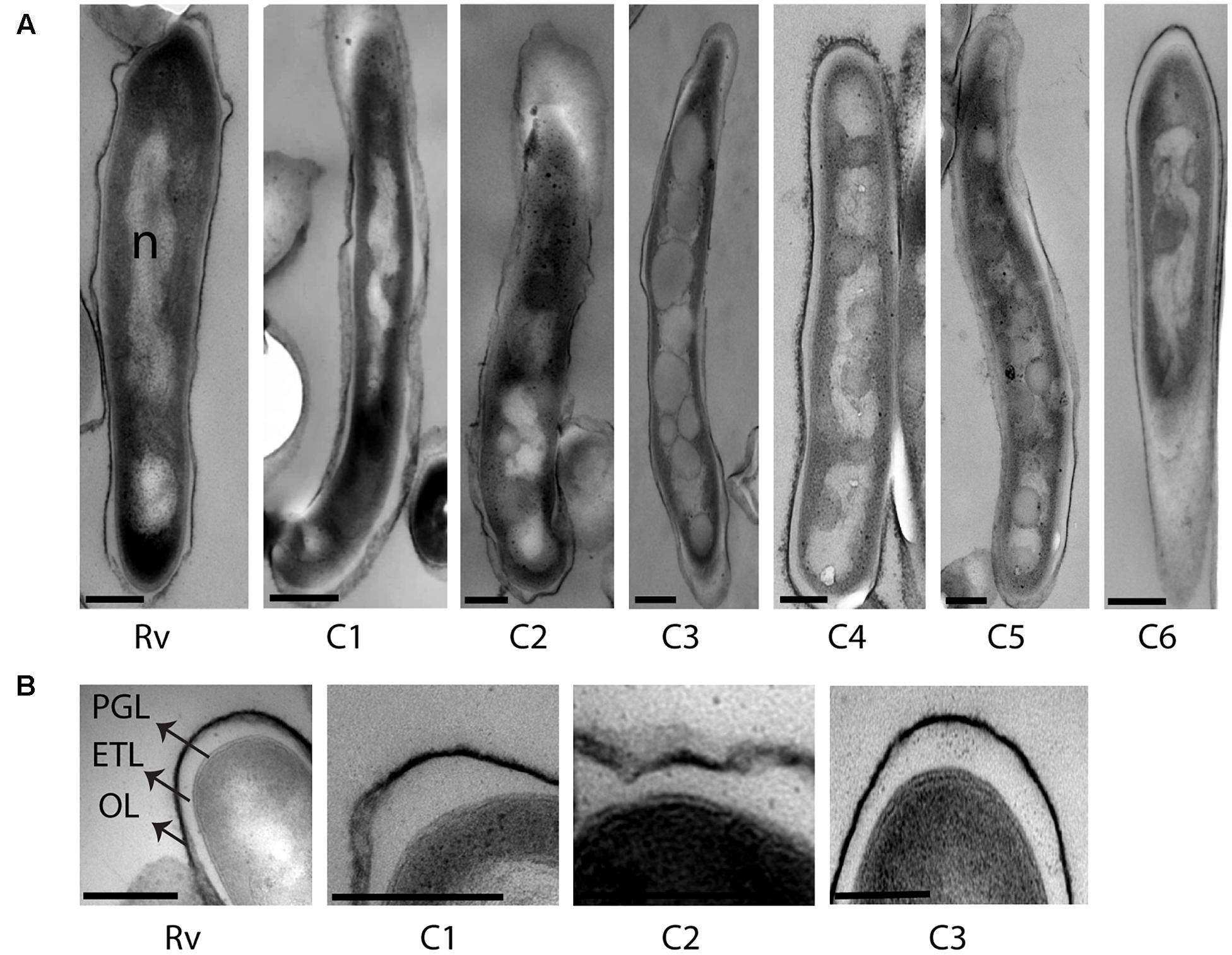
FIGURE 2. Cellular ultrastructure of clinical M. tuberculosis strains under mid-log culture condition. (A) TEM images of H37Rv (Rv) and six clinical M. tuberculosis strains from mid-log culture (C1–C6). (B) TEM images of H37Rv and three clinical M. tuberculosis strains (C1–C3) with detailed ultrastructure of cell envelope layers in mid-log culture. OL, outer layer; ETL, electron transparent layer; PGL, peptidoglycan layer; ILI, intracytoplasmic lipid inclusions; n, nucleoid. Scale bar = 200 nm.
Accumulation of Intracytoplasmic Lipid Inclusions Increased in Oxidative, Iron Deficiency, and Antibiotic Stresses
We observed M. tuberculosis cells with reduced acid fast staining and beaded appearance in sputum, oxidative stress, iron deficiency, and isoniazid treatment (Figure 3, n ∼ 100–300 cells), and then we characterized the ultrastructure of M. tuberculosis under these conditions (Figure 4). H2O2 and isoniazid treatment resulted in a significant accumulation of intracytoplasmic lipid inclusions in clinical M. tuberculosis isolate C1 (Figures 4A,B and Table 2), but not in H37Rv and C4 (Figures 4A,B and Table 2). H37Rv and clinical M. tuberculosis isolates exposed to 100 μM DFO did not accumulate lipid inclusions (Figure 4C and Table 2) while all isolates treated with 500 μM DFO exhibited accumulation of lipid inclusions (Figure 4C and Table 2). Both H2O2 and DFO treatments also resulted in a thinner ETL, with thickness of 13 (±11 nm) and 10.5 nm (± 4 nm), respectively, in M. tuberculosis cell envelope as compared to untreated mid-log control (Figure 4D compared to Figure 2B, P < 0.0001 Mann–Whitney U-test; Supplementary Figures S1B,C). Similar to the observations in M. tuberculosis cells from sputum, different host and antibiotic stresses increased the accumulation of intracytoplasmic lipid inclusions and reduced the cell envelope ETL in M. tuberculosis isolates.
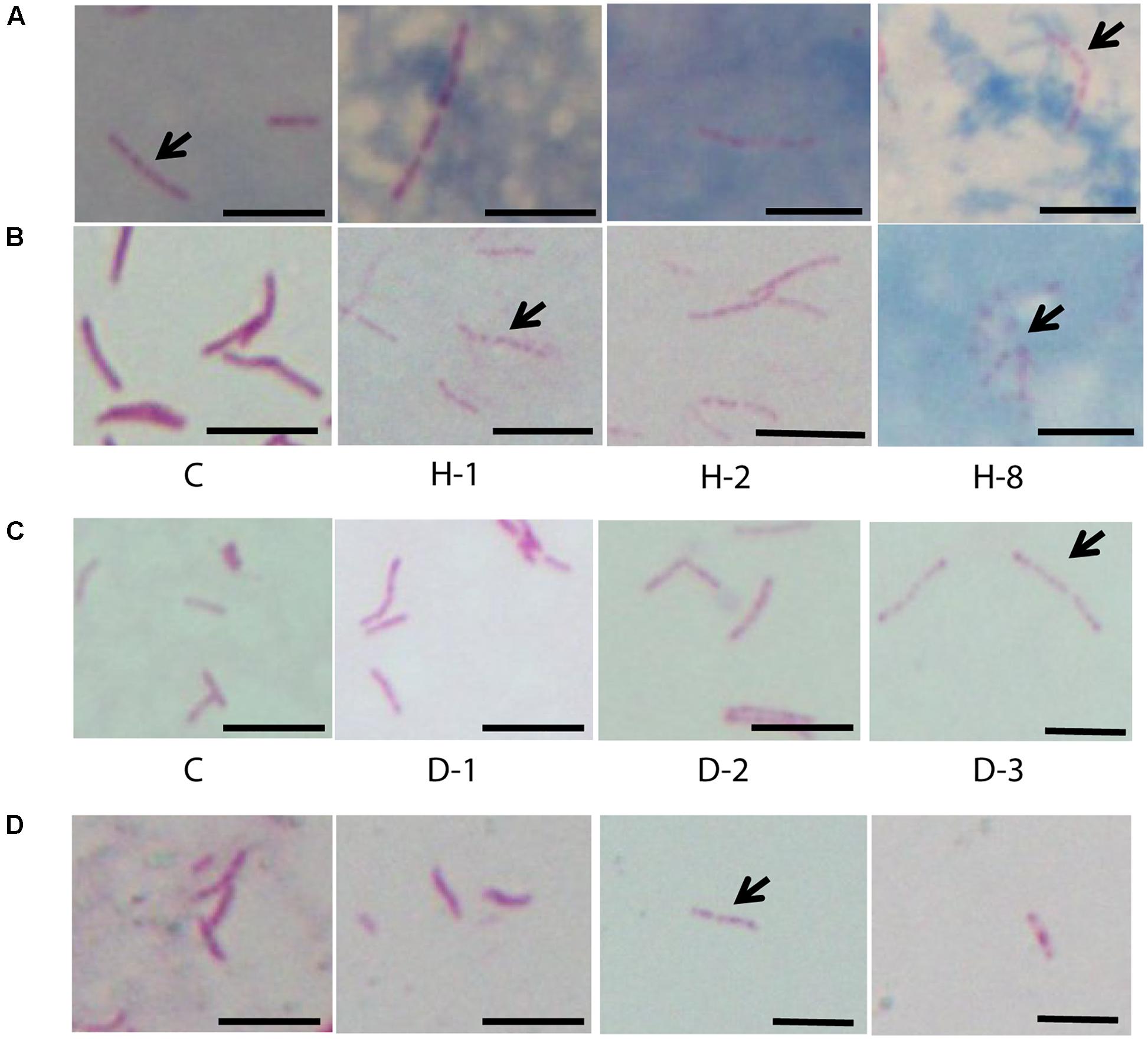
FIGURE 3. Acid fast staining of M. tuberculosis cells in sputum, oxidative stress, iron deficiency, and isoniazid treatment. (A) Four sputum samples with M. tuberculosis cells (n ∼ 100 cells). (B) Clinical M. tuberculosis strain treated with different concentrations of H2O2 for oxidative stress and (C) DFO for iron deficiency (n = 300 cells). (D) Clinical M. tuberculosis strains grown in the presence of isoniazid (0.015 μg/ml) (n ∼ 100 cells). C, untreated control; H2O2 concentrations used are 21 (H-1), 42 (H-2), and 168 mM (H-8) and the concentrations of DFO are 100 (D-1), 250 (D-2), and 500 μM (D-3), arrow indicates beaded cells and scale bar = 5 μm.
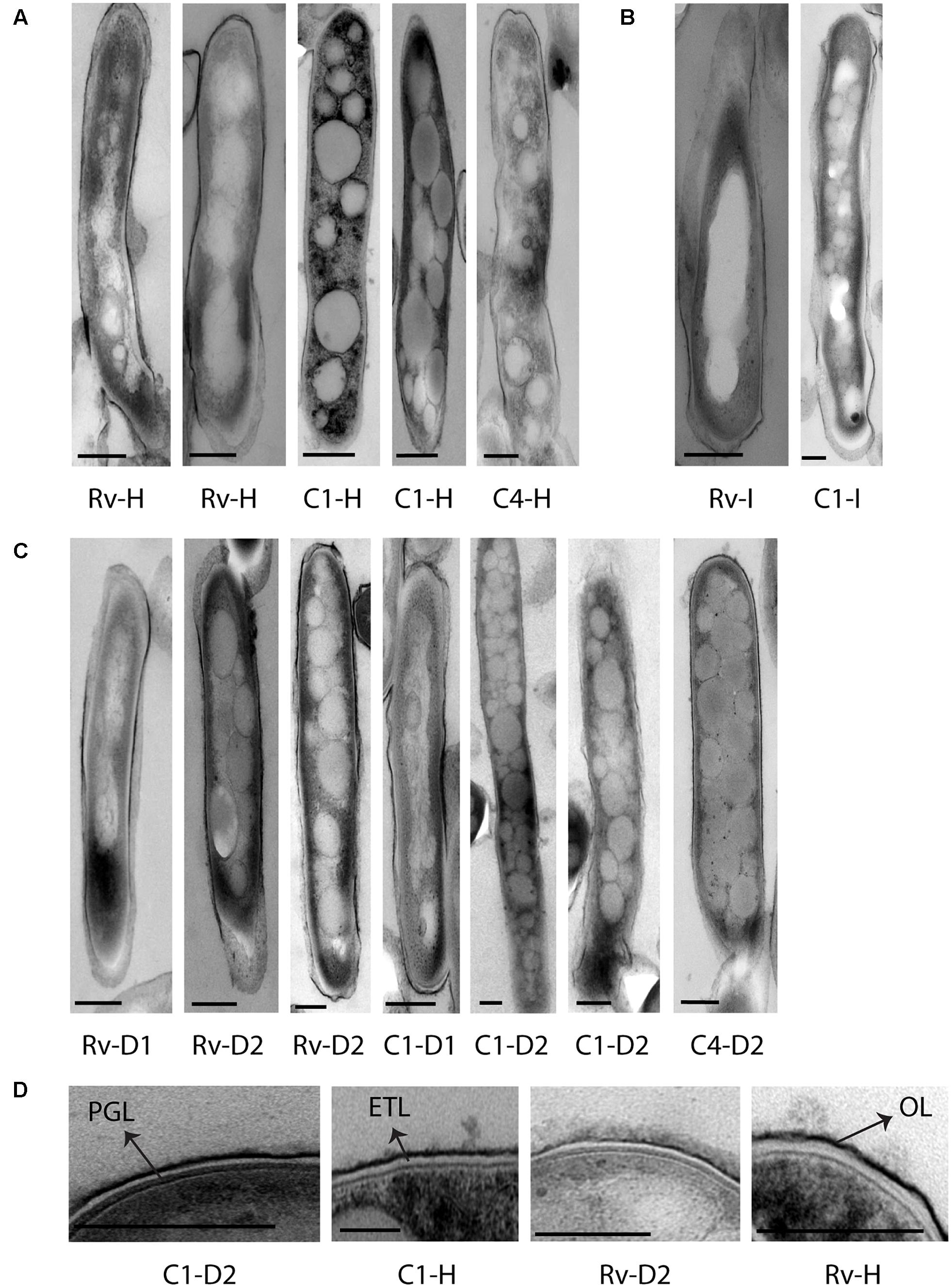
FIGURE 4. Cellular ultrastructure of M. tuberculosis strains under H2O2, isoniazid treatment, and iron deficiency displaying accumulation of lipid inclusions and thin cell envelope ETL. (A) TEM images of H37Rv [Rv-H (two images)] and clinical M. tuberculosis strains [C1-H (two images) and C4-H] under H2O2 treatment. (B) TEM image of H37Rv and clinical M. tuberculosis strain grown under isoniazid treatment (Rv-I and C1-I). (C,D) TEM images of H37Rv and clinical M. tuberculosis strains grown under iron deficiency DFO-100 (Rv-D1, C1-D1) and 500 μM [Rv-D2 (two images), C1-D2 (two images) and C4-D2]. DFO, deferoxamine mesylate salt; OL, outer layer; ETL, electron transparent layer; PGL, peptidoglycan layer; scale bar = 200 nm.
Unique Intracytoplasmic Compartment Observed in M. tuberculosis Cells under Iron Deficiency
In addition to the cellular adaptations observed above in different stress conditions, we also observed unique intracytoplasmic compartments in iron-deficient M. tuberculosis cells. This compartment was only observed in M. tuberculosis grown in the presence of 500 μM DFO and not in cells grown in 100 μM DFO and or the mid-log controls (Figure 5). Single intracytoplasmic compartments were observed in all three strains used in this experiment, H37Rv and clinical M. tuberculosis isolates (C1, C4), under iron deficiency (n = 50 cells observed in each strain) (Figure 5A). The average size of this compartment was 250 nm (±50 nm, n = 30 cells in total) (Figure 5B). At high magnification, we also observed membrane-like structure surrounding these intracytoplasmic compartments, some of which contained small circular units of diameter 17.4 nm (±3.6 nm) (Figure 5C).
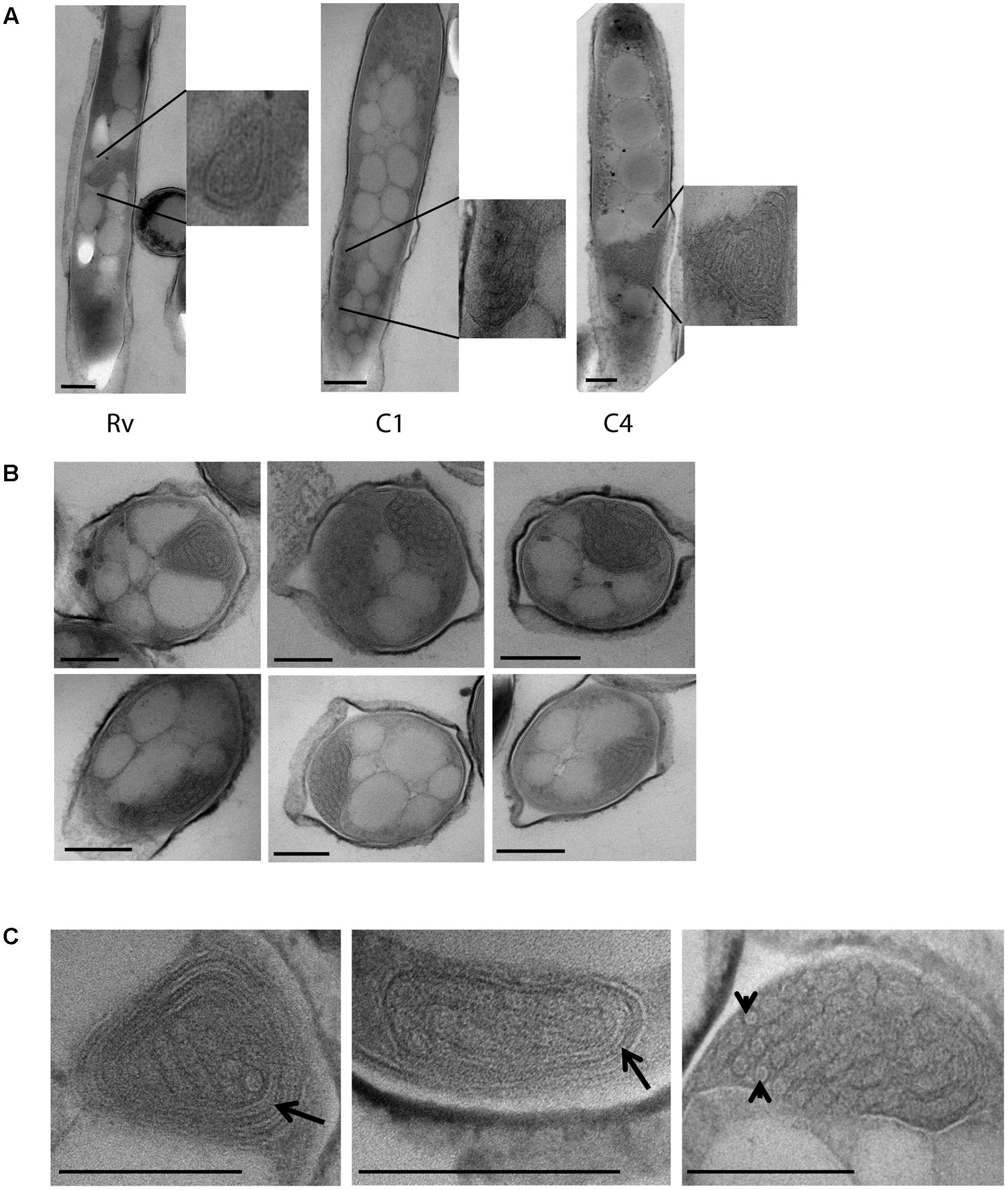
FIGURE 5. Cytoplasmic compartments in M. tuberculosis strains under iron deficiency. (A) H37Rv (Rv) and clinical M. tuberculosis strains (C1, C4) grown under 500 μm DFO, displaying cytoplasmic compartments in cells along with enlarged images. (B) Multiple sections of M. tuberculosis cells grown under 500 μm DFO, with cytoplasmic compartment as indicated by asterisks. (C) Higher magnification images (40–50 K X) of cytoplasmic compartments from M. tuberculosis cells grown under 500 μm DFO, arrow indicates membrane-like structures surrounding the cytoplasmic compartment and arrowhead indicates subunits assembled inside the compartment. DFO, deferoxamine mesylate salt; scale bar = 200 nm.
Discussion
We analyzed the lipid inclusions and cell envelope layers in clinical M. tuberculosis isolates ex vivo in sputum representing the host environment. We then compared this with the ultrastructure of clinical M. tuberculosis isolates and H37Rv in liquid culture and under different in vitro stress conditions. This revealed the accumulation of intracytoplasmic lipid inclusions in clinical M. tuberculosis isolates as a cellular adaptation in sputum, liquid culture, and under stress conditions. Analysis of six clinical M. tuberculosis isolates revealed isolate-to-isolate variation in the extent of lipid inclusions in mid-log culture and its increased accumulation under stress conditions. The thickness of M. tuberculosis cell envelope ETL was significantly reduced under different stress conditions. Formation of an intracytoplasmic compartment in M. tuberculosis cells was also observed under iron deficiency.
Mycobacterium tuberculosis cells with lipid inclusions have been associated with foamy macrophages and unfavorable treatment outcome in tuberculosis patients (Garton et al., 2002; Peyron et al., 2008; Kayigire et al., 2015; Sloan et al., 2015). In the present study, clinical M. tuberculosis isolates displayed lipid inclusions even in liquid culture, which was not observed in the laboratory strain H37Rv. Similarly, M. avium and M. marinum also do not accumulate lipid inclusions in macrophages and the extracellular environment, respectively (Caire-Brandli et al., 2014; Barisch and Soldati, 2017a). This indicates that accumulation of lipid inclusions is a more prominent cellular adaptation in clinical M. tuberculosis isolates compared to laboratory strains of mycobacteria. Supporting this, we also observed increased accumulation of lipid inclusions under both oxidative stress and sub-inhibitory concentration of isoniazid only in clinical M. tuberculosis isolates. Isoniazid can also induce oxidative stress and may therefore link these findings (Timmins and Deretic, 2006). It will be interesting to study how other antibiotic treatments influences the accumulation of lipid inclusions in clinical M. tuberculosis isolates, as its accumulation may have a role in M. tuberculosis persistence to antibiotics (Hammond et al., 2015; Kayigire et al., 2015; Sloan et al., 2015).
We observed increased accumulation of lipid inclusions in M. tuberculosis cells at 500 μM DFO compared to 100 μM DFO-treated cells under iron deficiency. DFO concentration-dependent accumulation of lipid inclusions were found in both clinical M. tuberculosis isolates and H37Rv. Supporting these observations it has also been reported that iron deficiency and oxidative stress can induce lipid accumulation in mycobacteria, which depends on host foamy macrophages (Bacon et al., 2007; Peyron et al., 2008). Host oxidative stress generates oxidized low-density lipoproteins, and oxygenated mycolic acids present in M. tuberculosis; both can trigger the differentiation of host macrophages into foamy cells (Peyron et al., 2008; Palanisamy et al., 2012). This in turn facilitates the accumulation of lipid inclusions in M. tuberculosis cells and provides a protective niche for its survival. Our host stress models were based on in vitro culture lacking foamy macrophages. Hence, accumulation of lipid inclusions in our host stress models in M. tuberculosis cells may have derived lipids from oleic acids present in the culture media, as seen in case of M. smegmatis (Garton et al., 2002; Anuchin et al., 2009).
Oxidative stress was also a co-factor in all of the stress conditions where we observed the increased accumulation of lipid inclusions in M. tuberculosis cells (Rodriguez and Smith, 2003; Timmins and Deretic, 2006). Transcriptional adaptation of M. tuberculosis in macrophages and under in vitro stress conditions strongly correlates with the ultrastructural adaptations observed here, indicating that under host stress M. tuberculosis shifts to a fatty acid-based metabolism (Schnappinger et al., 2003). Enzymes involved in fatty acid metabolism are also essential for in vivo growth and virulence (Munoz-Elias and McKinney, 2005; Reed et al., 2007). The accumulation of lipid inclusions is implicated in M. tuberculosis cell division arrest and induction of antibiotic tolerant dormant phenotype (Daniel et al., 2011; Caire-Brandli et al., 2014). This needs to be reinvestigated as our study shows that lipid inclusions per se may not inhibit cell division in M. tuberculosis. We observed M. tuberculosis cells with lipid inclusions growing in mid-log culture and under iron deficiency, similar to the growth observed in M. marinum with lipid inclusions (Barisch and Soldati, 2017a). It is possible that accumulation of lipids being a cellular adaptation that can facilitate M. tuberculosis entry into, and survival during dormancy (Barisch and Soldati, 2017b).
The unique triple layered cell envelope, reported in several laboratory mycobacterial strains and in clinical strains of M. tuberculosis (Takade et al., 1983; Brennan and Nikaido, 1995; Velayati et al., 2009; Vijay et al., 2012), was also observed in all of the clinical M. tuberculosis isolates in the present study. The ultrastructure of triple layered cell envelope from our study was also similar to the cell envelope ultrastructure of M. tuberculosis processed by cryofixation and rapid freeze substitution (Yamada et al., 2010, 2015). We also observed tearing of resin around M. tuberculosis cells in sputum, as observed in TEM images of M. marinum granulomas and M. tuberculosis cells (Bouley et al., 2001; Vijay et al., 2014). The thickness of the triple layers under mid-log growth conditions was consistent across the six clinical M. tuberculosis isolates and H37Rv used here. However, under stress conditions like sputum, oxidative stress, and iron deficiency, we observed a significant reduction in the thickness of cell envelope ETL, although the extent of this reduction varied between the two sputum samples despite a similar bacterial load. These findings suggest that the ETL can be reduced in thickness under host stress, which may vary from patient to patient. This needs to be investigated in a greater number of patients and correlated with aspects such as severity of tuberculosis symptoms and persistence to understand the clinical significance of such adaptations.
The ETL is mainly composed of lipids like mycolic acids (Mdluli et al., 1998; Wang et al., 2000) and transcriptional analysis of M. tuberculosis cells under host stress also indicate cell envelope remodeling and fatty acid degradation (Schnappinger et al., 2003). Cell envelope lipids are also involved in host immune modulation and virulence of M. tuberculosis strains (Karakousis et al., 2004; Makinoshima and Glickman, 2005). It has also been observed that under different stress conditions M. tuberculosis loses acid fastness due to loss of cell envelope lipids and it is associated with dormancy and antibiotic tolerance (Bhatt et al., 2007; Deb et al., 2009). We also observed reduced acid fast staining and M. tuberculosis cells with acid fast stained cytoplasmic beads in oxidative stress and iron deficiency. Such cells were also observed in some sputum samples and under isoniazid treatment, these observations strongly correlate with the ultrastructural adaptations such as reduced ETL and accumulation of lipid inclusions in our study. Further investigations are needed to understand the role of reduced cell envelope lipids on the accumulation of intracytoplasmic lipid inclusions in M. tuberculosis.
Reduction in the envelope lipids may enhance the permeability of cell envelope and influence the susceptibility of M. tuberculosis to antibiotics. Cell envelope modifications and enhanced antibiotic susceptibility in M. smegmatis have been observed under iron deficiency (Pal et al., 2015). Triacylglycerol is also a component of M. tuberculosis cell envelope and loss of acid fastness is observed under iron deficiency and in hypoxia (Rastogi et al., 2017). Such cell envelope modifications accompany non-replicative persistence and antibiotic tolerance of M. tuberculosis in vitro (Rastogi et al., 2017). These observations indicate the influence of host factors on cellular adaptations in M. tuberculosis and antibiotic susceptibility. As there are multiple host factors and complex interactions influencing antibiotic susceptibility, this needs to be investigated further to identify the factors that can enhance susceptibility to antibiotics. There was significant variation in the accumulation of lipid inclusions in clinical M. tuberculosis isolates in mid-log culture. Such differences between M. tuberculosis isolates may have a clinical significance in persistence against host stress and antibiotics. Hence, variations in cellular adaptations need to be correlated with persistence and antibiotic tolerance among clinical M. tuberculosis isolates to understand its role in treatment failure.
Beijing lineage was shown to accumulate triacylglycerides and has triacylglyceride synthase gene (Rv 3130c) upregulated during in vitro growth (Reed et al., 2007). This gene is a member of DosR regulon, and some of the regulon genes are constitutively overexpressed in Beijing lineage (Domenech et al., 2017). DosR and WhiB3 have been shown to modulate lipid accumulation in M. tuberculosis (Singh et al., 2009), and also contribute to bacilli adaptation to hypoxia and redox stresses, respectively (Park et al., 2003; Saini et al., 2004; Singh et al., 2009). These proteins may play a role in the accumulation of lipid inclusions under oxidative stress and iron deficiency in clinical M. tuberculosis isolates. The mechanism of formation of lipid inclusions in mycobacteria also involves interactions with host lipid droplets and membrane phospholipids (Barisch and Soldati, 2017b). Thus, host stresses may induce significant cell biological adaptations in clinical M. tuberculosis isolates; its molecular mechanism needs to be further investigated.
In addition to reduction in the thickness of ETL and accumulation of lipid inclusions in M. tuberculosis cells, we also observed intracytoplasmic compartments under iron deficiency. These compartments were approximately 200 nm in size and were specifically observed in all M. tuberculosis isolates cultured under 500 μM DFO. It is possible that these compartments are mesosomes as observed in bacteria treated with antibiotics (Santhana Raj et al., 2007; Li et al., 2008). Studies have also shown the formation of intracellular compartments which accumulate H2O2 under cellular damage (Ebersold et al., 1981; Li et al., 2008; Xin et al., 2014). Mesosomes and other such intracellular structures are considered as ultrastructural artifact induced under chemical fixation and dehydration process, and these are not observed under cryo-electron microscopy lacking such fixation (Pilhofer et al., 2010). In this study we have used primary fixation with osmium tetroxide for 1 h, and post-fixation with glutaraldehyde for 2 h. There is a possibility of such chemical fixation inducing the formation of intracellular structures, specifically under stress conditions. Cellular adaptations under iron deficiency may increase the probability of formation of such structures during chemical fixation, as we observed them only in M. tuberculosis cells under iron deficiency. In cryoelectron microscopy cells are imaged at frozen-hydrated state without chemical fixation or dehydration of cells and can avoid much of the fixation artifacts (Pilhofer et al., 2010). Cytoplasmic structure termed as stack has been reported in slow growing Pseudomonas deceptionensis M1 by TEM and also confirmed by cryo-electron microscopy (Delgado et al., 2013). If confirmed to be a true cellular structure by cryoelectron microscopy and specific for M. tuberculosis in iron deficiency. These compartments probably may have a role in iron storage.
Iron limitation has been a common host defense encountered by M. tuberculosis; hence, it has evolved mechanisms to sequester iron from the host by using siderophores like mycobactin (Rodriguez and Smith, 2003; Ratledge, 2004). Inside M. tuberculosis cells bacterioferritins BfrA and BfrB function as iron storage proteins. Recent observations have shown that BfrB can be encapsulated by the protein encapsulin to form nanocompartments in vitro (Reddy et al., 2012; Contreras et al., 2014). We observed arrangement of units with size ∼20 nm inside these intracytoplasmic compartments in M. tuberculosis cells, which is similar in size to the encapsulin observed in vitro (Contreras et al., 2014). These observations suggest that these intracytoplasmic compartments may be encapsulin-based nanocompartments in M. tuberculosis. They may be used to isolate excess of iron from generating oxidative cellular damage or a similar protective function under stress (Contreras et al., 2014). Iron storage has been essential for M. tuberculosis survival and virulence, hence has also been a potential novel drug target (Pandey and Rodriguez, 2012). It is important to investigate further the nature of these intracytoplasmic compartments in avirulent laboratory strains by cryo-electron microscopy and its role in M. tuberculosis survival under iron deficiency. Recent observations further implicate survival of M. tuberculosis in iron deficiency and accumulation of lipid inclusions to antibiotic tolerance and persistence (Baron et al., 2017; Kurthkoti et al., 2017).
In summary, we were able to demonstrate the major cellular adaptations of clinical M. tuberculosis isolates to host and antibiotic stress conditions. Further investigation of these cellular adaptations and their role in M. tuberculosis survival under stress is important. These will aide in our understanding of the ability of M. tuberculosis cells to persist during host and antibiotic stress. The variations in cellular response among clinical M. tuberculosis isolates may be associated with the persistence and treatment outcome among patients.
Author Contributions
SV, NT, GT, NP, and EJ conceived and designed the experiments. SV, HH, and DT did the experiments. SV and AP did TEM analysis. SV, NT, GT, EJ, and AP analyzed and interpreted the data. SV, HH, DT, NP, NT, GT, AP, and EJ drafted and revised the manuscript and approved the final version.
Funding
This work was supported by the Wellcome Trust Training Fellowship in Public Health and Tropical Medicine (grant 097124/Z/11/Z to NT); the Wellcome Trust supporting the Major Overseas Program in Vietnam (grant 106680/Z/14/Z to GT).
Conflict of Interest Statement
The authors declare that the research was conducted in the absence of any commercial or financial relationships that could be construed as a potential conflict of interest.
Acknowledgments
We acknowledge the work of staff from the District TB units in Districts 4 and 8, HCMC, particularly Drs. Pham Thi Thuy Lieu and Nguyen Van Thom, who initially diagnosed and studied the patients. We would like to thank all patients who participated in this study.
Supplementary Material
The Supplementary Material for this article can be found online at: https://www.frontiersin.org/articles/10.3389/fmicb.2017.02681/full#supplementary-material
References
Abrahams, K. A., and Besra, G. S. (2016). Mycobacterial cell wall biosynthesis: a multifaceted antibiotic target. Parasitology doi: 10.1017/S0031182016002377 [Epub ahead of print].
Anuchin, A. M., Mulyukin, A. L., Suzina, N. E., Duda, V. I., El-Registan, G. I., and Kaprelyants, A. S. (2009). Dormant forms of Mycobacterium smegmatis with distinct morphology. Microbiology 155(Pt 4), 1071–1079. doi: 10.1099/mic.0.023028-0
Bacon, J., Dover, L. G., Hatch, K. A., Zhang, Y., Gomes, J. M., Kendall, S., et al. (2007). Lipid composition and transcriptional response of Mycobacterium tuberculosis grown under iron-limitation in continuous culture: identification of a novel wax ester. Microbiology 153(Pt 5), 1435–1444. doi: 10.1099/mic.0.2006/004317-0
Baker, J. J., Johnson, B. K., and Abramovitch, R. B. (2014). Slow growth of Mycobacterium tuberculosis at acidic pH is regulated by phoPR and host-associated carbon sources. Mol. Microbiol. 94, 56–69. doi: 10.1111/mmi.12688
Barisch, C., and Soldati, T. (2017a). Mycobacterium marinum degrades both triacylglycerols and phospholipids from its Dictyostelium host to synthesise its own triacylglycerols and generate lipid inclusions. PLOS Pathog. 13:e1006095. doi: 10.1371/journal.ppat.1006095
Barisch, C., and Soldati, T. (2017b). Breaking fat! How mycobacteria and other intracellular pathogens manipulate host lipid droplets. Biochimie 141, 54–61. doi: 10.1016/j.biochi.2017.06.001
Baron, V. O., Chen, M., Clark, S. O., Williams, A., Hammond, R. J. H., Dholakia, K., et al. (2017). Label-free optical vibrational spectroscopy to detect the metabolic state of M. tuberculosis cells at the site of disease. Sci. Rep. 7:9844. doi: 10.1038/s41598-017-10234-z
Bhatt, A., Fujiwara, N., Bhatt, K., Gurcha, S. S., Kremer, L., Chen, B., et al. (2007). Deletion of kasB in Mycobacterium tuberculosis causes loss of acid-fastness and subclinical latent tuberculosis in immunocompetent mice. Proc. Natl. Acad. Sci. U.S.A. 104, 5157–5162. doi: 10.1073/pnas.0608654104
Bouley, D. M., Ghori, N., Mercer, K. L., Falkow, S., and Ramakrishnan, L. (2001). Dynamic nature of host-pathogen interactions in Mycobacterium marinum granulomas. Infect. Immun. 69, 7820–7831. doi: 10.1128/IAI.69.12.7820-7831.2001
Brennan, P. J., and Nikaido, H. (1995). The envelope of mycobacteria. Annu. Rev. Biochem. 64, 29–63. doi: 10.1146/annurev.bi.64.070195.000333
Briken, V., Porcelli, S. A., Besra, G. S., and Kremer, L. (2004). Mycobacterial lipoarabinomannan and related lipoglycans: from biogenesis to modulation of the immune response. Mol. Microbiol. 53, 391–403. doi: 10.1111/j.1365-2958.2004.04183.x
Caire-Brandli, I., Papadopoulos, A., Malaga, W., Marais, D., Canaan, S., Thilo, L., et al. (2014). Reversible lipid accumulation and associated division arrest of Mycobacterium avium in lipoprotein-induced foamy macrophages may resemble key events during latency and reactivation of tuberculosis. Infect. Immun. 82, 476–490. doi: 10.1128/IAI.01196-13
Chatterjee, D. (1997). The mycobacterial cell wall: structure, biosynthesis and sites of drug action. Curr. Opin. Chem. Biol 1, 579–588. doi: 10.1016/S1367-5931(97)80055-5
Cohen, N. R., Lobritz, M. A., and Collins, J. J. (2013). Microbial persistence and the road to drug resistance. Cell Host Microbe. 13, 632–642. doi: 10.1016/j.chom.2013.05.009
Contreras, H., Joens, M. S., McMath, L. M., Le, V. P., Tullius, M. V., Kimmey, J. M., et al. (2014). Characterization of a Mycobacterium tuberculosis nanocompartment and its potential cargo proteins. J. Biol. Chem. 289, 18279–18289. doi: 10.1074/jbc.M114.570119
Daniel, J., Maamar, H., Deb, C., Sirakova, T. D., and Kolattukudy, P. E. (2011). Mycobacterium tuberculosis uses host triacylglycerol to accumulate lipid droplets and acquires a dormancy-like phenotype in lipid-loaded macrophages. PLOS Pathog. 7:e1002093. doi: 10.1371/journal.ppat.1002093
Deb, C., Lee, C. M., Dubey, V. S., Daniel, J., Abomoelak, B., Sirakova, T. D., et al. (2009). A novel in vitro multiple-stress dormancy model for Mycobacterium tuberculosis generates a lipid-loaded, drug-tolerant, dormant pathogen. PLOS ONE 4:e6077. doi: 10.1371/journal.pone.0006077
Delgado, L., Carrion, O., Martinez, G., Lopez-Iglesias, C., and Mercade, E. (2013). The stack: a new bacterial structure analyzed in the Antarctic bacterium Pseudomonas deceptionensis M1(T) by transmission electron microscopy and tomography. PLOS ONE 8:e73297. doi: 10.1371/journal.pone.0073297
Domenech, P., Zou, J., Averback, A., Syed, N., Curtis, D., Donato, S., et al. (2017). Unique regulation of the DosR regulon in the Beijing lineage of Mycobacterium tuberculosis. J. Bacteriol 199:e00696-16. doi: 10.1128/JB.00696-16
Ebersold, H. R., Cordier, J. L., and Luthy, P. (1981). Bacterial mesosomes: method dependent artifacts. Arch. Microbiol. 130, 19–22. doi: 10.1007/BF00527066
Etienne, G., Laval, F., Villeneuve, C., Dinadayala, P., Abouwarda, A., Zerbib, D., et al. (2005). The cell envelope structure and properties of Mycobacterium smegmatis mc2155: is there a clue for the unique transformability of the strain? Microbiology 151(Pt 6), 2075–2086.
Etienne, G., Villeneuve, C., Billman-Jacobe, H., Astarie-Dequeker, C., Dupont, M. A., and Daffe, M. (2002). The impact of the absence of glycopeptidolipids on the ultrastructure, cell surface and cell wall properties, and phagocytosis of Mycobacterium smegmatis. Microbiology 148(Pt 10), 3089–3100. doi: 10.1099/00221287-148-10-3089
Garton, N. J., Christensen, H., Minnikin, D. E., Adegbola, R. A., and Barer, M. R. (2002). Intracellular lipophilic inclusions of mycobacteria in vitro and in sputum. Microbiology 148(Pt 10), 2951–2958. doi: 10.1099/00221287-148-10-2951
Hammond, R. J., Baron, V. O., Oravcova, K., Lipworth, S., and Gillespie, S. H. (2015). Phenotypic resistance in mycobacteria: is it because I am old or fat that I resist you? J. Antimicrob. Chemother. 70, 2823–2827. doi: 10.1093/jac/dkv178
Hett, E. C., and Rubin, E. J. (2008). Bacterial growth and cell division: a mycobacterial perspective. Microbiol. Mol. Biol. Rev. 72, 126–156. doi: 10.1128/MMBR.00028-07
Hoffmann, C., Leis, A., Niederweis, M., Plitzko, J. M., and Engelhardt, H. (2008). Disclosure of the mycobacterial outer membrane: cryo-electron tomography and vitreous sections reveal the lipid bilayer structure. Proc. Natl. Acad. Sci. U.S.A. 105, 3963–3967. doi: 10.1073/pnas.0709530105
Jarlier, V., and Nikaido, H. (1994). Mycobacterial cell wall: structure and role in natural resistance to antibiotics. FEMS Microbiol. Lett. 123, 11–18. doi: 10.1111/j.1574-6968.1994.tb07194.x
Karakousis, P. C., Bishai, W. R., and Dorman, S. E. (2004). Mycobacterium tuberculosis cell envelope lipids and the host immune response. Cell Microbiol. 6, 105–116. doi: 10.1046/j.1462-5822.2003.00351.x
Kayigire, X. A., Friedrich, S. O., van der Merwe, L., Donald, P. R., and Diacon, A. H. (2015). Simultaneous staining of sputum smears for acid-fast and lipid-containing Myobacterium tuberculosis can enhance the clinical evaluation of antituberculosis treatments. Tuberculosis 95, 770–779. doi: 10.1016/j.tube.2015.08.001
Kieser, K. J., and Rubin, E. J. (2014). How sisters grow apart: mycobacterial growth and division. Nat. Rev. Microbiol. 12, 550–562. doi: 10.1038/nrmicro3299
Kurthkoti, K., Amin, H., Marakalala, M. J., Ghanny, S., Subbian, S., Sakatos, A., et al. (2017). The capacity of Mycobacterium tuberculosis to survive iron starvation might enable it to persist in iron-deprived microenvironments of human granulomas. mBio 8:e01092-17. doi: 10.1128/mBio.01092-17
Li, X., Feng, H. Q., Pang, X. Y., and Li, H. Y. (2008). Mesosome formation is accompanied by hydrogen peroxide accumulation in bacteria during the rifampicin effect. Mol. Cell. Biochem. 311, 241–247. doi: 10.1007/s11010-007-9690-4
Makinoshima, H., and Glickman, M. S. (2005). Regulation of Mycobacterium tuberculosis cell envelope composition and virulence by intramembrane proteolysis. Nature 436, 406–409. doi: 10.1038/nature03713
Marrero, J., Trujillo, C., Rhee, K. Y., and Ehrt, S. (2013). Glucose phosphorylation is required for Mycobacterium tuberculosis persistence in mice. PLOS Pathog. 9:e1003116. doi: 10.1371/journal.ppat.1003116
Mdluli, K., Swanson, J., Fischer, E., Lee, R. E., and Barry, C. E. III. (1998). Mechanisms involved in the intrinsic isoniazid resistance of Mycobacterium avium. Mol. Microbiol. 27, 1223–1233. doi: 10.1046/j.1365-2958.1998.00774.x
Munoz-Elias, E. J., and McKinney, J. D. (2005). Mycobacterium tuberculosis isocitrate lyases 1 and 2 are jointly required for in vivo growth and virulence. Nat. Med. 11, 638–644. doi: 10.1038/nm1252
Pal, R., Hameed, S., and Fatima, Z. (2015). Iron deprivation affects drug susceptibilities of mycobacteria targeting membrane integrity. J. Pathog. 2015:938523. doi: 10.1155/2015/938523
Palanisamy, G. S., Kirk, N. M., Ackart, D. F., Obregon-Henao, A., Shanley, C. A., Orme, I. M., et al. (2012). Uptake and accumulation of oxidized low-density lipoprotein during Mycobacterium tuberculosis infection in guinea pigs. PLOS ONE 7:e34148. doi: 10.1371/journal.pone.0034148
Pandey, A. K., and Sassetti, C. M. (2008). Mycobacterial persistence requires the utilization of host cholesterol. Proc. Natl. Acad. Sci. U.S.A. 105, 4376–4380. doi: 10.1073/pnas.0711159105
Pandey, R., and Rodriguez, G. M. (2012). A ferritin mutant of Mycobacterium tuberculosis is highly susceptible to killing by antibiotics and is unable to establish a chronic infection in mice. Infect. Immun. 80, 3650–3659. doi: 10.1128/IAI.00229-12
Park, H. D., Guinn, K. M., Harrell, M. I., Liao, R., Voskuil, M. I., Tompa, M., et al. (2003). Rv3133c/dosR is a transcription factor that mediates the hypoxic response of Mycobacterium tuberculosis. Mol. Microbiol. 48, 833–843. doi: 10.1046/j.1365-2958.2003.03474.x
Peyron, P., Vaubourgeix, J., Poquet, Y., Levillain, F., Botanch, C., Bardou, F., et al. (2008). Foamy macrophages from tuberculous patients’ granulomas constitute a nutrient-rich reservoir for M. tuberculosis persistence. PLOS Pathog. 4:e1000204. doi: 10.1371/journal.ppat.1000204
Pilhofer, M., Ladinsky, M. S., McDowall, A. W., and Jensen, G. J. (2010). Bacterial TEM: new insights from cryo-microscopy. Methods Cell Biol. 96, 21–45. doi: 10.1016/S0091-679X(10)96002-0
Rastogi, S., Singh, A. K., Chandra, G., Kushwaha, P., Pant, G., Singh, K., et al. (2017). The diacylglycerol acyltransferase Rv3371 of Mycobacterium tuberculosis is required for growth arrest and involved in stress-induced cell wall alterations. Tuberculosis 104, 8–19. doi: 10.1016/j.tube.2017.02.001
Ratledge, C. (2004). Iron, mycobacteria and tuberculosis. Tuberculosis 84, 110–130. doi: 10.1016/j.tube.2003.08.012
Reddy, P. V., Puri, R. V., Khera, A., and Tyagi, A. K. (2012). Iron storage proteins are essential for the survival and pathogenesis of Mycobacterium tuberculosis in THP-1 macrophages and the guinea pig model of infection. J. Bacteriol. 194, 567–575. doi: 10.1128/JB.05553-11
Reed, M. B., Gagneux, S., Deriemer, K., Small, P. M., and Barry, C. E. III (2007). The W-Beijing lineage of Mycobacterium tuberculosis overproduces triglycerides and has the DosR dormancy regulon constitutively upregulated. J. Bacteriol. 189, 2583–2589. doi: 10.1128/JB.01670-06
Reynolds, E. S. (1963). The use of lead citrate at high pH as an electron-opaque stain in electron microscopy. J. Cell Biol. 17, 208–212. doi: 10.1083/jcb.17.1.208
Rodriguez, G. M., and Smith, I. (2003). Mechanisms of iron regulation in mycobacteria: role in physiology and virulence. Mol. Microbiol. 47, 1485–1494. doi: 10.1046/j.1365-2958.2003.03384.x
Russell, D. G., Cardona, P. J., Kim, M. J., Allain, S., and Altare, F. (2009). Foamy macrophages and the progression of the human tuberculosis granuloma. Nat. Immunol. 10, 943–948. doi: 10.1038/ni.1781
Saini, D. K., Malhotra, V., Dey, D., Pant, N., Das, T. K., and Tyagi, J. S. (2004). DevR-DevS is a bona fide two-component system of Mycobacterium tuberculosis that is hypoxia-responsive in the absence of the DNA-binding domain of DevR. Microbiology 150(Pt 4), 865–875. doi: 10.1099/mic.0.26218-0
Santhana Raj, L., Hing, H. L., Baharudin, O., Teh Hamidah, Z., Aida Suhana, R., Nor Asiha, C. P., et al. (2007). Mesosomes are a definite event in antibiotic-treated Staphylococcus aureus ATCC 25923. Trop. Biomed. 24, 105–109.
Schnappinger, D., Ehrt, S., Voskuil, M. I., Liu, Y., Mangan, J. A., Monahan, I. M., et al. (2003). Transcriptional adaptation of Mycobacterium tuberculosis within macrophages: insights into the phagosomal environment. J. Exp. Med. 198, 693–704. doi: 10.1084/jem.20030846
Schneider, C. A., Rasband, W. S., and Eliceiri, K. W. (2012). NIH Image to ImageJ: 25 years of image analysis. Nat. Methods 9, 671–675. doi: 10.1038/nmeth.2089
Sebastian, J., Swaminath, S., Nair, R. R., Jakkala, K., Pradhan, A., and Ajitkumar, P. (2017). De novo emergence of genetically resistant mutants of Mycobacterium tuberculosis from the persistence phase cells formed against antituberculosis drugs in vitro. Antimicrob. Agents Chemother. 61:e01343-16. doi: 10.1128/AAC.01343-16
Singh, A., Crossman, D. K., Mai, D., Guidry, L., Voskuil, M. I., Renfrow, M. B., et al. (2009). Mycobacterium tuberculosis WhiB3 maintains redox homeostasis by regulating virulence lipid anabolism to modulate macrophage response. PLOS Pathog. 5:e1000545. doi: 10.1371/journal.ppat.1000545
Sloan, D. J., Mwandumba, H. C., Garton, N. J., Khoo, S. H., Butterworth, A. E., Allain, T. J., et al. (2015). Pharmacodynamic modeling of bacillary elimination rates and detection of bacterial lipid bodies in sputum to predict and understand outcomes in treatment of pulmonary tuberculosis. Clin. Infect. Dis. 61, 1–8. doi: 10.1093/cid/civ195
Takade, A., Takeya, K., Taniguchi, H., and Mizuguchi, Y. (1983). Electron microscopic observations of cell division in Mycobacterium vaccae V1. J. Gen. Microbiol. 129, 2315–2320. doi: 10.1099/00221287-129-7-2315
Thanky, N. R., Young, D. B., and Robertson, B. D. (2007). Unusual features of the cell cycle in mycobacteria: polar-restricted growth and the snapping-model of cell division. Tuberculosis 87, 231–236. doi: 10.1016/j.tube.2006.10.004
Tima, H. G., Al Dulayymi, J. R., Denis, O., Lehebel, P., Baols, K. S., Mohammed, M. O., et al. (2017). Inflammatory properties and adjuvant potential of synthetic glycolipids homologous to mycolate esters of the cell wall of Mycobacterium tuberculosis. J. Innate Immun. 9, 162–180. doi: 10.1159/000450955
Timmins, G. S., and Deretic, V. (2006). Mechanisms of action of isoniazid. Mol. Microbiol. 62, 1220–1227. doi: 10.1111/j.1365-2958.2006.05467.x
Torrelles, J. B., and Schlesinger, L. S. (2010). Diversity in Mycobacterium tuberculosis mannosylated cell wall determinants impacts adaptation to the host. Tuberculosis 90, 84–93. doi: 10.1016/j.tube.2010.02.003
Velayati, A. A., Farnia, P., Ibrahim, T. A., Haroun, R. Z., Kuan, H. O., Ghanavi, J., et al. (2009). Differences in cell wall thickness between resistant and nonresistant strains of Mycobacterium tuberculosis: using transmission electron microscopy. Chemotherapy 55, 303–307. doi: 10.1159/000226425
Vijay, S., Anand, D., and Ajitkumar, P. (2012). Unveiling unusual features of formation of septal partition and constriction in mycobacteria–an ultrastructural study. J. Bacteriol. 194, 702–707. doi: 10.1128/JB.06184-11
Vijay, S., Nagaraja, M., Sebastian, J., and Ajitkumar, P. (2014). Asymmetric cell division in Mycobacterium tuberculosis and its unique features. Arch. Microbiol. 196, 157–168. doi: 10.1007/s00203-014-0953-7
Vijay, S., Vinh, D. N., Hai, H. T., Ha, V. T. N., Dung, V. T. M., Dinh, T. D., et al. (2017). Influence of stress and antibiotic resistance on cell-length distribution in Mycobacterium tuberculosis clinical isolates. Front. Microbiol. 8:2296. doi: 10.3389/fmicb.2017.02296
Voskuil, M. I., Bartek, I. L., Visconti, K., and Schoolnik, G. K. (2011). The response of mycobacterium tuberculosis to reactive oxygen and nitrogen species. Front. Microbiol. 2:105. doi: 10.3389/fmicb.2011.00105
Wang, L., Slayden, R. A., Barry, C. E. III, and Liu, J. (2000). Cell wall structure of a mutant of Mycobacterium smegmatis defective in the biosynthesis of mycolic acids. J. Biol. Chem. 275, 7224–7229. doi: 10.1074/jbc.275.10.7224
World Health Organization [WHO] (2015). Global Tuberculosis Report 2015. Geneva: World Health Organization.
Xin, L., Lipeng, Y., Jiaju, Q., Hanqing, F., Yunhong, L., Min, Z., et al. (2014). Revisiting the mesosome as a novel site of hydrogen peroxide accumulation in Escherichia coli. Curr. Microbiol. 69, 549–553. doi: 10.1007/s00284-014-0617-5
Yamada, H., Mitarai, S., Chikamatsu, K., Mizuno, K., and Yamaguchi, M. (2010). Novel freeze-substitution electron microscopy provides new aspects of virulent Mycobacterium tuberculosis with visualization of the outer membrane and satisfying biosafety requirements. J. Microbiol. Methods 80, 14–18. doi: 10.1016/j.mimet.2009.09.022
Yamada, H., Yamaguchi, M., Chikamatsu, K., Aono, A., and Mitarai, S. (2015). Structome analysis of virulent Mycobacterium tuberculosis, which survives with only 700 ribosomes per 0.1 fl of cytoplasm. PLOS ONE 10:e0117109. doi: 10.1371/journal.pone.0117109
Keywords: Mycobacterium tuberculosis, ultrastructure, intracytoplasmic lipid inclusions, cell envelope, oxidative stress, iron deficiency and mesosome
Citation: Vijay S, Hai HT, Thu DDA, Johnson E, Pielach A, Phu NH, Thwaites GE and Thuong NTT (2018) Ultrastructural Analysis of Cell Envelope and Accumulation of Lipid Inclusions in Clinical Mycobacterium tuberculosis Isolates from Sputum, Oxidative Stress, and Iron Deficiency. Front. Microbiol. 8:2681. doi: 10.3389/fmicb.2017.02681
Received: 22 September 2017; Accepted: 22 December 2017;
Published: 11 January 2018.
Edited by:
Daniela De Biase, Sapienza Università di Roma, ItalyReviewed by:
Etienne Dague, Centre National de la Recherche Scientifique (CNRS), FranceGerald Larrouy-Maumus, Imperial College London, United Kingdom
Marcos André Vannier-Santos, Instituto Oswaldo Cruz, Fundação Oswaldo Cruz, Brazil
Copyright © 2018 Vijay, Hai, Thu, Johnson, Pielach, Phu, Thwaites and Thuong. This is an open-access article distributed under the terms of the Creative Commons Attribution License (CC BY). The use, distribution or reproduction in other forums is permitted, provided the original author(s) or licensor are credited and that the original publication in this journal is cited, in accordance with accepted academic practice. No use, distribution or reproduction is permitted which does not comply with these terms.
*Correspondence: Nguyen T. T. Thuong, dGh1b25nbnR0QG91Y3J1Lm9yZw==