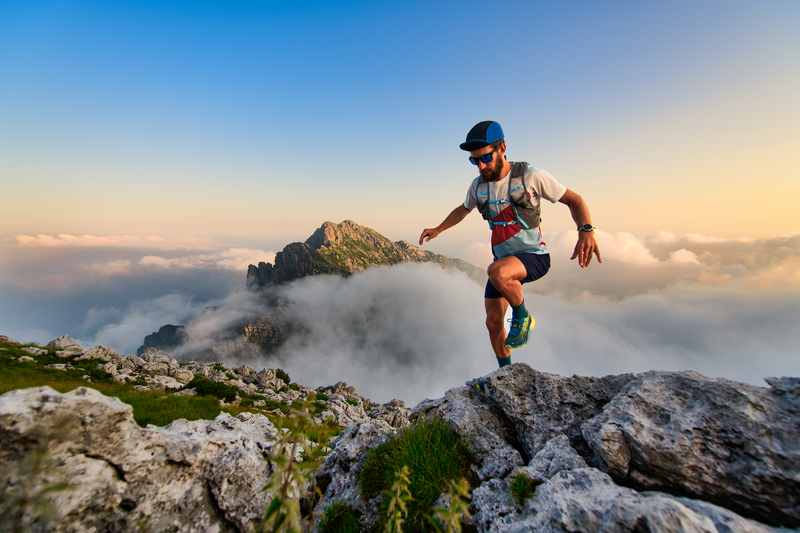
94% of researchers rate our articles as excellent or good
Learn more about the work of our research integrity team to safeguard the quality of each article we publish.
Find out more
MINI REVIEW article
Front. Microbiol. , 21 November 2017
Sec. Microbial Immunology
Volume 8 - 2017 | https://doi.org/10.3389/fmicb.2017.02309
This article is part of the Research Topic Shaping of Human Immune System and Metabolic Processes by Viruses and Microorganisms View all 64 articles
A very substantial progress has been made in our understanding of infectious diseases caused by invasive bacteria. Under their planktonic forms, bacteria transiently reside in the otherwise sterile mammal body tissues, as the physiological inflammation insures both their clearance and repair of any tissue damage. Yet, the bacteria prone to experience planktonic to biofilm developmental transition still need to be studied. Of note, sessile bacteria not only persist but also concur preventing the effectors and regulators of the physiological inflammation to operate. Thus, it is urgent to design biologically sound experimental approaches aimed to extract, at the earliest stage, immune signatures of mono-bacteria planktonic to biofilm developmental transition in vivo and ex vivo. Indeed, the transition is often the first event to which succeeds the “chronicization” process whereby classical bacteria-targeting therapies are no more efficacious. An in vivo model of micro-injection of Staphylococcus aureus planktonic or biofilm cells in the ear pinna dermis of laboratory transgenic mice with fluorescent immune cells is proposed. It allows visualizing, in real time, the range of the early interactions between the S. aureus and myeloid cell subsets- the resident macrophages and dendritic cells, the recruited neutrophil granulocytes/polymorphonuclear neutrophils, monocytes otherwise known to differentiate as macrophages or dendritic cells. One main objective is to extract contrasting immune signatures of the modulation of the physiological inflammation with respect to the two bacterial lifestyles.
Most invasive bacteria display two different lifestyles: whereas the free-floating planktonic bacteria’ life style dominates, in some clinical settings, bacteria sensing hostile conditions adhere to biotic or abiotic surfaces and form biofilms (Moormeier and Bayles, 2017). The planktonic to biofilm/sessile lifestyle transition is associated with important metabolic changes and self-production of proteins-lipids-exopolysaccharides-rich as well as extracellular DNA-containing extracellular matrix (Costerton et al., 1999).
According to the National Institutes of Health, biofilms have an enormous impact on human medicine, accounting for over 80% of infectious processes in otherwise sterile tissue (s). Whereas the physiological inflammation is able to both clear invasive planktonic bacteria and to repair tissue damages insuring the return to tissue structural and functional homeostasis, this physiological inflammation does not operate in tissues experiencing sustained colonization by bacterial biofilms. Moreover, contrasting with planktonic bacteria that are cleared by commonly used antibiotics, provided that they do not harbor genetic resistance determinants, the majority of bacteria within the biofilms are resistant to these antibiotics (Lebeaux et al., 2014).
In this review, our present understanding of the professional phagocyte sensors of microbial agonists expected to operate over the in vivo planktonic to biofilm lifestyle switch is briefly introduced. Transiently invasive planktonic bacteria are usually cleared by myeloid cells of either the neutrophil granulocyte lineage or/and by mononuclear phagocytes. However, the bilateral interactions engaged or not between sessile bacteria and the myeloid cells are still poorly studied. Until now, most experimentalists have conducted in vitro studies with sessile bacteria, exposing them to either one or the other myeloid cell lineage or both (Watters et al., 2016). This review focuses on recent developments obtained in rodent models to characterize inflammatory responses against Staphylococcus aureus or Pseudomonas aeruginosa sessile bacteria. A new experimental approach combining the mouse ear pinna model and the intravital imaging approach is proposed to analyze these innate immune responses at the dynamic level.
The in vivo developmental transition from planktonic to sessile bacteria reflects a range of bilateral cross talks in the fluctuating dynamic tissue milieu colonized by the bacteria under study. At the earliest stage of this developmental transition, communications between key bacterial messengers as well as interactions between bacterial agonists and sensors displayed by the resident and recruited myeloid cells such as the professional phagocytes are initiated and renewed.
The cyclic dinucleotides (c-di-NMPs) are recognized as Microbial Associated Molecular Patterns/MAMPs and induce a host type I interferon immune response prolonged by IFNγ production (Valle et al., 2013; Snyder et al., 2017). Moreover, the c-di-NMPs play a central role in many bacterial species during the lifestyle transition (Valle et al., 2013). Using the P. aeruginosa model organism, Valentini and Filloux (2016) showed that the bacteria use c-di-GMP as a checkpoint during the different steps of biofilm development. There is indeed a direct correlation between high levels of c-di-GMP in the bacteria and biofilm formation, and between low levels of c-di-GMP and motility (planktonic phenotype). The c-di-GMP second messenger is used by Escherichia coli and Salmonella enterica serovar Typhimurium over their planktonic to biofilm developmental transition (Allewell, 2016; Valentini and Filloux, 2016) whereas the c-di-AMP is used as a second messenger by other bacteria such as S. aureus (Corrigan et al., 2011).
The Guanosine tetraphosphate (ppGpp) and pentaphosphate (pppGpp), also called (p)ppGpp or alarmones are synthetized when bacteria are exposed to cells such as phagocytes in the infected tissue. Considered as bacterial signature of a so called stringent response, these alarmones represent intracellular signaling molecules known to participate to intracellular bacteria survival: Geiger et al. (2012) demonstrated that the stringent response is induced, in vitro, after S. aureus phagocytosis by neutrophil granulocytes or polymorphonuclears (PMN). The rapid (p)ppGpp synthesis leads to increased psm transcription and to participation of synthetized phenol soluble modulins (PSMs) concurring to bacteria survival after phagocytosis (Geiger et al., 2012). Depicted as pro-inflammatory agents, the PSMs also account for the bacteria escape from the transient intracellular niche, followed either by bacteria survival inside the cytosol or by lysis of the cell, all these rapid processes contributing to damages of the S. aureus- hosting tissues (Geiger et al., 2012; Peschel and Otto, 2013).
Quorum sensing (QS) is a cell-to-cell signaling process that allows bacteria to sense and process high cell densities. It involves the synthesis, release and accumulation of signaling molecules called auto-inducers (AIs) (Papenfort and Bassler, 2016). At high concentrations, AIs induce cellular signaling cascades that notably control biofilm formation. The QS system of S. aureus called accessory gene regulator (Agr) has been extensively studied (Paharik and Horswill, 2016). With other regulators, it constitutes a complex regulatory network that, at any moment, either modifies AGR activity itself, or its downstream signaling or metabolic pathways. At the early stage of S. aureus developmental transition from planktonic to biofilm lifestyle, the low Agr concentration allows the production of inter-bacteria/intercellular adhesins whereas toxins’ expression is repressed (Balasubramanian et al., 2016). Later, high levels of Agr induce biofilm structuration and dispersion by up-regulating the expression of the pro-inflammatory PSM molecules (Otto, 2008; Periasamy et al., 2012; Kavanaugh and Horswill, 2016; Paharik and Horswill, 2016). Environmental factors also modulate the Agr function in S. aureus, with a well-described inhibitory effect of the reactive oxygen species (ROS) produced by innate immune cells (Kavanaugh and Horswill, 2016).
Among other regulators that interact with the agr system at later stage of the biofilm development and maturation, the S. aureus exoprotein (Sae) two-component system promotes the synthesis and secretion of leucocidins and other virulence determinants that actively participate to S. aureus survival after contact with PMN in vitro (Paharik and Horswill, 2016). Sae mutants have indeed a decreased capacity to resist to the PMN- clearing functions after contact (Yarwood and Schlievert, 2003; Voyich et al., 2009; Paharik and Horswill, 2016).
In rodent laboratory models, the most rapidly recruited cells are phagocytes, namely the PMN. Over any local disruption of mouse tissue homeostasis, PMN stored in the bone marrow egress into the blood vascular bed. Through chemoattractants and cytokines produced by tissue resident mast cells and macrophages (Teng et al., 2017), the PMN rapidly cross the microvessels endothelial cells, reaching the extracellular compartment colonized by invasive bacteria. They represent the first wave of innate immune cells to be recruited from the blood circulation. Most often, PMN efficiently kill and degrade invasive planktonic bacteria by using different antimicrobial strategies: phagocytosis, production of ROS and antimicrobial peptides, as well as cytotoxic components released from their subcellular distinct granules. More recently, neutrophil extracellular traps (NETs) have been observed and included as an additional strategy (Brinkmann et al., 2004). Among the in vivo models that allow characterization of the bilateral interactions co-engaged by PMN and sessile bacteria, we selected those relying on either P. aeruginosa or S. aureus (Table 1).
Of note, the features of the biofilm-colonized devices condition the interaction profiles: whereas in devices of hollow type (catheter, silicon splint) bacterial biofilms are protected from the immune cells, on solid devices (K-wire, pin), the bacterial biofilms are directly exposed to the different waves of immune cells. Depending on the model used, many other parameters operate (listed in Table 1). In most models, an intense and rapid accumulation of PMN is observed at the proximity of the biofilms (Wagner et al., 2003; Prabhakara et al., 2011a; Torre et al., 2015; Moser et al., 2017; Wang et al., 2017), the PMN representing the most abundant population of recruited cells. Using a non- invasive bioluminescence-based approach, Bernthal et al. (2011) showed that this recruitment is IL-1β dependent, as a 50% decrease in PMNs numbers was observed in the bacteria colonized knee joints of IL-1β deficient mice, as compared to wild-type mice. This PMN infiltration is increased when diabetic mice are treated with insulin in a bacteria-hosting wound, the latter incorporating actin and DNA from lysed PMN, which therefore contributes to the building of biofilms (Watters et al., 2014). Of note, a low PMN recruitment in the target tissues (Thurlow et al., 2011; Scherr et al., 2014; Secor et al., 2017) can be also operating: in particular was noticed an association between the reduced PMN recruitment and the production of Filamentous Pf1-like bacteriophage (Pf phage) by P. aeruginosa (Secor et al., 2017). Taken globally, whatever the experimental conditions depicted in Table 1, there is a need to capture more comprehensive information documenting, at least at the earliest stages, the in vivo dynamic interactions between bacteria and PMN.
Could mature biofilms’ matrix components protect bacteria from activated PMN? PMN were indeed shown to be activated, in vitro, by bacterial DNA and polysaccharides components such as alginate, the measured outcome being an increase of their respiratory burst (Pedersen et al., 1990; Alvarez et al., 2006; Fuxman Bass et al., 2008; Jensen et al., 2010). In vivo, P. aeruginosa biofilm interactions with PMN lead to up regulation of the QS-dependent effectors such as rhamnolipids which cause PMN lysis (Alhede et al., 2014). This shielding specific property of rhamnolipids is described in mouse models relying upon the intraperitoneal implant of pre-colonized silicone device (Van Gennip et al., 2012) or on biofilm development in the respiratory tract (Bjarnsholt et al., 2005; Jakobsen et al., 2012). In these models, QS mutants are unable to produce rhamnolipids and are rapidly phagocytosed and cleared by PMN.
Monocytes/macrophages are other key actors recruited and sensing both the other inflammatory cells -e.g., PMN -as well as bacteria agonists. Under homeostatic conditions, circulating macrophage/monocytes qualified as classical/intermediate ones are continuously recruited from the blood and either mature into macrophages or remain as monocytes within tissues (Sprangers et al., 2016; Jakubzick et al., 2017). In the skin, long- lived resident macrophages are also present and maintain their population by self-renewal (Sprangers et al., 2016; Jakubzick et al., 2017). As for PMN, microbe-specific molecules participate to the rapid emigration of classical/intermediate monocytes in the extravascular space which contribute to the resolution of inflammation by recognizing and phagocytosing bacteria and dying cells, and by producing ROS and reactive nitrogen species (RNI) (Sprangers et al., 2016; Jakubzick et al., 2017; Kashem et al., 2017). Once PMNs experience apoptotic death, a second wave of monocytes qualified as non- classical monocytes contribute to the resolution of inflammation and repair of the disrupted tissue (Sprangers et al., 2016).
Only a few studies (Prabhakara et al., 2011a; Thurlow et al., 2011; Hanke et al., 2012, 2013; Scherr et al., 2015; Corrado et al., 2016; Silva-Santana et al., 2016; see Table 1) assess the complex recruitment waves and networks of both PMN and monocyte subsets. Of note, an early wave of monocyte recruitment is sometimes predominant, as compared to PMN recruitment (Thurlow et al., 2011). Two main studies were conducted to monitor the abundance and functional features of monocyte/macrophage recruitment in vivo at specific time points after contact with biofilms. The biofilms’ matrix components clearly modulate the functional properties of recruited cells. In a S. aureus pre-colonized catheter-based model, mobilized cells are mainly distant from the catheter and a non-significant proportion of F4/80+ macrophages are able to invade the biofilms. Cells present deeply into the biofilm rapidly die, therefore preventing phagocytosis of biofilm bacteria to be otherwise exerted by macrophages (Thurlow et al., 2011). A significant reduction of pro-inflammatory cytokines (IL-1β, TNF…) and chemokines (CXCL2, CCL2) production at the boundary of the biofilm- colonized tissue is also observed, associated with a reduced Nitric Oxide synthase (iNOS) induction and an increased arginase-1 expression. The authors conclude to a macrophage polarization toward a counter-inflammatory activated M2 phenotype and to fibrosis with MyD88- signaling as a major effector pathway regulating these two phenomena (Thurlow et al., 2011; Hanke and Kielian, 2012; Hanke et al., 2012). In a lung experiencing colonization with phage Pf- producing P. aeruginosa, Secor et al. (2017) also documented a M2 polarization profile. Taken globally, it appears that the macrophage M2 polarization and fibrosis do concur prolonging bacteria biofilm persistence, although the universal character of such responses remains to be assessed.
Intra-vital microscopy is increasingly used in different biomedical research fields to study dynamic processes at the cellular level in their specific tissue environment. Compared to classical methods such as ex vivo histology or flow cytometry, intra-vital confocal microscopy live imaging allows dynamic interactions to be captured once fluorescent reporters are expressed by both the microbes and the laboratory mouse cell lineages with which are engaged, more or less durably, dynamic interactions.
Several skin-related models were described in the literature to study the immunobiology of biofilm infections by using classical approaches (Table 1). In these models, the biofilm-loaded cutaneous sites were the back or the flank of animals, which represent unsuitable sites for intravital microscopy of cutaneous innate immune responses.
The ear pinnae is one of the most studied appendage in which are delivered microorganisms, enabling the observation of the early and either transient or prolonged dynamic interactions with resident or recruited myeloid cells (Amino et al., 2006, 2007; Peters et al., 2008; Ng et al., 2011; Sumaria et al., 2011; Jain and Weninger, 2013; Tavares et al., 2013; Carneiro et al., 2017). As an imaging site, the ear presents several technical advantages such as the accessibility of the tissue, easy and fast protocols of preparation to perform imaging experiments and obtain reproducible results, and the possibility of imaging for long periods of time in blocks of 20–40 min (Li et al., 2012).
The mouse ear pinna appendage harbors a thin epidermis and an underlying dermis, respectively avascularized and highly vascularized, which contain a broad range of lympho-myeloid cells (Jain and Weninger, 2013). Using a multiphoton microscopy approach and quantitative flow cytometry, Tong et al. (2015) elaborated a 3D immune cell atlas of mouse skin and compared the ear pinnae, dorsal back, footpad, and tail skin. Langerhans cells and dendritic epidermal T cells are present in the epidermis, whereas the dermis mainly harbors in the upper dermis myeloid cells such as dendritic cells, mast cells as well as lymphoid cells (αβT cells, γδT cells, and group 2 Innate Lymphoid Cells), and mainly resident macrophages in the deeper dermis (Tay et al., 2014; Tong et al., 2015). All these dermis-located immune cells are included in a collagen- and elastin- rich and more or less hyaluronan- rich extracellular matrix. In term of cell numbers, the specificities of the ear pinna cutaneous site are the following ones: the total leukocyte density is high, with around 4800 cells per mm2 and a majority of cells present in the dermis. Macrophages, dermal dendritic cells and mast cells represent the majority of ear dermal leukocytes with respectively around 6000 macrophages per mm3 and more than 2000 dermal dendritic cells and mast cells per mm3 (Tong et al., 2015). Of note, the ear pinna dermis presents two specificities regarding the presence of mast cells: their high prevalence and their perivascular localization, in close association to blood vessels, in contrast to dermal dendritic cells (Tong et al., 2015). By using intravital multi-photon microscopy, Ng et al. (2011) showed that PMNs are present in the ear dermis of naive mice, but in small numbers, and patrol, as do dermal dendritic cells. Both cell lineages are likely surveying the presence of either epidermis-restricted microbiota derived agonists that reach the dermis or endogenous agonists through the sensors displayed at the plasma membrane or within the macropinocytosis/endosomal machinery.
By combining intra-vital confocal microscopy approach and the mouse ear pinna infection model, inflammatory responses against biofilms could be analyzed for the first time at the dynamic level in the tissue environment. The Figure 1 shows the schematic workflow of methods proposed to characterize and compare the innate immune responses against S. aureus planktonic and sessile bacteria. Transgenic mice with fluorescent immune cells visible in the skin such as Lysozyme-EGFP (circulating PMNs and monocytes, dermal macrophages), CD11c-EYFP (dermal dendritic cells, epidermal langherans cells) and Mcpt5-Cre+R26Y+ (dermal mast cells) mouse strains have been selected (Faust et al., 2000; Lindquist et al., 2004; Dudeck et al., 2011). The conditions to prepare bacteria inoculum will be set up. The planktonic inoculum will be obtained from an overnight culture of fluorescent S. aureus Lyo-S2 strain in Trypticase Soja broth (Marquès et al., 2015). Biofilms will be generated from a planktonic culture incubated at 37°C under static conditions. After 24 h of incubation, biofilms will be gently collected (A). The first series of experiments will be performed with Lysozyme-EGFP mice inoculated into the ear tissue with the same number of CFU of either planktonic or sessile bacteria (B). Inoculum will be micro-injected in two injection points in the dermis of the ear pinna tissue with a nanofil syringe (Mac-Daniel et al., 2016). At early time-points, mice will be anesthetized and the cellular recruitment will be analyzed by confocal microscopy at the injection points (C1a and C1b). The behavior of recruited or resident innate immune cells (moving speed, trajectory, distance covered) will be analyzed, as well as their specific interactions with bacteria. In parallel, additional groups of mice will be inoculated with planktonic or biofilm bacteria to perform quantitative analyzes over 14 days in the ear tissue and the cutaneous draining lymph node (auricular lymph node), i.e., determination of the phenotype of recruited cells (C2), of the cytokine levels (C3), and counting of bacteria in the target tissues (C4). The inflammation visible to the eye and macroscopic cutaneous lesions (skin necrosis) will be observed and compared.
FIGURE 1. The three steps’ workflow proposed for extracting, post either Staphylococcus aureus planktonic or biofilm delivery, specific immune signatures displayed by mouse myeloid cells. (A) Inoculum preparation in vitro. (B) Inoculum micro-injection in the ear pinna of mouse (107 UFC of either planktonic or sessile bacteria). (C1a) Real time imaging of the ear pinna of a LysM-EGFP transgenic mouse harboring fluorescent phagocytes and fluorescent S. aureus bacteria by confocal microscopy. (C1b) Massive recruitment of GFP+ cells post-injection in the upper dermis of the ear pinna of a mouse inoculated with S. aureus sessile or planktonic bacteria. (C2), (C3), (C4) Quantitative analyses in the ear pinna and the auricular lymph node to determine (C2) the phenotype of recruited cells, (C3) the cytokine levels and (C4) the bacteria counts.
The objective of the review was to highlight the requirement of developing new in vivo models to analyze, at the early stage of infection, the dynamics of innate immune responses against S. aureus planktonic or sessile bacteria. A new experimental approach in the field combining the mouse ear pinna model and the intravital imaging approach is proposed. The long term objectives are to use this model as a pre-clinical model to test necessary new therapeutic approaches targeting the host immune system, as proposed initially by Hanke et al. (2013).
CF: intellectual contribution to the work; EB: intellectual contribution to the work; GM: substantial, direct and intellectual contribution to the work; PG: substantial, direct and major intellectual contribution to the work-corresponding author. All authors listed approved the work for publication.
The authors declare that the research was conducted in the absence of any commercial or financial relationships that could be construed as a potential conflict of interest.
The authors wish to acknowledge the contribution of Caroline Vachias at the Confocal Microscopy Facility ICCF (Imagerie Confocale Clermont-Ferrand) at Université Clermont Auvergne.
Alhede, M., Bjarnsholt, T., Givskov, M., and Alhede, M. (2014). Pseudomonas aeruginosa biofilms: mechanisms of immune evasion. Adv. Appl. Microbiol. 86, 1–40. doi: 10.1016/B978-0-12-800262-9.00001-9
Alhede, M., Bjarnsholt, T., Jensen, P. Ø., Phipps, R. K., Moser, C., Christophersen, L., et al. (2009). Pseudomonas aeruginosa recognizes and responds aggressively to the presence of polymorphonuclear leukocytes. Microbiol. Read. Engl. 155, 3500–3508. doi: 10.1099/mic.0.031443-0
Allewell, N. M. (2016). Introduction to biofilms thematic minireview series. J. Biol. Chem. 291, 12527–12528. doi: 10.1074/jbc.R116.734103
Alvarez, M. E., Fuxman Bass, J. I., Geffner, J. R., Fernández Calotti, P. X., Costas, M., Coso, O. A., et al. (2006). Neutrophil signaling pathways activated by bacterial DNA stimulation. J. Immunol. 1950, 4037–4046. doi: 10.4049/jimmunol.177.6.4037
Amino, R., Thiberge, S., Blazquez, S., Baldacci, P., Renaud, O., Shorte, S., et al. (2007). Imaging malaria sporozoites in the dermis of the mammalian host. Nat. Protoc. 2, 1705–1712. doi: 10.1038/nprot.2007.120
Amino, R., Thiberge, S., Martin, B., Celli, S., Shorte, S., Frischknecht, F., et al. (2006). Quantitative imaging of Plasmodium transmission from mosquito to mammal. Nat. Med. 12, 220–224. doi: 10.1038/nm1350
Balasubramanian, D., Ohneck, E. A., Chapman, J., Weiss, A., Kim, M. K., Reyes-Robles, T., et al. (2016). Staphylococcus aureus coordinates leukocidin expression and pathogenesis by sensing metabolic fluxes via RpiRc. mBio 7:e00818-16. doi: 10.1128/mBio.00818-16
Bernthal, N. M., Pribaz, J. R., Stavrakis, A. I., Billi, F., Cho, J. S., Ramos, R. I., et al. (2011). Protective role of IL-1β against post-arthroplasty Staphylococcus aureus infection. J. Orthop. Res. 29, 1621–1626. doi: 10.1002/jor.21414
Bjarnsholt, T., Jensen, P. Ø., Burmølle, M., Hentzer, M., Haagensen, J. A. J., Hougen, H. P., et al. (2005). Pseudomonas aeruginosa tolerance to tobramycin, hydrogen peroxide and polymorphonuclear leukocytes is quorum-sensing dependent. Microbiol. Read. Engl. 151, 373–383. doi: 10.1099/mic.0.27463-0
Brinkmann, V., Reichard, U., Goosmann, C., Fauler, B., Uhlemann, Y., Weiss, D. S., et al. (2004). Neutrophil extracellular traps kill bacteria. Science 303, 1532–1535. doi: 10.1126/science.1092385
Carneiro, M. B., Hohman, L. S., Egen, J. G., and Peters, N. C. (2017). Use of two-photon microscopy to study Leishmania major infection of the skin. Methods 127, 45–52. doi: 10.1016/j.ymeth.2017.04.012
Christensen, L. D., Moser, C., Jensen, P. Ø., Rasmussen, T. B., Christophersen, L., Kjelleberg, S., et al. (2007). Impact of Pseudomonas aeruginosa quorum sensing on biofilm persistence in an in vivo intraperitoneal foreign-body infection model. Microbiol. Read. Engl. 153, 2312–2320. doi: 10.1099/mic.0.2007/006122-0
Corrado, A., Donato, P., Maccari, S., Cecchi, R., Spadafina, T., Arcidiacono, L., et al. (2016). Staphylococcus aureus-dependent septic arthritis in murine knee joints: local immune response and beneficial effects of vaccination. Sci. Rep. 6:38043. doi: 10.1038/srep38043
Corrigan, R. M., Abbott, J. C., Burhenne, H., Kaever, V., and Gründling, A. (2011). c-di-AMP is a new second messenger in Staphylococcus aureus with a role in controlling cell size and envelope stress. PLOS Pathog. 7:e1002217. doi: 10.1371/journal.ppat.1002217
Costerton, J. W., Stewart, P. S., and Greenberg, E. P. (1999). Bacterial biofilms: a common cause of persistent infections. Science 284, 1318–1322. doi: 10.1126/science.284.5418.1318
Dudeck, A., Dudeck, J., Scholten, J., Petzold, A., Surianarayanan, S., Köhler, A., et al. (2011). Mast cells are key promoters of contact allergy that mediate the adjuvant effects of haptens. Immunity 34, 973–984. doi: 10.1016/j.immuni.2011.03.028
Faust, N., Varas, F., Kelly, L. M., Heck, S., and Graf, T. (2000). Insertion of enhanced green fluorescent protein into the lysozyme gene creates mice with green fluorescent granulocytes and macrophages. Blood 96, 719–726.
Fuxman Bass, J. I., Gabelloni, M. L., Alvarez, M. E., Vermeulen, M. E., Russo, D. M., Zorreguieta, A., et al. (2008). Characterization of bacterial DNA binding to human neutrophil surface. Lab. Invest. 88, 926–937. doi: 10.1038/labinvest.2008.59
Geiger, T., Francois, P., Liebeke, M., Fraunholz, M., Goerke, C., Krismer, B., et al. (2012). The stringent response of Staphylococcus aureus and its impact on survival after phagocytosis through the induction of intracellular PSMs expression. PLOS Pathog. 8:e1003016. doi: 10.1371/journal.ppat.1003016
Guo, Y., Ramos, R. I., Cho, J. S., Donegan, N. P., Cheung, A. L., and Miller, L. S. (2013). In vivo bioluminescence imaging to evaluate systemic and topical antibiotics against community-acquired methicillin-resistant Staphylococcus aureus-infected skin wounds in mice. Antimicrob. Agents Chemother. 57, 855–863. doi: 10.1128/AAC.01003-12
Hanke, M. L., Angle, A., and Kielian, T. (2012). MyD88-dependent signaling influences fibrosis and alternative macrophage activation during Staphylococcus aureus biofilm infection. PLOS ONE 7:e42476. doi: 10.1371/journal.pone.0042476
Hanke, M. L., Heim, C. E., Angle, A., Sanderson, S. D., and Kielian, T. (2013). Targeting macrophage activation for the prevention and treatment of Staphylococcus aureus biofilm infections. J. Immunol. 1950, 2159–2168. doi: 10.4049/jimmunol.1202348
Hanke, M. L., and Kielian, T. (2012). Deciphering mechanisms of staphylococcal biofilm evasion of host immunity. Front. Cell. Infect. Microbiol. 2:62. doi: 10.3389/fcimb.2012.00062
Hanses, F., Park, S., Rich, J., and Lee, J. C. (2011). Reduced neutrophil apoptosis in diabetic mice during staphylococcal infection leads to prolonged Tnfα production and reduced neutrophil clearance. PLOS ONE 6:e23633. doi: 10.1371/journal.pone.0023633
Heim, C. E., Vidlak, D., Scherr, T. D., Kozel, J. A., Holzapfel, M., Muirhead, D. E., et al. (2014). Myeloid-derived suppressor cells contribute to Staphylococcus aureus orthopedic biofilm infection. J. Immunol. 1950, 3778–3792. doi: 10.4049/jimmunol.1303408
Jain, R., and Weninger, W. (2013). Shedding light on cutaneous innate immune responses: the intravital microscopy approach. Immunol. Cell Biol. 91, 263–270. doi: 10.1038/icb.2012.76
Jakobsen, T. H., van Gennip, M., Phipps, R. K., Shanmugham, M. S., Christensen, L. D., Alhede, M., et al. (2012). Ajoene, a sulfur-rich molecule from garlic, inhibits genes controlled by quorum sensing. Antimicrob. Agents Chemother. 56, 2314–2325. doi: 10.1128/AAC.05919-11
Jakubzick, C. V., Randolph, G. J., and Henson, P. M. (2017). Monocyte differentiation and antigen-presenting functions. Nat. Rev. Immunol. 17, 349–362. doi: 10.1038/nri.2017.28
Jensen, P. Ø., Givskov, M., Bjarnsholt, T., and Moser, C. (2010). The immune system vs. Pseudomonas aeruginosa biofilms. FEMS Immunol. Med. Microbiol. 59, 292–305. doi: 10.1111/j.1574-695X.2010.00706.x
Jensen, P. Ø., Moser, C., Kobayashi, O., Hougen, H. P., Kharazmi, A., and Høiby, N. (2004). Faster activation of polymorphonuclear neutrophils in resistant mice during early innate response to Pseudomonas aeruginosa lung infection. Clin. Exp. Immunol. 137, 478–485. doi: 10.1111/j.1365-2249.2004.02554.x
Kashem, S. W., Haniffa, M., and Kaplan, D. H. (2017). Antigen-presenting cells in the skin. Annu. Rev. Immunol. 35, 469–499. doi: 10.1146/annurev-immunol-051116-052215
Kavanaugh, J. S., and Horswill, A. R. (2016). Impact of environmental cues on staphylococcal quorum sensing and biofilm development. J. Biol. Chem. 291, 12556–12564. doi: 10.1074/jbc.R116.722710
Lebeaux, D., Ghigo, J.-M., and Beloin, C. (2014). Biofilm-related infections: bridging the gap between clinical management and fundamental aspects of recalcitrance toward antibiotics. Microbiol. Mol. Biol. Rev. 78, 510–543. doi: 10.1128/MMBR.00013-14
Li, J. L., Goh, C. C., Keeble, J. L., Qin, J. S., Roediger, B., Jain, R., et al. (2012). Intravital multiphoton imaging of immune responses in the mouse ear skin. Nat. Protoc. 7, 221–234. doi: 10.1038/nprot.2011.438
Lindquist, R. L., Shakhar, G., Dudziak, D., Wardemann, H., Eisenreich, T., Dustin, M. L., et al. (2004). Visualizing dendritic cell networks in vivo. Nat. Immunol. 5, 1243–1250. doi: 10.1038/ni1139
Mac-Daniel, L., Buckwalter, M. R., Gueirard, P., and Ménard, R. (2016). Myeloid cell isolation from mouse skin and draining lymph node following intradermal immunization with live attenuated Plasmodium sporozoites. J. Vis. Exp. 111:e53796. doi: 10.3791/53796
Marquès, C., Tasse, J., Pracros, A., Collin, V., Franceschi, C., Laurent, F., et al. (2015). Effects of antibiotics on biofilm and unattached cells of a clinical Staphylococcus aureus isolate from bone and joint infection. J. Med. Microbiol. 64, 1021–1026. doi: 10.1099/jmm.0.000125
Mittal, R., Sharma, S., Chhibber, S., and Harjai, K. (2009). Evaluation of tumour necrosis factor-alpha and interleukin-1beta in an experimental pyelonephritis model induced with planktonic and biofilms cells of Pseudomonas aeruginosa. Can. J. Infect. Dis. Med. Microbiol. 20, e35–e42. doi: 10.1155/2009/810791
Moormeier, D. E., and Bayles, K. W. (2017). Staphylococcus aureus biofilm: a complex developmental organism. Mol. Microbiol. 104, 365–376. doi: 10.1111/mmi.13634
Moser, C., Pedersen, H. T., Lerche, C. J., Kolpen, M., Line, L., Thomsen, K., et al. (2017). Biofilms and host response - helpful or harmful. APMIS 125, 320–338. doi: 10.1111/apm.12674
Ng, L. G., Qin, J. S., Roediger, B., Wang, Y., Jain, R., Cavanagh, L. L., et al. (2011). Visualizing the neutrophil response to sterile tissue injury in mouse dermis reveals a three-phase cascade of events. J. Invest. Dermatol. 131, 2058–2068. doi: 10.1038/jid.2011.179
Nguyen, K. T., Seth, A. K., Hong, S. J., Geringer, M. R., Xie, P., Leung, K. P., et al. (2013). Deficient cytokine expression and neutrophil oxidative burst contribute to impaired cutaneous wound healing in diabetic, biofilm-containing chronic wounds. Wound Repair Regen. 21, 833–841. doi: 10.1111/wrr.12109
Nishitani, K., Sutipornpalangkul, W., de Mesy Bentley, K. L., Varrone, J. J., Bello-Irizarry, S. N., Ito, H., et al. (2015). Quantifying the natural history of biofilm formation in vivo during the establishment of chronic implant-associated Staphylococcus aureus osteomyelitis in mice to identify critical pathogen and host factors. J. Orthop. Res. 33, 1311–1319. doi: 10.1002/jor.22907
Niska, J. A., Meganck, J. A., Pribaz, J. R., Shahbazian, J. H., Lim, E., Zhang, N., et al. (2012). Monitoring bacterial burden, inflammation and bone damage longitudinally using optical and μCT imaging in an orthopaedic implant infection in mice. PLOS ONE 7:e47397. doi: 10.1371/journal.pone.0047397
Otto, M. (2008). Staphylococcal biofilms. Curr. Top. Microbiol. Immunol. 322, 207–228. doi: 10.1007/978-3-540-75418-3_10
Paharik, A. E., and Horswill, A. R. (2016). The staphylococcal biofilm: adhesins, regulation, and host response. Microbiol. Spectr. 4:VMBF-0022-2015. doi: 10.1128/microbiolspec.VMBF-0022-2015
Papenfort, K., and Bassler, B. L. (2016). Quorum sensing signal-response systems in Gram-negative bacteria. Nat. Rev. Microbiol. 14, 576–588. doi: 10.1038/nrmicro.2016.89
Pedersen, S. S., Kharazmi, A., Espersen, F., and Høiby, N. (1990). Pseudomonas aeruginosa alginate in cystic fibrosis sputum and the inflammatory response. Infect. Immun. 58, 3363–3368.
Periasamy, S., Joo, H.-S., Duong, A. C., Bach, T.-H. L., Tan, V. Y., Chatterjee, S. S., et al. (2012). How Staphylococcus aureus biofilms develop their characteristic structure. Proc. Natl. Acad. Sci. U.S.A. 109, 1281–1286. doi: 10.1073/pnas.1115006109
Peschel, A., and Otto, M. (2013). Phenol-soluble modulins and staphylococcal infection. Nat. Rev. Microbiol. 11, 667–673. doi: 10.1038/nrmicro3110
Peters, N. C., Egen, J. G., Secundino, N., Debrabant, A., Kimblin, N., Kamhawi, S., et al. (2008). In vivo imaging reveals an essential role for neutrophils in leishmaniasis transmitted by sand flies. Science 321, 970–974. doi: 10.1126/science.1159194
Prabhakara, R., Harro, J. M., Leid, J. G., Harris, M., and Shirtliff, M. E. (2011a). Murine immune response to a chronic Staphylococcus aureus biofilm infection. Infect. Immun. 79, 1789–1796. doi: 10.1128/IAI.01386-10
Prabhakara, R., Harro, J. M., Leid, J. G., Keegan, A. D., Prior, M. L., and Shirtliff, M. E. (2011b). Suppression of the inflammatory immune response prevents the development of chronic biofilm infection due to methicillin-resistant Staphylococcus aureus. Infect. Immun. 79, 5010–5018. doi: 10.1128/IAI.05571-11
Pribaz, J. R., Bernthal, N. M., Billi, F., Cho, J. S., Ramos, R. I., Guo, Y., et al. (2012). Mouse model of chronic post-arthroplasty infection: noninvasive in vivo bioluminescence imaging to monitor bacterial burden for long-term study. J. Orthop. Res. 30, 335–340. doi: 10.1002/jor.21519
Scherr, T. D., Hanke, M. L., Huang, O., James, D. B. A., Horswill, A. R., Bayles, K. W., et al. (2015). Staphylococcus aureus biofilms induce macrophage dysfunction through leukocidin AB and Alpha-toxin. mBio 6:e01021-15. doi: 10.1128/mBio.01021-15
Scherr, T. D., Heim, C. E., Morrison, J. M., and Kielian, T. (2014). Hiding in plain sight: interplay between staphylococcal biofilms and host immunity. Front. Immunol. 5:37. doi: 10.3389/fimmu.2014.00037
Secor, P. R., Michaels, L. A., Smigiel, K. S., Rohani, M. G., Jennings, L. K., Hisert, K. B., et al. (2017). Filamentous bacteriophage produced by Pseudomonas aeruginosa alters the inflammatory response and promotes noninvasive infection in vivo. Infect. Immun. 85: e00648-16. doi: 10.1128/IAI.00648-16
Shiels, S. M., Bedigrew, K. M., and Wenke, J. C. (2015). Development of a hematogenous implant-related infection in a rat model. BMC Musculoskelet. Disord. 16:255. doi: 10.1186/s12891-015-0699-7
Silva-Santana, G., Lenzi-Almeida, K. C., Lopes, V. G. S., and Aguiar-Alves, F. (2016). Biofilm formation in catheter-related infections by Panton-Valentine leukocidin-producing Staphylococcus aureus. Int. Microbiol. 19, 199–207. doi: 10.2436/20.1501.01.278
Snyder, D. T., Hedges, J. F., and Jutila, M. A. (2017). Getting “inside” type I IFNs: type I IFNs in intracellular bacterial infections. J. Immunol. Res. 2017:9361802. doi: 10.1155/2017/9361802
Sprangers, S., de Vries, T. J., and Everts, V. (2016). Monocyte heterogeneity: consequences for monocyte-derived immune cells. J. Immunol. Res. 2016:1475435. doi: 10.1155/2016/1475435
Sumaria, N., Roediger, B., Ng, L. G., Qin, J., Pinto, R., Cavanagh, L. L., et al. (2011). Cutaneous immunosurveillance by self-renewing dermal gammadelta T cells. J. Exp. Med. 208, 505–518. doi: 10.1084/jem.20101824
Tavares, J., Formaglio, P., Thiberge, S., Mordelet, E., Van Rooijen, N., Medvinsky, A., et al. (2013). Role of host cell traversal by the malaria sporozoite during liver infection. J. Exp. Med. 210, 905–915. doi: 10.1084/jem.20121130
Tay, S. S., Roediger, B., Tong, P. L., Tikoo, S., and Weninger, W. (2014). The skin-resident immune network. Curr. Dermatol. Rep. 3, 13–22. doi: 10.1007/s13671-013-0063-9
Teng, T.-S., Ji, A.-L., Ji, X.-Y., and Li, Y.-Z. (2017). Neutrophils and immunity: from bactericidal action to being conquered. J. Immunol. Res. 2017:9671604. doi: 10.1155/2017/9671604
Thurlow, L. R., Hanke, M. L., Fritz, T., Angle, A., Aldrich, A., Williams, S. H., et al. (2011). Staphylococcus aureus biofilms prevent macrophage phagocytosis and attenuate inflammation in vivo. J. Immunol. 1950, 6585–6596. doi: 10.4049/jimmunol.1002794
Tong, P. L., Roediger, B., Kolesnikoff, N., Biro, M., Tay, S. S., Jain, R., et al. (2015). The skin immune atlas: three-dimensional analysis of cutaneous leukocyte subsets by multiphoton microscopy. J. Invest. Dermatol. 135, 84–93. doi: 10.1038/jid.2014.289
Torre, A., Bacconi, M., Sammicheli, C., Galletti, B., Laera, D., Fontana, M. R., et al. (2015). Four-component Staphylococcus aureus vaccine 4C-staph enhances Fcγ receptor expression in neutrophils and monocytes and mitigates S. aureus infection in neutropenic mice. Infect. Immun. 83, 3157–3163. doi: 10.1128/IAI.00258-15
Trøstrup, H., Thomsen, K., Christophersen, L. J., Hougen, H. P., Bjarnsholt, T., Jensen, P. Ø., et al. (2013). Pseudomonas aeruginosa biofilm aggravates skin inflammatory response in BALB/c mice in a novel chronic wound model. Wound Repair Regen. 21, 292–299. doi: 10.1111/wrr.12016
Valentini, M., and Filloux, A. (2016). Biofilms and cyclic di-GMP (c-di-GMP) signaling: lessons from Pseudomonas aeruginosa and other bacteria. J. Biol. Chem. 291, 12547–12555. doi: 10.1074/jbc.R115.711507
Valle, J., Solano, C., García, B., Toledo-Arana, A., and Lasa, I. (2013). Biofilm switch and immune response determinants at early stages of infection. Trends Microbiol. 21, 364–371. doi: 10.1016/j.tim.2013.05.008
Van Gennip, M., Christensen, L. D., Alhede, M., Qvortrup, K., Jensen, P. Ø., Høiby, N., et al. (2012). Interactions between polymorphonuclear leukocytes and Pseudomonas aeruginosa biofilms on silicone implants in vivo. Infect. Immun. 80, 2601–2607. doi: 10.1128/IAI.06215-11
van Gennip, M., Moser, C., Christensen, L. D., Bjarnsholt, T., Calum, H., Jensen, P. Ø., et al. (2009). Augmented effect of early antibiotic treatment in mice with experimental lung infections due to sequentially adapted mucoid strains of Pseudomonas aeruginosa. J. Antimicrob. Chemother. 64, 1241–1250. doi: 10.1093/jac/dkp352
Verdrengh, M., and Tarkowski, A. (1997). Role of neutrophils in experimental septicemia and septic arthritis induced by Staphylococcus aureus. Infect. Immun. 65, 2517–2521.
Vidlak, D., and Kielian, T. (2016). Infectious dose dictates the host response during Staphylococcus aureus orthopedic-implant biofilm infection. Infect. Immun. 84, 1957–1965. doi: 10.1128/IAI.00117-16
Voyich, J. M., Vuong, C., DeWald, M., Nygaard, T. K., Kocianova, S., Griffith, S., et al. (2009). The SaeR/S gene regulatory system is essential for innate immune evasion by Staphylococcus aureus. J. Infect. Dis. 199, 1698–1706. doi: 10.1086/598967
Wagner, C., Kondella, K., Bernschneider, T., Heppert, V., Wentzensen, A., and Hänsch, G. M. (2003). Post-traumatic osteomyelitis: analysis of inflammatory cells recruited into the site of infection. Shock 20, 503–510. doi: 10.1097/01.shk.0000093542.78705.e3
Wang, Y., Cheng, L. I., Helfer, D. R., Ashbaugh, A. G., Miller, R. J., Tzomides, A. J., et al. (2017). Mouse model of hematogenous implant-related Staphylococcus aureus biofilm infection reveals therapeutic targets. Proc. Natl. Acad. Sci. U.S.A. 114, E5094–E5102. doi: 10.1073/pnas.1703427114
Watters, C., DeLeon, K., Trivedi, U., Griswold, J. A., Lyte, M., Hampel, K. J., et al. (2013). Pseudomonas aeruginosa biofilms perturb wound resolution and antibiotic tolerance in diabetic mice. Med. Microbiol. Immunol. 202, 131–141. doi: 10.1007/s00430-012-0277-7
Watters, C., Everett, J. A., Haley, C., Clinton, A., and Rumbaugh, K. P. (2014). Insulin treatment modulates the host immune system to enhance Pseudomonas aeruginosa wound biofilms. Infect. Immun. 82, 92–100. doi: 10.1128/IAI.00651-13
Watters, C., Fleming, D., Bishop, D., and Rumbaugh, K. P. (2016). Host responses to biofilm. Prog. Mol. Biol. Transl. Sci. 142, 193–239. doi: 10.1016/bs.pmbts.2016.05.007
Keywords: bacteria, biofilm, intravital imaging, macrophage/monocyte, mouse, polymorphonuclear neutrophil
Citation: Forestier C, Billard E, Milon G and Gueirard P (2017) Unveiling and Characterizing Early Bilateral Interactions between Biofilm and the Mouse Innate Immune System. Front. Microbiol. 8:2309. doi: 10.3389/fmicb.2017.02309
Received: 24 August 2017; Accepted: 08 November 2017;
Published: 21 November 2017.
Edited by:
Marina I. Arleevskaya, Kazan State Medical Academy, RussiaReviewed by:
Livia Visai, Universita degli Studi di Pavia and Istituti Clinici Scientifici Maugeri di Pavia, ItalyCopyright © 2017 Forestier, Billard, Milon and Gueirard. This is an open-access article distributed under the terms of the Creative Commons Attribution License (CC BY). The use, distribution or reproduction in other forums is permitted, provided the original author(s) or licensor are credited and that the original publication in this journal is cited, in accordance with accepted academic practice. No use, distribution or reproduction is permitted which does not comply with these terms.
*Correspondence: Pascale Gueirard, cGFzY2FsZS5ndWVpcmFyZEB1Y2EuZnI=
Disclaimer: All claims expressed in this article are solely those of the authors and do not necessarily represent those of their affiliated organizations, or those of the publisher, the editors and the reviewers. Any product that may be evaluated in this article or claim that may be made by its manufacturer is not guaranteed or endorsed by the publisher.
Research integrity at Frontiers
Learn more about the work of our research integrity team to safeguard the quality of each article we publish.