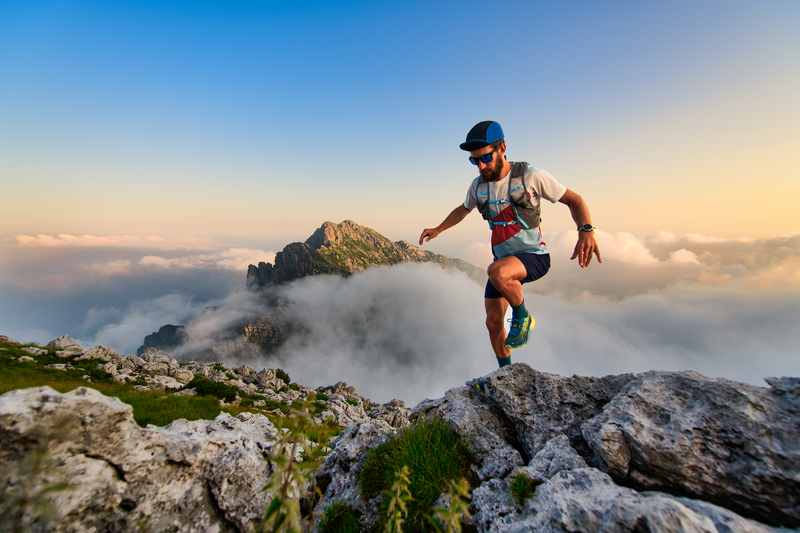
94% of researchers rate our articles as excellent or good
Learn more about the work of our research integrity team to safeguard the quality of each article we publish.
Find out more
ORIGINAL RESEARCH article
Front. Microbiol. , 17 October 2017
Sec. Microbial Physiology and Metabolism
Volume 8 - 2017 | https://doi.org/10.3389/fmicb.2017.02015
Transcriptional factors (TFs) play important roles in the responses to oxidative, acid, and other environmental stresses in Gram-positive bacteria, but the regulatory mechanism of TFs involved in oxidative stress remains unknown in lactic acid bacteria. In the present work, homologous overexpression strains with 43 TFs were constructed in the Lactobacillus plantarum CAUH2 parent strain. The strain overexpressing CopR displayed the highest sensitivity and a 110-fold decrease in survival rate under H2O2 challenge. The importance of CopR in the response to H2O2 stress was further confirmed by a 10.8-fold increase in the survival of a copR insertion mutant. In silico analysis of the genes flanking copR revealed putative CopR-binding “cop box” sequences in the promoter region of the adjacent gene copB encoding a Cu2+-exporting ATPase. Electrophoretic mobility shift assay (EMSA) analysis demonstrated the specific binding of CopR with copB in vitro, suggesting copB is a target gene of CopR in L. plantarum. The role of CopB involved in oxidative stress was verified by the significantly decreased survival in the copB mutant. Furthermore, a growth defect in copper-containing medium demonstrated that CopB functions as an export ATPase for copper ions. Furthermore, EMSAs revealed that CopR functions as a regulator that negatively regulates copB gene and Cu2+ serves as inducer of CopR to activate the expression of CopB in response to H2O2 stress in L. plantarum CAUH2. Our findings indicated that CopR plays an important role in enhancing oxidative resistance by regulating copB to modulate copper homeostasis.
Lactobacillus plantarum is an aerotolerant Gram-positive bacteria belonging to the facultatively heterofermentative group of lactobacilli (Kleerebezem et al., 2003). Among lactic acid bacteria (LAB), this species has one of the largest genomes and a particularly high proportion of regulatory genes, allowing it to survive in a variety of different environmental niches. Consequently, L. plantarum is widely used as a starter culture in fermented food and feed production, such as dairy, meat, and many vegetables and plants (Leroy and De Vuyst, 2004). Moreover, some L. plantarum strains have been declared to have health-promoting effects, such as increasing iron absorption and enhancing immunity, and thus can also function as probiotic active ingredients in fermented foods (Hoppe et al., 2015; Hulst et al., 2015). However, L. plantarum suffers from unavoidable oxidative stress caused by reactive oxygen species (ROS) during manufacturing. ROS, including the superoxide anion (), hydrogen peroxide (H2O2), the hydroxyl radical (HO•), and organic hydroperoxides (ROOH), can cause significant damage to various cellular structures such as DNA, proteins, and cell membranes (Imlay and Linn, 1988; Storz et al., 1990; Farr and Kogoma, 1991; Atack and Kelly, 2009). Therefore, L. plantarum has developed a variety of adaptive mechanism to cope with oxidative stress.
Bacterial cells respond to oxidative stress by inducing the expression of anti-oxidant enzymes. These mainly include superoxide dismutase (SOD), catalase, peroxidase, and glutathione peroxidase (Harris, 1992). However, L. plantarum lacks a SOD enzyme, and instead this bacterium accumulates a high intracellular concentration (20-30 mM) of manganese to scavenge superoxide and converts it to H2O2 during fermentative and aerobic growth (Archibald and Fridovich, 1981). In addition, the mannose phosphotransferase system (PTS) can enhance oxidative tolerance by transporting glucose to generate more ATP in L. plantarum WCFS1 (Stevens et al., 2010). Moreover, overexpression of the trxB1 gene-encoding thioredoxin reductase in L. plantarum WCFS1 can also enhance H2O2 tolerance by inducing a group of 16 gene transcripts involved in purine biosynthesis, cell wall biosynthesis, energy metabolism, cellular envelope biosynthesis, and amino acid metabolism (Serrano et al., 2007).
In LAB, a few transcriptional factors (TFs) have been identified as regulators to modulate gene expression in various physiological processes. In L. reuteri, the PocR-like TF controls the expression of its neighboring loci that harbors the genes for glycerol utilization and vitamin B12 synthesis (Santos et al., 2011). A TerR-like TF (lp_1153) functions as a repressor of the tarIJKL locus involved in teichoic acid synthesis in L. plantarum WCFS1 (Tomita et al., 2015). In addition, TF Ldb0677 has been identified to involve in acid tolerance, which was predicted to regulate 22 target genes by bacterial one-hybrid method in L. bulgaricus CAUH1 (Zhai et al., 2014). Furthermore, the copper homeostasis system controlled by the CopY-type repressor has been extensively studied in Enterococcus hirae and Lactococcus lactis (Solioz et al., 2010). The core element of this system in E. hirae is the cop operon encoding four proteins: the repressor CopY, copper chaperone CopZ, copper import ATPases CopA, and copper export ATPases CopB. Transcription of this operon is controlled by CopY through binding to the “cop box” in the promoter region. However, the corresponding core element in L. lactis contained a copRZA operon and a monocistronic copB, which were both controlled by CopR. CopA was identified as a copper-exporting ATPase while function of CopB is unclear (Solioz et al., 2011). In addition, another CopR regulon lctO encoding an NAD-independent lactate oxidase plays an antioxidant role by attenuating the formation of reactive oxygen radicals in L. lactis (Barré et al., 2007).
Lactobacillus plantarum CAUH2 was isolated from Szechuan pickle, a traditionally fermented vegetable product from China. Whole-genome sequencing indicated that CAUH2 contained about 8.5% regulatory genes (GenBank accession no. CPO15126–CPO15129). Furthermore, 45 isogenous genes of TF with known functions were identified in the CAUH2 genome. However, the roles of these TFs in H2O2 resistance remain unknown. In the present study, homologous overexpression of 43 TF genes in L. plantarum CAUH2 was performed to identify potential involvement in H2O2-induced oxidative stress. Genetic and physical evidence revealed that the TF CopR plays an important role in enhancing oxidative resistance by negatively regulating copB to modulate copper homeostasis. To our knowledge, this is the first report of a TF involved in protection against oxidative stress in L. plantarum.
Bacterial strains and plasmids used in this study are listed in Table 1. L. plantarum CAUH2 cultures were incubated at 37°C in De Man Rogosa Sharp (MRS) medium, and L. lactis NZ9000 was grown at 30°C in GM17 (M17 broth supplemented with 0.5% w/v D-glucose). Escherichia coli cells were propagated aerobically at 37°C in Luria Bertani (LB) broth. When required, media were supplemented with the relevant antibiotics at the following concentrations: 25 μg mL-1 kanamycin, 25 μg mL-1 erythromycin for E. coli, 10 μg mL-1 chloramphenicol for L. lactis, 20 μg mL-1 chloramphenicol, and 10 μg mL-1 erythromycin for L. plantarum.
General molecular techniques including DNA electrophoresis analysis, recovery, and storage were performed using standard protocols (Sambrook et al., 1989). Miniprep plasmid isolation from both E. coli and L. plantarum was performed using the Plasmid Mini Kit I according to the manufacturer’s instructions (OMEGA Bio-tek Inc., Doraville, GA, United States). Genomic DNA from lactobacilli was prepared using the genomic DNA Extraction Kit (Tiangen, Beijing, China). Lysis of bacterial cells was carried out by adding lysozyme to TES buffer (50 mM Tris–HCl, 1 mM EDTA, 25% sucrose; pH 8.0) to a final concentration of 30 mg mL-1, and mixtures were incubated at 37°C for 1 h (Vandeguchte et al., 1989). Plasmids were introduced into E. coli DH5α using standard heat shock transformation, and electroporation was used for plasmid transfer into L. plantarum as described previously (Thompson and Collins, 1996). PCR products were amplified using Ex Taq polymerase (Takara, Dalian, China). DNA digestions with restriction endonucleases including NcoI, HindIII, KpnI, PstI, XbaI, and EcoRI were performed according to the supplier’s instructions (Takara, Dalian, China). DNA ligation was performed using the T4 DNA Ligation Kit (Thermo Fisher Scientific, Beijing, China) according to the manufacturer’s instructions. All primers and probes used in this study were synthesized by Sangon Biotech (Beijing, China). DNA sequencing was performed by Sangon Biotech and the results were further analyzed with the DNAMAN software package.
Using the Collection of Manually Curated Inferences of Regulons in Prokaryotic Genomes database1, 57 isogenous TF genes with known functions were identified in the genome of L. plantarum WCFS1. Comparative genomics analysis showed that only 45 of these were present in the genome of L. plantarum CAUH2. These predicted TF genes were amplified using specific primers (listed in Supplementary Table S1) and inserted into the corresponding sites of pSlpA8148, resulting in a series of pSlpA8148-derived plasmids (designated as pSlpA-TF01 to pSlpA-TF43). The recombinant plasmids were sequenced and transformed into L. plantarum CAUH2 to generate corresponding recombinant strains CAUH2-TF01 to CAUH2-TF43. Meanwhile, a control strain (CAUH2-pSlpA8148) was constructed by introducing the empty pSlp8148 vector into L. plantarum CAUH2. To further investigate whether the TFs were successfully expressed, 5 of the 43 strains were randomly selected and SDS–PAGE analysis of cultures was performed.
In order to determine the tolerance to H2O2 stress in the 43 L. plantarum recombinant strains, overnight cultures were inoculated into 10 mL of fresh MRS medium containing chloramphenicol. When cells reached an OD600 of 0.8, 1 mL of each culture was collected and centrifuged at 6000 × g for 2 min, then resuspended in the same volume of fresh MRS medium supplemented with 5 mM H2O2. After incubation for 30 min at 37°C, the number of colony-forming units per milliliter (CFU/mL) was determined by plating 10-fold serial dilutions on MRS agar containing chloramphenicol and incubating for 16 h at 37°C. Survival rates were calculated by dividing the CFU/mL values after H2O2 challenge by the value obtained immediately after resuspension. All results were obtained by at least three independent experiments and each biological replicate was performed in replicates.
To study the role of CopR in oxidative stress, a copR mutant of CAUH2 was constructed using the suicide plasmid pUC19EM. The EMr cassette was amplified from the plasmid pGM36e using primers EM-F and EM-R (Supplementary Table S2) and inserted into the PstI and HindIII sites of pUC19, resulting in the suicide plasmid pUC19EM. A 316 bp internal region of the copR gene was chosen as a homologous sequence and amplified using the primer pair copRHA-F and copRHA-R with flanking XbaI and EcoRI sites, respectively. The digested PCR product was ligated with the corresponding restriction sites of pUC19EM, and the recombinant plasmid, designated pUC19EMcopR, was introduced into L. plantarum by electroporation. Recombinants were selected for growth on MRS medium containing erythromycin. The inability to replicate plasmid pUC19EMcopR resulted in its integration into the copR gene region of the CAUH2 genome under erythromycin selection pressure. The resulting mutant was designated CAUH2ΔcopR. PCR was performed with forward primer EMT-F and reverse primer copRT-R to confirm integration of pUC19EMcopR at the correct genome locus. The primer EMT-F was designed according to the DNA sequence of erythromycin resistance genes (GenBank accession no. KM017875.1) and the primer copRT-R was designed according to the DNA sequence of CopR (A1F92_RS13920). Meanwhile, insertional inactivation of the melA gene was carried out by the same procedure to construct a control strain (CAUH2ΔmelA). The forward primer melAHA-F and the reverse primer melAHA-R were used to amplify the mutagenic fragments of melA. To confirm the integration at the correct locus, PCR was performed using primers EMT-F and melAT-R.
The interaction between CopR and the upstream sequence of CopB was verified by electrophoretic mobility shift assay (EMSA). Primers (copR-F and copRHis6-R) used for amplifying the CopR gene are listed in Supplementary Table S2. A His6 tag was introduced at the C-terminus of CopR for nickel affinity purification. The digested PCR product was inserted into pNZ8148 at the corresponding sites to obtain the recombinant plasmid pNZCopRHis6. This plasmid was introduced into L. lactis NZ9000, and recombinant CopRHis6 was expressed and purified using Ni Sepharose 6 Fast Flow media (GE Healthcare, Uppsala, Sweden). Purified CopRHis6 protein was analyzed by SDS–PAGE, and the final protein concentration was measured using a NanoDrop 2000 microspectrophotometer (NanoDrop Technologies, Wilmington, DE, United States).
Electrophoretic mobility shift assays were performed using the Lightshift Chemiluminescent EMSA Kit (Thermo Fisher Scientific, Rockford, IL, United States). DNA probes used for EMSA were obtained by annealing complementary oligos (EMSA copB-F and -R, Supplementary Table S2), which were biotin-labeled using the Biotin 3′-End DNA Labeling Kit (Thermo Scientific). Binding reactions (20 μL) contained 1× binding buffer, 50 ng μL-1 Poly dl-dC, 2.5% (v/v) glycerol, 0.05% (v/v) Nonidet P-40, 5 mM MgCl2, 20 fmol labeled probe, and 2.5 μg CopRHis6. In order to verify specific binding between protein and DNA, a 200-fold molar excess of unlabeled probe competitor (4 pmol; Supplementary Table S2) was added to the EMSA reaction mixture. In addition, another EMSAs were further performed under the different concentrations of Cu2+ (5, 10, 25, and 50 μM). Subsequent steps were carried out following the manufacturer’s instructions in both two assays.
Starter cultures of CAUH2ΔcopR, CAUH2ΔcopB, and CAUH2ΔmelA were grown overnight at 37°C in MRS medium and inoculated into 10 mL of fresh MRS culture medium containing 1.5 mM CuSO4 at 37°C for 6 h. The OD600 was determined at 60-min intervals. All results were obtained by at least three independent experiments and each biological replicate was performed in replicates.
Means and standard deviations were calculated. The Student’s t-test was performed to investigate statistical differences. Differences between samples with p-values < 0.05 were considered statistically significant.
Although 45 TFs were detected in the genome of CAUH2, only 43 TF genes were successfully inserted into the vector pslpA8148. Recombinant plasmids were sequenced and verified by aligning with the database genome sequence of CAUH2. The correct plasmids were designated pSlpA-TF01 to pSlpA-TF43. These plasmids were transformed into CAUH2 to generate recombination strains, designated CAUH2-TF01 to TF43. SDS–PAGE analysis of cultures from five randomly selected strains revealed the overproduction of 29, 34, 14, 21, and 15 kD proteins (Figure 1), which corresponded to the expected sizes of TreR1, GntR1, GlnR, FlrR, and CopR, respectively. These results confirmed the successful overexpression of the five TFs in L. plantarum CAUH2. We therefore extrapolated that the other TF genes would also be successfully overexpressed in the host strain L. plantarum CAUH2.
FIGURE 1. Analysis of TF overexpression in five randomly selected recombinant strains by SDS–PAGE. Cells were grown to the early logarithmic phase (OD600 ∼0.8), soluble extracts were boiled in 5× sample buffer, and analyzed by 12% denaturing SDS–PAGE. Lane 1, CAUH2-pSlp8148; lane 2, CAUH2-TF04; lane 3, CAUH2-TF17; lane 4, CAUH2-TF21; lane 5, CAUH2-TF32; lane 6, CAUH2-TF36. Red arrows indicate the proteins overexpressed in each sample.
To probe the possible roles of the overexpressed TFs in oxidative stress in CAUH2, survival experiments of all 43 recombination strains were performed exposured to 5 mM H2O2. The results showed that the survival rates of strains CAUH2-TF04, CAUH2-TF13, and CAUH2-TF36 were 3-, 10-, and 110-fold lower than that of the control strain, which harbored TFs TreR1, MntR, and CopR, respectively (p < 0.05; Figure 2). These three TFs were involved in trehalose utilization, manganese homeostasis, and copper homeostasis. However, survivals of the other 40 recombinant strains were not significantly different from the control strain. These results indicate that MntR, TreR, and CopR might play an important role in H2O2 tolerance in CAUH2. Overexpression of copR caused the largest decrease in survival of the 43 TFs, and the mechanism of this gene in oxidative stress was therefore further investigated in this study.
FIGURE 2. Sensitivity of partial TF overexpression strains to 5 mM H2O2. Survival rate was calculated as the ratio of the number of colonies obtained on MRS plates after and before H2O2 challenge. Data are averages from at least three independent experiments, and error bars indicate SD.
The copR mutant was constructed as shown in Figure 3. When chromosomal DNA from the CAUH2ΔcopR mutant was used as template for PCR, the expected 750 kb product was obtained, and sequencing revealed amplification of the expected fragments of the Emr and copR genes, confirming the correct integration of Emr into the chromosome of CAUH2 by a single crossover homologous recombination event. To eliminate the growing effects of Em selective pressure between copR mutant and wild strain, a melA gene mutant CAUH2ΔmelA was constructed as the control strain. The melA gene (A1F92_14365) encoding the α-galactosidase predominantly hydrolyzes the galactooligosaccharides. Thus, the inactivation of melA has no significant effects on CAUH2 cells growth in MRS media with glucose as the sole carbon source, which was also confirmed by the growth experiment (Supplementary Figure S1). Challenge assays demonstrated that the copR mutant showed significantly higher H2O2 resistance than the control strain, i.e., 1.3-, 10.8-, and 3.4-fold increase in survival when exposed to 5, 7.5, and 10 mM H2O2, respectively (p < 0.05; Figure 4). These results further confirmed that CopR is involved in H2O2 resistance in L. plantarum CAUH2.
FIGURE 3. Construction of the L. plantarum mutant strain CAUH2ΔcopR. Genes are represented by arrows, promoters are indicated by triangles, and the internal fragment of copR is represented by a solid red box. Chromosomal DNA is represented by black lines, plasmid DNA is represented by blue lines, and the red arrow indicates the PCR products amplified using the forward primer EMT-F and the reverse primer copRT-R.
FIGURE 4. Survivals of the CAUH2ΔcopR and CAUH2ΔcopB mutants following 5, 7.5, and 10 mM H2O2 challenge. Survival rates were calculated as the ratio of the number of colonies obtained on MRS plates after and before H2O2 challenge. Data are averages from three independent experiments, and error bars indicate SD.
Amino acid sequence alignments showed that CopR of CAUH2 shares 46 and 30% identities with E. hirae CopY (GenBank accession no. CAA86835.1) and L. lactis CopR (GenBank accession no. NP_266988), respectively. The N-terminus sequences for putative DNA binding (Magnani et al., 2008) and the C-terminus CXCX6CXC motif for putative copper binding (Strausak and Solioz, 1997) were conserved in these three CopY-type proteins (Figure 5A). According to genome sequence, the putative CopR-related genes in L. plantarum CAUH2 show a different organization from either E. hirae or L. lactis. Analysis of the regions flanking copR in CAUH2 genome revealed two divergent genes: A1F92_RS13925 encoding a hypothetical protein, and copB encoding a copper-translocating P-type ATPase. Moreover, homologs of the copA and copZ genes were also detected in the CAUH2 genome. These two genes are located 27 kb downstream of copB and form a polycistron. The promoter regions of copR, copB, A1F92_RS13925, and copZ were further searched, the cop box consensus sequence TACAnnTGTA was only detected in the copB promoter region. These results indicated that transcription of copB is under the control of CopR in CAUH2.
FIGURE 5. Alignment of CopR and CopB in L. plantarum CAUH2, E. hirae, and L. lactis IL1403. Alignments were obtained using ClustalX 2.1 (Larkin et al., 2007) with default settings and visualized in CLC Sequence Viewer 7.8.1. (A) Alignment of CopR repressors and (B) alignment of CopB proteins.
Further alignments indicated that CopB from L. plantarum shares 54 and 49% amino acid sequence identity with CopB from E. hirae and CopB from L. lactis. The CPH motif for putative transmembrane metal binding site and DKTGT motif for a phosphorylation domain were conserved in these three CopB proteins (Odermatt et al., 1993; Figure 5B). In addition, only one conserved histidine- and methionine-rich domain for putative metal binding was observed in N-terminus of CopB from CAUH2.
Since the copB gene was identified as a putative target of CopR, protein–DNA interactions were analyzed by EMSA. CopR with a C-terminal His tag was expressed in L. lactis NZ9000 and purified by affinity chromatography. SDS–PAGE revealed a single protein band with a molecular mass of about 15 kDa, suggesting the recombinant CopRHis6 was successfully expressed and purified (Figure 6A). The EMSA results indicated that the purified CopRHis6 bound to biotin-labeled copB probes, and retarded their mobility (Figure 6B). Assays were further performed using unlabeled probes as specific competitors. The specific shift was abolished, which indicated specific binding of CopRHis6 to the copB probe. These results indicated that CopR specifically binds to the predicted binding sites upstream of copB and thereby regulates copB transcription.
FIGURE 6. SDS–PAGE analysis of the purified CopRHis6 and specific binding of CopRHis6 to the predicted binding sites upstream of the copB gene. (A) SDS–PAGE analysis of the purified CopRHis6. Lanes 1 and 2, L. lactis NZCK and NZCopRHis6 with 10 ng ml-1 nisin induction; lanes 3 and 4, purified products of NZCK and NZCopRHis6. (B) EMSAs showing the specific interaction of CopR and the predicted binding sites upstream of copB. Binding reactions consisted of the following: lane 1, 20 fmol labeled probe alone; lane 2, 20 fmol labeled probe, and 2.5 μg CopRHis6; lane 3, 20 fmol labeled probe, 4 pmol unlabeled probe, and 2.5 μg CopRHis6.
In order to investigate the role of CopB in H2O2 stress, a copB mutant, designated CAUH2ΔcopB, was constructed by homologous recombination and subjected to challenge assays. When exposed to 5, 7.5, and 10 mM H2O2, the survival rate of CAUH2ΔcopB was 2.0-, 2.0-, and 4.0-fold lower than that of the control strain, respectively (p < 0.05; Figure 4). These results demonstrated that CopB plays a critical role in resistance to H2O2-induced oxidative stress in CAUH2.
Given that copper can produce hydroxyl radicals from H2O2, we speculated that CopB may be involved in resistance by exporting Cu2+ ions from cells. To confirm whether CopB participates in the protection of CAUH2 cells as a copper ATPase, CAUH2ΔcopB cells were grown in MRS media containing 1.5 mM Cu2+ for 6 h. The growth was clearly retarded compared to the control strain, and significantly less biomass was achieved (Figure 7). These results suggest that CopB improves oxidative tolerance by exporting copper ions as an ATPase in L. plantarum CAUH2.
FIGURE 7. Growth of the CAUH2ΔcopB mutant in MRS containing 1.5 mM CuSO4. All experiments included three biologically independent replicates. The results are means of biological triplicate samples.
Since sequence alignment of CopR revealed a potential copper binding site, it was speculated that Cu binding may affect its ability to bind cop boxes. EMSA analysis showed that the binding intensity of CopR with the probes became weak with an increasing concentration of copper, suggesting that the CopR can be released from the cop box in the promoter of CopB by Cu2+ (Figure 8). These results revealed that CopR functions as a regulator that negatively regulates copB gene and Cu2+ serves as inducer of CopR to activate the ATPase CopB to export cupric ions under H2O2 stress in L. plantarum CAUH2.
FIGURE 8. EMSAs showing the interaction of CopR and the predicted binding sites upstream of copB in the presence of different concentrations of Cu2+. Binding reactions consisted of the following: lane 1, 20 fmol labeled probe alone; lane 2, 20 fmol labeled probe and 2.5 μg CopRHis6; lane 3, 20 fmol labeled probe and 2.5 μg CopRHis6 and 5 μM Cu2+; lane 4, 20 fmol labeled probe, 2.5 μg CopRHis6, and 10 μM Cu2+; lane 5, 20 fmol labeled probe, 2.5 μg CopRHis6, and 20 μM Cu2+; and lane 6, 20 fmol labeled probe, 2.5 μg CopRHis6, and 50 μM Cu2+.
The two oxidation states of copper, Cu+ and Cu2+, allow its participation in essential redox reactions, but can also promote the formation of ROS that causes cellular damage (Karlin, 1993). Thus, copper homeostasis has to be tightly regulated to preclude toxic effects in cells (Vulpe and Packman, 1995; Linder and Hazegh-Azam, 1996). Previous studies demonstrated that Cu+ deficiency can lead to a decrease in enzyme activity for heme-dependent catalase and glutathione peroxidase in various tissues (Chen et al., 1994; Strain, 1994). Based on the results of our transcriptome analysis on the H2O2 stress response of L. plantarum CAUH2 (NCBI GEO Series accession no. GSE99096), the genes encoding heme-dependent catalase (A1F92_RS14705) and glutathione peroxidase (A1F92_RS00895) were up-regulated 2.27- and 6.23-fold, respectively, suggesting CAUH2 employs these two enzymes as primary intracellular H2O2 scavengers (Abriouel et al., 2004). To maintain the activity of these enzymes in the presence of H2O2, CAUH2 cells must increase copper uptake. In E. hirae, the extracellular reductase CorA supplies Cu+ for uptake by CopA (Solioz and Stoyanov, 2003). Correspondingly, the ndh2 gene encoding a membrane-anchored type-2 NADH dehydrogenase (A1F92_RS04560) that reduces Cu2+ to Cu+ was also detected in the CAUH2 genome (Rodriguez-Montelongo et al., 2006). Transcriptome analysis showed that the ndh2 gene and the copA gene were up-regulated 4- and 14-fold under H2O2 stress, respectively, which indicates an increased requirement for copper ions in CAUH2 to maintain the activity of catalase and glutathione peroxidase under H2O2 stress.
Intracellular copper ions are generally bound to proteins as a cofactor, but they can be released to catalyze the formation of highly reactive hydroxyl radicals. In CAUH2, a copper chaperone protein (A1F92_RS14195) maybe involved in intracellular copper homeostasis as a Cu storage protein (Coyle et al., 2002; Tapia et al., 2004). A previous study found that the total Cu binding capacity of this chaperone protein was decreased when it was oxidized (Fabisiak et al., 1999). Under oxidative stress conditions, free copper ions likely accumulate in CAUH2 and damage cellular components through the formation of deleterious hydroxyl radicals. Up-regulation of CopB could enhance oxidative resistance by lowering the intracellular concentration of copper ions and preventing the damaging Fenton reaction.
In order to clarify the mechanism of CopR involved in H2O2 stress response of L. plantarum, the regulation network of CopR was summarized in Figure 9. Under the H2O2 stress, CAUH2 cells enhance the uptaking of cuprous ions by the up-regulated expression of genes ndh2 and copA to maintain the activity of heme-dependent catalase and glutathione peroxidase, which detoxify the cells by transforming H2O2 into H2O and O2. Meanwhile, both the free cuprous ions and the cuprous ions binding to copper chaperones are oxidized into cupric ions by the intracellular H2O2. As an inducer, the accumulated Cu2+ binds to CopR to release it from the cop box in copB promoter. Then the accumulated CopB enhances the exporting of Cu2+ for avoiding damage caused by copper ions. Taken altogether, our results revealed the role of copper ATPase CopB and its regulator CopR in H2O2 tolerance, which is of great importance for exploring novel H2O2-detoxifying mechanisms in L. plantarum and other bacteria.
FIGURE 9. Illustration of the mechanism of CopR involved in oxidative response to H2O2 in L. plantarum CAUH2. “CAT” represents catalase, “GPX” represents glutathione peroxidase, “Chaperone” represents the copper chaperone protein encoded by gene A1F92_RS14195.
YH and YY designed the study. YY, JY, JL, QX, and TL performed the experiments. YY analyzed and evaluated the data. YY and YH wrote the manuscript. YH revised the manuscript. All authors read and approved the final manuscript.
This work was supported by the National Natural Sciences Foundation of China (Contract No. 21476250).
The authors declare that the research was conducted in the absence of any commercial or financial relationships that could be construed as a potential conflict of interest.
We thank Prof. Willem M. de Vos (Wageningen University) for the gift of L. lactis NZ9000 and plasmid pNZ8148.
The Supplementary Material for this article can be found online at: https://www.frontiersin.org/articles/10.3389/fmicb.2017.02015/full#supplementary-material
FIGURE S1 | Growth of wild-type strain CAUH2 and the mutants CAUH2ΔcopR, CAUH2ΔcopB, and CAUH2ΔmelA. CAUH2 was grown in MRS media, CAUH2ΔcopR, CAUH2ΔcopB, and CAUH2ΔmelA were grown in MRS media supplemented with 10 μg mL-1 erythromycin.
Abriouel, H., Herrmann, A., Starke, J., Yousif, N. M. K., Wijaya, A., Tauscher, B., et al. (2004). Cloning and heterologous expression of hematin-dependent catalase produced by Lactobacillus plantarum CNRZ 1228. Appl. Environ. Microbiol. 70, 603–606. doi: 10.1128/Aem.70.1.603-606.2004
Archibald, F. S., and Fridovich, I. (1981). Manganese and defenses against oxygen-toxicity in Lactobacillus plantarum. J. Bacteriol. 145, 442–451.
Atack, J. M., and Kelly, D. J. (2009). Oxidative stress in Campylobacter jejuni: responses, resistance and regulation. Future Microbiol. 4, 677–690. doi: 10.2217/Fmb.09.44
Barré, O., Mourlane, F., and Solioz, M. (2007). Copper induction of lactate oxidase of Lactococcus lactis: a novel metal stress response. J. Bacteriol. 189, 5947–5954. doi: 10.1128/JB.00576-07
Chen, Y., Saari, J. T., and Kang, Y. J. (1994). Weak antioxidant defenses make the heart a target for damage in copper-deficient rats. Free Radic. Biol. Med. 17, 529–536. doi: 10.1016/0891-5849(94)90092-2
Coyle, P., Philcox, J. C., Carey, L. C., and Rofe, A. M. (2002). Metallothionein: the multipurpose protein. Cell. Mol. Life Sci. 59, 627–647. doi: 10.1007/s00018-002-8454-2
deRuyter, P. G., Kuipers, O. P., and deVos, W. M. (1996). Controlled gene expression systems for Lactococcus lactis with the food-grade inducer nisin. Appl. Environ. Microbiol. 62, 3662–3667.
Fabisiak, J. P., Tyurin, V. A., Tyurina, Y. Y., Borisenko, G. G., Korotaeva, A., Pitt, B. R., et al. (1999). Redox regulation of copper-metallothionein. Arch. Biochem. Biophys. 363, 171–181. doi: 10.1006/abbi.1998.1077
Farr, S. B., and Kogoma, T. (1991). Oxidative stress responses in Escherichia coli and Salmonella typhimurium. Microbiol. Rev. 55, 561–585.
Hoppe, M., Onning, G., Berggren, A., and Hulthen, L. (2015). Probiotic strain Lactobacillus plantarum 299v increases iron absorption from 2 an iron-supplemented fruit drink: a double-isotope cross-over single-blind 3 study in women of reproductive age–ERRATUM. Br. J. Nutr. 114, 1195–1202. doi: 10.1017/S0007114515004250
Hulst, M., Gross, G., Liu, Y., Hoekman, A., Niewold, T., van der Meulen, J., et al. (2015). Oral administration of Lactobacillus plantarum 299v modulates gene expression in the ileum of pigs: prediction of crosstalk between intestinal immune cells and sub-mucosal adipocytes. Genes Nutr. 10, 10. doi: 10.1007/s12263-015-0461-7
Imlay, J. A., and Linn, S. (1988). DNA damage and oxygen radical toxicity. Science 240, 1302–1309. doi: 10.1126/science.3287616
Karlin, K. D. (1993). Metalloenzymes, structural motifs, and inorganic models. Science 261, 701–708. doi: 10.1126/science.7688141
Kleerebezem, M., Boekhorst, J., van Kranenburg, R., Molenaar, D., Kuipers, O. P., Leer, R., et al. (2003). Complete genome sequence of Lactobacillus plantarum WCFS1. Proc. Natl. Acad. Sci. U.S.A. 100, 1990–1995. doi: 10.1073/pnas.0337704100
Larkin, M. A., Blackshields, G., Brown, N., Chenna, R., McGettigan, P. A., McWilliam, H., et al. (2007). Clustal W and Clustal X version 2.0. Bioinformatics 23, 2947–2948. doi: 10.1093/bioinformatics/btm404
Leroy, F., and De Vuyst, L. (2004). Lactic acid bacteria as functional starter cultures for the food fermentation industry. Trends Food Sci. Technol. 15, 67–78. doi: 10.1016/j.tifs.2003.09.004
Linder, M. C., and Hazegh-Azam, M. (1996). Copper biochemistry and molecular biology. Am. J. Clin. Nutr. 63, 797–811.
Magnani, D., Barre, O., Gerber, S. D., and Solioz, M. (2008). Characterization of the CopR regulon of Lactococcus lactis IL1403. J. Bacteriol. 190, 536–545. doi: 10.1128/Jb.01481-07
Odermatt, A., Suter, H., Krapf, R., and Solioz, M. (1993). Primary structure of 2 P-type ATPases involved in copper homeostasis in Enterococcus hairae. J. Biol. Chem. 268, 12775–12779.
Rodriguez-Montelongo, L., Volentini, S. I., Farias, R. N., Massa, E. M., and Rapisarda, V. A. (2006). The Cu(II)-reductase NADH dehydrogenase-2 of Escherichia coli improves the bacterial growth in extreme copper concentrations and increases the resistance to the damage caused by copper and hydroperoxide. Arch. Biochem. Biophys. 451, 1–7. doi: 10.1016/j.abb.2006.04.019
Sambrook, J., Fritsch, E. F., and Maniatis, T. (1989). Molecular Cloning: a Laboratory Manual. New York, NY: Cold spring harbor laboratory press.
Santos, F., Spinler, J. K., Saulnier, D. M., Molenaar, D., Teusink, B., Vos, W. M. D., et al. (2011). Functional identification in Lactobacillus reuteri of a PocR-like transcription factor regulating glycerol utilization and vitamin B12 synthesis. Microb. Cell Fact. 10:55. doi: 10.1186/1475-2859-10-55
Serrano, L. M., Molenaar, D., Wels, M., Teusink, B., Bron, P. A., de Vos, W. M., et al. (2007). Thioredoxin reductase is a key factor in the oxidative stress response of Lactobacillus plantarum WCFS1. Microb. Cell Fact. 6:29. doi: 10.1186/1475-2859-6-29
Solioz, M., Abicht, H. K., Mermod, M., and Mancini, S. (2010). Response of gram-positive bacteria to copper stress. J. Biol. Inorg. Chem. 15, 3–14. doi: 10.1007/s00775-009-0588-3
Solioz, M., Mermod, M., Abicht, H. K., and Mancini, S. (2011). Responses of Lactic Acid Bacteria to Heavy Metal Stress. Boston, MA: Springer.
Solioz, M., and Stoyanov, J. V. (2003). Copper homeostasis in Enterococcus hirae. FEMS Microbiol. Rev. 27, 183–195. doi: 10.1016/S0168-6445(03)00053-6
Stevens, M. J. A., Molenaar, D., de Jong, A., de Vos, W. M., and Kleerebezem, M. (2010). Involvement of the mannose phosphotransferase system of Lactobacillus plantarum WCFS1 in peroxide stress tolerance. Appl. Environ. Microbiol. 76, 3748–3752. doi: 10.1128/Aem.00073-10
Storz, G., Tartaglia, L. A., Farr, S. B., and Ames, B. N. (1990). Bacterial defenses against oxidative stress. Trends Genet. 6, 363–368. doi: 10.1016/0168-9525(90)90278-E
Strain, J. J. (1994). Newer aspects of micronutrients in chronic disease - copper. Proc. Nutr. Soc. 53, 583–598. doi: 10.1079/Pns19940067
Strausak, D., and Solioz, M. (1997). CopY is a copper-inducible repressor of the Enterococcus hirae copper ATPases. J. Biol. Chem. 272, 8932–8936. doi: 10.1074/jbc.272.14.8932
Tapia, L., Gonzalez-Aguero, M., Cisternas, M. F., Suazo, M., Cambiazo, V., Uauy, R., et al. (2004). Metallothionein is crucial for safe intracellular copper storage and cell survival at normal and supra-physiological exposure levels. Biochem. J. 378, 617–624. doi: 10.1042/Bj20031174
Thompson, K., and Collins, M. A. (1996). Improvement in electroporation efficiency for Lactobacillus plantarum by the inclusion of high concentrations of glycine in the growth medium. J. Microbiol. Methods 26, 73–79. doi: 10.1016/0167-7012(96)00845-7
Tomita, S., Lee, I. C., van Swam, I. I., Boeren, S., Vervoort, J., Bron, P. A., et al. (2015). Characterisation of the transcriptional regulation of the tarIJKL-locus involved in ribitol-containing wall teichoic acid biosynthesis in Lactobacillus plantarum. Microbiology 162, 420–432. doi: 10.1099/mic.0.000229
Vandeguchte, M., Vandervossen, J. M. B. M., Kok, J., and Venema, G. (1989). Construction of a lactococcal expression vector - expression of hen egg-white lysozyme in Lactococcus lactis subsp Lactis. Appl. Environ. Microbiol. 55, 224–228.
Vieira, J., and Messing, J. (1982). The Puc Plasmids, an M13mp7-derived system for insertion mutagenesis and sequencing with synthetic universal primers. Gene 19, 259–268. doi: 10.1016/0378-1119(82)90015-4
Vulpe, C. D., and Packman, S. (1995). Cellular copper transport. Annu. Rev. Nutr. 15, 293–322. doi: 10.1146/annurev.nu.15.070195.001453
Zhai, Z., Douillard, F. P., An, H., Wang, G., Guo, X., Luo, Y., et al. (2014). Proteomic characterization of the acid tolerance response in Lactobacillus delbrueckii subsp. bulgaricus CAUH1 and functional identification of a novel acid stress-related transcriptional regulator Ldb0677. Environ. Microbiol. 16, 1524–1537. doi: 10.1111/1462-2920.12280
Keywords: Lactobacillus plantarum CAUH2, H2O2 stress, CopR, Cu2+-exporting ATPase, copper homeostasis
Citation: Yang Y, Yin J, Liu J, Xu Q, Lan T, Ren F and Hao Y (2017) The Copper Homeostasis Transcription Factor CopR Is Involved in H2O2 Stress in Lactobacillus plantarum CAUH2. Front. Microbiol. 8:2015. doi: 10.3389/fmicb.2017.02015
Received: 24 May 2017; Accepted: 29 September 2017;
Published: 17 October 2017.
Edited by:
Michael Sauer, University of Natural Resources and Life Sciences, Vienna, AustriaReviewed by:
Armen Trchounian, Yerevan State University, ArmeniaCopyright © 2017 Yang, Yin, Liu, Xu, Lan, Ren and Hao. This is an open-access article distributed under the terms of the Creative Commons Attribution License (CC BY). The use, distribution or reproduction in other forums is permitted, provided the original author(s) or licensor are credited and that the original publication in this journal is cited, in accordance with accepted academic practice. No use, distribution or reproduction is permitted which does not comply with these terms.
*Correspondence: Yanling Hao, haoyl@cau.edu.cn
Disclaimer: All claims expressed in this article are solely those of the authors and do not necessarily represent those of their affiliated organizations, or those of the publisher, the editors and the reviewers. Any product that may be evaluated in this article or claim that may be made by its manufacturer is not guaranteed or endorsed by the publisher.
Research integrity at Frontiers
Learn more about the work of our research integrity team to safeguard the quality of each article we publish.