- Section for Experimental Animal Models, Department of Veterinary and Animal Science, Faculty of Health and Medical Science, University of Copenhagen, Frederiksberg, Denmark
Bacterial biofilm formation is one of the main reasons for a negative treatment outcome and a high recurrence rate for many chronic infections in humans. The optimal way to study both the biofilm forming bacteria and the host response simultaneously is by using discriminative, reliable, and reproducible animal models of the infections. In this review, the advantages of in vivo studies are compared to in vitro studies of biofilm formation in infectious diseases. The pig is the animal of choice when developing and applying large animal models of infectious diseases due to its similarity of anatomy, physiology, and immune system to humans. Furthermore, conventional pigs spontaneously develop many of the same chronic bacterial infections as seen in humans. Therefore, in this review porcine models of five different infectious diseases all associated with biofilm formation and chronicity in humans are described. The infectious diseases are: chronic wounds, endocarditis, pyelonephritis, hematogenous osteomyelitis, and implant-associated osteomyelitis (IAO).
Introduction
Chronic bacterial infections are a major healthcare problem and of increasing concern due to their high burden with respect to economic costs, increased bacterial antibiotic resistance, high morbidity, and mortality (Archer et al., 2011; Roberts et al., 2015). Biofilm forming bacteria have increased tolerance toward antimicrobials as well as mechanical removal and are one of the reasons why chronic bacterial infections are difficult to treat (Costerton et al., 1999; Stewart, 2014). In order to study bacterial biofilms in chronic infections, it is important to have discriminative and reproducible animal models (Costerton et al., 1999; Bjarnsholt, 2013). The pig resembles humans anatomically, physiologically and immunologically. Furthermore, conventional pigs spontaneously (naturally occurring infections) develop many of the same chronic bacterial infections as seen in humans (Harris and Alexander, 1999). Therefore, it is possible to induce the disease experimentally in a discriminative way making the pig a reliable model for the study of biofilm related infectious diseases. This is not the case for many spontaneous infections in rodents, which do not adequately represent features of human disorders. In general, the use of pigs as experimental animals is intensely increasing and it is reasonable to expect that more porcine models of bacterial biofilm infections will be developed in the future. Therefore, the aim of the present paper is to review and describe existing porcine models of bacterial biofilm infections in humans with special focus on pathomorphology.
Biofilm
Biofilm was described by Burmølle et al. (2010) as: “A coherent cluster of bacterial cells imbedded in a biopolymer matrix, which, compared with planktonic cells, shows increased tolerance to antimicrobials and resists the antimicrobial properties of the host defense” (Burmølle et al., 2010).
In other descriptions of biofilm it is stated that either an abiotic or a biotic surface must be present for biofilm to form (Costerton et al., 1995, 1999; Davey and O'toole, 2000; Flemming and Wingender, 2010). It has however, also been suggested that biofilm does not need a surface in order to be established, as bacteria may attach to each other and form biofilm (Donlan and Costerton, 2002; Archer et al., 2011; Bjarnsholt, 2013). Another important characteristic of biofilm, is that the bacteria change phenotypic expression in regard to growth, gene expression and protein synthesis (Costerton et al., 1995, 1999, 2003; Davey and O'toole, 2000; Brady et al., 2008; Burmølle et al., 2010). In a study by Costerton et al. (2003), it was observed that all chronic bacterial diseases examined during a 12 year period, contained biofilm (Costerton et al., 2003). Moreover, it has been recommended that all refractory chronic bacterial diseases should be analyzed for the presence of biofilm (Donlan and Costerton, 2002).
Bacteria form biofilm in a number of situations: (1) it can be a defense mechanism when bacteria are in a hostile environment, (2) it can serve as a favorable habitat, if the bacteria are in an environment with a low amount of nutrients and (3) it can result from a mutation or a so called default mode of the bacteria (Bjarnsholt, 2013).
The initiation of biofilm formation is usually mediated by flagella and/or pili (Costerton et al., 1999). After adhering to other bacteria and/or a surface, a monolayer of bacteria is formed and develops into micro-colonies (Costerton et al., 1999). Following formation of micro-colonies the extracellular matrix is formed (Costerton et al., 1999). The matrix, which can be formed within 48 h after infection, is usually produced by the bacteria (Davis et al., 2007; Bjarnsholt, 2013). However, sometimes host components are also embedded within biofilm (Bjarnsholt, 2013; Stewart, 2014). The matrix consists of several components; proteins, lipids, extracellular DNA, polysaccharides, and other bacterial macromolecular components. Moreover, it has been realized that different bacteria are embedded in different components of biofilm matrix (Bjarnsholt, 2013).
An increased tolerance of biofilm forming bacteria is seen toward antimicrobials which may be due to a number of factors. Matrix may possess the ability to bind and inactivate antibacterial agents (Bjarnsholt, 2013). Furthermore, bacteria growing as a biofilm are less metabolically active, due to the decreased amounts of nutrients available, making them more tolerant to several antimicrobials (Bjarnsholt, 2013). In addition, bacteria in biofilm also show increased tolerance toward the host's immune system (Bjarnsholt, 2013; Stewart, 2014). The mechanism behind this has not been fully elucidated, but it appears that the presence of biofilm constantly stimulates polymorphonuclear leucocytes (PMNs). PMNs are, however, not able to phagocytize biofilm, presumably due to the size of the biofilm, leading to the phenomenon “frustrated phagocytosis” (Bjarnsholt, 2013; Stewart, 2014). The role of the adaptive immune response has not been fully clarified with respect to biofilm. Although, in Staphylococcus aureus biofilm it has been realized that several factors inhibits the activation and effects of the adaptive immune response (Kim et al., 2012).
Several methods have been applied in order to demonstrate biofilm formation and function, in relation to different infectious diseases (Lebeaux et al., 2013). It has been proven difficult to culture biofilm embedded bacteria and some samples may need ultra-sonication before cultivation (Burmølle et al., 2010). Other methods used to identify biofilm embedded bacteria include peptide nucleic acid fluorescence in situ hybridization (PNA FISH; Figure 1B), electron microscopy, immunohistochemistry staining, and confocal scanning laser microscopy (CSLM; Costerton et al., 1999; Bjarnsholt, 2013). CSLM is becoming the method of choice, as it enables analysis of a fully hydrated and living biofilm in situ (Figures 1C,D; Costerton et al., 1995; Kirketerp-Møller et al., 2008).
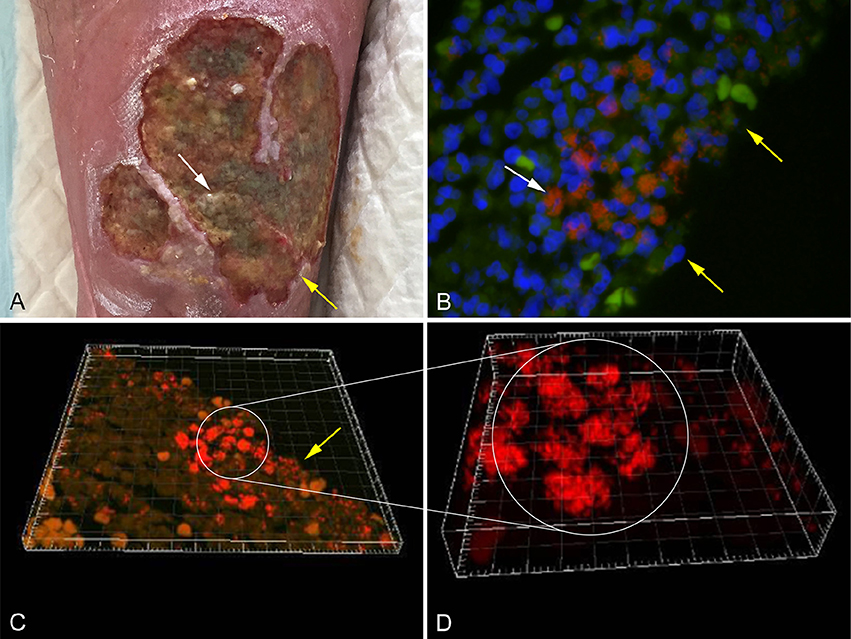
Figure 1. (A) Chronic venous leg ulcer. (B) Biofilm of P. aeruginosa [red stain] and S. aureus [green stain], identified by specific PNA FISH probes, surrounded by host cells (DAPI [blue stain]) in a human chronic wound. (C) CSLM three dimensional imaging of picture B. (D) Enlargement of picture C. The white arrows point to bacterial aggregates and the yellow arrows point to the wound surface (Kirketerp-Møller et al., 2008).
Biofilm in Vitro and in Vivo
Several approaches have been applied to study the complexity of bacterial biofilms. In vitro experiments were used in the early studies of biofilm and much knowledge about biofilm physiology and formation has been achieved using in vitro models (Bjarnsholt et al., 2013; Lebeaux et al., 2013). However, in vitro and also ex-vivo (Yang et al., 2017) studies have to be supplemented with in vivo studies if the response of the immune system toward bacterial biofilm infections is investigated (Rumbaugh and Carty, 2011; Roberts et al., 2015). This was also recently demonstrated with references to porcine infections due to Actinobacillus pleuropneumoniea (Tremblay et al., 2017).
Biofilm formation in vitro and in vivo is significantly different with respect to a number of characteristics (Bjarnsholt et al., 2013). In vitro biofilm of Pseudomonas aeruginosa forms “mushroom” like structures (Bjarnsholt, 2013; Ghanbari et al., 2016), which are not observed in vivo (Bjarnsholt et al., 2013). Another important difference is that in vitro biofilm is solely made up by bacterial derived components, whereas in vivo biofilm contains a mixture of bacterial and host derived elements (Bjarnsholt, 2013; Stewart, 2014). The size of biofilm formations is also different in vitro compared to in vivo. In vivo biofilms have a maximum diameter of 200 μm, whereas in vitro biofilm can reach up to several centimeters (Bjarnsholt et al., 2013). The limitation in size has been suggested to be related to oxygen depletion in the local environment (Roberts et al., 2015). Finally, the infectious biofilm formation in vivo, also enables the study of the host immune response toward biofilm (Coenye and Nelis, 2010). The pros and cons for in vitro and in vivo studies of bacterial biofilms are shown in Table 1 (Lebeaux et al., 2013).
Comparative Anatomy, Physiology, and Immunology of Pigs and Humans
Several different animal models have been applied in order to study biofilm in vivo. In relation to chronic bacterial diseases, mice, rats, and rabbits have been used most frequently (Rumbaugh and Carty, 2011). Only a few large animal models using pigs, sheep, goats, monkeys, and dogs have been developed (Rumbaugh and Carty, 2011). In recent years, the use of pigs as experimental animals has increased. Pigs have especially been applied in studies of toxicity, metabolism, cancer, dermatology, cardiology, and neurology (Swindle et al., 2012). However, during the last ten years several porcine models of bacterial diseases have been developed (Isling et al., 2011; Meurens et al., 2012; Christiansen et al., 2013b; Jensen et al., 2017). Figures 2, 3 shows the diversity of the pig as a model for human bacterial diseases. An advantage of using pigs as a model for bacterial infections is their immune response, which is quite comparable to that of humans (Meurens et al., 2012). In a study comparing the porcine, and human genome it was found that there is a 78% similarity, both structurally and functionally, between human and porcine immune related proteins (Dawson, 2011). The population of immune cells in humans and pigs is also alike. As in humans, pigs have a large percentage of PMNs in the peripheral blood (Meurens et al., 2012).
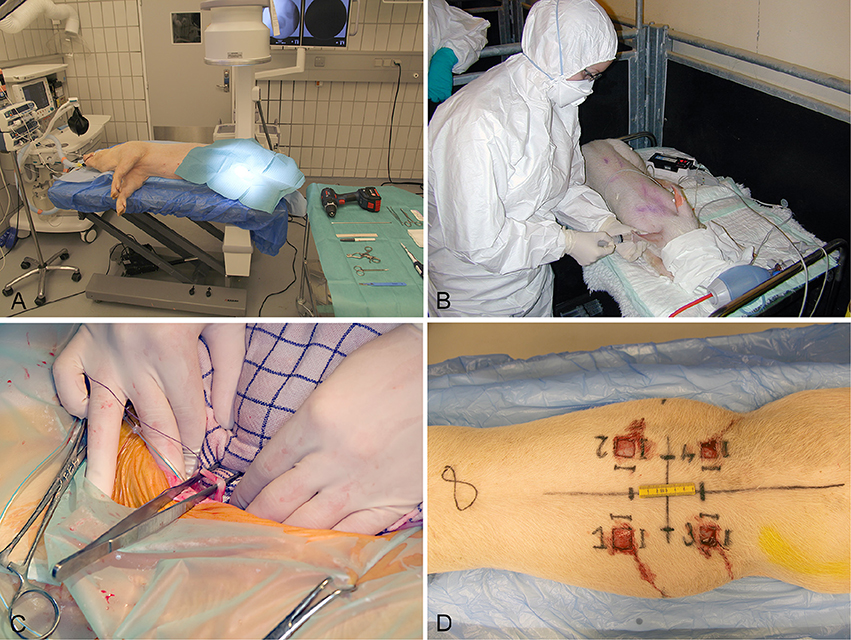
Figure 2. Establishment of four different models in conventional pigs of 30 kg. (A) Implant associated osteomyelitis, a drill hole is created in the right tibia followed by injection of bacteria and insertion of a small metal implant (Jensen et al., 2017). (B) Intravenous inoculation of bacteria for induction of endocarditis. Four days prior to inoculation, a permanent catheter was placed in the left ventricle (Christiansen et al., 2013b). (C) Free dissection of the right ureter, followed by insertion of a catheter used for inoculation of bacteria directly in the renal pelvis (Isling et al., 2011). (D) Four wounds created on the back at different time intervals for bacterial inoculation.
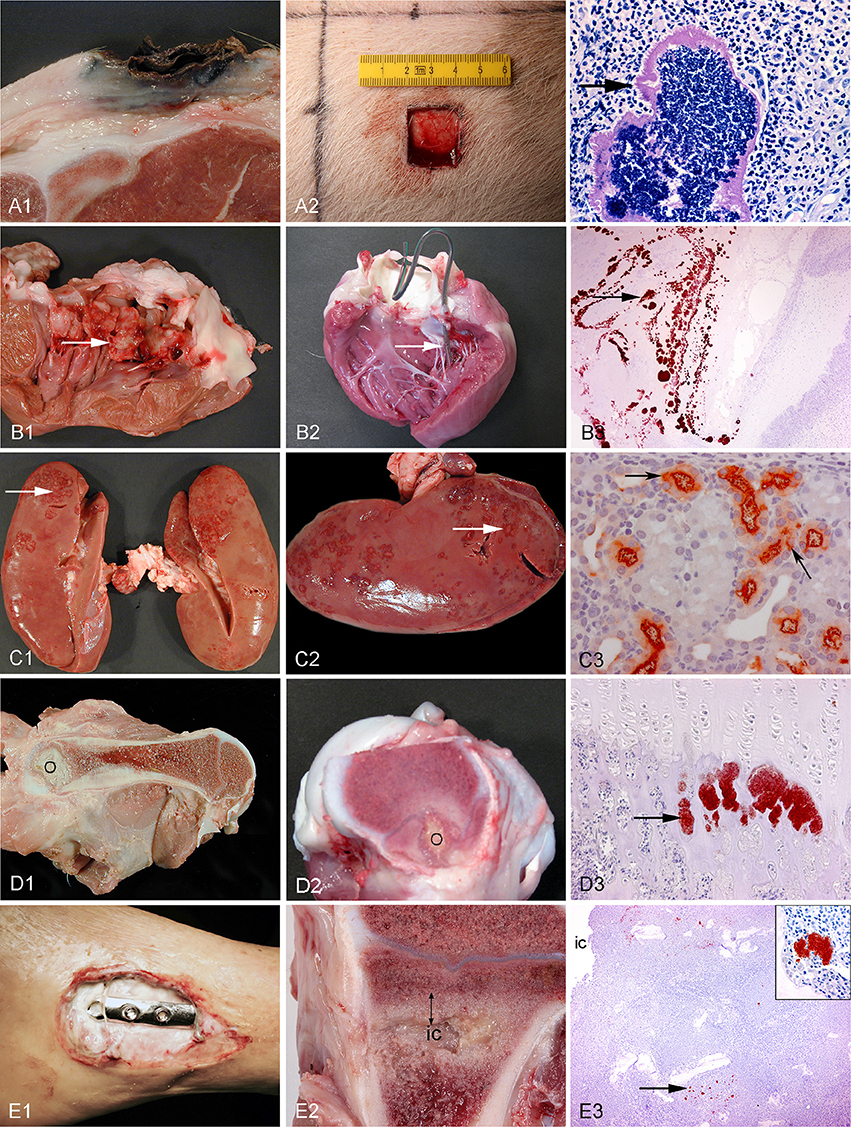
Figure 3. Left column: chronic spontaneous bacterial infections in conventional pigs (A–D) and a human (E). Middle column: experimental porcine models of human infections. Right column (except from picture A3): microscopy of the lesions present in the middle column. Row A: Chronic wounds. A1: Shoulder ulceration. A2: wound located on the back. A3: Bacteria (arrow) in a shoulder ulceration from a conventional pig. Row B: Endocarditis. B1: Left side, thrombotic valvular endocarditis (arrow). B2: A permanent catheter (arrow) inserted into the left ventricle prior to inoculation of bacteria. B3: Immunohistochemical staining of S. aureus (arrow) on the mitral valve (Christiansen et al., 2013b). Row C: Pyelonephritis. C1, C2: Polar located lesions of pyelonephritis (arrows). C3: Immunohistochemical staining of E. coli (arrows) in the proximal tubuli (Isling et al., 2011). Row D: Hematogenous osteomyelitis. D1, D2: Purulent osteomyelitis (O) in the femur. D3: Immunohistochemical staining of S. aureus (arrow) located in the capillary loops of the metaphysis (Johansen et al., 2012b). Row E: Implant-associated osteomyelitis. E1: Infected osteo-syntheses of the ankle. E2: Peri-implant infected bone tissue (double arrow) surrounding the implant cavity (ic), the implant has been removed. E3: Immunohistochemical staining of S. aureus (arrow) and in the insert (Jensen et al., 2017).
Porcine models can be based on conventional pigs or mini-pigs of different breeds. The growth rate of conventional pigs is high (1 kg at birth, 100 kg at 4 months and the body weight of an adult is >200 kg) compared to mini-pigs (0.5 kg at birth, 12–14 kg at 4 months and the body weight of an adult is 40–80 kg), making mini-pigs a more favorable model for adults (Swindle et al., 2012). Pigs are available as outbred and inbred. Using inbred pigs will allow a more uniform outcome of studies, in which the outbred pigs will allow the impact of biological diversity, just as in the human population (Meurens et al., 2012). Furthermore, the health status and full pedigree can usually be acquired for both conventional pigs and mini-pigs (Meurens et al., 2012). Min-pigs are available from Sinclair Bioresource (Hanford, Sinclair, Yucatan, Yucatan Micro) and Ellegaard Göttingen Minipigs (Göttingen Minipigs). Conventional pigs are supplied by conventional farmers.
Porcine Models of Chronic Bacterial Infectious Diseases
Pigs have been used to model the following chronic bacterial diseases, all known to be associated with biofilm formation in humans; chronic wounds, endocarditis, pyelonephritis, hematogenous osteomyelitis, and implant-associated osteomyelitis (IAO). In this review, these models will be described and the advantages of using pigs as a model for the five diseases will be elucidated with regard to how pigs are comparable to human's skin, heart, kidneys, and bones.
The inclusion and exclusion criteria for this review: The keywords “porcine model,” “biofilm,” “chronic wounds,” “endocarditis,” “pyelonephritis,” “hematogenous osteomyelitis,” “implant-associated osteomyelitis” were searched for in different combinations. The primary databases used were Google Scholar, REX, Web of Science and PubMed. The criteria which the studies had to fulfill in order to be enclosed in this review were as follows;
1. It had to be a study of chronic wounds, endocarditis, pyelonephritis, hematogenous osteomyelitis, or implant-associated osteomyelitis.
2. The study had to use a porcine model.
3. The pigs had to be inoculated with bacteria in order to develop infection.
Porcine Models of Chronic Wounds
Pigs are commonly applied in experimental wound studies (Figure 3A2). The skin of pigs is comparable to that of humans in a number of ways. Pig skin has little hair and is well attached to the subcutaneous layer, as in humans (Swindle and Smith, 1998). Wound healing in pigs has been found to be similar to that of humans (Sullivan et al., 2001). The major differences are the cutaneous blood supply as well as the thickness of the skin (Swindle and Smith, 1998; Liu et al., 2010). Although the thickness of the skin is greater in pigs, the ratio between dermis and epidermis is similar to that of humans (Meurens et al., 2012). The subcutis in pigs is divided into three different layers, which are all separated by fascia (Driskell et al., 2014). In humans, the subcutis is only divided into two layers (Driskell et al., 2014). Finally, the sweat glands in porcine skin are all apocrine, whereas in humans eccrine sweat glands are dominating (Liu et al., 2010).
The pig is suitable as a model for chronic wounds due to its similarity to humans with respect to the structure of the skin, but also because chronic wounds regularly are found in conventional pigs. Accidentally occurring chronic wounds may be found all over the skin of pigs; however, In recent years focus has been on shoulder ulcerations in sows (Figure 3A1). Shoulder ulcerations in pigs are caused by pressure and the pathogenesis is a progression of damage from the top and down through the dermal layers (Maxie, 2007; Jensen, 2009; Schomberg et al., 2016). This pathogenesis is also seen in humans, however, a “reversed” pathogenesis, in which the progression can be from the inside and out is also seen in humans (Anderson, 1976). A major difference in the pathogenesis of human pressure ulceration is that they can be complicated by osteitis/osteomyelitis, which has not been associated with pressure ulcers in pigs (Jensen, 2009). Another important difference between pressure ulcers in humans and pigs is that in pigs, they occur in otherwise healthy individuals, whereas in humans they usually develop due to an underlying disease (Dahl-Pedersen et al., 2013). In humans, the most important pathogen in chronic wounds is S. aureus (Bowler, 2002), whereas Trueperella pyogenes is the most common pathogen in shoulder ulcerations of sows (Lund, 2003; Dahl-Pedersen et al., 2013). Along with pressure ulcers, there are two other types of chronic wounds, which dominate in humans; diabetic ulcers and venous ulcers (Figure 1A; Medina et al., 2005). Although diabetic and venous ulcers do not occur in pigs under natural circumstances, the pig has been used as a model for diabetic wounds (Seaton et al., 2015). Porcine models of burn wounds and hypertrophic scars have also been developed (Seaton et al., 2015). In 2003 Breuing et al. established a partial thickness burn wound model with S. aureus as inoculum (Table 2). They used a chamber to cover the burn wound, by which it was possible to study the wounds continuously (Breuing et al., 2003). Davis et al. (2007) created a partial thickness wound using an electrokeratome, which was also inoculated with S. aureus. By using electron microscopy and epifluorescence microscopy (Table 2), they demonstrated the formation of biofilm after 48 h. Furthermore, they also showed that biofilm could not be eradicated with the antibiotics tested (Davis et al., 2007). A novel full thickness diabetic wound model developed by Hirsch et al. (2008) showed that diabetic pigs maintained a significant infection compared to the non-diabetic pigs (Table 2). Furthermore, a significant delay in wound healing was found in the diabetic wounds (Hirsch et al., 2008). A full thickness wound model by Roche et al. (2012; Table 2), showed that biofilm formation resulted in delayed healing (Roche et al., 2012). Nusbaum et al. (2012) inoculated S. aureus into a deep dermal wound model (Table 2) in order to study the effect of different types of wound debridement: plasma-mediated bipolar radiofrequency ablation, hydrosurgery system, and sharp debridement. They achieved a significant reduction of bacteria in all debridement groups and a significant reduction of S. aureus in the plasma-mediated bipolar radiofrequency ablation groups (Nusbaum et al., 2012). Finally, a full thickness porcine wound model was established using P. aeruginosa as inoculum (Table 2). In the model, the effect of different therapeutical strategies using negative pressure wound therapy was evaluated (Davis et al., 2013).
Porcine Models of Endocarditis
Due to porcine similarities in the cardiovascular system, pigs have been used as a model in a number of cardiac studies, e.g., transplantation, experimental atherosclerosis, and endocarditis (Swindle et al., 2012; Schomberg et al., 2016). The hemodynamics of the porcine cardiovascular system is similar to humans, however, there are differences between different breeds and ages of pigs (Swindle and Smith, 1998; Swindle et al., 2012). In contrast to humans, pigs have a large left azygos (hemiazygos) vein, which enters into the coronary sinus, instead of the superior vena cava (Swindle and Smith, 1998; Swindle et al., 2012). Another difference is that the semi-lunar valves in pigs are slightly smaller compared to that of humans (Ibrahim et al., 2006). The hearts of pigs come in many different sizes, however, the heart of a mini-pig is equivalent to about 0.3–0.5% of the total bodyweight, thus, a mini-pig of about 40–50 kg, has a heart of similar size as an adult human (Swindle and Smith, 1998).
Endocarditis occurs spontaneously in conventional pigs, and has been characterized, both histopathologically and microbiologically (Jensen et al., 2010a). In pigs, the lesions of endocarditis are mainly located on the mitral valves, sometimes with secondary lesions in the adjacent mural wall (Figure 3B1). Histopathologically, the vegetation is generally made up of granulation tissue, surrounded by fibrin. However, vegetation made up of granulomatous inflammation with mineralization may be seen, particularly in Streptococcus infections (Jensen et al., 2010a). Others have described the vegetation found in porcine endocarditis as “Cauliflower-like” (Geissinger et al., 1973). In humans, the appearance of the lesion is similar to that of pigs. The lesions can be quite large and are also made up by fibrin, and the embedded bacteria are surrounded by leukocytes and granulation tissue (Anderson, 1976). The most common infectious agents of porcine endocarditis are S. suis and Erysipelothrix rhusiopathiae (Jensen et al., 2010a). In contrast, the most important pathogen in humans is S. aureus (Murdoch et al., 2009). The pathogenesis in pigs has not been established, however, it is believed that there has to be a persistent or recurrent bacteremia (Maxie, 2007). In humans, infectious endocarditis mainly develops secondary to a state of non-bacterial thrombotic endocarditis (Christiansen et al., 2013b).
The first porcine model of endocarditis was described by Jones (1969) who established a non-traumatic endocarditis model, in which four different strains of Streptococci were inoculated intravenously. The strains were from Lancefield group C and L, respectively. Strain S85 (Group L), resulted in endocarditis in the pigs and was used in his further studies (Table 3; Jones, 1969, 1981, 1982). He found that macroscopic lesions developed as early as 18 h after inoculation and that the lesions in general matched those seen in humans (Jones, 1969). Geissinger et al. (1973) did a study using both conventional and gnotobiotic pigs, where they inoculated the bacteria sub-cutaneously. In that study, they also used two different strains of S. aureus (A and B) as well as one strain of E. rhusiopathiae. The study showed that strain A of S. aureus, was the only one which resulted in endocarditis in both conventional and gnotobiotic pigs (Geissinger et al., 1973). In another study by Jones (1981), he examined the lesions 3–14 days after inoculation, using the same experimental procedure as in 1969 (Jones, 1981). Finally, Jones examined the development of lesions 18–48 h after inoculation. The lesions were again macroscopically visible 18 h after inoculation (Jones, 1982). In 1986, Johnson et al. tried to reproduce the findings of Jones (Jones, 1969, 1981, 1982), however, only 11% of the pigs developed endocarditis (Johnson et al., 1986). Interestingly, insertion of a non-permanent catheter through the carotid artery and into the left ventricle followed by intravenous inoculation of Streptococci (Group C) resulted in endocarditis in 94% of the pigs (Johnson et al., 1986). Another study using catheterization was done by Dewar et al. (1987) who used mini-pigs and inoculations of S. sanguis (Group H), which resulted in the development of endocarditis in 75% of the pigs (Dewar et al., 1987). Recently, two studies were carried out by Christiansen et al. (2013a,b). In these studies a permanent catheter was also placed through the carotid artery and into the left side of the heart. The bacteria inoculated were two strains of S. aureus which were isolated from a pig and a human, respectively. The porcine strain produced endocarditis in both studies, using an inoculum dose of 107 CFU/ml, whereas infection by the human strain failed to induce endocarditis (Figures 3B2,B3; Christiansen et al., 2013a,b).
Porcine Models of Pyelonephritis
The kidneys of pigs are anatomically quite similar to those of humans as both have multirenculate and multipapillate kidneys. Another similarity between pigs and humans is the glomerular filtration rate (Ibrahim et al., 2006). An important difference is related to the vascularity of the kidneys. In pigs the vascular plane is parted transversely and not longitudinally as in humans (Swindle et al., 2012). The similarities have allowed the development of vesicourethral and intrarenal reflux models in pigs (Swindle and Smith, 1998).
In humans, the chronic form of pyelonephritis is usually caused by urinary reflux and urethral obstruction (Damjanov and Linder, 1996). The most common pathogens involved are Escherichia coli and Proteus sp. Pathologically, fibrotic scarring is present at the poles, due to urinary reflux as well as dilated calices. Histopathologically, interstitial fibrosis is seen along with mononuclear leukocyte infiltration and tubular atrophy (Damjanov and Linder, 1996). The lesions are asymmetrical which results in an irregular contraction of the kidneys. In pigs, the lesions are similar to those in humans and the most common pathogen is also E. coli (Figure 3C1). However, other pathogens such as Staphylococcus, Streptococcus, Enterobacter, Proteus, and Actinobaculum have also been isolated from cases of porcine pyelonephritis (Maxie, 2007).
Only a few porcine models of pyelonephritis have been created. A vesico-urethral reflux model was established in Sinclair mini-pigs for the study of chronic atrophic pyelonephritis (Table 4; Hodson et al., 1975). Both the inoculated and non-inoculated group developed scarring composed of fibrosis and leukocyte infiltration. Six of the inoculated pigs in the experiment eradicated the induced infection with E. coli, spontaneously, whereas 11 of the non-inoculated pigs, developed an infection (Hodson et al., 1975). Ransley and Risdon (1981) also developed a vesico-urethral reflux model using E. coli (Table 4). To sustain infection, the bacteria were inoculated within paraffin wax into the bladder. The aim of that study was to test different therapeutic methods for chronic pyelonephritis. The pigs developed marked interstitial renal fibrosis and were treated with different antibiotics without significant effect (Ransley and Risdon, 1981). In another study by Farhat et al. (2002), E. coli was also embedded in paraffin wax in order to sustain the infection. In the model, a total of 67% of the pigs developed renal scarring (Table 4; Farhat et al., 2002). Finally, in a novel model of acute pyelonephritis, a catheter was placed directly into the renal pelvis for inoculation (Table 4; Isling et al., 2011). Three different strains of E. coli with different virulence factors were used for inoculation (Isling et al., 2011). In the study, the strain which was positive for P fimbriae, an important virulence factor in the development of human pyelonephritis, developed the most pronounced lesions and mimicked the lesions seen in humans (Figures 3C2,C3; Isling et al., 2011).
Porcine Models of Hematogenous Osteomyelitis
The rate of bone remodeling as well as the cross-sectional diameter of the femoral bone is similar in pigs and humans (Pearce et al., 2007). Pigs also show similarities to human bone composition, especially with respect to mineral density and mineralization of the bones (Aerssens et al., 1998). The muscles and bones of pigs are more massive compared to humans which reflects that they are quadruped (Swindle and Smith, 1998). Although, pigs have a denser osseous trabecular network, the lamellar bone structure is similar to humans (Pearce et al., 2007). Due to the similarities in bone composition and regeneration, the pig has been used as a model for several studies of both hematogenous and IAO (Wood et al., 1971; Koschmieder et al., 1975; Patterson et al., 1993; Rink et al., 2001; Jensen et al., 2010b, 2016,2017; Johansen et al., 2010, 2012a,b, 2013; Tøttrup et al., 2016).
In children, osteomyelitis is predominantly caused by hematogenous spread of bacteria. The lesions are most often located in long bones especially within the metaphysis of femur and tibia (Lew and Waldvogel, 2004). In adults, the vertebral bones are most commonly infected (Lew and Waldvogel, 2004; Brady et al., 2008). Following infection, an abscess will be formed, with a fibrous layer surrounding leukocytes and bacteria (Damjanov and Linder, 1996). The most common bacterium causing human osteomyelitis is S. aureus, but S. epidermidis is also often involved. Other bacteria involved in hematogenous osteomyelitis are Streptococcus, Pneumococcus, E. coli, Klebsiella, Salmonella, and Bacteroides (Damjanov and Linder, 1996). In slaughter pigs, the dominant site of infection is also within the metaphysis of long bones and the vertebrae (Figure 3D1). In pigs, hematogenous osteomyelitis is often caused by T. pyogenes, but S. aureus may also be the cause (Zachary, 2017). Although the same histomorphology is present, there will often be multifocal lesions in pigs (Maxie, 2007). In conventional pigs, the portal of entrance is often tail bites causing pyemia (Bækbo et al., 2016).
Several porcine models of hematogenous osteomyelitis have been developed. The first was established in 1971 by Wood et al. In that study, two different strains of S. pyogenes were inoculated through vena cava cranialis and they were inoculated once a day for three consecutive days (Table 5; Wood et al., 1971). The pigs developed endocarditis, arthritis and osteomyelitis. Some of the osteomyelitis lesions were caused by extension of the arthritis lesions and some were due to a direct hematogenous spread (Wood et al., 1971). In another study, pigs were inoculated intravenously with S. aureus and they developed microscopic metaphyseal osteomyelitis lesions after 12 h (Table 5; Jensen et al., 2010b). In a study by Johansen et al. (2010), S. aureus was inoculated into the brachial artery, in order to induce osteomyelitis in the distal part of the forelimb (Table 5; Johansen et al., 2010). It was found that the minimum required dose for inoculation was 5 × 103 CFU/ml, in order to induce suppurative bone lesions (Johansen et al., 2010). In three other studies by Johansen et al. (2012a,b, 2013), S. aureus was inoculated into the femoral artery (Table 5; Johansen et al., 2012a,b, 2013). In the first study, three different strains of S. aureus were examined. The porcine strain, S54F9, resulted in the development of osteomyelitis lesions in all pigs. It was also shown that biofilm was present in the infected bone by PNA FISH (Johansen et al., 2012a). The novel technique of intraarterial inoculation used for inducing experimental osteomyelitis, was detailed in the second study by Johansen et al. (2012b; Figures 3D2,D3). In the third study from 2013, surgical debridement of experimental osteomyelitis was performed, and the lesions seen in the pigs were comparable to osteomyelitis lesions in kids. This study showed that the pig is a good model for evaluating surgical treatment methods for hematogenous osteomyelitis (Johansen et al., 2013).
Porcine Models of Implant-Associated Osteomyelitis
IAO is generally split into three groups depending on infection time following insertion of the implant, i.e., early, delayed, and late (Zimmerli et al., 2004). In humans, the most common type of infection is in the delayed group (3–24 months after surgery) and it is caused by local contamination by S. aureus or other bacteria during insertion of the implant (Figure 3E1; Zimmerli et al., 2004). The late type is caused by a hematogenous spread of bacteria colonizing the implant/prosthesis up to several years after insertion (Zimmerli et al., 2004). Histologically, a periprosthetic membrane surrounding the implant will be formed. This membrane can be divided into four different types (I–IV), where “Type II” is the infectious type. The infectious type is characterized by the proliferation of fibroblasts and small blood vessels, edema, and leukocyte infiltration, dominated by PMNs (Morawietz et al., 2006). Although, IAO does not occur spontaneously in pigs, it is assumed, that the pig will be a good model, due to its similarity in bone composition and remodeling (Pearce et al., 2007).
In a porcine model by Koschmieder et al. (1975), the effect of Gentamicin embedded in Palacos bone cement was examined (Koschmieder et al., 1975). In the study, IAO was established by traumatic intramedullary inoculation of S. aureus (Table 5), however, bacterial contamination was found and limited the conclusions of the study (Koschmieder et al., 1975). A traumatic mandibular IAO model was established in pigs using an 8 mm trephine (Table 5). In the model, three strains of S. aureus were inoculated intramedullary and afterwards, the trephine hole was filled with either bone cement or wax (Patterson et al., 1993). Another porcine IAO model was established by Rink et al. (2001) where an 18G needle was inserted in a mid-diaphysis fracture line and S. aureus was inoculated (Table 6). This was done in order to make a cDNA library of the cellular immune response in a porcine model of IAO (Rink et al., 2001) Recently, a novel porcine model of IAO was developed and comprehensively analyzed according to the local, regional and systemic response. In this model, a small Kirschner wire was inserted into the right tibial bone. Before the implant insertion, three different doses of S. aureus were applied (Table 6), and the model showed good reproducibility when an inoculum dose of 104 CFU/ml was used (Figures 3E2,E3; Jensen et al., 2017). In a later study by Jensen et al. (2016), the former porcine IAO model was used to examine the extension of infection into the peri-implanted bone tissue after 5 days (Table 6; Jensen et al., 2016). Recently, a study performed by Tøttrup et al. (2016), focused on the penetration of cefuroxime into the infected bone lesions also using the porcine IAO model (Table 6; Tøttrup et al., 2016). The models of IAO mentioned above were all established successfully, and showed advantages for analyzing the bioavailability of antibiotics in e.g., bone cement or given systemically and the impact of the immune system. Moreover, as it also is the situation in human clinical settings, one should be aware of contamination problems when inserting implants.
Summary
The basic knowledge of infectious biofilm has been achieved from in vitro studies (Lebeaux et al., 2013). However, biofilm grown in vitro is not in every respect comparable to biofilm produced in vivo (Bjarnsholt et al., 2013). Therefore, it is advantageous to perform studies of infectious biofilm using in vivo experiment, as this will allow studies of the host response with regard to the mechanisms of formation, immune response and therapeutically (Coenye and Nelis, 2010; Lebeaux et al., 2013; Stewart, 2014).
The pig has proven to be an appropriate animal model for the study of chronic bacterial biofilm diseases (Sullivan et al., 2001). The pig allows for several therapeutical trials and also shares a number of similarities to humans with respect to anatomy and the immune system (Swindle and Smith, 1998; Dawson, 2011; Meurens et al., 2012; Swindle et al., 2012). Although the present review highlights the many advantages of using pigs for modeling of human bacterial infectious diseases, small animal models are still used more extensively for the same purposes (Reizner et al., 2014). Common reported advantages of small animal models are related to cost, housing, and handling (Reizner et al., 2014). However, when applying an animal model one of the most important issues should be that the model is discriminative for the disease seen in humans. Due to the general increase in the use of pigs as experimental animals and an increased awareness of reliability in animal models, more porcine models of human bacterial infections are expected to be developed in the future.
Another important factor, which makes the pig a preferable model for many chronic infectious diseases, is that pigs develop many of the diseases spontaneously (Anderson, 1976; Damjanov and Linder, 1996; Maxie, 2007; Jensen, 2009; Jensen et al., 2010a). The pathogenesis and lesions of spontaneously occurring chronic pressure wounds, endocarditis, pyelonephritis, and hematogenous osteomyelitis are similar to those seen in humans and are also often caused by the same bacteria (Anderson, 1976; Damjanov and Linder, 1996; Maxie, 2007; Jensen, 2009; Jensen et al., 2010a). Although IAO does not occur naturally in pigs, porcine models have proven useful as a model because the induced lesions show great similarities to those of humans (Jensen et al., 2017).
When developing a chronic bacterial infectious animal model, it is important to validate whether or not biofilm is formed. Biofilm can be formed both in tissue and on implants, therefore, in future studies it is important to analyze both (Donlan and Costerton, 2002). Biofilm is an important factor in sustaining chronic infections in humans (Donlan and Costerton, 2002; Costerton et al., 2003; Burmølle et al., 2010). The ability of bacteria to form biofilm in porcine models has been demonstrated in a number of studies (Davis et al., 2007, 2013; Johansen et al., 2012a; Roche et al., 2012; Jensen et al., 2016). However, when looking at the studies in this review (Tables 2–6), only five out of the thirty studies, actually commend on the formation of biofilm (Davis et al., 2007, 2013; Johansen et al., 2012a; Roche et al., 2012; Jensen et al., 2016). This probably reflects that most of the studies were carried out decades ago and biofilm is a new focus of interest in regard to chronic infectious diseases. This may also be the reason why biofilm is usually not mentioned in chronic, spontaneous porcine infections (Maxie, 2007). However, as seen from the descriptions of infections in the present review (Figure 3) it is likely that all chronic porcine infections are the cause of biofilm formation as in humans (Donlan and Costerton, 2002; Costerton et al., 2003; Brady et al., 2008). This assumption has also been supported in porcine pneumonia due to Actinobacillus pleuropnumoniae (Tremblay et al., 2017).
In the future, more models of chronic bacterial biofilm infections should be examined in pigs. Among others, studies of cystic fibrosis and otitis media based on porcine models would be relevant, as the pig has proven to have great similarity to humans with respect to anatomy of the respiratory tract and the conformation of the middle and inner ear (Pracy et al., 1998; Meurens et al., 2012; Schomberg et al., 2016).
Author Contributions
LJ and HJ designed the structure of the review. AJ collected all the references. LJ, AJ, and HJ drafted the manuscript.
Funding
This review was financed by grant no. 4005-00035B from the Danish Research Council and the European Union's Horizon 2020 research and innovation program under NOMORFILM project grant agreement No 634588.
Conflict of Interest Statement
The authors declare that the research was conducted in the absence of any commercial or financial relationships that could be construed as a potential conflict of interest.
Acknowledgments
Kristiane Barrington, Johanna Christensen, Klaus Kirketerp-Møller, Louise Krag Isling, and Thomas Bjarnsholt are acknowledged for donation of pictures.
References
Aerssens, J., Boonen, S., Lowet, G., and Dequeker, J. (1998). Interspecies differences in bone composition, density, and quality: potential implications for in vivo bone research. Endocrinology 139, 663–670.
Archer, N. K., Mazaitis, M. J., Costerton, J. W., Leid, J. G., Powers, M. E., and Shirtliff, M. E. (2011). Staphylococcus aureus biofilms: properties, regulation and roles in human disease. Virulence 2, 445–459. doi: 10.4161/viru.2.5.17724
Bækbo, A. K., Petersen, J. V., Larsen, M. H., and Alban, L. (2016). The food safety value of de-boning finishing pig carcasses with lesions indicative of prior septicaemia. Food Control 69, 177–184. doi: 10.1016/j.foodcont.2016.04.030
Bjarnsholt, T. (2013). The role of bacterial biofilms in chronic infections. APMIS 121, 1–51. doi: 10.1111/apm.12099
Bjarnsholt, T., Alhede, M., Alhede, M., Eickhardt-Sørensen, S. R., Moser, C., Kühl, M., et al. (2013). The in vivo biofilm. Trends Microbiol. 21, 466–474. doi: 10.1016/j.tim.2013.06.002
Bowler, P. G. (2002). Wound pathophysiology, infection and therapeutic options. Ann. Med. 34, 419–427. doi: 10.1080/078538902321012360
Brady, R. A., Leid, J. G., Calhoun, J. H., Costerton, J. W., and Shirtliff, M. E. (2008). Osteomyelitis and the role of biofilms in chronic infection. FEMS Immunol. Med. Microbiol. 52, 13–22. doi: 10.1111/j.1574-695X.2007.00357.x
Breuing, K., Kaplan, S., Liu, P., Onderdonk, A. B., and Eriksson, E. (2003). Wound fluid bacterial levels exceed tissue bacterial counts in controlled porcine partial-thickness burn infections. Plast. Reconstr. Surg. 111, 781–788. doi: 10.1097/01.PRS.0000041540.22057.89
Burmølle, M., Thomsen, T. R., Fazli, M., Dige, I., Christensen, I. L., Homøe, P., et al. (2010). Biofilms in chronic infections - A matter of opportunity - Monospecies biofilms in multispecies infections. FEMS Immunol. Med. Microbiol. 59, 324–336. doi: 10.1111/j.1574-695X.2010.00714.x
Christiansen, J. G., Jensen, H. E., Jensen, L. K., Koch, J., Aalbæk, B., Nielsen, O. L., et al. (2013a). Systemic inflammatory response and local cytokine expression in porcine models of endocarditis. APMIS 122, 292–300. doi: 10.1111/apm.12145
Christiansen, J. G., Jensen, H. E., Johansen, L. K., Koch, J., Koch, J., Aalbaek, B., et al. (2013b). Porcine models of non-bacterial thrombotic endocarditis (NBTE) and infective endocarditis (IE) caused by Staphylococcus aureus: a preliminary study. J. Heart Valve Dis. 22, 368–376.
Coenye, T., and Nelis, H. J. (2010). In vitro and in vivo model systems to study microbial biofilm formation. J. Microbiol. Methods 83, 89–105. doi: 10.1016/j.mimet.2010.08.018
Costerton, J. W., Lewandowski, Z., Caldwell, D. E., Korber, D. R., and Lappin-scott, H. M. (1995). Microbial biofilms. Annu. Rev. Microbiol. 49, 711–745. doi: 10.1146/annurev.mi.49.100195.003431
Costerton, J. W., Stewart, P., and Greenberg, E. P. (1999). Bacterial biofilms: a common cause of persistent infections. Science 284, 1318–1322. doi: 10.1126/science.284.5418.1318
Costerton, W., Veeh, R., Shirtliff, M., Pasmore, M., Post, C., and Ehrlich, G. (2003). The application of biofilm science to the study and control of chronic bacterial infections. J. Clin. Invest. 112, 1466–1477. doi: 10.1172/JCI200320365
Dahl-Pedersen, K., Bonde, M. K., Herskin, M. S., Jensen, K. H., Kaiser, M., and Jensen, H. E. (2013). Pathogenesis and pathology of shoulder ulcerations in sows with special reference to peripheral nerves and behavioural responses to palpation. Vet. J. 198, 666–671. doi: 10.1016/j.tvjl.2013.09.059
Davey, M. E., and O'toole, G. A. (2000). Microbial biofilms: from ecology to molecular genetics. Microbiol. Mol. Biol. Rev. 64, 847–867. doi: 10.1128/MMBR.64.4.847-867.2000
Davis, K., Bills, J., Barker, J., Kim, P., and Lavery, L. (2013). Simultaneous irrigation and negative pressure wound therapy enhances wound healing and reduces wound bioburden in a porcine model. Wound Repair Regen. 21, 869–875. doi: 10.1111/wrr.12104
Davis, S. C., Ricotti, C., Cazzaniga, A., Welsh, E., Eaglstein, W. H., and Mertz, P. M. (2007). Microscopic and physiologic evidence for biofilm-associated wound colonization in vivo. Wound Repair Regen. 16, 23–29. doi: 10.1111/j.1524-475X.2007.00303.x
Dawson, H. D. (2011). “Chapter 22: A comparative assessment of the pig, mouse and human genomes,” in The Minipig in Biomedical Research, eds P. A. McAnulty, A. D. Dayan, N.-C. Ganderup, and K. L. Hastings (CRC Press), 323–342. doi: 10.1201/b11356-28
Dewar, H. A., Jones, M. R., Griffin, S. G., Oxley, A., and Marriner, J. (1987). A study of experimental endocarditis in pigs. J. Comp. Pathol. 97, 567–574. doi: 10.1016/0021-9975(87)90007-7
Donlan, R. M., and Costerton, J. W. (2002). Biofilms: survival mechanisms of clinically relevant microorganisms. Clin. Microbiol. Rev. 15, 167–193. doi: 10.1128/CMR.15.2.167-193.2002
Driskell, R., Jahoda, C. A. B., Chuong, C. M., Watt, F., and Horsley, V. (2014). Defining dermal adipose tissue. Exp. Dermatol. 23, 629–631. doi: 10.1111/exd.12450
Farhat, W., Traubici, J., Sherman, C., Williams, T., Babyn, P., and McLorie, G. (2002). Reliability of contrast enhanced sonography with harmonic imaging for detecting early renal scarring in experimental pyelonephritis in a porcine model: preliminary results. J. Urol. 168, 1114–1117. doi: 10.1097/01.ju.0000025869.03295.79
Flemming, H. C., and Wingender, J. (2010). The biofilm matrix. Nat. Rev. Microbiol. 8, 623–633. doi: 10.1038/nrmicro2415
Geissinger, H. D., Miniats, O. P., Runhke, L. H., and Djurickovic, D. G. (1973). Experimental staphylococcal endocarditis in pigs: bacteriological, histopathological and scanning electron microscopic observations. J. Comp. Pathol. 83, 323–335. doi: 10.1016/0021-9975(73)90056-X
Ghanbari, A., Dehghany, J., Schwebs, T., Müsken, M., Häussler, S., and Meyer-Hermann, M. (2016). Inoculation density and nutrient level determine the formation of mushroom-shaped structures in Pseudomonas aeruginosa biofilms. Sci. Rep. 6:32097. doi: 10.1038/srep32097
Harris, D. L., and Alexander, T. J. L. (1999). “Methods of disease control,” in B. E. Straw, S. Y. Dallaire, W. L. Mengeling, and D. J. Taylor Diseases of Swine, 8th Edn (Iowa, IA: Iowa State University Press), 1077.
Hirsch, T., Spielmann, M., Zuhaili, B., Koehler, T., Fossum, M., Steinau, H.-U., et al. (2008). Enhanced susceptibility to infections in a diabetic wound healing model. BMC Surg. 8:5. doi: 10.1186/1471-2482-8-5
Hodson, C. J., Maling, T. M., McManamom, P. J., and Lewis, M. G. (1975). The pathogenesis of reflux nephropathy (Chronic atrophic pyelonephritis). Br. J. Radiol. 13, 1–26.
Ibrahim, Z., Busch, J., Awwad, M., Wagner, R., Wells, K., and Cooper, D. K. (2006). Selected physiologic compatibilities and incompatibilities between human and porcine organ systems. Xenotransplantation 13, 488–499. doi: 10.1111/j.1399-3089.2006.00346.x
Isling, L. K., Aalbæk, B., Birck, M. M., Heegaard, P. M. H., and Leifsson, P. S. (2011). Host response to porcine strains of Escherichia coli in a novel pyelonephritis model. J. Comp. Pathol. 144, 257–268. doi: 10.1016/j.jcpa.2010.10.002
Jensen, H. E. (2009). Investigation into the pathology of shoulder ulcerations in sows. Vet. Rec. 165, 171–174. doi: 10.1136/vr.165.6.171
Jensen, H. E., Gyllensten, J., Hofman, C., Leifsson, P. S., Agerholm, J. S., Boye, M., et al. (2010a). Histologic and bacteriologic findings in valvular endocarditis of slaughter-age pigs. J. Vet. Diagn. Invest. 22, 921–927. doi: 10.1177/104063871002200611
Jensen, H. E., Nielsen, O. L., Agerholm, J. S., Iburg, T., Johansen, L. K., Johannesson, E., et al. (2010b). A non-traumatic Staphylococcus aureus osteomyelitis model in pigs. in vivo 24, 257–264.
Jensen, L. K., Koch, J., Aalbæk, B., Moodley, A., Bjarnsholt, T., Kragh, K. N., et al. (2016). Early implant-associated osteomyelitis results in a peri-implanted bacterial reservoir. APMIS 125, 38–45. doi: 10.1111/apm.12597
Jensen, L. K., Koch, J., Dich-Jorgensen, K., Aalbaek, B., Petersen, A., Fuursted, K., et al. (2017). Novel porcine model of implant-associated osteomyelitis: a comprehensive analysis of local, regional, and systemic response. J. Orthop. Res. 35, 2211–2221. doi: 10.1002/jor.23505
Johansen, L. K., Frees, D., Aalbæk, B., Koch, J., Iburg, T., Nielsen, O. L., et al. (2010). A porcine model of acute, haematogenous, localized osteomyelitis due to Staphylococcus aureus: a pathomorphological study. APMIS 119, 111–118. doi: 10.1111/j.1600-0463.2010.02700.x
Johansen, L. K., Koch, J., Frees, D., Aalbæk, B., Nielsen, O., Leifsson, P. S., et al. (2012a). Pathology and biofilm formation in a porcine model of staphylococcal osteomyelitis. J. Comp. Pathol. 147, 343–353. doi: 10.1016/j.jcpa.2012.01.018
Johansen, L. K., Koch, J., Kirketerp-Moller, K., Wamsler, O. J., Nielsen, O. L., Leifsson, P. S., et al. (2013). Therapy of haematogenous osteomyelitis - A comparative study in a porcine model and angolan children. in vivo 27, 305–312.
Johansen, L. K., Svalastoga, E. L., Frees, D., Aalbæk, B., Koch, J., Iburg, T., et al. (2012b). A new technique for modeling of hematogenous osteomyelitis in pigs: inoculation into femoral artery. J. Invest. Surg. 26, 149–153. doi: 10.3109/08941939.2012.718043
Johnson, C. M., Bahn, R. C., and Fass, D. N. (1986). Experimental porcine infective endocarditis: description of a clinical model. Vet. Pathol. 23, 780–782. doi: 10.1177/030098588602300620
Jones, J. E. T. (1969). The experimental production of streptococcal endocarditis in the pig. J. Pathol. 99, 307–318. doi: 10.1002/path.1710990406
Jones, J. E. T. (1981). Experimental streptococcal endocarditis in the pig: the development of lesions 3 to 14 days after inoculation. J. Comp. Pathol. 91, 51–62. doi: 10.1016/0021-9975(81)90044-X
Jones, J. E. T. (1982). Experimental streptococcal endocarditis in the pig: the development of lesions 18 to 48 hours after inoculation. J. Comp. Pathol. 92, 301–308. doi: 10.1016/0021-9975(82)90089-5
Kim, H. K., Thammavongsa, V., Schneewind, O., and Missiakas, D. (2012). Recurrent infections and immune evasion strategies of Staphylococcus aureus. Curr. Opin. Microbiol. 15, 92–99. doi: 10.1016/j.mib.2011.10.012
Kirketerp-Møller, K., Jensen, P., Fazli, M., Madsen, K. G., Pedersen, J., Moser, C., et al. (2008). Distribution, organization, and ecology of bacteria in chronic wounds. J. Clin. Microbiol. 46, 2717–2722. doi: 10.1128/JCM.00501-08
Koschmieder, R., Ritzerfeld, W., and Homeyer, L. (1975). Gentamycinzusatz zum polymethylmethacrylat zur behandlung von knocheninfektionen. Z. Orthop. 113, 147–149.
Lebeaux, D., Chauhan, A., Rendueles, O., and Beloin, C. (2013). From in vitro to in vivo models of bacterial biofilm-related infections. Pathogens 2, 288–356. doi: 10.3390/pathogens2020288
Lew, D. P., and Waldvogel, F. A. (2004). Osteomyelitis. Lancet 364, 369–379. doi: 10.1016/S0140-6736(04)16727-5
Liu, Y., Chen, J. Y., Shang, H. T., Liu, C. E., Wang, Y., Niu, R., et al. (2010). Light microscopic, electron microscopic, and immunohistochemical comparison of bama minipig (sus scrofa domestica) and human skin. Comp. Med. 60, 142–148.
Lund, M. (2003). Skuldersår hos søer [Shoulder ulcerations in sows]. Danish Veterinary Record 22, 8–11.
Medina, A., Scott, P. G., Ghahary, A., and Tredget, E. E. (2005). Pathophysiology of chronic nonhealing wounds. J. Burn Care Rehabil. 26, 306–319. doi: 10.1097/01.BCR.0000169887.04973.3A
Meurens, F., Summerfield, A., Nauwynck, H., Saif, L., and Gerdts, V. (2012). The pig: a model for human infectious diseases. Trends Microbiol. 20, 50–57. doi: 10.1016/j.tim.2011.11.002
Morawietz, L., Classen, R. A., Schroder, J. H., Dynybil, C., Perka, C., Skwara, A., et al. (2006). Proposal for a histopathological consensus classification of the periprosthetic interface membrane. J. Clin. Pathol. 59, 591–597. doi: 10.1136/jcp.2005.027458
Murdoch, D. R., Corey, G. R., Hoen, B., Miró, J., Fowler, V. G. Jr., Bayer, A. S., et al. (2009). Clinical presentation, etiology, and outcome of infective endocarditis in the 21st century. Arch. Intern. Med. 169, 463–473. doi: 10.1001/archinternmed.2008.603
Nusbaum, A. G., Gil, J., Rippy, M. K., Warne, B., Valdes, J., Claro, A., et al. (2012). Effective method to remove wound bacteria: comparison of various debridement modalities in an in vivo porcine model. J. Surg. Res. 176, 701–707. doi: 10.1016/j.jss.2011.11.1040
Patterson, A. L., Galloway, R. H., Baumgartner, J. C., and Barsoum, I. S. (1993). Development of chronic mandibular osteomyelitis in a miniswine model. J. Oral Maxillofac. Surg. 51, 1358–1362. doi: 10.1016/S0278-2391(10)80142-9
Pearce, A. I., Richards, R. G., Milz, S., Schneider, E., and Pearce, S. G. (2007). Animal models for implant biomaterial research in bone: a review. Eur. Cells Mater. 13, 1–10. doi: 10.22203/eCM.v013a01
Pracy, J. P., White, A., Mustafa, Y., Smith, D., and Perry, M. E. (1998). The comparative anatomy of the pig middle ear cavity: a model for middle ear inflammation in the human? J. Anat. 192, 359–368. doi: 10.1046/j.1469-7580.1998.19230359.x
Ransley, P. G., and Risdon, R. A. (1981). Reflux nephropathy: effects of antimicrobial therapy on the evolution of the early pyelonephritic scar. Kidney Int. 20, 733–742. doi: 10.1038/ki.1981.204
Reizner, W., Hunter, J. G., O'Malley, N. T., Southgate, R. D., Schwarz, E. M., Kates, S. L., et al. (2014). A systematic review of animal models for Staphylococcus aureus osteomyelitis. Eur. Cells Mater. 27, 196–212. doi: 10.22203/eCM.v027a15
Rink, A., Santschi, E. M., and Beattie, C. W. (2001). Normalized cDNA libraries from a porcine model of orthopedic implant-associated infection. Mamm. Genome 13, 198–205. doi: 10.1007/s00335-001-2120-0
Roberts, A. E. L., Kragh, K. N., Bjarnsholt, T., and Diggle, S. P. (2015). The limitations of in vitro experimentation in understanding biofilms and chronic infection. J. Mol. Biol. 427, 3646–3661. doi: 10.1016/j.jmb.2015.09.002
Roche, E. D., Renick, P. J., Tetens, S. P., Ramsay, S. J., Daniels, E. Q., and Carson, D. L. (2012). Increasing the presence of biofilm and healing delay in a porcine model of MRSA-infected wounds. Wound Repair Regen. 20, 537–543. doi: 10.1111/j.1524-475X.2012.00808.x
Rumbaugh, K. P., and Carty, N. L. (2011). “In vivo model of biofilm infections,” in Biofilm Infections, eds T. Bjarnsholt, P. Jensen, C. Moser, N. Høiby (New York, NY: Springer), 267–290.
Schomberg, D. T., Tellez, A., Meudt, J. J., Brady, D. A., Dillon, K. N., Arowolo, F. K., et al. (2016). Miniature swine for preclinical modeling of complexities of human disease for translational scientific discovery and accelerated development of therapies and medical devices. Toxicol. Pathol. 44, 299–314. doi: 10.1177/0192623315618292
Seaton, M., Hocking, A., and Gibran, N. S. (2015). Porcine models of cutaneous wound healing. ILAR J. 56, 127–138. doi: 10.1093/ilar/ilv016
Stewart, P. S. (2014). Biophysics of biofilm infection. Pathog. Dis. 70, 212–218. doi: 10.1111/2049-632X.12118
Sullivan, T. P., Eaglstein, W. H., Davis, S. C., and Mertz, P. (2001). The pig as a model for human wound healing. Wound Repair Regen. 9, 66–76. doi: 10.1046/j.1524-475x.2001.00066.x
Swindle, M. M., and Smith, A. C. (1998). “Comparative anatomy and physiology of the pig,” in Information Resources on Swine in Biomedical Research 1990-2000, Vol. 25, ed C. P. Smith (Beltsville, MD: United States Department of Agriculture, Agricultural Research Service, National Agricultural Library, Animal Welfare Information Center), 11–21.
Swindle, M. M., Makin, A., Herron, A. J., Clubb, F. J., and Frazier, K. S. (2012). Swine as models in biomedical research and toxicology testing. Vet. Pathol. 49, 344–356. doi: 10.1177/0300985811402846
Tøttrup, M., Bue, M., Koch, J., Jensen, L. K., Hanberg, P., Aalbæk, B., et al. (2016). Effects of implant-associated osteomyelitis on cefuroxime bone pharmacokinetics: assessment in a porcine model. J. Bone Joint Surg. Am. 2, 363–369. doi: 10.2106/JBJS.O.00550
Tremblay, Y. D. N., Labrie, J., Chénier, S., and Jacques, M. (2017). Actinobacillus pleuropneumoniae grows as aggregates in the lung of pigs: is it time to refine our in vitro biofilm assays? Microb. Biotechnol. 10, 756–760. doi: 10.1111/1751-7915.12432
Wood, R. L., Cutlip, R. C., and Shuman, R. D. (1971). Osteomyelitis and arthritis induced in swine by Lancefield's group A streptococci (Streptococcus pyogenes). Cornell Vet. 61, 457–470.
Yang, Q., Larose, C., Della Porta, A. C., Schultz, G. S., and Gibson, D. J. (2017). A surfactant-based wound dressing can reduce bacterial biofilms in a porcine skin explant model. Int. Wound J. 14, 408–413. doi: 10.1111/iwj.12619
Keywords: biofilm, pig, animal model, hematogenous osteomyelitis, implant-associated osteomyelitis, chronic wounds, endocarditis, pyelonephritis
Citation: Jensen LK, Johansen ASB and Jensen HE (2017) Porcine Models of Biofilm Infections with Focus on Pathomorphology. Front. Microbiol. 8:1961. doi: 10.3389/fmicb.2017.01961
Received: 26 June 2017; Accepted: 22 September 2017;
Published: 10 October 2017.
Edited by:
Sara María Soto, ISGlobal, SpainReviewed by:
Paul Cos, University of Antwerp, BelgiumDevendra Hiraman Dusane, The Ohio State University Columbus, United States
Dipankar Ghosh, Jawaharlal Nehru University, India
Copyright © 2017 Jensen, Johansen and Jensen. This is an open-access article distributed under the terms of the Creative Commons Attribution License (CC BY). The use, distribution or reproduction in other forums is permitted, provided the original author(s) or licensor are credited and that the original publication in this journal is cited, in accordance with accepted academic practice. No use, distribution or reproduction is permitted which does not comply with these terms.
*Correspondence: Louise K. Jensen, bG91aXNlLWtAc3VuZC5rdS5kaw==