- 1Departamento de Genética, Instituto de Biociências, Universidade Federal do Rio Grande do Sul, Porto Alegre, Brazil
- 2Departamento de Bioquímica e Biologia Molecular, Centro Politécnico, Universidade Federal do Paraná, Curitiba, Brazil
- 3Department of Genetics of Prokaryotes, Faculty of Biology & CeBiTec, Bielefeld University, Bielefeld, Germany
Species from the genus Paenibacillus are widely studied due to their biotechnological relevance. Dozens of novel species descriptions of this genus were published in the last couple of years, but few utilized genomic data as classification criteria. Here, we demonstrate the importance of using genome-based metrics and phylogenetic analyses to identify and classify Paenibacillus strains. For this purpose, Paenibacillus riograndensis SBR5T, Paenibacillus sonchi X19-5T, and their close relatives were compared through phenotypic, genotypic, and genomic approaches. With respect to P. sonchi X19-5T, P. riograndensis SBR5T, Paenibacillus sp. CAR114, and Paenibacillus sp. CAS34 presented ANI (average nucleotide identity) values ranging from 95.61 to 96.32%, gANI (whole-genome average nucleotide identity) values ranging from 96.78 to 97.31%, and dDDH (digital DNA–DNA hybridization) values ranging from 68.2 to 73.2%. Phylogenetic analyses of 16S rRNA, gyrB, recA, recN, and rpoB genes and concatenated proteins supported the monophyletic origin of these Paenibacillus strains. Therefore, we propose to assign Paenibacillus sp. CAR114 and Paenibacillus sp. CAS34 to P. sonchi species, and reclassify P. riograndensis SBR5T as a later heterotypic synonym of P. sonchi (type strain X19-5T), with the creation of three novel genomovars, P. sonchi genomovar Sonchi (type strain X19-5T), P. sonchi genomovar Riograndensis (type strain SBR5T), P. sonchi genomovar Oryzarum (type strain CAS34T = DSM 102041T; = BR10511T).
Introduction
The genus Paenibacillus includes nitrogen-fixing species that were isolated from roots of wheat (Beneduzi et al., 2010), maize (Berge et al., 2002), rice (Beneduzi et al., 2008; de Souza et al., 2014), and other plants (Ambrosini et al., 2015). Many of these bacterial isolates arose from the search for strains that exert positive effects on the development of agricultural plants. The inoculation of plants or seeds with these plant-growth promoting bacteria (PGPB) can help to reduce the use of chemical fertilizers and pesticides, improving agricultural sustainability (Beneduzi et al., 2008; Seldin, 2011).
In 2010, the species Paenibacillus riograndensis (Beneduzi et al., 2010) and Paenibacillus sonchi (Hong et al., 2009) were described in an interval of only 9 days, according to electronic publication dates. P. riograndensis is a nitrogen fixer and phytormone producer (Beneduzi et al., 2010), which in later studies was found to be very closely related to P. sonchi, although their metabolic repertoire seemed to be distinct (Jin et al., 2011). Recently, the complete genome sequence of P. riograndensis SBR5T (Brito et al., 2015) and a draft genome sequence of P. sonchi X19-5T (Xie et al., 2014) were determined, and preliminary computation of their ANI (average nucleotide identity) suggested that they belong to the same species.
Genomic data are portable (i.e., data obtained from different laboratories can be compared), and highly informative with respect to evolutionary relationships, which are desired features for any taxonomic scheme. Although high-throughput sequencing technologies facilitated access to genome sequences, taxonomic reports rarely present this kind of data. For example, since 2016, from more than 20 novel Paenibacillus species descriptions in the International Journal of Systematic and Evolutionary Microbiology, only one of them utilized genomic analyses (Liu et al., 2016).
Here, we demonstrate the importance of using genomic analyses to clarify the taxonomic assignment of P. riograndensis and P. sonchi. For this purpose, we compared P. riograndensis SBR5T, P. sonchi X19-5T, and their close relatives, Paenibacillus graminis DSM 15220T, Paenibacillus jilunlii DSM 23019T, and two strains preliminarily identified as P. riograndensis/P. sonchi (Paenibacillus sp. CAS34 and Paenibacillus sp. CAR114) through genomic, genotypic, and phenotypic approaches.
Materials and Methods
Bacterial Strains
Paenibacillus graminis DSM 15220T (= RSA19T) and Paenibacillus jilunlii DSM 23019T were purchased from Deutsche Sammlung von Mikroorganismen und Zellkulturen (DSMZ), Braunschweig, Germany. P. sonchi X19-5T (= LMG 24727T) was acquired from Belgian Coordinated Collections of Microorganisms – Laboratory for Microbiology of Ghent University (BCCM/LMG), Ghent, Belgium. P. riograndensis SBR5T (= CCGB 1313T; = CECT 7330T) (Beneduzi et al., 2010), Paenibacillus sp. CAR114 (= DSM 102250; = BR10512), and Paenibacillus sp. CAS34 (= DSM 102041; = BR10511) were obtained from our bacterial collection. Paenibacillus sp. CAR114 and CAS34 strains were originally isolated from roots of rice (Oryza sativa) cultivated in Cachoeirinha, Southern Brazil (de Souza et al., 2014). Paenibacillus polymyxa ATCC 842T (= BGSC 25A1T), the type strain of the genus Paenibacillus, was purchased from Bacillus Genetic Stock Center (BGSC).
Culture Conditions and Biochemical Tests
All Paenibacillus strains were routinely grown in King B broth [Peptone 20 g/liter; K2HPO4 1.15 g/liter; MgSO4.7H2O, 1.5 g/liter; Glycerol, 1.5% (vol/vol)] at 28°C previously to the physiological tests (Glickmann and Dessaux, 1995). Morphological and biochemical characterization were carried out as described in standard protocols (Holt, 1986, 2000; MacFaddin, 2000). For biochemical profiling, five independent experiments were performed. As described elsewhere, bacterial strains were also tested for the production of indolic compounds (IC) (Glickmann and Dessaux, 1995), siderophores (Schwyn and Neilands, 1987), and for the ability to perform biological nitrogen fixation (Boddey and Knowles, 2008; Ambrosini et al., 2012). Three independent cultures were evaluated for each assay.
Multivariate Analyses Based on Phenotypic Data
Principal component analysis (PCA) was used to verify the statistical variance-covariance of cellular fatty acids among five Paenibacillus species through PAST software (Hammer et al., 2001).
Genome Sequences
All genomes utilized in this study are listed in Supplementary Table S1. Genome sequences of P. jilunlii DSM 23019T, Paenibacillus sp. CAR114 and Paenibacillus sp. CAS34 were obtained using the Illumina Miseq platform. Total DNA was extracted as described elsewhere (Ambrosini et al., 2012). Libraries were constructed using Nextera XT kit and sequenced with MiSeq Reagent Kit V3 (2 × 300 bp). Draft genomes were assembled using SPAdes version 3.5 (careful option and k-mer values equal to 21, 33, 55, 77, 99, 127) (Bankevich et al., 2012), and annotated using the NCBI Prokaryotic Genome Automatic Annotation Pipeline (PGAAP), which combines Hidden Markov Model (HMM)-based gene prediction methods with homology-based methods (Angiuoli et al., 2008).
Genome assembly quality was assessed with Quast version 2.3 (Gurevich et al., 2013) and Checkm version 0.9.6 (Parks et al., 2014).
R2cat (Husemann and Stoye, 2010) is a contig arrangement tool used to order a set of contigs with respect to a single reference genome, in which mapping of the contigs onto the reference is based on a q-gram filter. The results are visualized by plotting contigs onto the reference sequence of a complete genome. EDGAR (Efficient Database framework for comparative Genome Analyses using BLAST score Ratios) was utilized to automatically perform genome comparisons in a high throughput approach (Blom et al., 2009). EDGAR generates synteny plots, describing the physical co-localization of genes, which may change order during evolution by rearrangement events like inversions, deletions, insertions, or translocations.
Protein counterparts of Paenibacillus strains were detected in P. riograndensis SBR5 translated genome sequence through tblastn searches, implemented in BRIG software version 0.95 (Alikhan et al., 2011).
16S rRNA, gyrB, recA, recN, and rpoB Gene Phylogenies
16S rRNA gene sequences of Paenibacillus type-strains were retrieved from RDP (Ribosomal Database Project) (Cole et al., 2014). 16S rRNA genes were also obtained from annotated genomes (Supplementary Table S1). 16S rRNA gene sequences were aligned using SINA software version 1.2.11 with default parameters (Pruesse et al., 2012). The 16S rRNA gene alignment without gaps contained 775 positions. The gyrB, recA, recN, and rpoB genes were retrieved from the genomes (Supplementary Table S2), and aligned using MUSCLE software version 3.8.31 using default parameters (Edgar, 2004). Positions containing gaps of the sequence alignments were removed. The gyrB, recA, recN, and rpoB alignments without gaps contained 1911, 1059, 1215 and 2596 positions, respectively.
Maximum-likelihood phylogenies were conducted with the Phylogeny.fr platform with the PhyML program (v3.0 aLRT) (Dereeper et al., 2008). GTR (Generalized Time Reversible) substitution model was selected assuming an estimated proportion of invariant sites and four gamma-distributed rate categories to account for rate heterogeneity across sites. Gamma shape parameters were estimated directly from the data. Reliability for internal branching was assessed using the aLRT (approximate Likelihood Ratio Test) (Anisimova and Gascuel, 2006). All phylogenetic trees were rooted using P. polymyxa ATCC 842T as outgroup. The 16S rRNA gene phylogenetic tree was pruned using Newick Utilities (Junier and Zdobnov, 2010), maintaining strains closely related to the P. riograndensis and P. sonchi species.
Core-Proteome and AMPHORA Multiprotein Phylogenetic Reconstructions
Ortholog protein groups were defined using bidirectional best hits algorithm implemented in Get_homologues build 20170609. For this purpose, the core-proteome was compiled using minimum BLAST searches and clusters containing inparalogs were excluded. Each of the 1102 single-copy proteins was aligned with MUSCLE software using default parameters. Alignments were concatenated with Phyutility (Smith and Dunn, 2008). Phylogenetic tree of the core-proteome was reconstructed with Mega 6 software build 6140226 (Tamura et al., 2013), using Neighbor Joining approach with Jones-Taylor-Thornton substitution model, deleting positions containing gaps and using 500 bootstrap replicates.
Thirty-one marker proteins were detected in the genomes using Amphora pipeline (Wu and Eisen, 2008) implemented at the AmphoraNet (Kerepesi et al., 2014). Since our analysis included draft genome sequences, few protein sequences were found fragmented in more than one contig. Therefore, if more than one hit for a protein was found in a genome, the largest one was kept for subsequent analyses in order to maximize the information for phylogenetic inference. All Amphora proteins are listed in Supplementary Table S3. Proteins were aligned and concatenated as described above. Aligned positions were curated using Gblocks (Castresana, 2000) with default parameters (implemented in Phylogeny.fr pipeline). The concatenated alignment without gaps containing 6289 positions. Phylogenetic analysis of AMPHORA concatenated multiprotein sequence was performed with the Phylogeny.fr platform as described in the previous section, although the WAG substitution model was utilized in this case.
Both phylogenies were rooted using P. polymyxa ATCC 842T as the outgroup.
ANI, dDDH, and MiSI Estimations
Average nucleotide identity values based on Blast alignments from all pairwise genome comparisons were computed at JspeciesWS1 (Richter and Rosselló-Móra, 2009). dDDH values were estimated at GGDC (Genome-to-Genome Distance Calculator)2 using GGDC 2.0 BLAST+ and recommended formula 2 (Meier-Kolthoff et al., 2013a). MiSI method was utilized as described in Varghese et al. (2015). For this purpose, all CDS from each genome were extracted using a script written in BioPython (Cock et al., 2009), which can be downloaded from https://github.com/fhsantanna/bioinfo_scripts.
Results
Phenotypic Analyses
The type strains of P. graminis and P. jilunlii, and two strains, Paenibacillus sp. CAR114 and Paenibacillus sp. CAS34, which are all closely related to P. riograndensis and P. sonchi, had their phenotypic characteristics evaluated. Table 1 and Supplementary Table S4 show the biochemical capabilities of these bacteria. Concerning the tests performed, P. sonchi X19-5T and P. riograndensis SBR5T only diverged with respect to starch degradation.
As demonstrated in Supplementary Table S5, Paenibacillus sp. CAR114 and Paenibacillus sp. CAS34 were able to fix nitrogen and synthesize indolic compounds as P. sonchi X19-5T and P. riograndensis SBR5T.
Considering each species at a time in the scatter plot generated from multivariate analysis of the fatty acid profiles, the points representing independent profiles of P. graminis DSM 15220T, P. jilunlii DSM 23019T and P. sonchi X19-5T were more distant from each other than those from the other Paenibacillus strains (Supplementary Figure S1 and Table S6).
16S rRNA Gene Analyses
The 16S rRNA genes were analyzed concerning their identity levels and phylogenetic history. P. sonchi, P. riograndensis, P. graminis, and P. jilunlii strains shared 16S rRNA gene identity values higher or equal than the species cutoff (Supplementary Table S7). In the 16S rRNA gene phylogeny containing all sequences from Paenibacillus type-species deposited in RDP, P. sonchi X19-5T, P. riograndensis SBR5T, Paenibacillus sp. CAR114 and Paenibacillus sp. CAS34 formed a clade (Figure 1 and Supplementary Figure S2). However, no subclades were present in this monophyletic group. It is worth noting that both P. riograndensis SBR5T and P. graminis DSM 15220T have multiple copies of 16S rRNA gene (9 and 10, respectively) in their complete sequenced genomes (not all copies were available from strains with draft genomes). Even though 16S rRNA gene paralogs were distinct, they were not variable enough to be dispersed in different clades (Supplementary Figure S2).
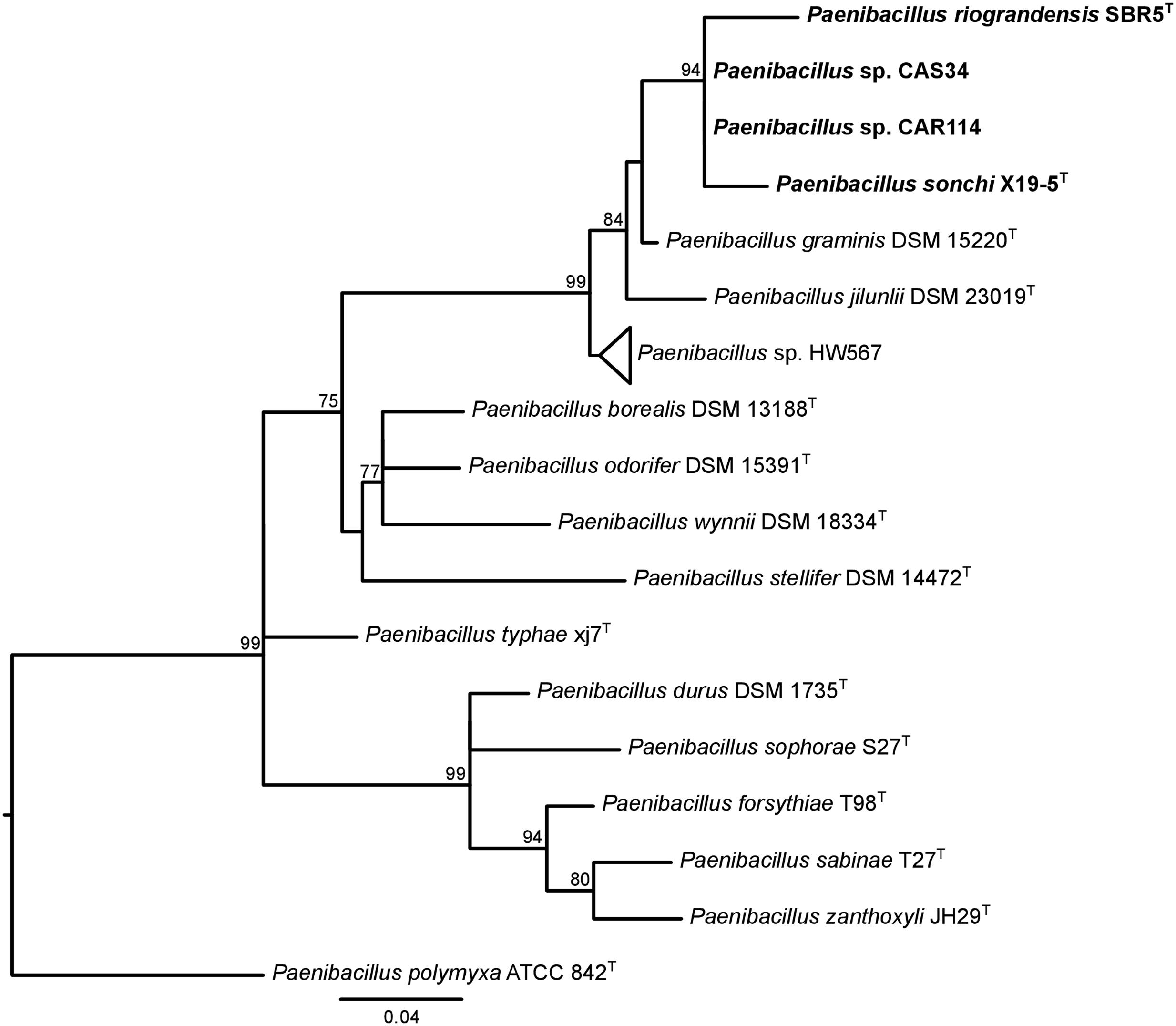
FIGURE 1. 16S rRNA gene phylogeny of Paenibacillus species. The 16S rRNA gene rooted tree was constructed using the maximum-likelihood method. aLRT values greater than 70% are shown next to the branches. The tree is drawn to scale, with branch lengths in the same units as those of the evolutionary distances used to infer the phylogenetic tree. P. polymyxa is the outgroup. Bacteria of P. riograndensis/P. sonchi clade are in bold. This tree is the same of Supplementary Figure S2, although only taxa of interest were kept.
Genomic Analyses
For some genomic analyses, we also considered other Paenibacillus strains from sister groups of P. riograndensis-P. sonchi-P. graminis-P. jilunlii cluster, whose genome sequences were publicly available (Supplementary Table S1).
The G+C content of Paenibacillus strains ranged from 44.2 to 53.5%, although the values among P. graminis DSM 15220T, P. jilunlii DSM 23019T, P. riograndensis SBR5T, P. sonchi X19-5T, Paenibacillus sp. CAR114, and Paenibacillus sp. CAS34 did not vary more than 1 percentual point (Supplementary Table S1). In order to analyze genome structural conservation in selected Paenibacillus species, we generated synteny plots to compare the complete genome sequence of P. riograndensis SBR5T to those from P. graminis DSM 15220T and Paenibacillus sp. HW567 (Figure 2A). Also, the draft genome sequences of P. sonchi X19-5T, Paenibacillus sp. CAR114, Paenibacillus sp. CAS34, P. graminis DSM 15220T, P. jilunlii DSM 23019T, and P. polymyxa ATCC 842T were mapped to the complete genome sequence of P. riograndensis SBR5T (Figure 2B). With the exception of P. polymyxa ATCC 842T graph, the plots presented long diagonal lines, interrupted only by few short gaps (Figures 2A,B).
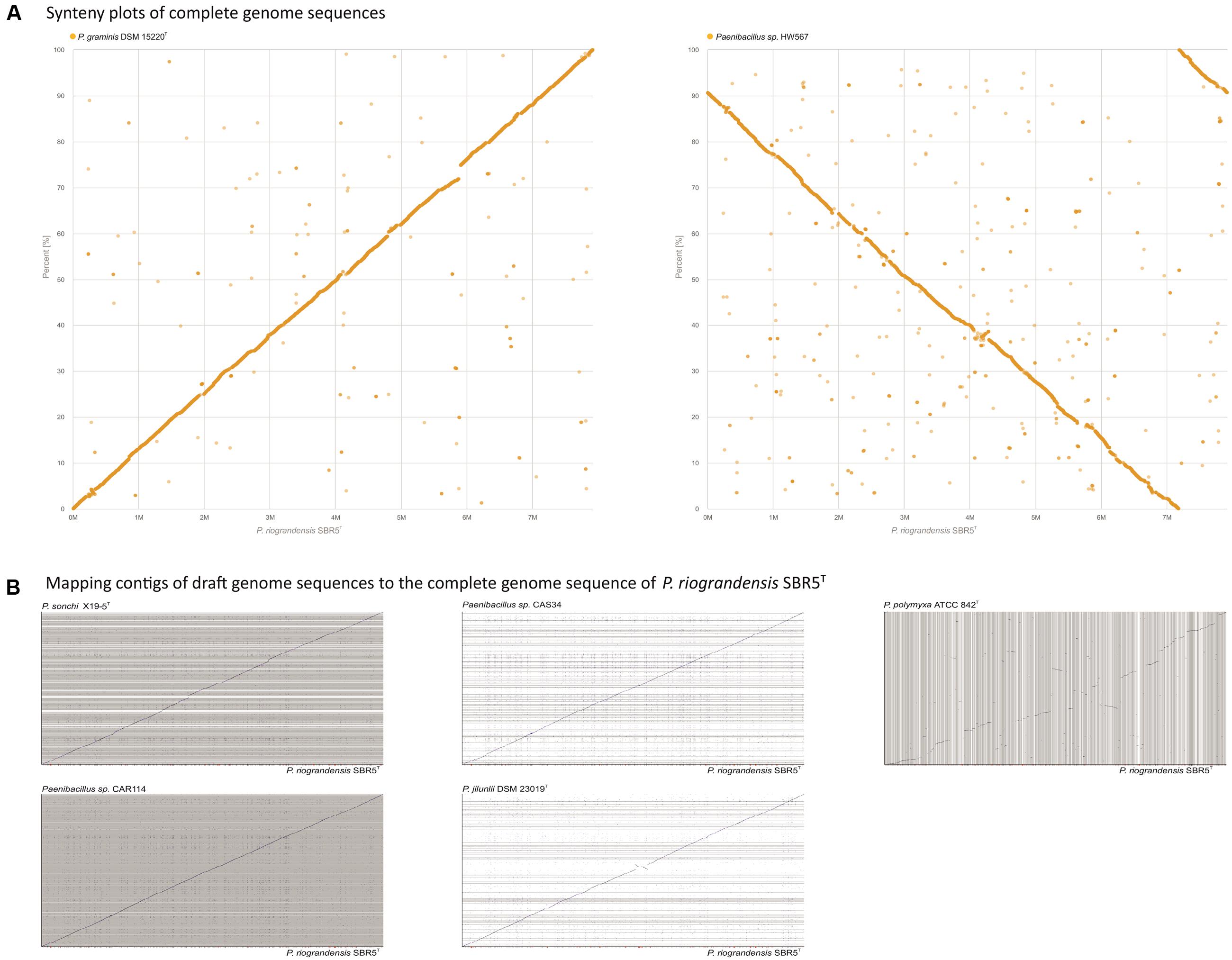
FIGURE 2. Synteny analysis of P. riograndensis SBR5T and the complete genome sequences of related species (A), and mapping of draft genome sequences of related species to the P. riograndensis SBR5T genome (B). Blue line denotes syntenic scaffold alignments. The horizontal bar at the bottom indicates coverage of the matches to the reference scaffold, SBR5T, with maximal coverage indicated with black, fading to light gray with less coverage. Red indicates uncovered regions.
The content of homolog proteins of the Paenibacillus strains in relation to the translated genome sequence of P. riograndensis SBR5T was also verified (Figure 3). Closely related strains, represented by the six inner rings in Figure 3, presented more color dense regions than other strains. On the other hand, the 10 outer rings of Figure 3, representing relatively more distant strains in relation to P. riograndensis SBR5T, presented more gaps.
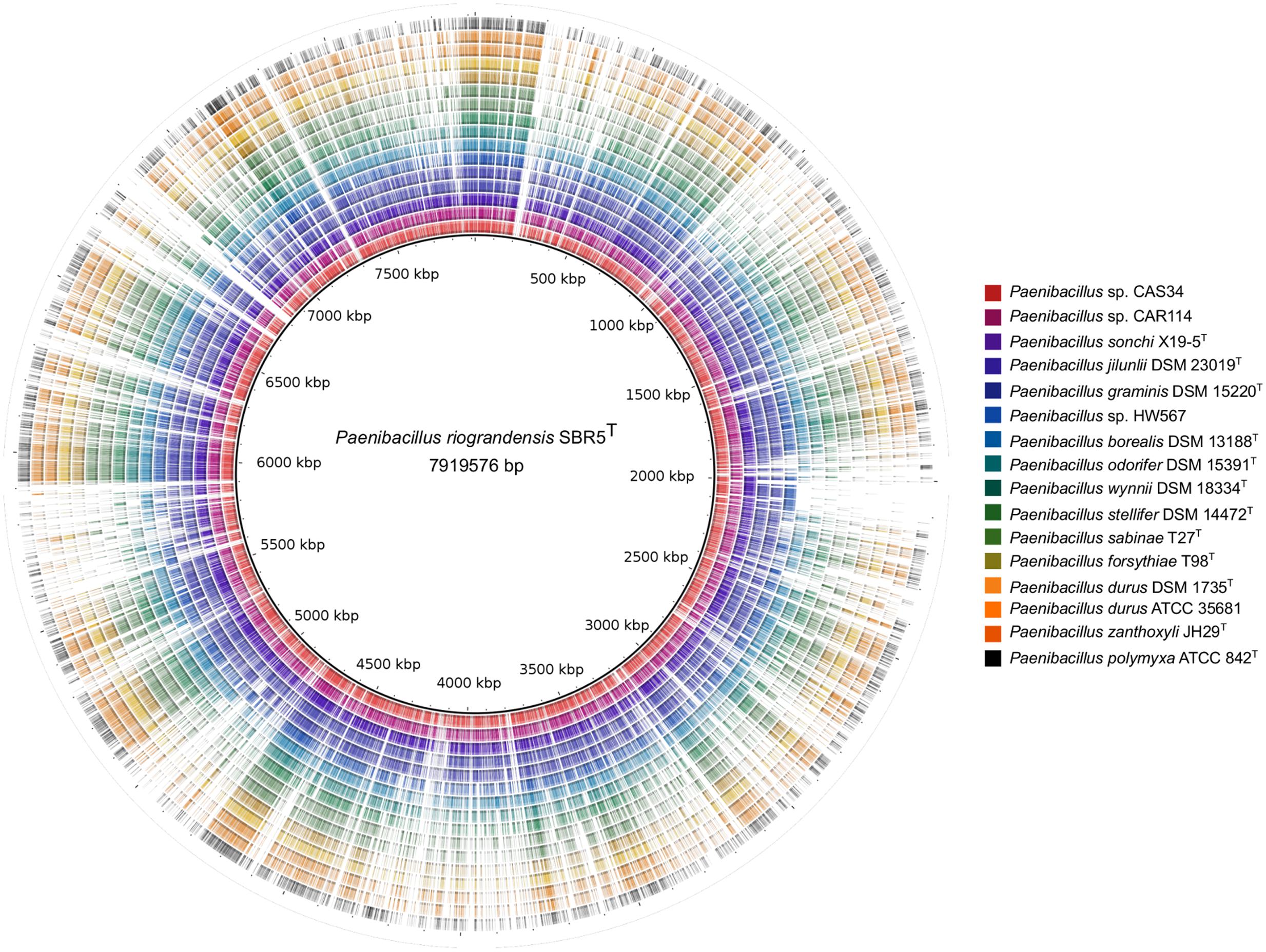
FIGURE 3. Comparison of predicted proteins of Paenibacillus spp. against the P. riograndensis SBR5 translated genome sequence. The innermost black line circle represents the P. riograndensis SBR5 genome sequence. Each one of the outer rings represents a Paenibacillus strain, as depicted in the legend box. Colored regions of each ring symbolize tblastn hits with at least 30% of identity.
Genomic metrics computed from comparisons among P. riograndensis SBR5T, P. sonchi X19-5T, Paenibacillus sp. CAR114, and Paenibacillus sp. CAS34 presented values above the species circumscription thresholds (Table 2 and Supplementary Tables S8–S10).
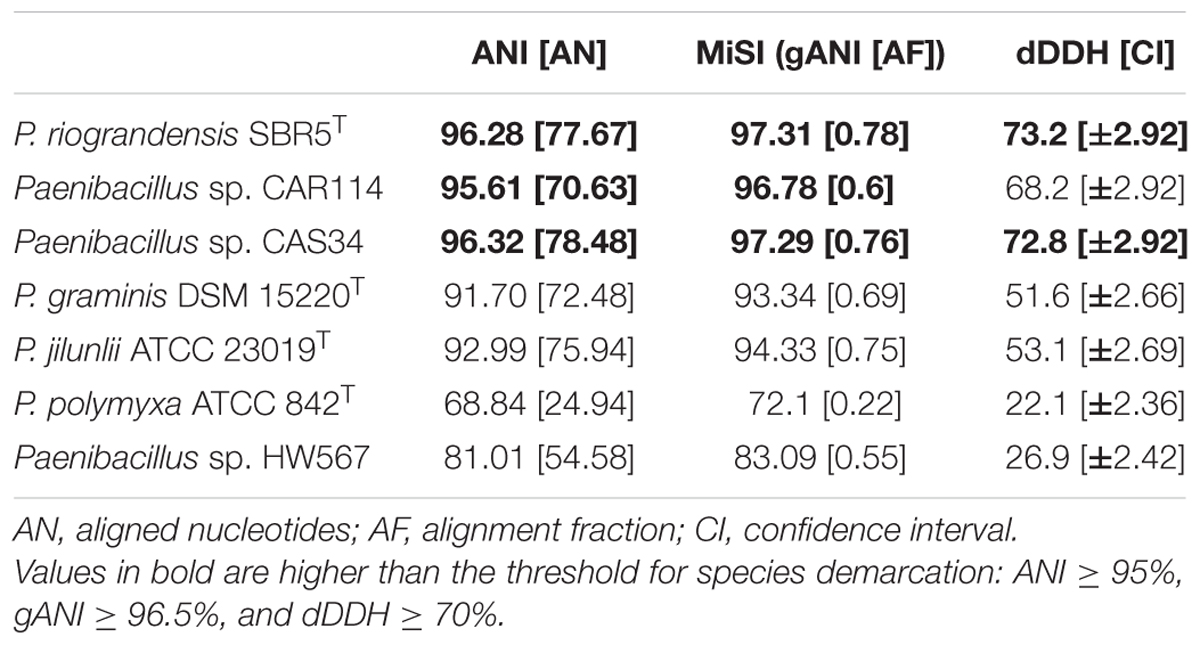
TABLE 2. Comparison of P. sonchi X19-5T with Paenibacillus strains through different whole-genome based methods.
Although the dDDH value of Paenibacillus sp. CAR114 versus P. sonchi X19-5T was 68.2%, the superior limit of the confidence interval (71.12%) surpassed the dDDH species threshold of 70% (Table 2). Paenibacillus sp. CAR114 and Paenibacillus sp. CAS34 presented dDDH values higher than the subspecies circumscription threshold of 79% between themselves, but lower than that in relation to P. riograndensis SBR5T and P. sonchi X19-5T (Supplementary Table S10). Furthermore, P. riograndensis SBR5T and P. sonchi X19-5T presented 73.2% of dDDH, below the subspecies threshold (Table 2).
Figure 4 and Supplementary Table S11 show the correspondence between the 16S rRNA gene identity values and ANI values computed in a pairwise manner. Dozens of strain-strain comparisons presented 16S rRNA gene identity values higher than the species threshold for this marker, although their ANI values were below 95%. Only those comparisons among P. riograndensis SBR5T, P. sonchi X19-5T, Paenibacillus sp. CAR114 and Paenibacillus CAS34 presented values above the ANI and 16S rRNA gene identity thresholds.
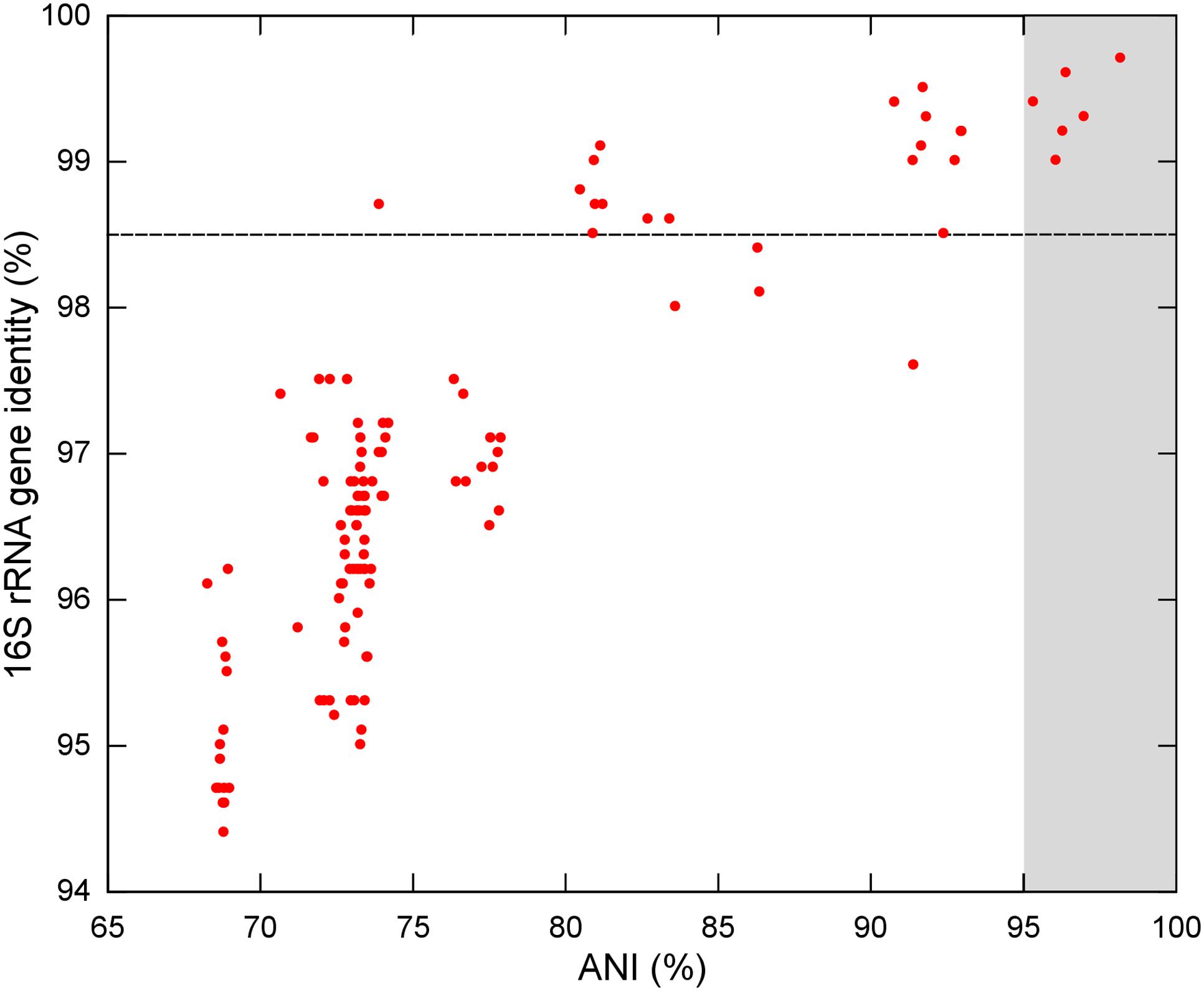
FIGURE 4. Relationship between ANI and 16S rRNA gene identity levels among Paenibacillus species. Each red circle represents a comparison between two Paenibacillus species. Traced line shows the 16S rRNA gene identity threshold for species demarcation. Gray background represents the ANI range for species demarcation. All six red circles over the gray background are pairwise comparisons of P. riograndensis, P. sonchi, Paenibacillus sp. CAR114, and Paenibacillus CAS34.
Phylogenetic Analyses
The phylogenetic history of P. sonchi, P. riograndensis, and their closely related species was also reconstructed utilizing gyrB, recA, recN and rpoB genes, a combined dataset of 31 marker proteins (AMPHORA pipeline) and the concatenated core-proteome. The clade composed of P. riograndensis SBR5T, P. sonchi X19-5T, Paenibacillus sp. CAR114 and Paenibacillus CAS34 was consistent in all of these phylogenetic reconstructions (Figures 5–7), as well as in the 16S rRNA gene phylogeny (Figure 1). Furthermore, with the exception of recA phylogeny, all trees contained a subclade of Paenibacillus sp. CAR114 and Paenibacillus sp. CAS34, which grouped with P. riograndensis SBR5T. However, this latter grouping pattern was only supported in the rpoB gene and core-proteome phylogenies.
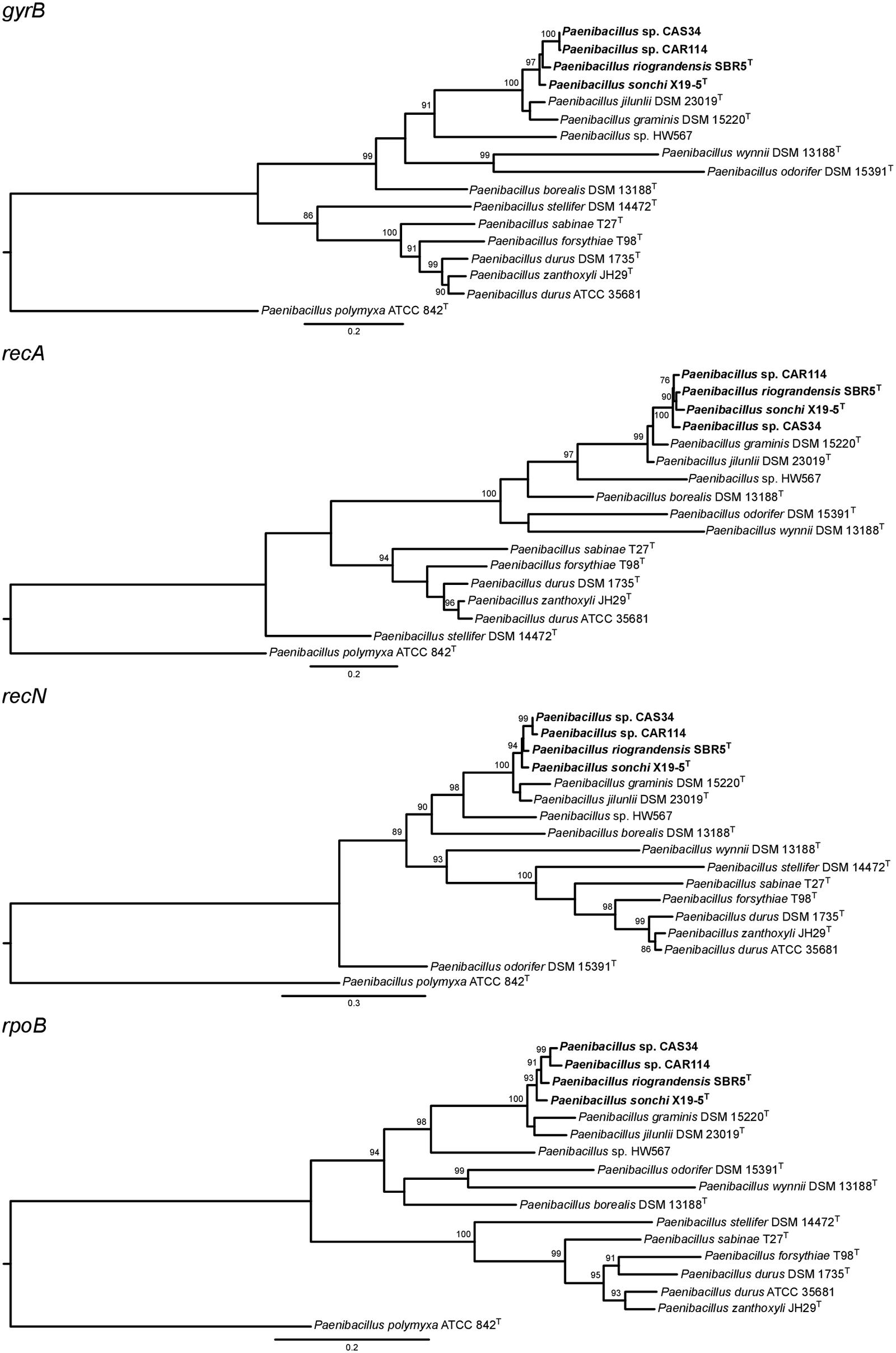
FIGURE 5. Phylogenies of genes other than 16S rRNA gene of Paenibacillus species. The trees were built using the maximum-likelihood method. Details are as shown in Figure 1, unless specified otherwise.
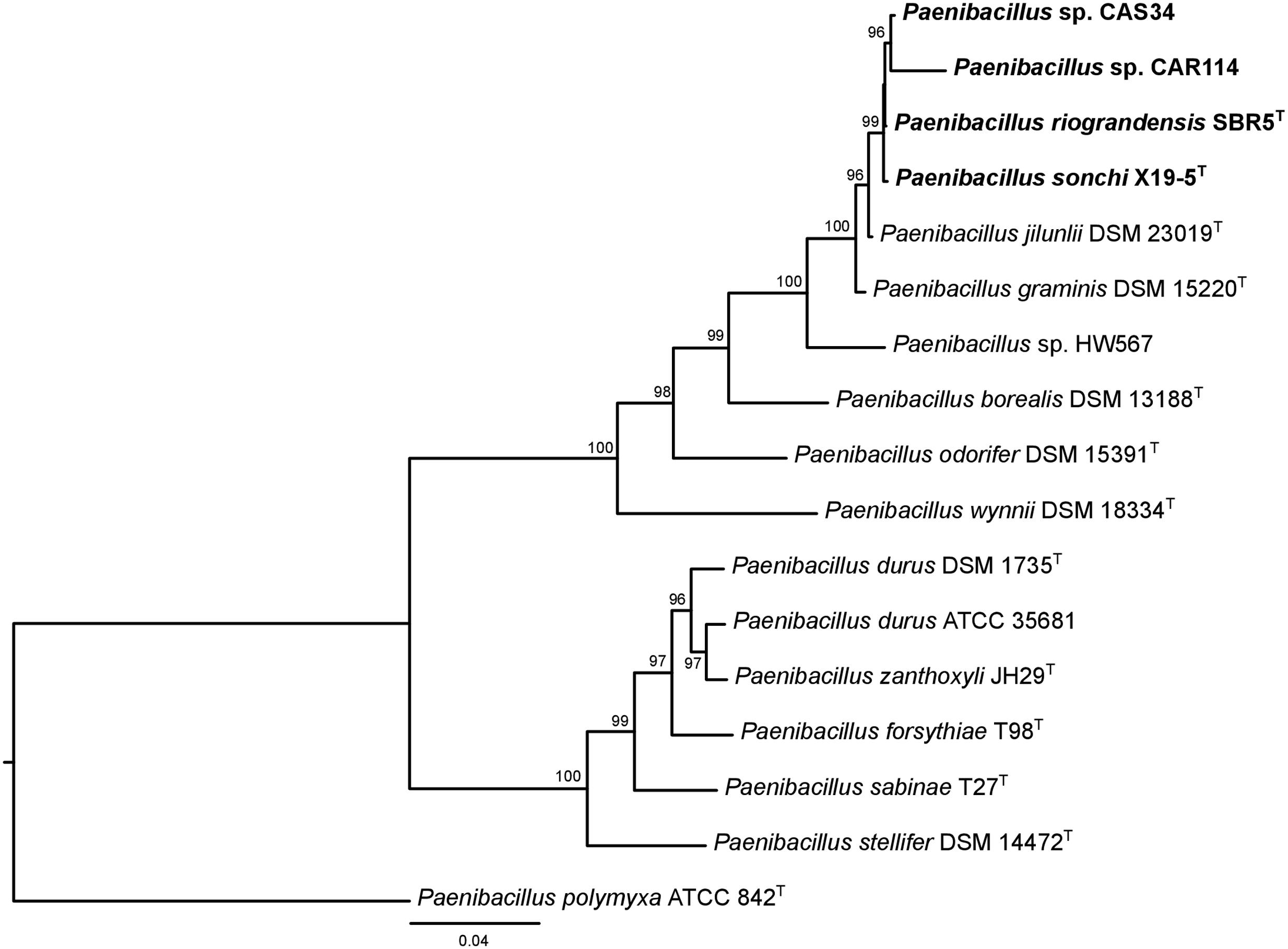
FIGURE 6. AMPHORA multiprotein phylogeny of Paenibacillus species. The multiprotein rooted tree was constructed using the maximum-likelihood method. Details are as shown in Figure 1, unless specified otherwise.
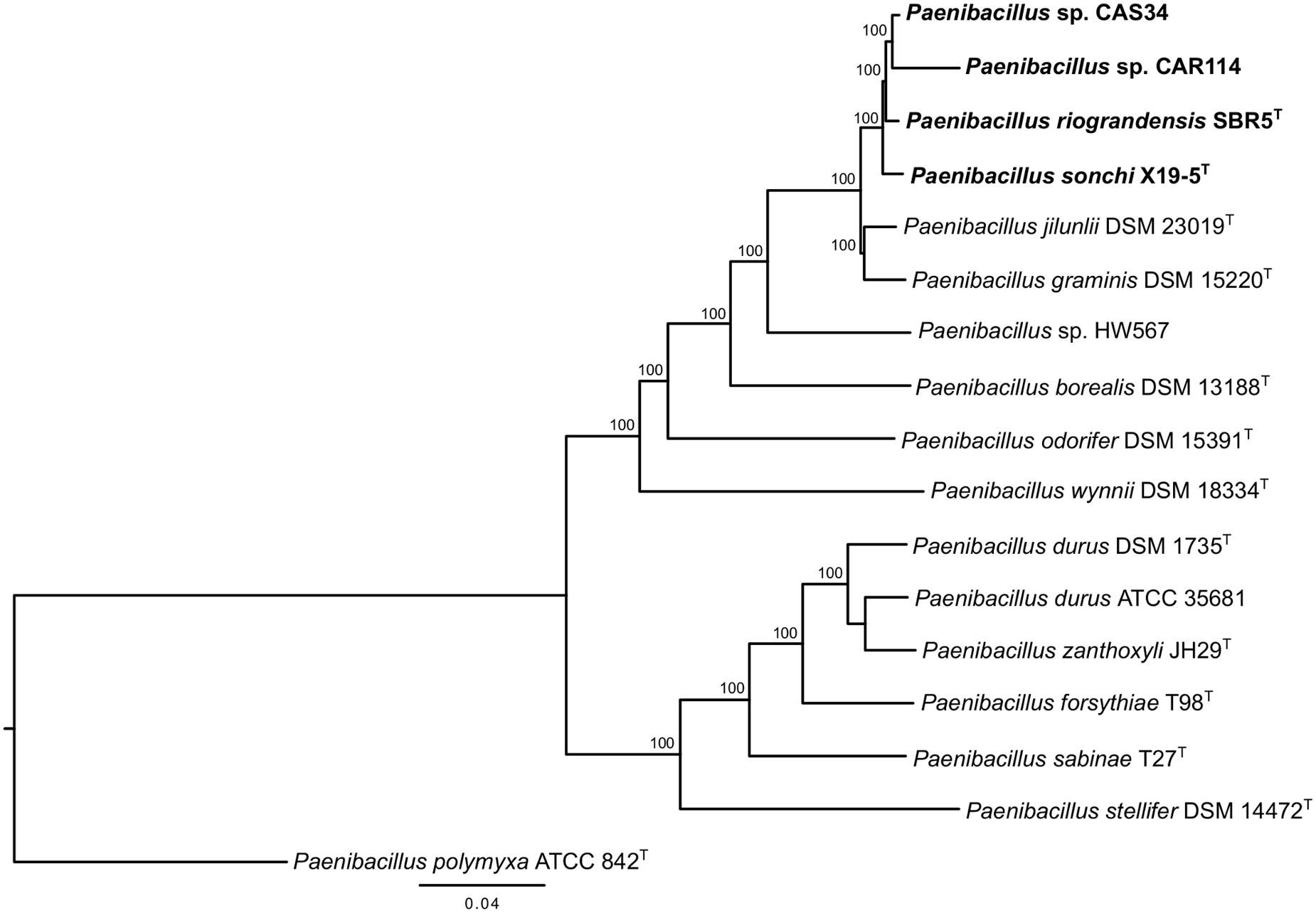
FIGURE 7. Core-proteome phylogeny of Paenibacillus species. The core-proteome rooted tree was constructed using the Neighbor Joining method. Details are as shown in Figure 1, unless specified otherwise. Bootstrap values greater than 70% are shown next to the branches.
Discussion
Polyphasic taxonomy relies on phenotypic and genotypic analyses to identify and classify bacterial specimens. In line with this principle, we investigated different characteristics of P. riograndensis and P. sonchi in order to clarify their taxonomic statuses. For this purpose, we performed comparative analyses using closely related species to P. riograndensis and P. sonchi as references. As observed by Sutcliffe et al. (2012), most publications describing new prokaryotic taxa are based on only one representative strain. To overcome this limitation, we included in our analyses the strains Paenibacillus sp. CAR114 and Paenibacillus sp. CAS34, preliminarily identified as P. sonchi/P. riograndensis based on 16S rRNA gene comparisons. Both strains were isolated from rice rhizosphere (de Souza et al., 2014), and were able to fix nitrogen and synthesize indolic compounds as P. sonchi X19-5T and P. riograndensis SBR5T. These features are commonly implicated with plant-growth promotion (Lugtenberg and Kamilova, 2009).
For biochemical profiling, we selected tests in which P. sonchi X19-5T and P. riograndensis SBR5T were initially distinguishable, based on their species description reports. However, in our experiments not only P. sonchi X19-5T and P. riograndensis SBR5T presented similar biochemical profiles, but also all strains evaluated did (variation among strains occurred in few tests). In fact, our results are in contrast to some previously published biochemical profiles of Paenibacillus species. For instance, from all Paenibacillus strains considered here, only P. sonchi X19-5T would be unable to produce acid from D-glucose, D-xylose, glycerol, lactose, and maltose. Nevertheless, despite the original description of P. sonchi (Hong et al., 2009), we verified through five independent experiments that its strain X19-5T is actually able to form acid from these carbon sources. Another finding was that P. riograndensis SBR5T is indeed capable to reduce nitrate, as predicted by genome sequence analysis (Brito et al., 2015), contradicting its original report (Beneduzi et al., 2010). As a matter of fact, divergent biochemical profiles were relatively common among different published reports, and at least one of them was problematic because of typographic errors. Therefore, taking these observations into account, at least for the Paenibacillus species studied here, biochemical profiling lacked reproducibility.
This same problem was found while revising fatty acid profiles from independent taxonomic reports, which presented many inconsistencies among Paenibacillus strains. Although fatty acid profiling is an essential prerequisite for bacterial species description, at least in our revision it did not prove to be portable, a desirable feature for chemotaxonomic characters (Logan et al., 2009).
Bacterial identification based on phenotypic traits tend to be less accurate than identification based on genotypic methods (Maughan and Van der Auwera, 2011). First, species may be composed of phenotypically heterogeneous strains (Kumar et al., 2015), i.e., sometimes it is difficult to obtain a common phenotypic pattern among strains of a species. Besides that, reliable phenotypic data are only obtained when strains are assayed simultaneously under carefully controlled culture conditions (Rosselló-Móra, 2012), since minimum variations can affect gene expression, and consequently, the phenotype. Moreover, as such data are usually descriptive, their criteria for species circumscription are not clear; therefore they should be carefully inspected for taxonomic classification purposes.
The homogeneity of P. riograndensis, P. sonchi, P. graminis, and P. jilunlii was also observed regarding their genotypic and genomic traits. Based on the correlation between 16S rRNA identity and genomic relatedness, it was recommended that a 16S rRNA gene identity of ∼98.5% is the adequate minimum threshold for species demarcation (Stackebrandt and Ebers, 2006; Meier-Kolthoff et al., 2013b; Kim et al., 2014). However, this recommendation was not applicable to differentiate the species P. graminis, P. jilunlii, P. sonchi, and P. riograndensis, whose 16S rRNA genes are highly conserved. As demonstrated by other studies, depending on the organisms investigated, 16S rRNA gene may not be a proper taxonomic marker to discriminate organisms at the species level (Fox et al., 1992; Chan et al., 2012).
Similarly, G+C content is also a characteristic with limited taxonomic value for these Paenibacillus species. A G+C content variation of at most 1 percentual point is expected for intra-species comparisons computed from genome sequences (Meier-Kolthoff et al., 2014b). However, the G+C contents of P. graminis DSM 15220T, P. jilunlii DSM 23019T, P. riograndensis SBR5T, P. sonchi X19-5T, Paenibacillus sp. CAR114, and Paenibacillus sp. CAS34 are very similar, not varying more than 1 percentual point among themselves. It is worth noting that the G+C content of P. riograndensis SBR5T and P. sonchi X19-5T were originally measured as 55.1 and 46.8%, respectively. This difference is substantial, since at least 10 percentual points of difference is expected to be found in species of distinct genus (Schleifer, 2009). However, nucleotide compositions were determined using indirect methods, which are less accurate in relation to those obtained using genomic data (Meier-Kolthoff et al., 2014b).
The structural conservation over the whole length of genomes from P. riograndensis SBR5T and other Paenibacillus strains, namely P. sonchi X19-5T, P. jilunlii DSM 23019T, P. graminis DSM 15220T, Paenibacillus sp. CAR114, and Paenibacillus sp. CAS34 was discernible, and the genomic collinearity and proteomic conservation denoted the close phylogenetic relationship among these strains. Indeed, these Paenibacillus strains were only discriminated using methods based on genomic metrics. In this sense, whole genome data of P. riograndensis SBR5T, P. sonchi X19-5T, Paenibacillus sp. CAR114, and Paenibacillus sp. CAS34 generated by ANI, MiSI and dDDH were congruent. Therefore, these four bacterial strains would compose a single species, P. sonchi, which has name priority, since it was published first. Moreover, the results confirmed that P. graminis DSM 15220T and P. jilunlii DSM 23019T belong to other species.
Genome based metrics showed better resolution than 16S rRNA gene identity values. In fact, the resolving power of genome based methods was already explored to discriminate taxa at the infra-specific level. Meier-Kolthoff et al. (2014a) suggested that bacterial subspecies could be discerned based on dDDH values higher than 79%. Given this, P. sonchi could be divided in three subspecies, one harboring P. sonchi X19-5T, other harboring P. riograndensis SBR5T, and finally, another one containing Paenibacillus sp. CAR114 and Paenibacillus sp. CAS34. Although a quantitative measure is an important initiative for subspecies definition, bacterial taxonomy still relies on the interpretation of phenotypic data for this purpose. Therefore, these strains could not be considered subspecies, but genomovars, i.e., they represent genotypic entities at the subspecies level, but they do not present differential phenotypic characters required for categorization as subspecies (Schloter et al., 2000).
Gevers et al. (2005) stated that threshold methods such as ANI and dDDH could fail for delineating species when they contradict species phylogeny (for example, species having ANI values greater than 95% may not form a monophyletic group). Therefore, for taxonomic purposes, it is indispensable for ANI and dDDH values to be assisted by phylogenetic analyses.
The 16S rRNA gene phylogeny supported the results found in analyses based on genomic metrics, since P. riograndensis SBR5T, P. sonchi X19-5T, Paenibacillus sp. CAR114 and Paenibacillus sp. CAS34 formed a clade. This phylogenetic reconstruction showed that P. sonchi and P. riograndensis are closer to each other than to any other Paenibacillus species.
Logan et al. (2009) suggested that genes other than 16S rRNA gene could provide higher resolution for taxonomic analyses at species level. Indeed, the evolutionary relationships among P. riograndensis SBR5T, P. sonchi X19-5T, Paenibacillus sp. CAR114 and Paenibacillus sp. CAS34 were not clear in 16S rRNA gene phylogeny. Phylogenetic reconstructions based on gyrB, recA, recN and rpoB genes, and concatenated multiprotein sequences confirmed the monophyly of P. riograndensis SBR5T, P. sonchi X19-5T, Paenibacillus sp. CAR114, and Paenibacillus sp. CAS34. Furthermore, they also were more discriminative than the 16S rRNA gene phylogeny. With the exception of recA phylogeny, the closest relationship of Paenibacillus sp. CAR114 and Paenibacillus sp. CAS34 predicted by genome-genome comparisons was also demonstrated in these phylogenetic reconstructions.
All above findings strongly support that Paenibacillus sp. CAR114 and Paenibacillus sp. CAS34 belong to P. sonchi species, and that P. riograndensis is a later heterotypic synonym of P. sonchi. Considering phylogenetic reconstructions and dDDH values, we propose that three P. sonchi genomovars should be defined. Since genome approaches were essential for taxonomic classification of these Paenibacillus species, we consider that genomic metrics and phylogenies of genes other than 16S rRNA gene should be compulsory in the guidelines for describing new taxa.
Emended Description of Paenibacillus sonchi Hong et al. (2009)
Paenibacillus sonchi (son’chi. L. n. sonchus -i, the herb sow-thistle, and also a botanical genus name; L. gen. n. sonchi of Sonchus, referring to the plant Sonchus oleraceus, the source of the rhizosphere soil from which the type strain was isolated).
Description as that given by Hong et al. (2009), except for the following modifications. Acid is produced from glucose, sucrose, lactose, D-xylose, maltose, D-sorbitol. Catalase-positive. Nitrate is reduced to nitrite. The G+C content of the DNA of the type strain X19-5T is 50.36 mol%.
Data Accessibility
The Whole Genome Shotgun projects have been deposited at DDBJ/ENA/GenBank under the accession numbers: LIRA00000000, for P. riograndensis CAR114, LIRB00000000 for P. riograndensis CAS34 and LIPY00000000 for P. jilunlii DSM 23019T. Phylogenetic data were deposited at TreeBASE under the accession URL http://purl.org/phylo/treebase/phylows/study/TB2:S18337.
Author Contributions
FS and LP conceived and designed the experiments. FS, AA, LB, VW, and EdS wrote the paper. RdS, GdC, and EvB performed the biochemical assays. EdB and VB carried out the genome sequencing. EdS and FdO contributed with reagents and equipments. LB and VW performed the synteny analysis. AA performed the PCA of fatty acid profiles and analyzed the biochemical profiles of Paenibacillus strains. FS performed most of comparative genome and phylogenetic analyses and generated most of the artwork and tables. FS, AA, EvB, VW, EdS, and LP discussed the data and reviewed the manuscript.
Funding
This work was funded by CNPq/INCT-FBN (Conselho Nacional de Desenvolvimento Científico e Tecnológico/Instituto Nacional de Ciência e Tecnologia da Fixação Biológica de Nitrogênio, Brazil). FS and LB received scholarships from CAPES (Coordenação de Aperfeiçoamento de Pessoal de Nível Superior, Brazil).
Conflict of Interest Statement
The authors declare that the research was conducted in the absence of any commercial or financial relationships that could be construed as a potential conflict of interest.
Acknowledgments
We thank Dr. Tiziano Dalla Rosa and M.Sc. José Evandro Saraiva Pereira for helping us to freeze-dry the Paenibacillus cultures. We acknowledge Dr. Jean-François Pombert for allowing us to assemble the genomes on Mozart supercomputer. We also thank CESUP-UFRGS for letting us use their computer clusters.
Supplementary Material
The Supplementary Material for this article can be found online at: http://journal.frontiersin.org/article/10.3389/fmicb.2017.01849/full#supplementary-material
Footnotes
References
Alikhan, N.-F., Petty, N. K., Ben Zakour, N. L., and Beatson, S. A. (2011). BLAST Ring Image Generator (BRIG): simple prokaryote genome comparisons. BMC Genomics 12:402. doi: 10.1186/1471-2164-12-402
Ambrosini, A., Beneduzi, A., Stefanski, T., Pinheiro, F. G., Vargas, L. K., and Passaglia, L. M. P. (2012). Screening of plant growth promoting Rhizobacteria isolated from sunflower (Helianthus annuus L.). Plant Soil 356, 245–264. doi: 10.1007/s11104-011-1079-1
Ambrosini, A., Stefanski, T., Lisboa, B. B., Beneduzi, A., Vargas, L. K., and Passaglia, L. M. P. (2015). Diazotrophic bacilli isolated from the sunflower rhizosphere and the potential of Bacillus mycoides B38V as biofertiliser. Ann. Appl. Biol. 168, 93–110. doi: 10.1111/aab.12245
Angiuoli, S. V., Gussman, A., Klimke, W., Cochrane, G., Field, D., Garrity, G., et al. (2008). Toward an online repository of standard operating procedures (SOPs) for (meta)genomic annotation. OMICS 12, 137–141. doi: 10.1089/omi.2008.0017
Anisimova, M., and Gascuel, O. (2006). Approximate likelihood-ratio test for branches: a fast, accurate, and powerful alternative. Syst. Biol. 55, 539–552. doi: 10.1080/10635150600755453
Bankevich, A., Nurk, S., Antipov, D., Gurevich, A. A., Dvorkin, M., Kulikov, A. S., et al. (2012). SPAdes: a new genome assembly algorithm and its applications to single-cell sequencing. J. Comput. Biol. 19, 455–477. doi: 10.1089/cmb.2012.0021
Beneduzi, A., Costa, P. B., Parma, M., Melo, I. S., Bodanese-Zanettini, M. H., and Passaglia, L. M. P. (2010). Paenibacillus riograndensis sp. nov., a nitrogen-fixing species isolated from the rhizosphere of Triticum aestivum. Int. J. Syst. Evol. Microbiol. 60, 128–133. doi: 10.1099/ijs.0.011973-0
Beneduzi, A., Peres, D., Vargas, L. K., Bodanese-Zanettini, M. H., and Passaglia, L. M. P. (2008). Evaluation of genetic diversity and plant growth promoting activities of nitrogen-fixing bacilli isolated from rice fields in South Brazil. Appl. Soil Ecol. 39, 311–320. doi: 10.1016/j.apsoil.2008.01.006
Berge, O., Guinebretière, M.-H., Achouak, W., Normand, P., and Heulin, T. (2002). Paenibacillus graminis sp. nov. and Paenibacillus odorifer sp. nov., isolated from plant roots, soil and food. Int. J. Syst. Evol. Microbiol. 52, 607–616. doi: 10.1099/00207713-52-2-607
Blom, J., Albaum, S. P., Doppmeier, D., Pühler, A., Vorhölter, F.-J., Zakrzewski, M., et al. (2009). EDGAR: a software framework for the comparative analysis of prokaryotic genomes. BMC Bioinformatics 10:154. doi: 10.1186/1471-2105-10-154
Boddey, R. M., and Knowles, R. (2008). Methods for quantification of nitrogen fixation associated with gramineae. Crit. Rev. Plant Sci. 6, 209–266. doi: 10.1080/07352688709382251
Brito, L. F., Bach, E., Kalinowski, J., Rückert, C., Wibberg, D., Passaglia, L. M., et al. (2015). Complete genome sequence of Paenibacillus riograndensis SBR5(T), a Gram-positive diazotrophic rhizobacterium. J. Biotechnol. 207, 30–31. doi: 10.1016/j.jbiotec.2015.04.025
Castresana, J. (2000). Selection of conserved blocks from multiple alignments for their use in phylogenetic analysis. Mol. Biol. Evol. 17, 540–552. doi: 10.1093/oxfordjournals.molbev.a026334
Chan, J. Z.-M., Halachev, M. R., Loman, N. J., Constantinidou, C., and Pallen, M. J. (2012). Defining bacterial species in the genomic era: insights from the genus Acinetobacter. BMC Microbiol. 12:302. doi: 10.1186/1471-2180-12-302
Cock, P. J. A., Antao, T., Chang, J. T., Chapman, B. A., Cox, C. J., Dalke, A., et al. (2009). Biopython: freely available Python tools for computational molecular biology and bioinformatics. Bioinformatics 25, 1422–1423. doi: 10.1093/bioinformatics/btp163
Cole, J. R., Wang, Q., Fish, J. A., Chai, B., McGarrell, D. M., Sun, Y., et al. (2014). Ribosomal database project: data and tools for high throughput rRNA analysis. Nucleic Acids Res. 42, D633–D642. doi: 10.1093/nar/gkt1244
de Souza, R., Meyer, J., Schoenfeld, R., da Costa, P. B., and Passaglia, L. M. P. (2014). Characterization of plant growth-promoting bacteria associated with rice cropped in iron-stressed soils. Ann. Microbiol. 65, 951–964. doi: 10.1007/s13213-014-0939-3
Dereeper, A., Guignon, V., Blanc, G., Audic, S., Buffet, S., Chevenet, F., et al. (2008). Phylogeny.fr: robust phylogenetic analysis for the non-specialist. Nucleic Acids Res. 36, W465–W469. doi: 10.1093/nar/gkn180
Edgar, R. C. (2004). MUSCLE: multiple sequence alignment with high accuracy and high throughput. Nucleic Acids Res. 32, 1792–1797. doi: 10.1093/nar/gkh340
Fox, G. E., Wisotzkey, J. D., and Jurtshuk, P. (1992). How close is close: 16S rRNA sequence identity may not be sufficient to guarantee species identity. Int. J. Syst. Bacteriol. 42, 166–170. doi: 10.1099/00207713-42-1-166
Gevers, D., Cohan, F. M., Lawrence, J. G., Spratt, B. G., Coenye, T., Feil, E. J., et al. (2005). Opinion: re-evaluating prokaryotic species. Nat. Rev. Microbiol. 3, 733–739. doi: 10.1038/nrmicro1236
Glickmann, E., and Dessaux, Y. (1995). A critical examination of the specificity of the Salkowski reagent for indolic compounds produced by phytopathogenic bacteria. Appl. Environ. Microbiol. 61, 793–796.
Gurevich, A., Saveliev, V., Vyahhi, N., and Tesler, G. (2013). QUAST: quality assessment tool for genome assemblies. Bioinformatics 29, 1072–1075. doi: 10.1093/bioinformatics/btt086
Hammer,Ø., Harper, D., and Ryan, P. (2001). PAST: paleontological statistics software package for education and data analysis. Paleontol. Electrón. 4, 1–9.
Holt, J. (2000). Bergey’s Manual of Determinative Bacteriology. Philadelphia, PA: Lippincott Williams & Wilkins.
Holt, J. (ed.). (1986). “Gram-positive bacteria other than Actinomycetes,” in Bergey’s Manual of Systematic Bacteriology, Vol. 2 (Baltimore, MD: Williams & Wilkins).
Hong, Y.-Y., Ma, Y.-C., Zhou, Y.-G., Gao, F., Liu, H.-C., and Chen, S.-F. (2009). Paenibacillus sonchi sp. nov., a nitrogen-fixing species isolated from the rhizosphere of Sonchus oleraceus. Int. J. Syst. Evol. Microbiol. 59, 2656–2661. doi: 10.1099/ijs.0.009308-0
Husemann, P., and Stoye, J. (2010). r2cat: synteny plots and comparative assembly. Bioinformatics 26, 570–571. doi: 10.1093/bioinformatics/btp690
Jin, H.-J., Zhou, Y.-G., Liu, H.-C., and Chen, S.-F. (2011). Paenibacillus jilunlii sp. nov., a nitrogen-fixing species isolated from the rhizosphere of Begonia semperflorens. Int. J. Syst. Evol. Microbiol. 61, 1350–1355. doi: 10.1099/ijs.0.025056-0
Junier, T., and Zdobnov, E. M. (2010). The Newick utilities: high-throughput phylogenetic tree processing in the UNIX shell. Bioinformatics 26, 1669–1670. doi: 10.1093/bioinformatics/btq243
Kerepesi, C., Bánky, D., and Grolmusz, V. (2014). AmphoraNet: the webserver implementation of the AMPHORA2 metagenomic workflow suite. Gene 533, 538–540. doi: 10.1016/j.gene.2013.10.015
Kim, M., Oh, H.-S., Park, S.-C., and Chun, J. (2014). Towards a taxonomic coherence between average nucleotide identity and 16S rRNA gene sequence similarity for species demarcation of prokaryotes. Int. J. Syst. Evol. Microbiol. 64, 346–351. doi: 10.1099/ijs.0.059774-0
Kumar, N., Lad, G., Giuntini, E., Kaye, M. E., Udomwong, P., Shamsani, N. J., et al. (2015). Bacterial genospecies that are not ecologically coherent: population genomics of Rhizobium leguminosarum. Open Biol. 5:140133. doi: 10.1098/rsob.140133
Liu, Z., Xu, X., Han, J., Gao, C., Wu, Z., and Hang, F. (2016). Paenibacillus bovis sp. nov., isolated from raw yak (Bos grunniens) milk. Int. J. Syst. Evol. Microbiol. 66, 1413–1418. doi: 10.1099/ijsem.0.000900
Logan, N. A., Berge, O., Bishop, A. H., Busse, H.-J., De Vos, P., Fritze, D., et al. (2009). Proposed minimal standards for describing new taxa of aerobic, endospore-forming bacteria. Int. J. Syst. Evol. Microbiol. 59, 2114–2121. doi: 10.1099/ijs.0.013649-0
Lugtenberg, B., and Kamilova, F. (2009). Plant-growth-promoting rhizobacteria. Annu. Rev. Microbiol. 63, 541–556. doi: 10.1146/annurev.micro.62.081307.162918
MacFaddin, J. (2000). Biochemical Tests for Identification of Medical Bacteria. Philadelphia, PA: Lippincott Williams and Wilkins.
Maughan, H., and Van der Auwera, G. (2011). Bacillus taxonomy in the genomic era finds phenotypes to be essential though often misleading. Infect. Genet. Evol. 11, 789–797. doi: 10.1016/j.meegid.2011.02.001
Meier-Kolthoff, J. P., Auch, A. F., Klenk, H.-P., and Göker, M. (2013a). Genome sequence-based species delimitation with confidence intervals and improved distance functions. BMC Bioinformatics 14:60. doi: 10.1186/1471-2105-14-60
Meier-Kolthoff, J. P., Göker, M., Spröer, C., and Klenk, H.-P. (2013b). When should a DDH experiment be mandatory in microbial taxonomy? Arch. Microbiol. 195, 413–418. doi: 10.1007/s00203-013-0888-4
Meier-Kolthoff, J. P., Hahnke, R. L., Petersen, J., Scheuner, C., Michael, V., Fiebig, A., et al. (2014a). Complete genome sequence of DSM 30083T, the type strain (U5/41T) of Escherichia coli, and a proposal for delineating subspecies in microbial taxonomy. Stand. Genomic Sci. 9:2. doi: 10.1186/1944-3277-9-2
Meier-Kolthoff, J. P., Klenk, H.-P., and Göker, M. (2014b). Taxonomic use of DNA G+C content and DNA-DNA hybridization in the genomic age. Int. J. Syst. Evol. Microbiol. 64, 352–356. doi: 10.1099/ijs.0.056994-0
Parks, D. H., Imelfort, M., Skennerton, C. T., Hugenholtz, P., and Tyson, G. W. (2014). CheckM: assessing the quality of microbial genomes recovered from isolates, single cells, and metagenomes. Genome Res. 25, 1043–1055. doi: 10.7287/peerj.preprints.554v1
Pruesse, E., Peplies, J., and Glöckner, F. O. (2012). SINA: accurate high-throughput multiple sequence alignment of ribosomal RNA genes. Bioinformatics 28, 1823–1829. doi: 10.1093/bioinformatics/bts252
Richter, M., and Rosselló-Móra, R. (2009). Shifting the genomic gold standard for the prokaryotic species definition. Proc. Natl. Acad. Sci. U.S.A. 106, 19126–19131. doi: 10.1073/pnas.0906412106
Rosselló-Móra, R. (2012). Towards a taxonomy of Bacteria and Archaea based on interactive and cumulative data repositories. Environ. Microbiol. 14, 318–334. doi: 10.1111/j.1462-2920.2011.02599.x
Schleifer, K. H. (2009). Classification of Bacteria and Archaea: past, present and future. Syst. Appl. Microbiol. 32, 533–542. doi: 10.1016/j.syapm.2009.09.002
Schloter, M., Lebuhn, M., Heulin, T., and Hartmann, A. (2000). Ecology and evolution of bacterial microdiversity. FEMS Microbiol. Rev. 24, 647–660. doi: 10.1016/S0168-6445(00)00051-6
Schwyn, B., and Neilands, J. B. (1987). Universal chemical assay for the detection and determination of siderophores. Anal. Biochem. 160, 47–56. doi: 10.1016/0003-2697(87)90612-9
Seldin, L. (2011). “Paenibacillus, nitrogen fixation and soil fertility,” in Endospore-forming Soil Bacteria Soil Biology, eds N. A. Logan and P. Vos (Berlin: Springer), 287–307. doi: 10.1007/978-3-642-19577-8_15
Smith, S. A., and Dunn, C. W. (2008). Phyutility: a phyloinformatics tool for trees, alignments and molecular data. Bioinformatics 24, 715–716. doi: 10.1093/bioinformatics/btm619
Stackebrandt, E., and Ebers, J. (2006). Taxonomic parameters revisited: tarnished gold standards. Microbiol. Today 33, 152–155.
Sutcliffe, I. C., Trujillo, M. E., and Goodfellow, M. (2012). A call to arms for systematists: revitalising the purpose and practises underpinning the description of novel microbial taxa. Antonie Van Leeuwenhoek 101, 13–20. doi: 10.1007/s10482-011-9664-0
Tamura, K., Stecher, G., Peterson, D., Filipski, A., and Kumar, S. (2013). MEGA6: molecular evolutionary genetics analysis version 6.0. Mol. Biol. Evol. 30, 2725–2729. doi: 10.1093/molbev/mst197
Varghese, N. J., Mukherjee, S., Ivanova, N., Konstantinidis, K. T., Mavrommatis, K., Kyrpides, N. C., et al. (2015). Microbial species delineation using whole genome sequences. Nucleic Acids Res. 43, 6761–6771. doi: 10.1093/nar/gkv657
Wu, M., and Eisen, J. A. (2008). A simple, fast, and accurate method of phylogenomic inference. Genome Biol. 9:R151. doi: 10.1186/gb-2008-9-10-r151
Keywords: Paenibacillus, Paenibacillus riograndensis, Paenibacillus sonchi, taxonomy, average nucleotide identity, dDDH, phylogeny
Citation: Sant’Anna FH, Ambrosini A, de Souza R, de Carvalho Fernandes G, Bach E, Balsanelli E, Baura V, Brito LF, Wendisch VF, de Oliveira Pedrosa F, de Souza EM and Passaglia LMP (2017) Reclassification of Paenibacillus riograndensis as a Genomovar of Paenibacillus sonchi: Genome-Based Metrics Improve Bacterial Taxonomic Classification. Front. Microbiol. 8:1849. doi: 10.3389/fmicb.2017.01849
Received: 31 May 2017; Accepted: 11 September 2017;
Published: 04 October 2017.
Edited by:
Vasco Ariston De Carvalho Azevedo, Universidade Federal de Minas Gerais, BrazilReviewed by:
Henrique César Pereira Figueiredo, Universidade Federal de Minas Gerais, BrazilAnton Hartmann, Helmholtz Zentrum München (HZ), Germany
Copyright © 2017 Sant’Anna, Ambrosini, de Souza, de Carvalho Fernandes, Bach, Balsanelli, Baura, Brito, Wendisch, de Oliveira Pedrosa, de Souza and Passaglia. This is an open-access article distributed under the terms of the Creative Commons Attribution License (CC BY). The use, distribution or reproduction in other forums is permitted, provided the original author(s) or licensor are credited and that the original publication in this journal is cited, in accordance with accepted academic practice. No use, distribution or reproduction is permitted which does not comply with these terms.
*Correspondence: Luciane M. P. Passaglia, bHVjaWFuZS5wYXNzYWdsaWFAdWZyZ3MuYnI=