- 1Department of Microbial Ecology, Netherlands Institute of Ecology (NIOO-KNAW), Wageningen, Netherlands
- 2Laboratory of Phytopathology, Wageningen University and Research, Wageningen, Netherlands
- 3Biointeractions and Plant Health, Plant Research International, Wageningen University and Research, Wageningen, Netherlands
- 4Institute of Biology, Leiden University, Leiden, Netherlands
Disease suppressive soils offer effective protection to plants against infection by soil-borne pathogens, including fungi, oomycetes, bacteria, and nematodes. The specific disease suppression that operates in these soils is, in most cases, microbial in origin. Therefore, suppressive soils are considered as a rich resource for the discovery of beneficial microorganisms with novel antimicrobial and other plant protective traits. To date, several microbial genera have been proposed as key players in disease suppressiveness of soils, but the complexity of the microbial interactions as well as the underlying mechanisms and microbial traits remain elusive for most disease suppressive soils. Recent developments in next generation sequencing and other ‘omics’ technologies have provided new insights into the microbial ecology of disease suppressive soils and the identification of microbial consortia and traits involved in disease suppressiveness. Here, we review the results of recent ‘omics’-based studies on the microbial basis of disease suppressive soils, with specific emphasis on the role of rhizosphere bacteria in this intriguing microbiological phenomenon.
Introduction
Disease suppressive soils are the best examples of microbiome-mediated protection of plants against root infections by soil-borne pathogens. Disease suppressive soils were originally defined by Baker and Cook (1974) as “soils in which the pathogen does not establish or persist, establishes but causes little or no damage, or establishes and causes disease for a while but thereafter the disease is less important, although the pathogen may persist in the soil.” In contrast, disease readily occurs in non-suppressive (or conducive) soils where abiotic and biotic conditions are favorable to the pathogen. Two types of disease suppressiveness are distinguished. General suppressiveness of soils is attributed to the activity of the collective microbial community and is often associated with competition for available resources (Mazzola, 2002; Weller et al., 2002). General suppressiveness of soils can be boosted by addition of organic matter (Bonanomi et al., 2010; Klein et al., 2013; Vitullo et al., 2013; Postma and Schilder, 2015; Mazzola and Freilich, 2016; Tomihama et al., 2016). Specific suppressiveness is due to the concerted activities of specific groups of microorganisms that interfere with some stage of the life cycle of the soil-borne pathogen. Specific suppressiveness of soils can, in contrast to general suppressiveness, be transferred to conducive soils by mixing small amounts (1–10% w/w) of the suppressive soil into the conducive soil (Mendes et al., 2011; Raaijmakers and Mazzola, 2016; van der Voort et al., 2016). The characteristics of general and specific suppressiveness have remarkable similarities with the innate and adaptive immune responses in animals (Raaijmakers and Mazzola, 2016). That is, the innate immune response in animals gives a primary and non-specific defensive response similar to what occurs in general suppressiveness of soils. The adaptive immune response in animals and specific disease suppression in soils both require specialized cells to suppress the pathogen, require time and have a memory (Lapsansky et al., 2016; Raaijmakers and Mazzola, 2016). Hence, a mechanistic understanding of the soil immune response may enable us to engineer the soil and plant microbiomes to enrich for specific groups of antagonistic microbes and activities as a sustainable alternative to control plant diseases and to enhance crop productivity (Berendsen et al., 2012; Mendes et al., 2013; Mueller and Sachs, 2015). Here, we will first highlight the most important findings of past studies on microbes and mechanisms involved in specific disease suppressiveness of soils. We will then review recent findings from ‘omics’-based studies on the role of soil and rhizosphere bacteria in this intriguing microbiological phenomenon and finally provide a brief outlook.
Brief History of Disease Suppressive Soils
The first suppressive soil was reported in 1892 by Atkinson for Fusarium wilt disease of cotton (Atkinson, 1892; Scher and Baker, 1980; Amir and Alabouvette, 1993; Lemanceau et al., 2006). Since then, specific suppressiveness of soils has been reported for a range of pathogens, including fungi such as Gaeumannomyces graminis var tritici (Raaijmakers and Weller, 1998; De Souza et al., 2003), Fusarium oxysporum (Scher and Baker, 1980; Alabouvette, 1986; Klein et al., 2013), Fusarium solani (Burke, 1954; Kobayashi and Komada, 1995), Rhizoctonia solani (Wijetunga and Baker, 1979; Chern and Ko, 1989; Postma et al., 2010; Mendes et al., 2011), Verticillium dahliae (Keinath and Fravel, 1992), Pyrenochaeta lycopersici (Workneh and Van Bruggen, 1994), Sclerotinia sclerotiorum (Rodríguez et al., 2015), Alternaria triticina (Siddiqui, 2007), oomycetes such as Phytophthora cinnamomi (Broadbent and Baker, 1974), Pythium ultimum (Martin and Hancock, 1986), and Aphanomyces euteiches (Persson and Olsson, 2000), bacteria such as Streptomyces scabies (Menzies, 1959; Weinhold et al., 1964; Kinkel et al., 2012; Meng et al., 2012; Rosenzweig et al., 2012), Ralstonia solanacearum (Shiomi et al., 1999) and Agrobacterium radiobacter var tumefaciens (New and Kerr, 1972), protists such as Plasmodiophora brassicae (Hjort et al., 2007) and nematodes such as Meloidogyne incognita (Pyrowolakis et al., 2002; Giné et al., 2016), Heterodera schachtii (Olatinwo et al., 2006), Heterodera glycines (Song et al., 2016), and Criconemella xenoplax (Kluepfel et al., 1993).
The microbiological basis of disease suppressive soils was first addressed by Henry (1931a,b) and later widely demonstrated in other studies via soil pasteurization, application of biocides (Scher and Baker, 1980; Alabouvette, 1986; Mazzola, 2002; Weller et al., 2002; Garbeva et al., 2004) and via soil transplantation (Scher and Baker, 1980; Alabouvette, 1986; Wiseman et al., 1996; Weller et al., 2002; Mendes et al., 2011). Furthermore, higher microbial diversities have been detected in disease suppressive soils than in conducive soils (Garbeva et al., 2006). Following these observations and approaches, various microbes and underlying mechanisms involved in specific disease suppressiveness were proposed and, in several cases, identified. The mechanisms underlying specific suppressiveness identified in these early studies include competition, parasitism and antibiosis (Kloepper et al., 1980; Scher and Baker, 1980; Neeno-Eckwall et al., 2001; Mazzola, 2002; Alabouvette et al., 2009; Junaid et al., 2013; Jambhulkar et al., 2015). For Fusarium wilt suppressive soils, competition for carbon by non-pathogenic F. oxysporum (Alabouvette, 1986; Couteaudier and Alabouvette, 1990; Neeno-Eckwall et al., 2001) and siderophore-mediated competition for iron by rhizosphere bacteria (Kloepper et al., 1980; Scher and Baker, 1982; Lemanceau et al., 1988) were shown to be key mechanisms. Addition of siderophore-producing Pseudomonas from suppressive soils or their siderophores into conducive soils rendered these soils suppressive to F. oxysporum and also G. graminis, the take-all pathogen of wheat and barley (Kloepper et al., 1980; Scher and Baker, 1982; Lemanceau et al., 1988; Couteaudier and Alabouvette, 1990). The role of parasitism in disease suppressive soils has been studied for several soil-borne pathogens including the fungi S. sclerotiorum (Gerlagh et al., 1999; Rey et al., 2005; Li et al., 2006; Whipps et al., 2008), S. minor (Partridge et al., 2006), R. solani (Chet et al., 1981; Velvis and Jager, 1983; van den Boogert et al., 1989), F. oxysporum (Toyota and Kimura, 1993), and Cochliobolus spp. (Fradkin and Patrick, 1985), and the oomycetes P. ultimum and P. aphanidermatum (Chet et al., 1981). Parasitic microorganisms identified in these studies were mostly fungi (e.g., Trichoderma spp., Coniothyrium minitans, Verticillium biguttatum) or oomycetes (Pythium oligandrum). Despite the widespread distribution of rhizosphere bacteria with parasitic traits, such as the production of cell wall degrading enzymes, there are no studies that have conclusively demonstrated their role in specific disease suppressiveness of soils. For example, strains of Stenotrophomonas maltophilia can suppress the oomycete P. ultimum and the nematode Bursaphelenchus xylophilus via the production of proteases (Dunne et al., 1997; Huang et al., 2009). Likewise, Pseudomonas fluorescens CHA0 reduces root-knot caused by M. incognita, at least in part, via the production of a protease (Siddiqui et al., 2005). Furthermore, bacteria within the genus Collimonas produce chitinases and have been reported to feed on fungi (De Boer et al., 2001). Whether these or other mycoparasitic rhizobacterial genera are enriched or more active in disease suppressive soils is, to our knowledge, not yet known. Antibiosis, defined as the inhibition of the growth and/or activity of one organism by another organism via the production of specific or non-specific metabolites (Thomashow and Pierson, 1991), is the most widely studied mechanism of disease suppressive soils. Among the antibiotics with a role in disease suppressive soils, 2,4-diacetylphloroglucinol (DAPG) and phenazines (PHZ) have been studied in more depth (Haas and Defago, 2005; Raaijmakers and Mazzola, 2012). Both DAPG and PHZ are produced by several strains of (fluorescent) Pseudomonas species associated with soils suppressive to take-all of wheat or Fusarium wilt of flax (Raaijmakers and Weller, 1998; Weller et al., 2002; Mazurier et al., 2009). DAPG and pyrrolnitrin were shown to be involved in suppression of R. solani (Latz et al., 2012), whereas PHZ and pyoluteorin were associated with suppression of Thielaviopsis basicola (Laville et al., 1992; Haas and Defago, 2005). Volatile compounds with antimicrobial activities have also been proposed to play a role in disease suppressiveness of soils. Early studies indicated a role of ammonia (Ko et al., 1974; Howell et al., 1988) and hydrogen cyanide (Voisard et al., 1989) in disease suppressiveness.
Old and New Approaches to Study Disease Suppressive Soils
After demonstrating the microbial basis of disease suppressiveness of soils by heat treatment, biocides and/or soil transplantations, the next steps taken in past and several present studies typically comprises untargeted, large-scale isolation of microbes from bulk soil, rhizosphere or endosphere of plants grown in disease suppressive soils, followed by testing their activities against the target pathogen both in vitro (i.e., plate assays) and in vivo (i.e., introduction into conducive soils). Following this line of research, several microbial genera have been proposed for their role in specific disease suppressiveness. These include (fluorescent) Pseudomonas (Kloepper et al., 1980; Scher and Baker, 1980, 1982; Wong and Baker, 1984; Lemanceau and Alabouvette, 1991; Raaijmakers and Weller, 1998; De Souza et al., 2003; Perneel et al., 2007; Mazurier et al., 2009; Mendes et al., 2011; Michelsen and Stougaard, 2011), Streptomyces (Liu et al., 1996; Cha et al., 2016), Bacillus (Sneh et al., 1984; Cazorla et al., 2007; Abdeljalil et al., 2016; Zhang et al., 2016), Paenibacillus (Haggag and Timmusk, 2008), Enterobacter (Schisler and Slininger, 1994; Abdeljalil et al., 2016), Alcaligenes (Yuen and Schroth, 1986), Pantoea (Schisler and Slininger, 1994), non-pathogenic F. oxysporum (Sneh et al., 1987; Couteaudier and Alabouvette, 1990; Larkin et al., 1996; Larkin and Fravel, 2002; Nel et al., 2006; Mazurier et al., 2009; Raaijmakers et al., 2009), Trichoderma (Harman et al., 1980; Liu and Baker, 1980; Chet and Baker, 1981; Hadar et al., 1984; Smith et al., 1990; Mghalu et al., 2007), Penicillium janczewskii (Madi and Katan, 1998), V. biguttatum (Jager and Velvis, 1983; Velvis and Jager, 1983), Pochonia chlamydosporia (Yang et al., 2012), Clonostachys/Gliocadium (Smith et al., 1990; Rodríguez et al., 2015) and P. oligandrum (Martin and Hancock, 1986).
Although several microorganisms efficiently controlled the target pathogen under in vitro or greenhouse conditions, the majority failed under field environments. This inconsistency in in vivo activity has been mainly attributed to an insufficient ability to survive and colonize the rhizosphere or to express their protective characteristics under field conditions at the right time and right place (Alabouvette et al., 2009). Also, disease suppressiveness is generally thought to be attributed to microbial consortia rather than to one microbial species only. For example, PHZ-producing Pseudomonas isolated from a Fusarium wilt suppressive soil could suppress Fusarium wilt disease of flax only when re-introduced with non-pathogenic F. oxysporum Fo47 (Mazurier et al., 2009). Hence, application of synthetic communities composed of different microbial species with different modes of action has been suggested as an alternative to improve the consistency of controlling the pathogen in vivo (Großkopf and Soyer, 2014; Lebeis et al., 2015; Mazzola and Freilich, 2016).
To obtain a more comprehensive picture of the microbial consortia and specific activities operating in disease suppressive soils, several new, cultivation-independent technologies are now available, including community profiling by restriction fragment length polymorphism (RFLP) or denaturing gradient gel electrophoresis (DGGE), quantitative PCR (qPCR), DNA-Stable Isotope Probing (DNA-SIP), PhyloChip analysis, 16S- or ITS-amplicon sequencing, shotgun sequencing of metagenomic DNA, metatranscriptomics, metaproteomics and metabolomics. For example, bacterial and fungal diversity analyzed by DGGE and subsequent sequencing of the isolated bands showed a higher abundance of the fungi Aspergillus penicillioides, Eurotium sp., Ganoderma applanatum and Cylindrocarpon olidum and the bacteria Solirubrobacter soli, Ochrobactrum anthropi, Anderseniella sp., and Pseudomonas koreensis in soils suppressive to M. hapla (Adam et al., 2014). Also dominance of Fusarium spp., Cladosporium sphaerospermum and Aspergillus versicolor in a soil suppressive to H. glycines (Song et al., 2016) and higher abundances of the bacteria Sphingobacteriales, Flavobacteriaceae, Xanthomonadaceae, or Cyanobacteria and the fungi Fusarium, Preussia, Mortierella, or Cladosporium in soils suppressive to Meloidoyne spp. (Giné et al., 2016) were observed. Additionally, Cretoiu et al. (2013) analyzed the bacterial and fungal communities of a soil that became more suppressive toward V. dahliae upon addition of chitin and found, based on DGGE and qPCR analyses, that suppressiveness was mainly associated with higher abundances of Oxalobacteraceae and Actinobacteria and expression of the chitinase gene chiA. Using PhyloChip analyses of the rhizobacterial community compositions in soils suppressive or conducive to the fungal root pathogen R. solani, Mendes et al. (2011) and Chapelle et al. (2015) revealed that suppressiveness is not due to the exclusive presence or absence of specific rhizobacterial families but due to a change in their relative abundance and specific activities. The results of these and other recent studies are summarized in Table 1 and discussed below with emphasis on the role of soil and rhizosphere bacteria.
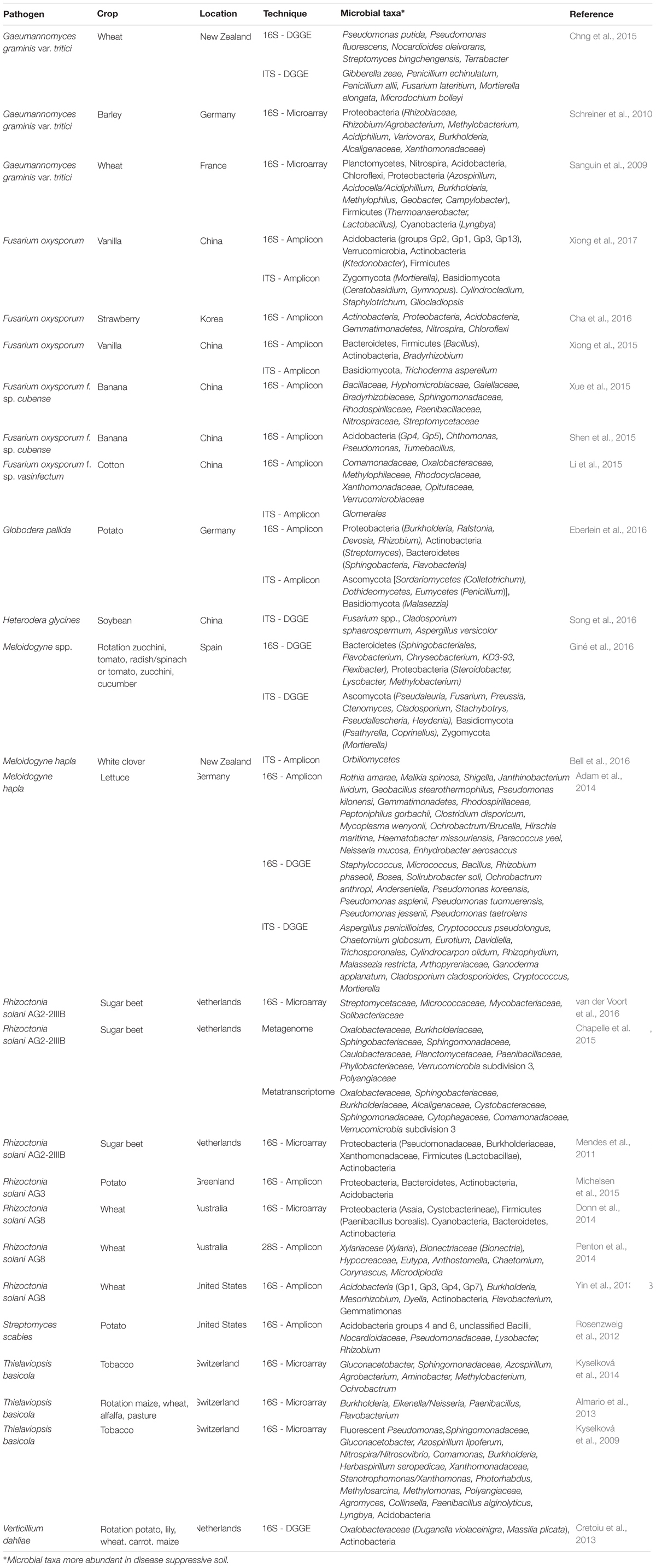
TABLE 1. Summary of microbial taxa associated with disease suppressive soils and identified by different cultivation-independent techniques.
New Insights into the Role of Rhizosphere Bacteria in Disease Suppressive Soils
Several ‘omics’-based studies have been conducted recently to compare the microbial (mainly bacterial) community composition of soils suppressive or conducive for specific plant pathogens, including F. oxysporum, G. graminis var. tritici, T. basicola, R. solani, S. scabies, or M. hapla. A wide range of bacterial taxa were found in higher abundance in suppressive soils (Table 1). With regard to fungi, Penton et al. (2014) further revealed that differences associated with disease suppressiveness of soils to R. solani on wheat were attributed to less than 40 fungal genera, including a number of endophytic species and mycoparasites. Among the fungi most frequently associated with disease suppressive soils to other pathogens are Mortierella, Trichoderma, Fusarium, and Malasezzia (Table 1). Recently, Poudel et al. (2016) highlighted the importance of constructing microbial networks to determine microbial community structure and assemblage for disease management. Documenting differences in relative abundance between bacterial and/or fungal communities in suppressive and conducive soils by network analyses can be highly instrumental to zoom in on specific microbial consortia. However, these descriptive analyses need to be combined with other techniques to pinpoint the specific microbial traits involved in suppressiveness and to distinguish between cause and effect.
Mechanistically, recent studies pointed to antimicrobial volatiles, including sesquiterpenes (Minerdi et al., 2009), methyl 2-methylpentanoate and 1,3,5-trichloro-2-methoxy benzene (Cordovez et al., 2015), 2-methylfuran, 2-furaldehyde, 2-(methythio)benzo thiazole and murolool (Hol et al., 2015) for their potential role in disease suppressive soils. In these studies, however, these volatile compounds were detected under in vitro conditions and their production in vivo should be validated to provide more conclusive proof of the role of antimicrobial volatiles in disease suppressiveness of soils. Nevertheless, its validation in situ has technical challenges since volatile-producing microorganisms should be positioned in their ecological context (the rhizosphere) but also physically separated from the pathogen to exclude the role of compounds other than volatiles. Among the antimicrobial peptides, specific emphasis has been given in recent studies to the role of lipopeptides in disease suppressive soils. In independent studies, the two structurally similar, chlorinated lipopeptides thanamycin and nunapeptin were shown to contribute to suppressiveness of soils against the fungal root pathogen R. solani (Mendes et al., 2011; Watrous et al., 2012; Michelsen et al., 2015). Furthermore, using a combination of different techniques, Cha et al. (2016) elegantly revealed that the production of the thiopeptide conprimycin by Streptomyces played a role in a soil suppressive to Fusarium wilt of strawberry. Next-generation sequencing analyses revealed an increase of Actinobacteria in this suppressive soil leading to the isolation and genomic characterization of Streptomyces isolate S4-7. Genome mining of Streptomyces S4-7 pointed at the production of conprimycin as a metabolite involved in suppressing Fusarium. A chemogenomic approach further suggested that conprimycin acts by interfering with fungal cell wall biosynthesis (Cha et al., 2016).
To further target the active microbial communities and to identify other microbial traits involved in disease suppressive soils, DNA-SIP (Radajewski et al., 2000) or metatranscriptomics (Ofek-Lalzar et al., 2014; Ofek et al., 2014; Tkacz et al., 2015) should be applied and/or combined. For example, by using metagenomic approaches Hjort et al. (2014) obtained a clone from a soil suppressive to club-root disease on cabbage producing the antifungal chitinase Chi18H8, and Cretoiu et al. (2015) obtained a clone producing the salt-tolerant chitinase 53D1 from a soil suppressive to V. dahliae. Within the METACONTROL project, several novel polyketide antibiotics were identified (Van Elsas et al., 2008). Furthermore, Chapelle et al. (2015) combined metagenomic and metatranscriptomic analyses to resolve the transcriptional changes in the rhizobacterial community of sugar beet plants in a Rhizoctonia-suppressive soil challenged with the fungal pathogen. They found that upon pathogen exposure, stress-related genes were upregulated in rhizobacteria belonging to the Oxalobacteraceae, Sphingobacteriaceae, Burkholderiaceae, Alcaligenaceae, Cystobacteraceae, Sphingomonadaceae, Cytophagaceae, Comamonadaceae, and Verrucomicrobia. Based on these results they proposed a model in which the fungal pathogen secretes oxalic and phenylacetic acid during colonization of the root system, thereby exerting oxidative stress in the rhizobacterial community as well as in the plant. This stress response in turn leads to the activation of survival strategies of the rhizobacterial community leading to enhanced motility, biofilm formation and the production of yet unknown secondary metabolites. Collectively, these recent studies exemplify that combining different approaches and technologies allows a more in-depth analysis of the microbial and chemical ecology of disease suppressive soils, as depicted in Figure 1.
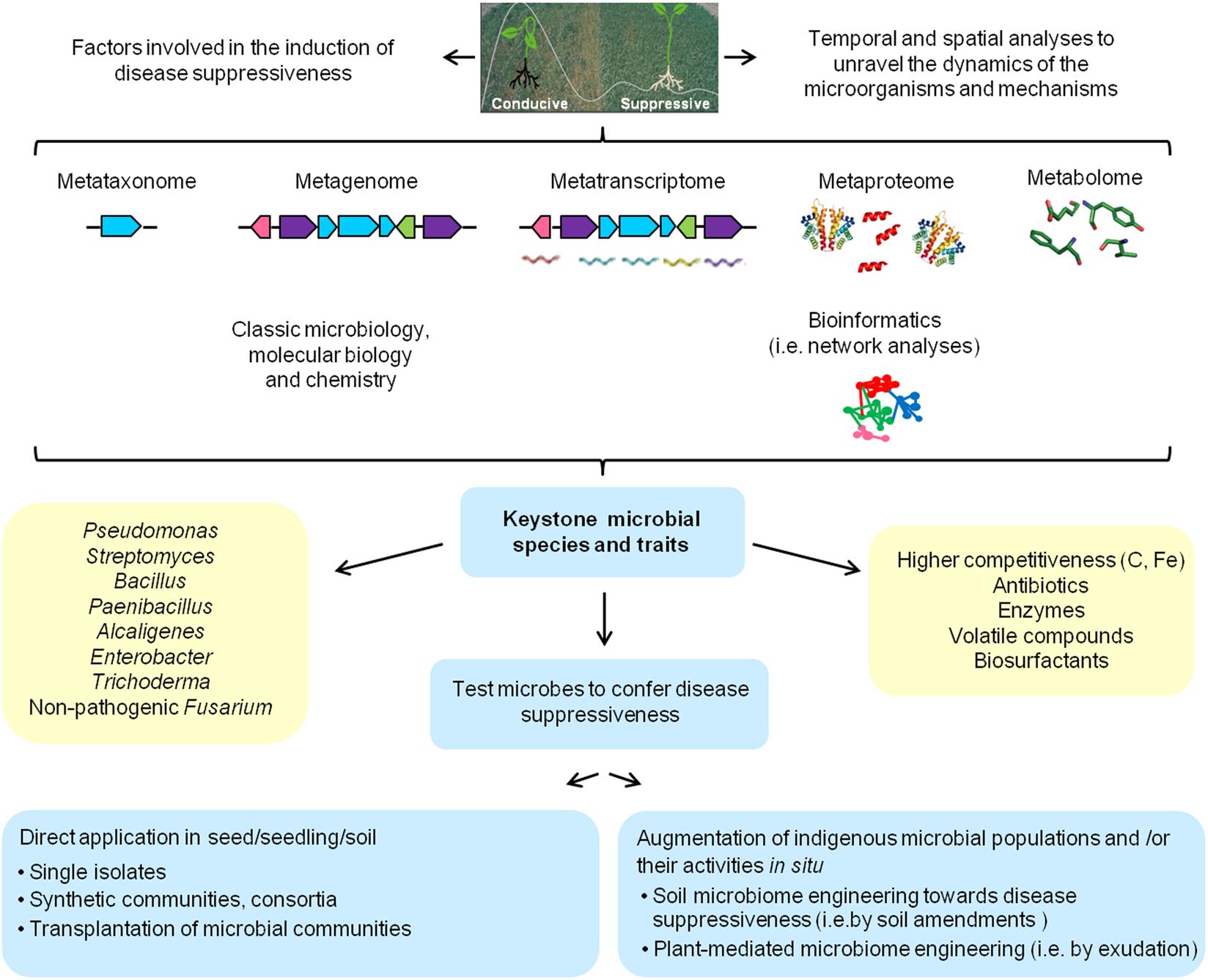
FIGURE 1. Schematic overview of currently available approaches involving microbiological, molecular, chemical and bioinformatic methods that can be adopted and integrated to generate a more complete picture of the microbial consortia and mechanisms involved in disease suppressive soils.
Future Perspectives
In the early days of research on disease suppressive soils, several valuable insights were obtained for the role of individual microbial genera (Weller et al., 2002). In most disease suppressive soils, however, suppressiveness appears to be due to the concerted activities of multiple microbial genera working together at specific sites or operating at different stages of the infection process of the pathogen. Understanding the temporal and spatial microbial dynamics of disease suppressive soils as well as the corresponding modes of action will be needed to facilitate the development of effective, consistent and durable disease management tools. A model predicting Fusarium wilt suppressiveness, including several soil factors combined with the abundance of three keystone microbial taxa, was designed recently by Trivedi et al. (2017) to support the choice of crops or cultivars referred to as “Know before you Sow.” Suppressive soils constitute a valuable source of biocontrol agents. Isolation of these microorganisms follows a “taxonomy-based” approach and their activities are typically tested in in vitro assays that do not mimic field conditions. Additionally, the re-introduction of these microorganisms in non-suppressive soils often lead to inconsistent protective activities, mostly driven by a lack of a sufficient root colonization and/or inhibition of their modes of action under field conditions. Relevant functions involved in disease suppressiveness can be executed by multiple microbial taxa, but metatranscriptome, metaproteome and metabolome studies of disease suppressive soils are still underrepresented. The combination of the “taxonomy-based” approaches with “trait-based” approaches would be preferred to unravel the complexity of the specific microorganisms and mechanisms underlying disease suppressiveness. Thus, rather than introducing beneficial microorganisms, agricultural research should focus on identifying the factors that influence key microorganisms or traits responsible for suppressiveness (Kinkel et al., 2014), meanwhile eliminating the practical and legislative difficulties of introducing microorganisms in the environment. Hence, research on management practices aiming to select or stimulate resident microbial communities or activities that enhance suppressiveness is emerging. Examples are the use of specific soil amendments including chitin (Cretoiu et al., 2013, 2014; Larkin, 2015; Postma and Schilder, 2015), chitosan (Ben-Shalom et al., 2003; Liu et al., 2012) or fish emulsion (Abbasi, 2013), the introduction of agricultural practices such as crop rotation or minimum tillage (Stirling et al., 2012; Schillinger and Paulitz, 2014; Duchene et al., 2017), or the use of cover crops (Ji et al., 2012), or by host-mediated microbiome engineering, where the protective microbiome is artificially selected over multiple generations (Mueller and Sachs, 2015).
Concluding Remarks
Crop losses due to plant pests and diseases are a common problem worldwide. Improving productivity is crucial to reduce rural poverty and to increase food security worldwide (Flood, 2010; Cerda et al., 2017). Therefore, managing and preserving soil health is essential for sustainable agriculture and optimum ecosystem functioning (Larkin, 2015). The use of pesticides is a traditional control strategy, but the development of pathogen resistance and an increasing public concern about the adverse effects on plant, animal and human health necessitate alternative and sustainable control methods. Engineering the soil and plant microbiome has been suggested as a novel and promising means for plant health (Mueller and Sachs, 2015). Moreover, Kinkel et al. (2011), Mazzola and Freilich (2016), and Raaijmakers and Mazzola (2016) further emphasized the need for analyzing the co-evolutionary processes leading to the assembly of a disease suppressive microbiome in soils. Understanding these processes will unravel “how” a soil becomes suppressive, allowing us to engineer the soil microbiome to jumpstart the onset of disease suppressiveness prior to pathogen invasion.
Author Contributions
RGE drafted the manuscript. IdB, JP, and JR contributed to the revision of the manuscript.
Conflict of Interest Statement
The authors declare that the research was conducted in the absence of any commercial or financial relationships that could be construed as a potential conflict of interest.
Acknowledgments
This manuscript is publication number 6444 of Netherlands Institute of Ecology (NIOO-KNAW). This research was funded by the Dutch Technology Foundation (NWO-TTW), project number STW11755.
References
Abbasi, P. A. (2013). Establishing suppressive conditions against soilborne potato diseases with low rates of fish emulsion applied serially as a pre-plant soil amendment. Can. J. Plant Pathol. 35, 10–19. doi: 10.1080/07060661.2012.740076
Abdeljalil, N. O.-B., Vallance, J., Gerbore, J., Rey, P., and Daami-Remadi, M. (2016). Bio-suppression of Sclerotinia stem rot of tomato and biostimulation of plant growth using tomato-associated rhizobacteria. J. Plant Pathol. Microbiol. 7:331. doi: 10.4172/2157-7471.1000331
Adam, M., Westphal, A., Hallmann, J., and Heuer, H. (2014). Specific microbial attachment to root knot nematodes in suppressive soil. Appl. Environ. Microbiol. 80, 2679–2686. doi: 10.1128/AEM.03905-13
Alabouvette, C. (1986). Fusarium-wilt suppressive soils from the Châteaurenard region: review of a 10-year study. Agronomie 6, 273–284. doi: 10.1051/agro:19860307
Alabouvette, C., Olivain, C., Migheli, Q., and Steinberg, C. (2009). Microbiological control of soil-borne phytopathogenic fungi with special emphasis on wilt-inducing Fusarium oxysporum. New Phytol. 184, 529–544. doi: 10.1111/j.1469-8137.2009.03014.x
Almario, J., Kyselková, M., Kopecký, J., Ságová-Marečková, M., Muller, D., Grundmann, G. L., et al. (2013). Assessment of the relationship between geologic origin of soil, rhizobacterial community composition and soil receptivity to tobacco black root rot in Savoie region (France). Plant Soil 371, 397–408. doi: 10.1007/s11104-013-1677-1
Amir, H., and Alabouvette, C. (1993). Involvement of soil abiotic factors in the mechanisms of soil suppressiveness to Fusarium wilts. Soil Biol. Biochem. 25, 157–164. doi: 10.1016/0038-0717(93)90022-4
Atkinson, G. F. (1892). Some Diseases of Cotton, Vol. 41. Auburn, AL: Agricultural Experiment Station of the Agricultural and Mechanical College, 65.
Baker, K., and Cook, R. J. (1974). Biological Control of Plant Pathogens. New York, NY: WH Freeman and Company, 433.
Bell, N. L., Adam, K. H., Jones, R. J., Johnson, R. D., Mtandavari, Y. F., Burch, G., et al. (2016). Detection of invertebrate suppressive soils, and identification of a possible biological control agent for Meloidogyne nematodes using high resolution rhizosphere microbial community analysis. Front. Plant Sci. 7:1946. doi: 10.3389/fpls.2016.01946
Ben-Shalom, N., Ardi, R., Pinto, R., Aki, C., and Fallik, E. (2003). Controlling gray mould caused by Botrytis cinerea in cucumber plants by means of chitosan. Crop Prot. 22, 285–290. doi: 10.1016/S0261-2194(02)00149-7
Berendsen, R. L., Pieterse, C. M., and Bakker, P. A. (2012). The rhizosphere microbiome and plant health. Trends Plant Sci. 17, 478–486. doi: 10.1016/j.tplants.2012.04.001
Bonanomi, G., Antignani, V., Capodilupo, M., and Scala, F. (2010). Identifying the characteristics of organic soil amendments that suppress soilborne plant diseases. Soil Biol. Biochem. 42, 136–144. doi: 10.1016/j.soilbio.2009.10.012
Broadbent, P., and Baker, K. F. (1974). Behaviour of Phytophthora cinnamomi in soils suppressive and conducive to root rot. Crop Pasture Sci. 25, 121–137. doi: 10.1071/AR9740121
Burke, D. (1954). “Pathogenicity of Fusarium solani f phaseoli in different soils”, in Phytopathology, ed. K. V. Subbarao (St Paul, MN: American Phytopathological Society), 483–483.
Cazorla, F., Romero, D., Pérez-García, A., Lugtenberg, B. J., Vicente, A. D., and Bloemberg, G. (2007). Isolation and characterization of antagonistic Bacillus subtilis strains from the avocado rhizoplane displaying biocontrol activity. J. Appl. Microbiol. 103, 1950–1959. doi: 10.1111/j.1365-2672.2007.03433.x
Cerda, R., Avelino, J., Gary, C., Tixier, P., Lechevallier, E., and Allinne, C. (2017). Primary and secondary yield losses caused by pests and diseases: assessment and modeling in coffee. PLOS ONE 12:e0169133. doi: 10.1371/journal.pone.0169133
Cha, J.-Y., Han, S., Hong, H. J., Cho, H., Kim, D., Kwon, Y., et al. (2016). Microbial and biochemical basis of a Fusarium wilt-suppressive soil. ISME J. 10, 119–129. doi: 10.1038/ismej.2015.95
Chapelle, E., Mendes, R., Bakker, P. A., and Raaijmakers, J. M. (2015). Fungal invasion of the rhizosphere microbiome. ISME J. 10, 265–268. doi: 10.1038/ismej.2015.82
Chern, L., and Ko, W. (1989). Characteristics of inhibition of suppressive soil created by monoculture with radish in the presence of Rhizoctonia solani. J. Phytopathol. 126, 237–245. doi: 10.1111/j.1439-0434.1989.tb01110.x
Chet, H., and Baker, R. (1981). Isolation and biocontrol potential of Trichoderma hamatum from soil naturally suppressive to Rhizoctonia solani. Phytopathology 71, 286–290. doi: 10.1094/Phyto-71-286
Chet, I., Harman, G. E., and Baker, R. (1981). Trichoderma hamatum: its hyphal interactions with Rhizoctonia solani and Pythium spp. Microb. Ecol. 7, 29–38. doi: 10.1007/BF02010476
Chng, S., Cromey, M., Dodd, S., Stewart, A., Butler, R. C., and Jaspers, M. V. (2015). Take-all decline in New Zealand wheat soils and the microorganisms associated with the potential mechanisms of disease suppression. Plant Soil 397, 239–259. doi: 10.1007/s11104-015-2620-4
Cordovez, V., Carrion, V. J., Etalo, D. W., Mumm, R., Zhu, H., van Wezel, G. P., et al. (2015). Diversity and functions of volatile organic compounds produced by Streptomyces from a disease-suppressive soil. Front. Microbiol. 6:1081. doi: 10.3389/fmicb.2015.01081
Couteaudier, Y., and Alabouvette, C. (1990). Quantitative comparison of Fusarium oxysporum competitiveness in relation to carbon utilization. FEMS Microbiol. Lett. 74, 261–267. doi: 10.1111/j.1574-6968.1990.tb04072.x
Cretoiu, M. S., Berini, F., Kielak, A. M., Marinelli, F., and van Elsas, J. D. (2015). A novel salt-tolerant chitobiosidase discovered by genetic screening of a metagenomic library derived from chitin-amended agricultural soil. Appl. Microbiol. Biotechnol. 99, 8199–8215. doi: 10.1007/s00253-015-6639-5
Cretoiu, M. S., Kielak, A. M., Schlüter, A., and van Elsas, J. D. (2014). Bacterial communities in chitin-amended soil as revealed by 16S rRNA gene based pyrosequencing. Soil Biol. Biochem. 76, 5–11. doi: 10.1016/j.soilbio.2014.04.027
Cretoiu, M. S., Korthals, G. W., Visser, J. H., and van Elsas, J. D. (2013). Chitin amendment increases soil suppressiveness toward plant pathogens and modulates the actinobacterial and oxalobacteraceal communities in an experimental agricultural field. Appl. Environ. Microbiol. 79, 5291–5301. doi: 10.1128/AEM.01361-13
De Boer, W., Klein Gunnewiek, P. J., Kowalchuk, G. A., and Van Veen, J. A. (2001). Growth of chitinolytic dune soil β-subclass Proteobacteria in response to invading fungal hyphae. Appl. Environ. Microbiol. 67, 3358–3362. doi: 10.1128/AEM.67.8.3358-3362.2001
De Souza, J. T., Weller, D. M., and Raaijmakers, J. M. (2003). Frequency, diversity, and activity of 2,4-diacetylphloroglucinol-producing fluorescent Pseudomonas spp. in Dutch take-all decline soils. Phytopathology 93, 54–63. doi: 10.1094/PHYTO.2003.93.1.54
Donn, S., Almario, J., Mullerc, D., Moenne-Loccoz, Y., Gupta, V. V. S. R., Kirkegaard, J. A., et al. (2014). Rhizosphere microbial communities associated with Rhizoctonia damage at the field and disease patch scale. Appl. Soil Ecol. 78, 37–47. doi: 10.1016/j.apsoil.2014.02.001
Duchene, O., Vian, J.-F., and Celette, F. (2017). Intercropping with legume for agroecological cropping systems: complementarity and facilitation processes and the importance of soil microorganisms. A review. Agric. Ecosyst. Environ. 240, 148–161. doi: 10.1016/j.agee.2017.02.019
Dunne, C., Crowley, J. J., Moenne-Loccoz, Y., Dowling, D. N., De Bruijn, F. J., and O’Gara, F. (1997). Biological control of Pythium ultimum by Stenotrophomonas maltophilia W81 is mediated by an extracellular proteolytic activity. Microbiology 143, 3921–3931. doi: 10.1099/00221287-143-12-3921
Eberlein, C., Heuer, H., Vidal, S., and Westphal, A. (2016). Microbial communities in Globodera pallida females raised in potato monoculture soil. Phytopathology 106, 581–590. doi: 10.1094/PHYTO-07-15-0180-R
Flood, J. (2010). The importance of plant health to food security. Food Sec. 2, 215–231. doi: 10.1007/s12571-010-0072-5
Fradkin, A., and Patrick, Z. (1985). Effect of matric potential, pH, temperature, and clay minerals on bacterial colonization of conidia of Cochliobolus sativus and on their survival in soil. Can. J. Plant Pathol. 7, 19–27. doi: 10.1080/07060668509501509
Garbeva, P., Postma, J., van Veen, J. A., and van Elsas, J. D. (2006). Effect of above-ground plant species on soil microbial community structure and its impact on suppression of Rhizoctonia solani AG3. Environ. Microbiol. 8, 233–246. doi: 10.1111/j.1462-2920.2005.00888.x
Garbeva, P., van Veen, J. A., and van Elsas, J. D. (2004). Microbial diversity in soil: selection microbial populations by plant and soil type and implications for disease suppressiveness. Annu. Rev. Phytopathol. 42, 243–270. doi: 10.1146/annurev.phyto.42.012604.135455
Gerlagh, M., Goossen-van de Geijn, H. M., Fokkema, N. J., and Vereijken, P. F. (1999). Long-term biosanitation by application of Coniothyrium minitans on Sclerotinia sclerotiorum-infected crops. Phytopathology 89, 141–147. doi: 10.1094/PHYTO.1999.89.2.141
Giné, A., Carrasquilla, M., Martínez-Alonso, M., Gaju, N., and Sorribas, F. J. (2016). Characterization of soil suppressiveness to root-knot nematodes in organic horticulture in plastic greenhouse. Front. Plant Sci. 7:164. doi: 10.3389/fpls.2016.00164
Großkopf, T., and Soyer, O. S. (2014). Synthetic microbial communities. Curr. Opin. Microbiol. 18, 72–77. doi: 10.1016/j.mib.2014.02.002
Haas, D., and Defago, G. (2005). Biological control of soil-borne pathogens by fluorescent Pseudomonads. Nat. Rev. Microbiol. 3, 307–319. doi: 10.1038/nrmicro1129
Hadar, Y., Harman, G. E., and Taylor, A. G. (1984). Evaluation of Trichoderma koningii and T. harzianum from New York soils for biological control of seed rot caused by Pythium spp. Phytopathology 74, 106–110. doi: 10.1094/Phyto-74-106
Haggag, W., and Timmusk, S. (2008). Colonization of peanut roots by biofilm-forming Paenibacillus polymyxa initiates biocontrol against crown rot disease. J. Appl. Microbiol. 104, 961–969. doi: 10.1111/j.1365-2672.2007.03611.x
Harman, G., Chet, I., and Baker, R. (1980). Trichoderma hamatum effects on seed and seedling disease induced in radish and pea by Pythium spp. or Rhizoctonia solani. Phytopathology 70, 1167–1171. doi: 10.1094/Phyto-70-1167
Henry, A. (1931a). Occurrence and sporulation of Helminthosporium sativum PKB in the soil. Can. J. Res. 5, 407–413. doi: 10.1139/cjr31-078
Henry, A. (1931b). The natural microflora of the soil in relation to the foot-rot problem of wheat. Can. J. Res. 4, 69–77. doi: 10.1139/cjr31-006
Hjort, K., Lembke, A., Speksnijder, A., Smalla, K., and Jansson, J. K. (2007). Community structure of actively growing bacterial populations in plant pathogen suppressive soil. Microb. Ecol. 53, 399–413. doi: 10.1007/s00248-006-9120-2
Hjort, K., Presti, I., Elväng, A., Marinelli, F., and Sjöling, S. (2014). Bacterial chitinase with phytopathogen control capacity from suppressive soil revealed by functional metagenomics. Appl. Microbiol. Biotechnol. 98, 2819–2828. doi: 10.1007/s00253-013-5287-x
Hol, W., Garbeva, P., Hordijk, C., Hundscheid, M. P. J., Klein Gunnewiek, P. J. A., van Agtmaal, M., et al. (2015). Non-random species loss in bacterial communities reduces antifungal volatile production. Ecology 96, 2042–2048. doi: 10.1890/14-2359.1
Howell, C., Beier, R. C., and Stipanovic, R. D. (1988). Production of ammonia by Enterobacter cloacae and its possible role in the biological control of Pythium preemergence damping-off by the bacterium. Phytopathology 78, 1075–1078. doi: 10.1094/Phyto-78-1075
Huang, X., Liu, J., Ding, J., He, Q., Xiong, R., and Zhang, K. (2009). The investigation of nematocidal activity in Stenotrophomonas maltophilia G2 and characterization of a novel virulence serine protease. Can. J. Microbiol. 55, 934–942. doi: 10.1139/w09-045
Jager, G., and Velvis, H. (1983). Suppression of Rhizoctonia solani in potato fields. II. Effect of origin and degree of infection with Rhizoctonia solani of seed potatoes on subsequent infestation and on formation of sclerotia. Eur. J. Plant Pathol. 89, 141–152. doi: 10.1016/j.syapm.2012.11.007
Jambhulkar, P. P., Sharma, M., Lakshman, D., and Sharma, P. (2015). “Natural mechanisms of soil suppressiveness against diseases caused by Fusarium, Rhizoctonia, Pythium, and Phytophthora,” in Organic Amendments and Soil Suppressiveness in Plant Disease Management, eds M. K. Meghvansi and A. Varma (Berlin: Springer), 95–123.
Ji, P., Koné, D., Yin, J., Jackson, K. L., and Csinos, A. S. (2012). Soil amendments with Brassica cover crops for management of Phytophthora blight on squash. Pest Manag. Sci. 68, 639–644. doi: 10.1002/ps.2308
Junaid, J. M., Dar, N. A., Bhat, T. A., Bhat, A. H., and Bhat, M. A. (2013). Commercial biocontrol agents and their mechanism of action in the management of plant pathogens. Int. J. Mod. Plant Anim. Sci. 1, 39–57. doi: 10.3109/07388551.2010.487258
Keinath, A. P., and Fravel, D. R. (1992). Induction of soil suppressiveness to Verticillium wilt of potato by successive croppings. Am. Potato J. 69, 503–513. doi: 10.1007/BF02853839
Kinkel, L. L., Bakker, M. G., and Schlatter, D. C. (2011). A coevolutionary framework for managing disease-suppressive soils. Annu. Rev. Phytopathol. 49, 47–67. doi: 10.1146/annurev-phyto-072910-095232
Kinkel, L. L., Schlatter, D. C., Bakker, M. G., and Arenz, B. E. (2012). Streptomyces competition and co-evolution in relation to plant disease suppression. Res. Microbiol. 163, 490–499. doi: 10.1016/j.resmic.2012.07.005
Kinkel, L. L., Schlatter, D. C., Xiao, K., and Baines, A. D. (2014). Sympatric inhibition and niche differentiation suggest alternative coevolutionary trajectories among Streptomycetes. ISME J. 8, 249–256. doi: 10.1038/ismej.2013.175
Klein, E., Ofek, M., Katan, J., Minz, D., and Gamliel, A. (2013). Soil suppressiveness to fusarium disease: shifts in root microbiome associated with reduction of pathogen root colonization. Phytopathology 103, 23–33. doi: 10.1094/phyto-12-11-0349
Kloepper, J. W., Leong, J., Teintze, M., and Schroth, M. N. (1980). Pseudomonas siderophores: a mechanism explaining disease-suppressive soils. Curr. Microbiol. 4, 317–320. doi: 10.1007/bf02602840
Kluepfel, D., McInnis, T. M., and Zehr, E. I. (1993). Involvement of root-colonizing bacteria in peach orchard soils suppressive of the nematode Criconemella xenoplax. Phytopathology 83, 1240–1240. doi: 10.1094/Phyto-83-1240
Ko, W., Hora, F. K., and Herlicska, E. (1974). Isolation and identification of a volatile fungistatic substance from alkaline soil [Ammonia]. Phytopathology 64, 1042–1043. doi: 10.1094/Phyto-64-1042
Kobayashi, N., and Komada, H. (1995). Screening of suppressive soils to Fusarium wilt from Kanto, Tozan and Tokai areas in Japan, and analysis of their suppressiveness. Soil Microorg. 45, 21–32.
Kyselková, M., Almario, J., Kopecký, J., Ságová-Marečková, M., Haurat, J., Muller, D., et al. (2014). Evaluation of rhizobacterial indicators of tobacco black root rot suppressiveness in farmers’ fields. Environ. Microbiol. Rep. 6, 346–353. doi: 10.1111/1758-2229.12131
Kyselková, M., Kopecký, J., Frapolli, M., Défago, G., Ságová-Marecková, M., Grundmann, G. L., et al. (2009). Comparison of rhizobacterial community composition in soil suppressive or conducive to tobacco black root rot disease. ISME J. 3, 1127–1138. doi: 10.1038/ismej.2009.61
Lapsansky, E. R., Milroy, A. M., Andales, M. J., and Vivanco, J. M. (2016). Soil memory as a potential mechanism for encouraging sustainable plant health and productivity. Curr. Opin. Biotechnol. 38, 137–142. doi: 10.1016/j.copbio.2016.01.014
Larkin, R. P. (2015). Soil health paradigms and implications for disease management. Annu. Rev. Phytopathol. 53, 199–221. doi: 10.1146/annurev-phyto-080614-120357
Larkin, R. P., and Fravel, D. R. (2002). Effects of varying environmental conditions on biological control of Fusarium wilt of tomato by nonpathogenic Fusarium spp. Phytopathology 92, 1160–1166. doi: 10.1094/PHYTO.2002.92.11.1160
Larkin, R. P., Hopkins, D. L., and Martin, F. N. (1996). Suppression of Fusarium wilt of watermelon by non-pathogenic Fusarium oxysporum and other microorganisms recovered from a disease-suppressive soil. Phytopathology 86, 812–819. doi: 10.1094/Phyto-86-812
Latz, E., Eisenhauer, N., Rall, B. C., Allan, E., Roscher, C., Scheu, S., et al. (2012). Plant diversity improves protection against soil-borne pathogens by fostering antagonistic bacterial communities. J. Ecol. 100, 597–604. doi: 10.1111/j.1365-2745.2011.01940.x
Laville, J., Voisard, C., Keel, C., Maurhofer, G., Défago, G., and Haas, D. (1992). Global control in Pseudomonas fluorescens mediating antibiotic synthesis and suppression of black root rot of tobacco. Proc. Natl. Acad. Sci. U.S.A. 89, 1562–1566. doi: 10.1073/pnas.89.5.1562
Lebeis, S. L., Paredes, S. H., Lundberg, D. S., Breakfield, N., Gehring, J., McDonald, M., et al. (2015). Salicylic acid modulates colonization of the root microbiome by specific bacterial taxa. Science 349, 860–864. doi: 10.1126/science.aaa8764
Lemanceau, P., and Alabouvette, C. (1991). Biological control of fusarium diseases by fluorescent Pseudomonas and non-pathogenic Fusarium. Crop Prot. 10, 279–286. doi: 10.1016/0261-2194(91)90006-D
Lemanceau, P., Alabouvette, C., and Couteaudier, Y. (1988). Recherches sur la résistance des sols aux maladies. XIV. Modification du niveau de réceptivité d’un sol résistant et d’un sol sensible aux fusarioses vasculaires en réponse à des apports de fer ou de glucose. Agronomie 8, 155–162. doi: 10.1051/agro:19880209
Lemanceau, P., Maurhofer, M., and Défago, G. (2006). “Contribution of studies on suppressive soils to the identification of bacterial biocontrol agents and to the knowledge of their modes of action,” in Plant-Associated Bacteria, ed. S. Gnanamanickam (Dordrecht: Springer), 231–267.
Li, G. Q., Huang, H. C., Miao, H. J., Erickson, R. S., Jiang, D. H., and Xiao, Y. N. (2006). Biological control of Sclerotinia diseases of rapeseed by aerial applications of the mycoparasite Coniothyrium minitans. Eur. J. Plant Pathol. 114, 345–355. doi: 10.1007/s10658-005-2232-6
Li, X., Zhang, Y., Ding, C., Jia, Z., He, Z., Zhang, T., et al. (2015). Declined soil suppressiveness to Fusarium oxysporum by rhizosphere microflora of cotton in soil sickness. Biol. Fertil. Soils 51, 935–946. doi: 10.1007/s00374-015-1038-8
Liu, D., Anderson, N. A., and Kinkel, L. L. (1996). Selection and characterization of strains of Streptomyces suppressive to the potato scab pathogen. Can. J. Microbiol. 42, 487–502. doi: 10.1139/m96-066
Liu, H., Tian, W., Li, B., Wu, G., Ibrahim, M., Tao, Z., et al. (2012). Antifungal effect and mechanism of chitosan against the rice sheath blight pathogen. Rhizoctonia solani. Biotechnol. Lett. 34, 2291–2298. doi: 10.1007/s10529-012-1035-z
Liu, S.-D., and Baker, R. (1980). Mechanism of biological control in soil suppressive to Rhizoctonia solani. Phytopathology 70, 404–412. doi: 10.1094/Phyto-70-404
Madi, L., and Katan, J. (1998). Penicillium janczewskii and its metabolites, applied to leaves, elicit systemic acquired resistance to stem rot caused by Rhizoctonia solani. Physiol. Mol. Plant Pathol. 53, 163–175. doi: 10.1006/pmpp.1998.0174
Martin, F., and Hancock, J. (1986). Association of chemical and biological factors in soils suppressive to Pythium ultimum. Phytopathology 76, 1221–1231. doi: 10.1094/Phyto-76-1221
Mazurier, S., Corberand, T., Lemanceau, P., and Raaijmakers, J. M. (2009). Phenazine antibiotics produced by fluorescent Pseudomonads contribute to natural soil suppressiveness to Fusarium wilt. ISME J. 3, 977–991. doi: 10.1038/ismej.2009.33
Mazzola, M. (2002). Mechanisms of natural soil suppressiveness to soilborne diseases. Antonie Van Leeuwenhoek 81, 557–564. doi: 10.1023/A:1020557523557
Mazzola, M., and Freilich, S. (2016). Prospects for biological soil-borne disease control: application of indigenous versus synthetic microbiomes. Phytopathology 107, 256–263. doi: 10.1094/PHYTO-09-16-0330-RVW
Mendes, R., Garbeva, P., and Raaijmakers, J. M. (2013). The rhizosphere microbiome: significance of plant beneficial, plant pathogenic, and human pathogenic microorganisms. FEMS Microbiol. Rev. 37, 634–663. doi: 10.1111/1574-6976.12028
Mendes, R., Kruijt, M., de Bruijn, I., Dekkers, E., van der Voort, M., and Schneider, J. H. M. (2011). Deciphering the rhizosphere microbiome for disease-suppressive bacteria. Science 332, 1097–1100. doi: 10.1126/science.1203980
Meng, Q., Yin, J. F., Rosenzweig, N., Douches, D., and Hao, J. J. (2012). Culture-based assessment of microbial communities in soil suppressive to potato common scab. Plant Dis. 96, 712–717. doi: 10.1094/PDIS-05-11-0441
Menzies, J. (1959). Occurrence and transfer of a biological factor in soil that suppresses potato scab. Phytopathology 49, 648–652.
Mghalu, M. J., Tsuji, T., Kubo, N., Kubota, M., and Hyakumachi, M. (2007). Selective accumulation of Trichoderma species in soils suppressive to radish damping-off disease after repeated inoculations with Rhizoctonia solani, binucleate Rhizoctonia and Sclerotium rolfsii. J. Gen. Plant Pathol. 73:250. doi: 10.1007/s10327-007-0016-x
Michelsen, C. F., and Stougaard, P. (2011). A novel antifungal Pseudomonas fluorescens isolated from potato soils in Greenland. Curr. Microbiol. 62, 1185–1192. doi: 10.1007/s00284-010-9846-4
Michelsen, C. F., Watrous, J., Glaring, M. A., Kersten, R., Koyama, N., Dorrestein, P. C., et al. (2015). Nonribosomal peptides, key biocontrol components for Pseudomonas fluorescens In5, isolated from a Greenlandic suppressive soil. mBio 6:e00079-15. doi: 10.1128/mBio.00079-15
Minerdi, D., Bossi, S., Gullino, M. L., and Garibaldi, A. (2009). Volatile organic compounds: a potential direct long-distance mechanism for antagonistic action of Fusarium oxysporum strain MSA 35. Environ. Microbiol. 11, 844–854. doi: 10.1111/j.1462-2920.2008.01805.x
Mueller, U. G., and Sachs, J. L. (2015). Engineering microbiomes to improve plant and animal health. Trends Microbiol. 23, 606–617. doi: 10.1016/j.tim.2015.07.009
Neeno-Eckwall, E. C., Kinkel, L. L., and Schottel, J. L. (2001). Competition and antibiosis in the biological control of potato scab. Can. J. Microbiol. 47, 332–340. doi: 10.1139/w01-010
Nel, B., Steinberg, C., Labuschagne, N., and Viljoen, A. (2006). The potential of nonpathogenic Fusarium oxysporum and other biological control organisms for suppressing Fusarium wilt of banana. Plant Pathol. 55, 217–223. doi: 10.1111/j.1365-3059.2006.01344.x
New, P., and Kerr, A. (1972). Biological control of crown gall: field measurements and glasshouse experiments. J. Appl. Microbiol. 35, 279–287. doi: 10.1111/j.1365-2672.1972.tb03699.x
Ofek, M., Voronov-Goldman, M., Hadar, Y., and Minz, D. (2014). Host signature effect on plant root-associated microbiomes revealed through analyses of resident vs. active communities. Environ. Microbiol. 16, 2157–2167. doi: 10.1111/1462-2920.12228
Ofek-Lalzar, M., Sela, N., Goldman-Voronov, M., Green, S. J., Hadar, Y., and Minz, D. (2014). Niche and host-associated functional signatures of the root surface microbiome. Nat. Commun. 5:4950. doi: 10.1038/ncomms5950
Olatinwo, R., Yin, B., Becker, J. O., and Borneman, J. (2006). Suppression of the plant-parasitic nematode Heterodera schachtii by the fungus Dactylella oviparasitica. Phytopathology 96, 111–114. doi: 10.1094/PHYTO-96-0111
Partridge, D., Sutton, T. B., Jordan, D. L., and Curtis, V. L. (2006). Management of Sclerotinia blight of peanut with the biological control agent Coniothyrium minitans. Plant Dis. 90, 957–963. doi: 10.1094/PD-90-0957
Penton, C. R., Gupta, V. V., Tiedje, J. M., Neate, S. M., Ophel-Keller, K., Gillings, M., et al. (2014). Fungal community structure in disease suppressive soils assessed by 28S LSU gene sequencing. PLOS ONE 9:e93893. doi: 10.1371/journal.pone.0093893
Perneel, M., Heyrman, J., Adiobo, A., De Maeyer, K., Raaijmakers, J. M., and De Vos, P. (2007). Characterization of CMR5c and CMR12a, novel fluorescent Pseudomonas strains from the cocoyam rhizosphere with biocontrol activity. J. Appl. Microbiol. 103, 1007–1020. doi: 10.1111/j.1365-2672.2007.03345.x
Persson, L., and Olsson, S. (2000). Abiotic characteristics of soils suppressive to Aphanomyces root rot. Soil Biol. Biochem. 32, 1141–1150. doi: 10.1016/S0038-0717(00)00030-4
Postma, J., Scheper, R. W. A., and Schilder, M. T. (2010). Effect of successive cauliflower plantings and Rhizoctonia solani AG 2-1 inoculations on disease suppressiveness of a suppressive and a conducive soil. Soil Biol. Biochem. 42, 804–812. doi: 10.1016/j.soilbio.2010.01.017
Postma, J., and Schilder, M. T. (2015). Enhancement of soil suppressiveness against Rhizoctonia solani in sugar beet by organic amendments. Appl. Soil Ecol. 94, 72–79. doi: 10.1016/j.apsoil.2015.05.002
Poudel, R., Jumpponen, A., Schlatter, D. C., Paulitz, T. C., Gardener, B. B., Kinkel, L. L., et al. (2016). Microbiome networks: a systems framework for identifying candidate microbial assemblages for disease management. Phytopathology 106, 1083–1096. doi: 10.1094/PHYTO-02-16-0058-FI
Pyrowolakis, A., Westphal, A., Sikora, R. A., and Beckerb, J. O. (2002). Identification of root-knot nematode suppressive soils. Appl. Soil Ecol. 19, 51–56. doi: 10.1016/S0929-1393(01)00170-6
Raaijmakers, J. M., and Mazzola, M. (2012). Diversity and natural functions of antibiotics produced by beneficial and plant pathogenic bacteria. Annu. Rev. Phytopathol. 50, 403–424. doi: 10.1146/annurev-phyto-081211-172908
Raaijmakers, J. M., and Mazzola, M. (2016). Soil immune responses. Science 352, 1392–1393. doi: 10.1126/science.aaf3252
Raaijmakers, J. M., Paulitz, T. C., Steinberg, C., Alabouvette, C., and Moënne-Loccoz, Y. (2009). The rhizosphere: a playground and battlefield for soilborne pathogens and beneficial microorganisms. Plant Soil 321, 341–361. doi: 10.1007/s11104-008-9568-6
Raaijmakers, J. M., and Weller, D. M. (1998). Natural plant protection by 2, 4-diacetylphloroglucinol-producing Pseudomonas spp. in take-all decline soils. Mol. Plant Microbe Interact. 11, 144–152. doi: 10.1094/MPMI.1998.11.2.144
Radajewski, S., Ineson, P., Parekh, N. R., and Murrell, J. C. (2000). Stable-isotope probing as a tool in microbial ecology. Nature 403, 646–649. doi: 10.1038/35001054
Rey, P., Le Floch, G., Benhamou, N., Salerno, M. I., Thuillier, E., and Tirilly, Y. (2005). Interactions between the mycoparasite Pythium oligandrum and two types of sclerotia of plant-pathogenic fungi. Mycol. Res. 109, 779–788. doi: 10.1017/S0953756205003059
Rodríguez, M. A., Rothen, C., Lo, T. E., Cabrera, G. M., and Godeas, A. M. (2015). Suppressive soil against Sclerotinia sclerotiorum as a source of potential biocontrol agents: selection and evaluation of Clonostachys rosea BAFC1646. Biocontrol Sci. Technol. 25, 1388–1409. doi: 10.1080/09583157.2015.1052372
Rosenzweig, N., Tiedje, J. M., Quensen, J. F., Meng, Q., and Hao, J. J. (2012). Microbial communities associated with potato common scab-suppressive soil determined by pyrosequencing analyses. Plant Dis. 96, 718–725. doi: 10.1094/PDIS-07-11-0571
Sanguin, H., Sarniguet, A., Gazengel, K., Moënne-Loccoz, Y., and Grundmann, G. L. (2009). Rhizosphere bacterial communities associated with disease suppressiveness stages of take-all decline in wheat monoculture. New Phytol. 184, 694–707. doi: 10.1111/j.1469-8137.2009.03010.x
Scher, F. M., and Baker, R. (1980). Mechanism of biological control in a Fusarium-suppressive soil. Phytopathology 70, 412–417. doi: 10.1094/Phyto-70-412
Scher, F. M., and Baker, R. (1982). Effect of Pseudomonas putida and a synthetic iron chelator on induction of soil suppressiveness to Fusarium wilt pathogens. Phytopathology 72, 1567–1573. doi: 10.1094/Phyto-72-1567
Schillinger, W. F., and Paulitz, T. (2014). Natural suppression of Rhizoctonia bare patch in a long-term no-till cropping systems experiment. Plant Dis. 98, 389–394. doi: 10.1094/PDIS-04-13-0420-RE
Schisler, D., and Slininger, P. (1994). Selection and performance of bacterial strains for biologically controlling Fusarium dry rot of potatoes incited by Gibberella pulicaris. Plant Dis. 78, 251–255. doi: 10.1094/PD-78-0251
Schreiner, K., Hagn, A., Kyselková, M., Moënne-Loccoz, Y., Welzl, G., Munch, J. C., et al. (2010). Comparison of barley succession and take-all disease as environmental factors shaping the rhizobacterial community during take-all decline. Appl. Environ. Microbiol. 76, 4703–4712. doi: 10.1128/AEM.00481-10
Shen, Z., Ruan, Y., Chao, X., Zhang, J., Li, R., and Shen, Q. (2015). Rhizosphere microbial community manipulated by 2 years of consecutive biofertilizer application associated with banana Fusarium wilt disease suppression. Biol. Fertil. Soils 51, 553–562. doi: 10.1007/s00374-015-1002-7
Shiomi, Y., Nishiyama, M., Onizuka, T., and Marumoto, T. (1999). Comparison of bacterial community structures in the rhizoplane of tomato plants grown in soils suppressive and conducive towards bacterial wilt. Appl. Environ. Microbiol. 65, 3996–4001.
Siddiqui, S., Siddiqui, Z. A., and Ahmad, I. (2005). Evaluation of fluorescent Pseudomonads and Bacillus isolates for the biocontrol of a wilt disease complex of pigeonpea. World J. Microbiol. Biotechnol. 21, 729–732. doi: 10.1007/s11274-004-4799-z
Siddiqui, Z. (2007). Biocontrol of Alternaria triticina by plant growth promoting rhizobacteria on wheat. Arch. Phytopathol. Plant Prot. 40, 301–308. doi: 10.1080/03235400600587391
Smith, V. L., Wilcox, W. F., and Harman, G. E. (1990). Potential for biological control of Phytophthora root and crown rots of apple by Trichoderma and Gliocladium spp. Phytopathology 80, 880–885. doi: 10.1094/Phyto-80-880
Sneh, B., Dupler, M., Elad, Y., and Basker, R. (1984). Chlamydospore germination of Fusarium oxysporum f. sp. cucumerinum as affected by fluorescent and lytic bacteria from a Fusarium-suppressive soil. Phytopathology 74, 1115–1124. doi: 10.1094/Phyto-74-1115
Sneh, B., Pozniak, D., and Salomon, D. (1987). Soil suppressiveness to Fusarium wilt of melon, induced by repeated croppings of resistant varieties of melons. J. Phytopathol. 120, 347–354. doi: 10.1111/j.1439-0434.1987.tb00498.x
Song, J., Li, S., Xu, Y., Wei, W., Yao, Q., and Panet, F. (2016). Diversity of parasitic fungi from soybean cyst nematode associated with long-term continuous cropping of soybean in black soil. Acta Agric. Scand. B Soil Plant Sci. 66, 432–442.
Stirling, G., Smith, M. K., Smith, J. P., Stirling, A. M., and Hamill, S. D. (2012). Organic inputs, tillage and rotation practices influence soil health and suppressiveness to soilborne pests and pathogens of ginger. Australas. Plant Pathol. 41, 99–112. doi: 10.1007/s13313-011-0096-0
Thomashow, L., and Pierson, L. (1991). “Genetic aspects of phenazine antibiotic production by fluorescent Pseudomonads that suppress take-all disease of wheat,” in Advances in Molecular Genetics of Plant-Microbe Interactions, Vol. 1, eds M. J. Daniels, J. Allan Downie, and A. E. Osbourn (Dordrecht: Springer), 443–449.
Tkacz, A., Cheema, J., Chandra, G., Grant, A., and Poole, P. S. (2015). Stability and succession of the rhizosphere microbiota depends upon plant type and soil composition. ISME J. 9, 2349–2359. doi: 10.1038/ismej.2015.41
Tomihama, T., Nishi, Y., Mori, K., Shirao, T., Iida, T., Uzuhashi, S., et al. (2016). Rice bran amendment suppresses potato common scab by increasing antagonistic bacterial community levels in the rhizosphere. Phytopathology 106, 719–728. doi: 10.1094/PHYTO-12-15-0322-R
Toyota, K., and Kimura, M. (1993). Colonization of chlamydospores of Fusarium oxysporum f. sp. raphani by soil bacteria and their effects on germination. Soil Biol. Biochem. 25, 193–197. doi: 10.1016/0038-0717(93)90026-8
Trivedi, P., Delgado-Baquerizobc, M., Trivedi, C., Hamonts, K., Anderson, I. C., Singh, B. K., et al. (2017). Keystone microbial taxa regulate the invasion of a fungal pathogen in agro-ecosystems. Soil Biol. Biochem. 111, 10–14. doi: 10.1016/j.soilbio.2017.03.013
van der Voort, M., Kempenaar, M., van Driel, M., Raaijmakers, J. M., and Mendes, R. (2016). Impact of soil heat on reassembly of bacterial communities in the rhizosphere microbiome and plant disease suppression. Ecol. Lett. 19, 375–382. doi: 10.1111/ele.12567
van den Boogert, P. H., Reinartz, H., Sjollema, K. A., and Veenhuis, M. (1989). Microscopic observations on the interaction of the mycoparasite Verticillium biguttatum with Rhizoctonia solani and other soil-borne fungi. Antonie Van Leeuwenhoek 56, 161–174. doi: 10.1007/BF00399979
Van Elsas, J. D., Costa, R., Jansson, J., Sjöling, S., Bailey, M., Nalin, R., et al. (2008). The metagenomics of disease-suppressive soils–experiences from the METACONTROL project. Trends Biotechnol. 26, 591–601. doi: 10.1016/j.tibtech.2008.07.004
Velvis, H., and Jager, G. (1983). Biological control of Rhizoctonia solani on potatoes by antagonists. 1. Preliminary experiments with Verticillium biguttatum, a sclerotium-inhabiting fungus. Eur. J. Plant Pathol. 89, 113–123. doi: 10.1007/BF01976350
Vitullo, D., Altieri, R., Esposito, A., Nigro, F., Ferrara, M., Alfano, G., et al. (2013). Suppressive biomasses and antagonist bacteria for an eco-compatible control of Verticillium dahliae on nursery-grown olive plants. Int. J. Environ. Sci. Technol. 10, 209–220. doi: 10.1007/s13762-012-0145-4
Voisard, C., Keel, C., Haas, D., and Dèfago, G. (1989). Cyanide production by Pseudomonas fluorescens helps suppress black root rot of tobacco under gnotobiotic conditions. EMBO J. 8, 351–358.
Watrous, J., Roach, P., Alexandrov, T., Heath, B. S., Yang, J. Y., Kersten, R. D., et al. (2012). Mass spectral molecular networking of living microbial colonies. Proc. Natl. Acad. Sci. U.S.A. 109, E1743–E1752. doi: 10.1073/pnas.1203689109
Weinhold, A. R., Oswald, J. W., Bowman, T., Bishop, J., and Wrightet, D. (1964). Influence of green manures and crop rotation on common scab of potato. Am. Potato J. 41, 265–273. doi: 10.1007/bf02854863
Weller, D. M., Raaijmakers, J. M., Gardener, B. B., and Thomashow, L. S. (2002). Microbial populations responsible for specific soil suppressiveness to plant pathogens. Annu. Rev. Phytopathol. 40, 309–348. doi: 10.1146/annurev.phyto.40.030402.110010
Whipps, J., Sreenivasaprasad, S., Muthumeenakshi, S., Rogers, C. W., and Challen, M. P. (2008). Use of Coniothyrium minitans as a biocontrol agent and some molecular aspects of sclerotial mycoparasitism. Eur. J. Plant Pathol. 121, 323–330. doi: 10.1007/s10658-007-9238-1
Wijetunga, C., and Baker, R. (1979). Modeling of phenomena associated with soil suppressive to Rhizoctonia solani. Phytopathology 69, 1287–1293. doi: 10.1094/Phyto-69-1287
Wiseman, B. M., Neate, S. M., Ophel Keller, K., and Smith, S. E. (1996). Suppression of Rhizoctonia solani anastomosis group 8 in Australia and its biological nature. Soil Biol. Biochem. 28, 727–732. doi: 10.1016/0038-0717(95)00178-6
Wong, P., and Baker, R. (1984). Suppression of wheat take-all and Ophiobolus patch by fluorescent Pseudomonads from a Fusarium-suppressive soil. Soil Biol. Biochem. 16, 397–403. doi: 10.1016/0038-0717(84)90040-3
Workneh, F., and Van Bruggen, A. (1994). Microbial density, composition, and diversity in organically and conventionally managed rhizosphere soil in relation to suppression of corky root of tomatoes. Appl. Soil Ecol. 1, 219–230. doi: 10.1016/0929-1393(94)90013-2
Xiong, W., Rong, L., Ren, Y., and Shen, Q. (2017). Distinct roles for soil fungal and bacterial communities associated with the suppression of vanilla Fusarium wilt disease. Soil Biol. Biochem. 107, 198–207. doi: 10.1016/j.soilbio.2017.01.010
Xiong, W., Zhao, Q., Zhao, J., Xun, W., Li, R., Zhang, R., et al. (2015). Different continuous cropping spans significantly affect microbial community membership and structure in a vanilla-grown soil as revealed by deep pyrosequencing. Microb. Ecol. 70, 209–218. doi: 10.1007/s00248-014-0516-0
Xue, C., Ryan Penton, C., Shen, Z., Zhang, R., Huang, Q., Li, R., et al. (2015). Manipulating the banana rhizosphere microbiome for biological control of Panama disease. Sci. Rep. 5:11124. doi: 10.1038/srep11124
Yang, J. I., Loffredo, A., Borneman, J., and Becker, J. O. (2012). Biocontrol efficacy among strains of Pochonia chlamydosporia obtained from a root-knot nematode suppressive soil. J. Nematol. 44, 67–71.
Yin, C., Hulbert, S. H., Schroeder, K. L., Mavrodi, O., Mavrodi, D., Dhingra, A., et al. (2013). Role of bacterial communities in the natural suppression of Rhizoctonia solani bare patch disease of wheat (Triticum aestivum L.). Appl. Environ. Microbiol. 79, 7428–7438. doi: 10.1128/AEM.01610-13
Yuen, G., and Schroth, M. (1986). Inhibition of Fusarium oxysporum f. sp. dianthi by iron competition with an Alcaligenes sp. Phytopathology 76, 171–176. doi: 10.1094/Phyto-76-171
Keywords: disease suppressive soil, omics technologies, rhizosphere microbiome, antagonism by rhizobacteria, pathogen suppression
Citation: Gómez Expósito R, de Bruijn I, Postma J and Raaijmakers JM (2017) Current Insights into the Role of Rhizosphere Bacteria in Disease Suppressive Soils. Front. Microbiol. 8:2529. doi: 10.3389/fmicb.2017.02529
Received: 27 August 2017; Accepted: 05 December 2017;
Published: 18 December 2017.
Edited by:
Jan Dirk Van Elsas, University of Groningen, NetherlandsReviewed by:
Fernando Dini Andreote, University of São Paulo, BrazilBrajesh Singh, Western Sydney University, Australia
Copyright © 2017 Gómez Expósito, de Bruijn, Postma and Raaijmakers. This is an open-access article distributed under the terms of the Creative Commons Attribution License (CC BY). The use, distribution or reproduction in other forums is permitted, provided the original author(s) or licensor are credited and that the original publication in this journal is cited, in accordance with accepted academic practice. No use, distribution or reproduction is permitted which does not comply with these terms.
*Correspondence: Jos M. Raaijmakers, ai5yYWFpam1ha2Vyc0BuaW9vLmtuYXcubmw=