- 1VISAVET Health Surveillance Centre, Universidad Complutense Madrid, Madrid, Spain
- 2The Milner Centre for Evolution, Department of Biology and Biochemistry, University of Bath, Bath, United Kingdom
- 3Departamento de Bioquímica, Biología Molecular y Genética, Facultad de Veterinaria, Universidad de Extremadura, Badajoz, Spain
- 4Subdirección General de Sanidad e Higiene Animal y Trazabilidad, Dirección General de Sanidad de la Producción Agraria, Ministerio de Agricultura y Pesca, Alimentación y Medio Ambiente, Madrid, Spain
- 5Department of Animal Health, Faculty of Veterinary Medicine, Universidad Complutense Madrid, Madrid, Spain
Pathogens in the genus Campylobacter are the most common cause of food-borne bacterial gastro-enteritis. Campylobacteriosis, caused principally by Campylobacter jejuni and Campylobacter coli, is transmitted to humans by food of animal origin, especially poultry. As for many pathogens, antimicrobial resistance in Campylobacter is increasing at an alarming rate. Erythromycin prescription is the treatment of choice for clinical cases requiring antimicrobial therapy but this is compromised by mobility of the erythromycin resistance gene erm(B) between strains. Here, we evaluate resistance to six antimicrobials in 170 Campylobacter isolates (133 C. coli and 37 C. jejuni) from turkeys. Erythromycin resistant isolates (n = 85; 81 C. coli and 4 C. jejuni) were screened for the presence of the erm(B) gene, that has not previously been identified in isolates from turkeys. The genomes of two positive C. coli isolates were sequenced and in both isolates the erm(B) gene clustered with resistance determinants against aminoglycosides plus tetracycline, including aad9, aadE, aph(2″)-IIIa, aph(3′)-IIIa, and tet(O) genes. Comparative genomic analysis identified identical erm(B) sequences among Campylobacter from turkeys, Streptococcus suis from pigs and Enterococcus faecium and Clostridium difficile from humans. This is consistent with multiple horizontal transfer events among different bacterial species colonizing turkeys. This example highlights the potential for dissemination of antimicrobial resistance across bacterial species boundaries which may compromise their effectiveness in antimicrobial therapy.
Introduction
The World Health Organization (WHO) has recently published a list of bacteria for which new antibiotic therapies are urgently needed, with Campylobacter classified as high priority (WHO, 2017). This is of concern as campylobacteriosis is the most commonly notified bacterial foodborne infection in the European Union (European Food Safety Authority [EFSA]/European Centre for Disease Prevention and Control [ECDC], 2016a). The disease is principally caused by Campylobacter jejuni and Campylobacter coli following the ingestion of contaminated food and drink, with consumption of poultry meat recognized as a major risk factor (Wilson et al., 2008; Whiley et al., 2013). Infection can be associated with extra intestinal pathologies and sequelae such as reactive arthritis or Guillain-Barré syndrome (Nachamkin et al., 1998), but it is usually self-limiting. Treatment of severe infection occasionally requires antimicrobial therapy, often with erythromycin (European Food Safety Authority [EFSA]/European Centre for Disease Prevention and Control [ECDC], 2016b) and to a lesser extent with gentamicin, the later used occasionally when infection becomes systemic (Lehtopolku et al., 2009). Although fluoroquinolones were commonly used in the past, the rising of resistance among Campylobacter isolates makes these antibiotics ineffective (Kassem et al., 2016).
Erythromycin inhibits protein synthesis by binding to the ribosome and blocking the exit of the nascent peptide chain (Fyfe et al., 2016). Erythromycin resistance in bacterial isolates from animals and humans is associated with the presence of erm genes (Weisblum, 1995). The most widely distributed erm gene class is erm(B), which encodes an rRNA methylase which produces cross-resistance to macrolides, lincosamides and streptogramins B (MLSB phenotype) (Leclercq, 2002). The erm(B) encoded enzyme acts on the 23S rRNA gene by methylating an adenine residue that hinders antibiotic binding-to the ribosome (Weisblum, 1995). The erm(B) gene is present in a variety of Gram-positive bacteria, including enterococci, streptococci, and staphylococci (Jensen et al., 1999). The potential for interspecies horizontal gene transfer (HGT) (Conwell et al., 2017) has facilitated the emergence of resistance in multiple species including Gram-negative bacteria of the genera Bacteroides, Shigella, Escherichia, Klebsiella, and recently Campylobacter (Shoemaker et al., 2001; Soge et al., 2006; Phuc Nguyen et al., 2009; Qin et al., 2014). Resistance in Campylobacter has been associated with ribosomal mutations, efflux pumps and the erm(B) gene that has been identified in isolates from China and Spain (Qin et al., 2014; Florez-Cuadrado et al., 2016; Fyfe et al., 2016). Recent work has reported high-level erythromycin resistance (MIC ≥ 1024 mg/L) in a C. coli isolate carrying erm(B)- in a genomic island along with other determinants conferring resistance to aminoglycosides, tetracycline and streptothricin (Florez-Cuadrado et al., 2016). Thus, eight types of erm(B)-carrying genomic islands have been differentiated (Qin et al., 2014; Wang et al., 2014; Florez-Cuadrado et al., 2016), all of which share aminoglycoside resistance genes in addition to other determinants, likely leading to co-selection after genetic mobilization (Chapman, 2003).
Improved understanding of the distribution of resistance genes within bacterial species in different host niches, and the mobility of these genes between populations, could be important for identifying source and sink populations. In the case of Campylobacter, the erm(B) gene has been identified in C. coli isolates from chicken, ducks, swine, and humans and from C. jejuni isolated from chicken (Qin et al., 2014; Wang et al., 2014; Deng et al., 2015) but other host species may be relevant. Turkeys are among the top 10 farmed animals in Europe and the United States with an estimated 323 million birds reared anually (FAOSTAT, 2017). While studies have shown that turkeys are an important host species harboring large numbers of C. jejuni and C. coli, the resistance status of these strains is not well-characterized. This has lead to the inclusion of this animal species in international surveillance programs to evaluate the levels of antibiotic resistance. In this study we carried out combined molecular microbiology and whole genome sequencing approaches to evaluate the presence of erm(B) and it’s genetic background in Campylobacter isolates obtained from turkeys sampled in Spain. The comparison with erm(B) sequences from other host species might allow further description of the microevolutionary events associated with the acquisition of this antibiotic resistance genes in Campylobacter.
Materials and Methods
Strains and Growth Conditions
Campylobacter isolates were recovered in 2014 (n = 170; 133 C. coli and 37 C. jejuni) from turkey samples obtained in the framework of the European Antimicrobial Resistance Surveillance program (DC 652/2013) in Spain (European Comission, 2013). Samples were collected at the largest turkey slaughterhouses in Spain located in different regions within the country. Each Campylobacter isolate represented a single farm and they were obtained by culturing pooled feces from turkeys (117 pooled samples: 10 animals per pool, 1170 individual fecal samples analyzed). Each pooled sample was cultured on Campylobacter blood-free selective medium (CCDA) (Oxoid). Inoculated media were incubated at 42°C for 48 h under microaerobic conditions with a commercial gas-generating system (atmosphere generator system, Oxoid). Suspected colonies were subcultured onto blood agar (BioMérieux) at 37°C for 48 h. All strains were identified by conventional multiplex PCR of the genus Campylobacter that allows the differentiation between C. coli and C. jejuni with specific primers, as described previously (Ugarte-Ruiz et al., 2012).
Antimicrobial Susceptibility Testing
Broth microdilution methods were performed to determine the antimicrobial susceptibility of the Campylobacter isolates [minimum inhibitory concentrations (MICs)]. The following antimicrobials were tested: tetracycline, ciprofloxacin, nalidixic acid, erythromycin, streptomycin, and gentamicin. Isolates were grown on blood agar plates (bioMérieux) and incubated for 48 h at 37°C under microaerophilic conditions. Growth from these cultures was suspended in sterilized water and adjusted at 0.5 McFarland. Fifty microliters of these inocula were added to 11 mL of cation-adjusted Mueller-Hinton broth (TREK Diagnostics Systems), and supplemented with 600 μL of lysed horse blood prepared in house from defibrinated horse blood (Oxoid). EUCAMP2 microdilution plates (TREK Diagnostics Systems) were inoculated and incubated under microaerophilic conditions at 37°C for 48 h. C. jejuni strain ATCC 33560 was used as a control for antimicrobial susceptibility test. Following the commission decision 2013/652/UE, the epidemiological cut-offs values considered were those described by EUCAST (EUCAST, 2017). Campylobacter isolates resistant to erythromycin (MICs: >8 mg/L to C. coli and >4 mg/L to C. jejuni) were selected to evaluate the presence of erm(B) gene.
Identification of erm(B) Gene and Whole-Genome Sequencing
The RNA methylase gene erm(B) was identified by PCR as described previously (Chen et al., 2007). Amplicons were detected by gel electrophoresis using 2% agarose gels containing 10 mg/ml SYBR Safe DNA gel stain (Invitrogen) for 40 min at 100 mA. DNA fractions were sequenced and compared using Sanger sequencing and MEGA software (version 5.05) respectively (Tamura et al., 2011; Heather and Chain, 2016). erm(B)-positive Campylobacter isolates were selected for whole genome sequencing. For DNA extraction, Campylobacter isolates were grown on blood agar plates (48 h at 42°C under microaerophilic conditions) and DNA was extracted using a QiAmp DNA mini kit (Qiagen). DNA was quantified using a Nanodrop spectrophotometer before sequencing. High-throughput genome sequencing was performed using a benchtop MiSeq sequencer (Illumina), and the short read paired-end data was assembled using the de novo assembly algorithm, SPAdes (Bankevich et al., 2012). Genome sequences were archived in the web-accessible Bacterial Isolate Genome Sequence Database: BIGSdb (Jolley and Maiden, 2010), which included functionality for identifying MLST profiles based on the pubMLST database1. Allelic diversity was evaluated using a gene-by-gene approach for genome alignment and comparison with the BLAST algorithm as previously described (Sheppard et al., 2012).
Results
Antimicrobial Susceptibility Testing
A total of 170 Campylobacter isolates (133 C. coli and 37 C. jejuni) were tested for susceptibility to six antimicrobials. Antimicrobial resistance profiles (Table 1) and MIC distributions were recorded (Supplementary Table S1). The highest proportion of antimicrobial resistance was to tetracycline (168/170; 98.8%) followed by nalidixic acid/ciprofloxacin (164/170; 96.4%), erythromycin (85/170; 50%), streptomycin (82/170; 48.2%), and finally gentamicin (13/170; 7.6%). Considering C. coli and C. jejuni separately, higher prevalence of resistance was observed in C. coli for erythromycin, streptomycin, and gentamicin (Fisher’s exact test: p < 0.001). Based upon The European Food Safety Authority (EFSA) criteria for quantifying multi-drug resistance (MDR; resistance to at least three classes of antimicrobials tested), seven MDR profiles were recorded for Campylobacter isolates (Table 1) (European Food Safety Authority [EFSA]/European Centre for Disease Prevention and Control [ECDC], 2017). Seventy-nine C. coli isolates (59.4%) and three C. jejuni isolates (8.5%) showed resistance to ciprofloxacin and erythromycin (treatment used against campylobacteriosis before onset of resistance and the current treatment against this bacteria). Campylobacter isolates resistant to erythromycin (Table 1) were analyzed for the presence of RNA methylase gene erm(B).
erm(B)-Carrying Campylobacter Isolates from Turkeys
Among the 85 erythromycin-resistant isolates, two (2.4%) carried the erm(B) gene: C. coli ZTA14/01086 and C. coli ZTA14/01426. The C. coli ZTA14/01086 is resistant to ciprofloxacin/nalidixic acid, tetracycline, erythromycin and streptomycin, whereas C. coli ZTA14/01426 shares the same MDR pattern and gentamicin resistance. The erythromycin-resistance MICs were 256 mg/L for C. coli ZTA14/01086 and >1024 mg/L for C. coli ZTA14/01426. Whole genome sequencing revealed that the erm(B) gene in C. coli ZTA14/01086 was located in a cluster along with other resistance genes, including tet(O) and aad9 which confer resistance to tetracycline and spectinomycin respectively (Figure 1; GenBank accession number: MF134831). The genome of C. coli ZTA14/01426 isolate contained the erm(B) gene in a cluster with aminoglycoside resistance genes aph(2″)-IIIa, aph(3′)-IIIa, and aadE (Figure 1; GenBank accession number: MF134832). Both genomic islands were compared with the erm(B)-carrying genomic island from C. coli ZTA09/02204, identified in Spain (GenBank accession number: KT953380) (Figure 1 and Supplementary Table S2). Other than these resistance determinants, analysis of 23S rRNA and ribosomal protein (L4 and L22) genes from genomes of the erm(B) positive strains from turkeys, revealed the absence of ribosomal mutations previously related with erythromycin resistance (Gibreel et al., 2005).
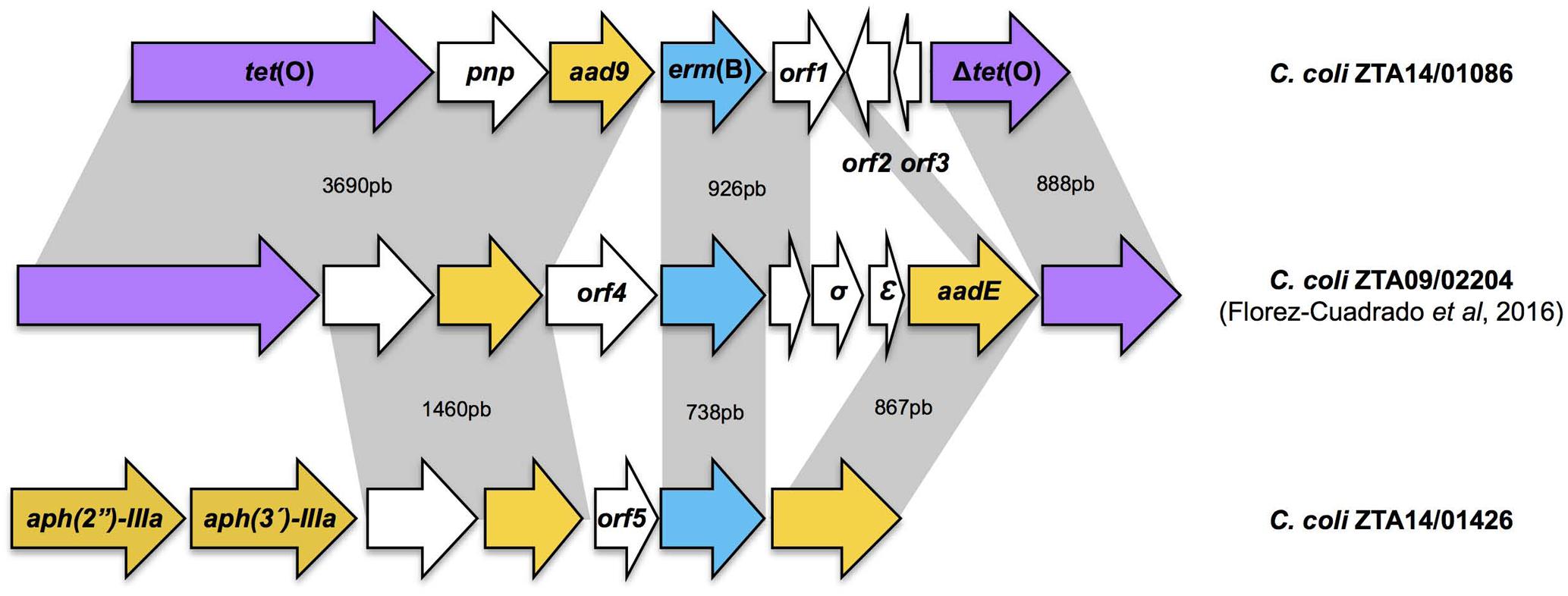
FIGURE 1. Comparative genetic organization of erm(B)-carrying genomic islands from Campylobacter coli ZTA14/01086 (this study), C. coli ZTA 14/01426 (this study) and previously identified C. coli ZTA09/02204 (17). Antimicrobial resistance genes are colored as follows: erm(B) gene (blue); aminoglycoside resistance genes (yellow); the tetracycline resistance gene tet(O) (purple). Genes with other predicted functions or encoding hypothetical proteins are shown in white. Gray shading indicates regions sharing 98% DNA identity.
erm(B) Allelic Variation among Bacterial Genera, Hosts, and Countries
Comparison of sequence homology can provide information about the horizontal transfer of resistance genes, including erm(B), among bacterial species. The nucleotide sequences of 10 erm(B) genes present in Campylobacter were compared, two from this study and eight of Chinese and Spanish origin. Using the first erm(B) sequence described in Campylobacter as reference (C. coli ZC113; GenBank accession number: KC575115), four alleles have been identified in the erm(B) sequences from Campylobacter (Supplementary Table S3). Allele 1 was the most common (7/10) and was used as reference. Alleles 2 and 3 were present in only one C. coli isolate each from Spain, with SNPs A299G (Asn-100-Ser) and A353G (His-118-Arg), respectively. Allele 4 was found in a C. jejuni isolate from China and is characterized by the synonymous SNP C726T. Bacterial genera, hosts and origins with erm(B) sequence identical to the four alleles observed in Campylobacter were identified in the NCBI database, compiled (Supplementary Table S3) and compared (Figure 2). The erm(B) alleles detected in Campylobacter had been previously identified mainly in Enterococcus and Staphylococcus isolated from humans and pigs in Asia and Europe. Allele 1 of erm(B) is represented in 31 sequences from eight bacterial species, including Streptococcus suis (11/31; 35.5%), Enterococcus faecium (7/31; 22.5%), and C. coli (7/31; 22,5%). S. suis isolates were mainly isolated in China (7/11; 63.6%) and all were from swine hosts. Sequences of E. faecium were mainly from Japan (4/7; 57.1%) and all were of human origin. The majority of erm(B) allele 1 sequences from Campylobacter were of Chinese origin (6/7; 85.7%) and sampled from humans, swine, and chickens. Allele 2 of erm(B) was detected in 16 bacterial species mainly Clostridium difficile (10/39; 25.6%) and E. faecium (7/39; 17.9%), from European and United States people and pigs of Chinese origin, respectively. Allele 2 of erm(B) is slightly less common in NCBI database than allele 1, being the only one identified in six bacterial genomes, mainly E. faecium (10/22; 45.4%) sampled from humans in the United States, Australia, Japan and South Korea, whereas allele 4 of erm(B) was not identified in any other sequence available in public databases.
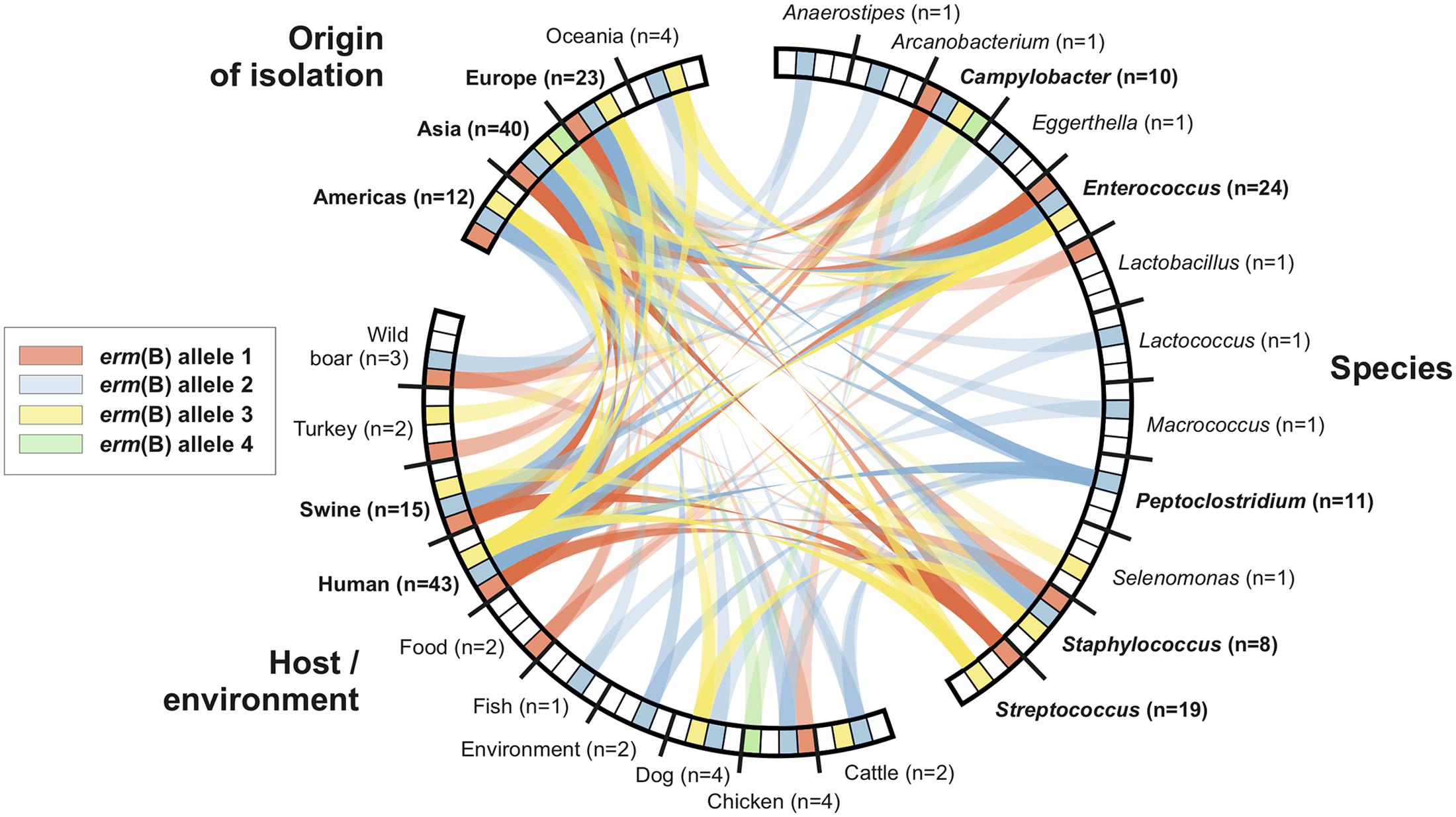
FIGURE 2. Host and geographical distribution of the erm(B) alleles identified in Campylobacter in this study among other bacterial genera. Allele 1 belongs to C. coli ZTA14/01426 (MF134832), allele 2 belongs to C. coli ZTA09/02204 (KT953380), allele 3 belongs to C. coli ZTA14/01086 (MF134831) and allele 4 belongs to C. jejuni C179b (KF864551). erm(B) homologs were identified in GenBank using BLAST with a coverage and similarity of 100%. Accession numbers of each sequence is given in Supplementary Table S3. Sequences without host data and geographical location have not been included. Exact identity of erm(B) alleles between species, host/environment and origin of isolation is represented by a connection (width proportional to relative prevalence).
Discussion
Definitive characterization of erythromycin resistance in bacterial pathogens is an important objective defined by the EFSA and the European Centre for Disease Prevention and Control (ECDC) (European Food Safety Authority [EFSA]/European Centre for Disease Prevention and Control [ECDC], 2017). To date, the erm(B) gene has been identified in Campylobacter isolates from swine, chickens, ducks and humans from China and in one broiler sample from Spain (Wang et al., 2014; Florez-Cuadrado et al., 2016). Monitoring antimicrobial resistance in Campylobacter isolated from turkeys has been mandatory since 2014 in European countries where the production of turkey meat exceeds 10,000 tons per year (2016). The occurrence of erythromycin resistant C. coli isolates from turkeys in Germany, Romania and Spain had risen to 43.3% in 2014 (2016), compared to previous surveys where lower frequencies were detected for poultry (14.5%) and swine (20.7%) (European Food Safety Authority [EFSA]/European Centre for Disease Prevention and Control [ECDC], 2015, 2016b). Since Campylobacter infections are related to consumption of food from animal origin, these levels of resistance could potentially produce therapeutic failure of antibiotic treatment for campylobacteriosis in humans.
Increased antimicrobial resistance among Campylobacter populations is a consequence of the widespread acquisition of antimicrobial resistance and clonal expansion of resistant lineages (Wimalarathna et al., 2013). Campylobacter can acquire DNA, including antimicrobial resistance genes, from relatively distantly related lineages through HGT, involving the replacement of homologous sequences, or the acquisition of mobile genetic elements (MGEs). There is evidence that plasmid acquisition mediates Campylobacter resistance to tetracycline, chloramphenicol and aminoglycosides (Courvalin et al., 1978; Taylor et al., 1987; Wang and Taylor, 1990) but in some cases, these resistances might be conferred by polymorphism of chromosomal sequences. This is also the case in Campylobacter for resistance to fluoroquinolones and macrolides, mediated by mutations in gyrA or 23S rDNA sequences, respectively (Engberg et al., 2001). Mutations that confer antimicrobial resistance can occur independently in multiple lineages but can also spread by natural transformation followed by homologous recombination, leading to the dissemination of antimicrobial resistance among bacteria that share an ecological niche (Meric et al., 2015).
The increasing number of studies identifying erm(B) genes in Campylobacter suggest that horizontal transfer is related to macrolide resistance in Campylobacter (Qin et al., 2014; Wang et al., 2014; Deng et al., 2015; Florez-Cuadrado et al., 2016; European Food Safety Authority [EFSA]/European Centre for Disease Prevention and Control [ECDC], 2017). High levels of resistance among C. coli isolates have been previously reported in isolates from livestock (Thakur and Gebreyes, 2005), potentially reflecting a selective advantage in these niches and clonal expansion of resistance lineages. This study shows the location of erm(B) gene in a genomic island along with other antimicrobial resistance genes including tet(O), encoding resistance to tetracycline and aph(3′)-IIIa, aacA-aphD, aad9 and aadE, conferring resistance to different aminoglycosides (Qin et al., 2014; Wang et al., 2014; Florez-Cuadrado et al., 2016), a clustering that implies the possibility of co-selection as an evolutionary mechanism (Chapman, 2003). In addition, linked erm(B) and aph(2”)-IIIa genes detected in the genome of C. coli ZTA14/01426 isolate, could be transferred to virulent strains limiting seriously the effectiveness of the two main choices for treatment of severe campylobacteriosis, erythromycin and gentamicin (Lehtopolku et al., 2009).
Although the presence of the erm(B) gene was evaluated on a total of 85 Campylobacter isolates, the scope of the genomic comparison was limited to only two C. coli because they were positive for the erythromycin resistance gene. Despite the limited number of genomes sequenced, the erm(B)-carrying genomic islands identified in Campylobacter isolated from Spain show genetic differences in comparison with ones from China. Thus, the genomic islands identified in Spain do not correspond to any of the six types of genomic islands identified in C. coli from China (Qin et al., 2014; Wang et al., 2014; Florez-Cuadrado et al., 2016). All of the erm(B)-carrying genomic islands posses aminoglycoside resistance genes but the gentamicin resistance gene aph(2”)-IIIa is present only in Spanish isolates. Resistance to gentamicin was represented in the Chinese erm(B)-carrying sequences with the presence of the aacA-aphD gene and was identified in C. coli isolates from humans, poultry, and swine (Wang et al., 2014).
Identical antibiotic resistance gene nucleotide sequences have been detected in both Gram-positive and Gram-negative bacteria. This is consistent with HGT facilitating the spread of resistance genes between distantly related species (Trieu-Cuot et al., 1985; Courvalin, 1994). Gram-positive bacteria including Enterococcus, Streptococcus, and Staphylococcus are widely known to harbor various resistance genes (Zilhao et al., 1988; Pinto-Alphandary et al., 1990) and recently, tetracycline and aminoglycoside resistance genes from Gram-positive bacteria have been identified in Gram-negative bacteria including Escherichia coli or Klebsiella pneumoniae (Arthur et al., 1987; Brisson-Noel et al., 1988) and Campylobacter (Lambert et al., 1985; Zilhao et al., 1988; Pinto-Alphandary et al., 1990). Four erm(B) alleles were identified in Campylobacter in this study (Supplementary Table S3) and comparison of these alleles with those from other bacteria provides useful information about possible routes of transmission (Figure 2). First, 100% nucleotide identity with erm(B) genes from Enterococcus, Streptococcus, Peptoclostridium, Anaerostipes, Arcanobacterium, Eggerthella, Lactobacillus, Lactococcus, Macrococcus, and Selenomonas suggests horizontal acquisition from Gram-positive bacteria, as previously described (Qin et al., 2014; Wang et al., 2014; Florez-Cuadrado et al., 2016). Second, the erm(B) alleles identified in this study have been previously identified mainly in human and porcine bacterial pathogens of Asian and European origin (Figure 2). The pig and human pathogen S. suis is considered a reservoir of antibiotic resistance genes (Palmieri et al., 2011) and transferable genetic elements carrying the erm(B), tet(O) and aminoglycoside resistance genes have previously been reported in S. suis isolates (Palmieri et al., 2012). The use of combined antibiotic therapies on swine farms potentially selects for bacterial isolates carrying resistance to different antibiotics (Zhu et al., 2013).
Allele 2 of the erm(B) gene has been identified in more bacterial genera than the other alleles in this study (Figure 2). All bacterial genera where erm(B) allele 2 has been identified belong to the Firmicutes, with two exceptions that belong to the Actinobacteria. These bacterial genera are present in the gastrointestinal tract and species like Enterococcus, Streptococcus, and Staphylococcus share the niche with Campylobacter (Zilhao et al., 1988). The transfer of antibiotic resistance genes in the human colon between bacteria of different genera has been reported (Huddleston, 2014) and it is possible that the antibiotics supplied in both animals and humans could facilitate the selection of strains carrying these multiresistant genomic islands and favor their dispersion. Identifying erm(B) genes and MDR genomic islands in Campylobacter isolates from turkeys, adds another component to the already extensive network of bacterial genera and hosts involved in the possible dispersion of critical antibiotic resistance genes and MGEs. This study highlights the need to sequence a greater number of Campylobacter genomes with the objective of evaluating the impact of genomic islands on the dispersion of antimicrobial resistance genes in this genus.
Author Contributions
Contributions to the conception: DF-C, AQ, MP, LD, and SS; design of the work: DF-C, MU-R, GM, BP, JS-L, and GO; analysis or interpretation of data: DF-C, MU-R, GM, and BP; drafting the work or revising it critically: DF-C, MU-R, GM, AQ, MP, BP, JS-L, GO, LD, and SS; final approval of the version published: DF-C, MU-R, GM, AQ, MP, BP, JS-L, GO, LD, and SS.
Funding
This work was partially supported by the Ministry of Science and Innovation (AGL2009-07550; AGL2012-39028), Ministry of Agriculture, Food and Environment (2014/000223), Autonomous Community of Madrid, Spain (S2009/AGR-1489; S2013/ABI-2747), and by the Spanish Ministry of Economy and Competitiveness (AGL2012-39028). DF-C is supported by the FPI program (BES-2013-065003) from the Spanish Ministry of Economy and Competitiveness. SS is funded by grants from the Medical Research Council (MR/L015080/1).
Conflict of Interest Statement
The authors declare that the research was conducted in the absence of any commercial or financial relationships that could be construed as a potential conflict of interest.
Acknowledgment
We wish to thank our technicians María García, Estefanía Rivero, and Nisrin Maasoumi for their excellent technical assistance.
Supplementary Material
The Supplementary Material for this article can be found online at: https://www.frontiersin.org/articles/10.3389/fmicb.2017.02240/full#supplementary-material
Footnotes
- ^https://pubmlst.org/campylobacter; accessed May 9, 2017
References
Arthur, M., Andremont, A., and Courvalin, P. (1987). Distribution of erythromycin esterase and rRNA methylase genes in members of the family Enterobacteriaceae highly resistant to erythromycin. Antimicrob. Agents Chemother. 31, 404–409. doi: 10.1128/AAC.31.3.404
Bankevich, A., Nurk, S., Antipov, D., Gurevich, A. A., Dvorkin, M., Kulikov, A. S., et al. (2012). SPAdes: a new genome assembly algorithm and its applications to single-cell sequencing. J. Comput. Biol. 19, 455–477. doi: 10.1089/cmb.2012.0021
Brisson-Noel, A., Arthur, M., and Courvalin, P. (1988). Evidence for natural gene transfer from gram-positive cocci to Escherichia coli. J. Bacteriol. 170, 1739–1745. doi: 10.1128/jb.170.4.1739-1745.1988
Chapman, J. S. (2003). Disinfectant resistance mechanisms, cross-resistance, and co-resistance. Int. Biodeterior. Biodegradation 51, 271–276. doi: 10.1016/S0964-8305(03)00044-1
Chen, J., Yu, Z., Michel, F. C. Jr., Wittum, T., and Morrison, M. (2007). Development and application of real-time PCR assays for quantification of erm genes conferring resistance to macrolides-lincosamides-streptogramin B in livestock manure and manure management systems. Appl. Environ. Microbiol. 73, 4407–4416. doi: 10.1128/AEM.02799-06
Conwell, M., Daniels, V., Naughton, P. J., and Dooley, J. S. (2017). Interspecies transfer of vancomycin, erythromycin and tetracycline resistance among Enterococcus species recovered from agrarian sources. BMC Microbiol. 17:19. doi: 10.1186/s12866-017-0928-3
Courvalin, P. (1994). Transfer of antibiotic resistance genes between gram-positive and gram-negative bacteria. Antimicrob. Agents Chemother. 38, 1447–1451. doi: 10.1128/AAC.38.7.1447
Courvalin, P. M., Shaw, W. V., and Jacob, A. E. (1978). Plasmid-mediated mechanisms of resistance to aminoglycoside-aminocyclitol antibiotics and to chloramphenicol in group D streptococci. Antimicrob. Agents Chemother. 13, 716–725. doi: 10.1128/AAC.13.5.716
Deng, F., Wang, Y., Zhang, Y., and Shen, Z. (2015). Characterization of the genetic environment of the ribosomal RNA methylase gene erm(B) in Campylobacter jejuni. J. Antimicrob. Chemother. 70, 613–615. doi: 10.1093/jac/dku418
Engberg, J., Aarestrup, F. M., Taylor, D. E., Gerner-Smidt, P., and Nachamkin, I. (2001). Quinolone and macrolide resistance in Campylobacter jejuni and C. coli: resistance mechanisms and trends in human isolates. Emerg. Infect. Dis. 7, 24–34. doi: 10.3201/eid0701.700024
EUCAST European Committee on Antimicrobial Susceptibility Testing. Available at: http://www.eucast.org
European Comission (2013). Commission implementing decision of 12 November 2013 on the monitoring and reporting of antimicrobial resistance in zoonotic and commensal bacteria (2013/652/EU). Off. J. Eur. Union 26-39, 303.
European Food Safety Authority [EFSA]/European Centre for Disease Prevention and Control [ECDC] (2015). The European Union summary report on antimicrobial resistance in zoonotic and indicator bacteria from humans, animals and food in 2013. EFSA Journal 11, 3196.
European Food Safety Authority [EFSA]/European Centre for Disease Prevention and Control [ECDC] (2016a). The European Union summary report on trends and sources of zoonoses, zoonotic agents and food-borne outbreaks in 2015. EFSA J. 14, e04634.
European Food Safety Authority [EFSA]/European Centre for Disease Prevention and Control [ECDC] (2016b). The European Union summary report on antimicrobial resistance in zoonotic and indicator bacteria from humans, animals and food in 2014. EFSA J. 14, 4380–4587.
European Food Safety Authority [EFSA]/European Centre for Disease Prevention and Control [ECDC] (2017). The European Union summary report on antimicrobial resistance in zoonotic and indicator bacteria from humans, animals and food in 2015. EFSA J. 15, 212.
FAOSTAT (2017). Food and Agriculture Organization of the United Nations (FAO), 2017. Available at: http://www.fao.org/faostat/en
Florez-Cuadrado, D., Ugarte-Ruiz, M., Quesada, A., Palomo, G., Dominguez, L., and Porrero, M. C. (2016). Description of an erm(B)-carrying Campylobacter coli isolate in Europe. J. Antimicrob. Chemother. 71, 841–843. doi: 10.1093/jac/dkv383
Fyfe, C., Grossman, T. H., Kerstein, K., and Sutcliffe, J. (2016). Resistance to macrolide antibiotics in public health pathogens. Cold Spring Harb. Perspect. Med. 6, a025395. doi: 10.1101/cshperspect.a025395
Gibreel, A., Kos, V. N., Keelan, M., Trieber, C. A., Levesque, S., Michaud, S., et al. (2005). Macrolide resistance in Campylobacter jejuni and Campylobacter coli: molecular mechanism and stability of the resistance phenotype. Antimicrob. Agents Chemother. 49, 2753–2759. doi: 10.1128/aac.49.7.2753-2759.2005
Heather, J. M., and Chain, B. (2016). The sequence of sequencers: the history of sequencing DNA. Genomics 107, 1–8. doi: 10.1016/j.ygeno.2015.11.003
Huddleston, J. R. (2014). Horizontal gene transfer in the human gastrointestinal tract: potential spread of antibiotic resistance genes. Infect. Drug Resist. 7, 167–176. doi: 10.2147/IDR.S48820
Jensen, L. B., Frimodt-Moller, N., and Aarestrup, F. M. (1999). Presence of erm gene classes in gram-positive bacteria of animal and human origin in Denmark. FEMS Microbiol. Lett. 170, 151–158. doi: 10.1111/j.1574-6968.1999.tb13368.x
Jolley, K. A., and Maiden, M. C. (2010). BIGSdb: Scalable analysis of bacterial genome variation at the population level. BMC Bioinformatics 11:595. doi: 10.1186/1471-2105-11-595
Kassem, I., Kehinde, O., Helmy, Y., Pina-Mimbela, R., Kumar, A., Chandrashekhar, K., et al. (2016). “Campylobacter in poultry: the conundrums of highly adaptable and ubiquitous foodborne pathogens,” in Foodborne Diseases, eds J. M. Soon, L. Manning, and C. AWallace (Boca Raton, FL: CRC Press), 79–112.
Lambert, T., Gerbaud, G., Trieu-Cuot, P., and Courvalin, P. (1985). Structural relationship between the genes encoding 3’-aminoglycoside phosphotransferases in Campylobacter and in gram-positive cocci. Ann. Inst. Pasteur Microbiol. 136B, 135–150. doi: 10.1016/S0769-2609(85)80040-5
Leclercq, R. (2002). Mechanisms of resistance to macrolides and lincosamides: nature of the resistance elements and their clinical implications. Clin. Infect. Dis. 34, 482–492. doi: 10.1086/324626
Lehtopolku, M., Nakari, U.-M., Kotilainen, P., Huovinen, P., Siitonen, A., and Hakanen, A. J. (2009). Antimicrobial susceptibilities of multidrug-resistant Campylobacter jejuni and C. coli strains: in vitro activities of 20 antimicrobial agents. Antimicrob. Agents Chemother. 54, 1232–1236. doi: 10.1128/AAC.00898-09
Meric, G., Miragaia, M., de Been, M., Yahara, K., Pascoe, B., Mageiros, L., et al. (2015). Ecological overlap and horizontal gene transfer in Staphylococcus aureus and Staphylococcus epidermidis. Genome Biol. Evol. 7, 1313–1328. doi: 10.1093/gbe/evv066
Nachamkin, I., Allos, B. M., and Ho, T. (1998). Campylobacter species and Guillain-Barre syndrome. Clin. Microbiol. Rev. 11, 555–567.
Palmieri, C., Magi, G., Mingoia, M., Bagnarelli, P., Ripa, S., Varaldo, P. E., et al. (2012). Characterization of a Streptococcus suis tet(O/W/32/O)-carrying element transferable to major streptococcal pathogens. Antimicrob. Agents Chemother. 56, 4697–4702. doi: 10.1128/AAC.00629-12
Palmieri, C., Varaldo, P. E., and Facinelli, B. (2011). Streptococcus suis, an emerging drug-resistant animal and human pathogen. Front. Microbiol. 2:235. doi: 10.3389/fmicb.2011.00235
Phuc Nguyen, M. C., Woerther, P. L., Bouvet, M., Andremont, A., Leclercq, R., and Canu, A. (2009). Escherichia coli as reservoir for macrolide resistance genes. Emerg. Infect. Dis. 15, 1648–1650. doi: 10.3201/eid1510.090696
Pinto-Alphandary, H., Mabilat, C., and Courvalin, P. (1990). Emergence of aminoglycoside resistance genes aadA and aadE in the genus Campylobacter. Antimicrob. Agents Chemother. 34, 1294–1296. doi: 10.1128/AAC.34.6.1294
Qin, S., Wang, Y., Zhang, Q., Zhang, M., Deng, F., Shen, Z., et al. (2014). Report of ribosomal RNA methylase gene erm(B) in multidrug-resistant Campylobacter coli. J. Antimicrob. Chemother. 69, 964–968. doi: 10.1093/jac/dkt492
Sheppard, S. K., Jolley, K. A., and Maiden, M. C. (2012). A gene-by-gene approach to bacterial population genomics: whole genome MLST of Campylobacter. Genes 3, 261–277. doi: 10.3390/genes3020261
Shoemaker, N. B., Vlamakis, H., Hayes, K., and Salyers, A. A. (2001). Evidence for extensive resistance gene transfer among Bacteroides spp. and among Bacteroides and other genera in the human colon. Appl. Environ. Microbiol. 67, 561–568. doi: 10.1128/AEM.67.2.561-568.2001
Soge, O. O., Adeniyi, B. A., and Roberts, M. C. (2006). New antibiotic resistance genes associated with CTX-M plasmids from uropathogenic Nigerian Klebsiella pneumoniae. J. Antimicrob. Chemother. 58, 1048–1053. doi: 10.1093/jac/dkl370
Tamura, K., Peterson, D., Peterson, N., Stecher, G., Nei, M., and Kumar, S. (2011). MEGA5: molecular evolutionary genetics analysis using maximum likelihood, evolutionary distance, and maximum parsimony methods. Mol. Biol. Evol. 28, 2731–2739. doi: 10.1093/molbev/msr121
Taylor, D. E., Hiratsuka, K., Ray, H., and Manavathu, E. K. (1987). Characterization and expression of a cloned tetracycline resistance determinant from Campylobacter jejuni plasmid pUA466. J. Bacteriol. 169, 2984–2989. doi: 10.1128/jb.169.7.2984-2989.1987
Thakur, S., and Gebreyes, W. A. (2005). Campylobacter coli in swine production: antimicrobial resistance mechanisms and molecular epidemiology. J. Clin. Microbiol. 43, 5705–5714. doi: 10.1128/JCM.43.11.5705-5714.2005
Trieu-Cuot, P., Gerbaud, G., Lambert, T., and Courvalin, P. (1985). In vivo transfer of genetic information between gram-positive and gram-negative bacteria. EMBO J. 4, 3583–3587.
Ugarte-Ruiz, M., Gomez-Barrero, S., Porrero, M. C., Alvarez, J., Garcia, M., Comeron, M. C., et al. (2012). Evaluation of four protocols for the detection and isolation of thermophilic Campylobacter from different matrices. J. Appl. Microbiol. 113, 200–208. doi: 10.1111/j.1365-2672.2012.05323.x
Wang, Y., and Taylor, D. E. (1990). Chloramphenicol resistance in Campylobacter coli: nucleotide sequence, expression, and cloning vector construction. Gene 94, 23–28. doi: 10.1016/0378-1119(90)90463-2
Wang, Y., Zhang, M., Deng, F., Shen, Z., Wu, C., Zhang, J., et al. (2014). Emergence of multidrug-resistant Campylobacter species isolates with a horizontally acquired rRNA methylase. Antimicrob. Agents Chemother. 58, 5405–5412. doi: 10.1128/AAC.03039-14
Weisblum, B. (1995). Erythromycin resistance by ribosome modification. Antimicrob. Agents Chemother. 39, 577–585. doi: 10.1128/AAC.39.3.577
Whiley, H., van den Akker, B., Giglio, S., and Bentham, R. (2013). The role of environmental reservoirs in human campylobacteriosis. Int. J. Environ. Res. Public Health 10, 5886–5907. doi: 10.3390/ijerph10115886
WHO (2017). WHO Publishes List of Bacteria for Which New Antibiotics are Urgently Needed. Available at: http://www.who.int/mediacentre/news/releases/2017/bacteria-antibiotics-needed/en/
Wilson, D. J., Gabriel, E., Leatherbarrow, A. J., Cheesbrough, J., Gee, S., Bolton, E., et al. (2008). Tracing the source of campylobacteriosis. PLOS Genet. 4:e1000203. doi: 10.1371/journal.pgen.1000203
Wimalarathna, H. M., Richardson, J. F., Lawson, A. J., Elson, R., Meldrum, R., Little, C. L., et al. (2013). Widespread acquisition of antimicrobial resistance among Campylobacter isolates from UK retail poultry and evidence for clonal expansion of resistant lineages. BMC Microbiol. 13:160. doi: 10.1186/1471-2180-13-160
Zhu, Y. G., Johnson, T. A., Su, J. Q., Qiao, M., Guo, G. X., Stedtfeld, R. D., et al. (2013). Diverse and abundant antibiotic resistance genes in Chinese swine farms. Proc. Natl. Acad. Sci. U.S.A. 110, 3435–3440. doi: 10.1073/pnas.1222743110
Keywords: Campylobacter, erythromycin, erm(B), turkey, antimicrobial, transmission
Citation: Florez-Cuadrado D, Ugarte-Ruiz M, Meric G, Quesada A, Porrero MC, Pascoe B, Sáez-Llorente JL, Orozco GL, Domínguez L and Sheppard SK (2017) Genome Comparison of Erythromycin Resistant Campylobacter from Turkeys Identifies Hosts and Pathways for Horizontal Spread of erm(B) Genes. Front. Microbiol. 8:2240. doi: 10.3389/fmicb.2017.02240
Received: 24 August 2017; Accepted: 31 October 2017;
Published: 15 November 2017.
Edited by:
Javier Carballo, University of Vigo, SpainReviewed by:
Heriberto Fernandez, Universidad Austral de Chile, ChileIssmat Kassem, American University of Beirut, Lebanon
Copyright © 2017 Florez-Cuadrado, Ugarte-Ruiz, Meric, Quesada, Porrero, Pascoe, Sáez-Llorente, Orozco, Domínguez and Sheppard. This is an open-access article distributed under the terms of the Creative Commons Attribution License (CC BY). The use, distribution or reproduction in other forums is permitted, provided the original author(s) or licensor are credited and that the original publication in this journal is cited, in accordance with accepted academic practice. No use, distribution or reproduction is permitted which does not comply with these terms.
*Correspondence: María Ugarte-Ruiz, bWFyaWEudWdhcnRlQHZpc2F2ZXQudWNtLmVz